DOI:
10.1039/C8MT00174J
(Perspective)
Metallomics, 2018,
10, 1359-1367
Cadmium exposure during pregnancy and lactation: materno-fetal and newborn repercussions of Cd(II), and Cd–metallothionein complexes
Received
2nd July 2018
, Accepted 3rd September 2018
First published on 17th September 2018
Abstract
Cadmium (Cd) is a non-physiological heavy metal that can be harmful at low concentrations. Increasing anthropogenic activities are incrementing the risk of accumulation of this heavy metal in different organs and tissues of the body. In the case of pregnant women, the threat is more serious due to the implications affecting not only their own health but also fetal development as well. Metallothioneins (MTs), small cysteine-rich proteins, are involved in zinc (Zn) and copper homeostasis in mammals but can, however, also bind with Cd if present. The accumulation of Cd in maternal tissues (e.g. placenta, maternal blood, and mammary glands) induces the synthesis of MTs, preferably MT2, in an attempt to sequester the metal to avoid toxicity. The formed Cd–MT complexes will avoid the Cd transport from the placenta to the fetus and end up accumulating in the maternal kidneys. At the same time, high concentrations of MTs will increase the formation of Zn–MT complexes, therefore decreasing the amount of Zn ions available to be transported to the fetus by means of Zn transporters such as ZnT2, ZIP14 and DMT1. Although MTs cannot transport Cd from the mother to the fetus, the divalent DMT1 transporter is suggested to carry the metal to the fetus. As a consequence, the low levels of Zn(II) in the fetus, together with the presence of Cd(II) coming from the mother either via the placenta and cord blood or via breast milk induce changes in the fetal development including fetal growth retardation, and low weight or height of the newborn. Likewise, the concentrations of Cd(II) in the newborn can cause alterations such as cognitive disabilities. In summary, the presence of Cd(II) in the maternal tissues will induce MT synthesis in an attempt to detoxify these tissues and reduce the possible toxicity of Cd in fetal and newborn tissues.
Significance to metallomics
Environmental health has been a growing concern in recent years. Understanding the molecular mechanisms involved in the alterations caused by pollutants is essential in order to act accordingly. Cadmium (Cd) is a toxic metal, whose presence may threaten the health of the mother and the fetus. The main points (topics) to be addressed are the following: where Cd(II) is mainly accumulated, where and why metallothionein (MT) synthesis is induced, how Cd is bound to MTs and what happens with Zn and Cd transport from the mother to the fetus. Knowledge of the main implications caused by the presence of Cd will allow us to establish future strategies at a different level.
|
1. Introduction
1.1. Cadmium exposure overview
Cadmium (Cd) is a heavy metal that is naturally present at low levels in the environment. Besides the natural sources of Cd production (i.e. mainly volcanic activity, erosion and river transport), anthropogenic activities have turned Cd into a serious pollutant in different settings, in recent decades. The most common sources of Cd pollution caused by humans are agricultural (manufacture of phosphate fertilizers), industrial (such as mining, smelting and refining of different metals, coating and plating and pigment manufacture) and urban-life related (including waste and recycling products containing Cd: batteries, electronic devices and plastic components).1–3
It is known that Cd is toxic to living beings as well as carcinogenic, according to the International Agency for Research on Cancer (IARC);4 however, only the ionized form – Cd(II) – is able to cause cellular damage. Unlike other heavy metals with similar chemical properties, in terms of protein binding, such as zinc (Zn) or copper (Cu),5 Cd does not participate in physiological functions in the human body, which means that Cd at any concentration can be harmful.6 In fact, the chemical similarities that Cd shares with different elements – Zn, iron (Fe) or calcium – mean that Cd can replace them in the prosthetic group of several proteins, altering not only the structure, but also some functions such as enzymatic reactions by means of the depletion of the thiol groups of antioxidants and enzymes.7,8 So, the main toxic effects induced by Cd include oxidative stress, apoptosis in embryonic cells or epigenetic changes, mainly by DNA methylation modifications.9–11
In humans, the main route of Cd exposure is by air, which is responsible for up to 50% of the total Cd incorporation into the organism. Specifically, tobacco is one of the major sources of Cd in active and passive smokers. Nevertheless, Cd can also enter, to a lesser extent, through contaminated food and drinking-water.1,2,12 As a direct consequence, the presence of Cd in the organism is associated with alterations in the respiratory tract, the gastrointestinal tract, and the cardiovascular, renal and nervous systems and it even affects bone health, being associated with osteoporosis and osteomalacia.13
To deal with the harmful effects that the presence of Cd can cause, organisms use specific mechanisms that besides their specific function can help to avoid the toxicity of Cd. Among them, metallothioneins (MTs) play a relevant role.
1.2. Basics of mammalian metallothioneins
MTs are a superfamily of ubiquitous, small to medium-sized (<30 kDa)14,15 cysteine-rich (15–30%) proteins with the ability to coordinate metal ions through metal–thiolate bonds and have been identified in all eukaryotes and some prokaryotes thus far analysed.16–18 The variety in the metal ion coordination that MTs show, reveal particularities in the three-dimensional metal–MT complexes. So, when the vertebrate MTs coordinate divalent metal ions (Zn(II) or Cd(II)), two domains (β and α) can be distinguished.19,20
The MT system comprises four extremely similar isoforms (MT1 to MT4) which are specific to mammals even if in humans MTs can differ into several subisoforms, as a result of amplifications through evolution.21 As many as nine subisoforms of MT1 have been described, while only one isoform of MT2, one of MT3 and one of MT4 have been characterized thus far. MT1 and MT2 are found in virtually all tissues. These are responsible for metal ion homeostasis and transport, as well as protection against metal toxicity and oxidative stress, either by protein overexpression and sequestration of toxic metals, or by the direct involvement of the free radical binding activity of MTs, and avoiding DNA, RNA and cell structure damage.22 Their expression is significantly high in the kidneys and the liver. Nevertheless, unlike the MT1 gene, MT2 exhibits a basal expression globally, except in the spleen where MT2 is not detectable. MT3 is mostly expressed in the brain, where it plays specific roles in the central nervous system, and finally, MT4 is expressed exclusively in the stratified squamous epithelia.23–28 All four MTs are synthesized in the maternal decidua in mice.29
Regarding the MT gene expression, they are commonly expressed at a basal level; nevertheless, the expression of the MT1/2 genes can increase in the presence of metal and non-metal agents, which interact with the transcription factors inducing MT synthesis.30,31 The induction of the mammalian MT1/2 genes is sensitive to several metals – including Cd and Zn – reactive oxygen species, glucocorticoids or cytokines. It is worth noting that to induce MTs at the same level, the concentration of Zn needs to be higher than the Cd concentration.32 Likewise, it has long been known that the MT2 gene preferably responds to Zn(II) and glucocorticoids, while MT1 does the same in the presence of Cd(II) and to a lesser extent, to hormonal agents. With regards to the MT3 gene, its induction is not influenced by these factors.33,34
At the same time, specific biological and environmental conditions in human life, such as pregnancy and lactation, ageing, smoking or pollution, may indirectly regulate the MT concentrations in the tissues and organs, to deal with specific changes that may occur in such conditions.9,35,36
1.3. Cd exposure during pregnancy and lactation
Cd reaches pregnant and nursing mothers mainly by smoking, intake of Cd-contaminated food or water and even as a consequence of the polluted environment.1–3 The physiological changes that occur during pregnancy, such as an increased respiratory rate or alterations in the gastrointestinal system in order to meet the nutritional needs during embryo/fetus development, facilitate the absorption of Cd.37,38 At the molecular level, its toxicity can reduce Zn transport from the mother to the fetus/newborn, induce MT gene expression in specific tissues32 or displace the Zn in the Zn–MT complexes to form Cd–MT complexes.39,40 These modifications alone or in combination threaten both good materno-fetal and newborn health.
As a result, specific tissues and fluids involved in pregnancy and lactation processes (e.g. placenta, cord blood, embryonic/fetal tissues, and mammary glands), can accumulate Cd as free or bound metal ions. Its presence has been negatively associated with alterations in the mother, the fetus and the newborn's health.41,42 Thus, studies conducted in rats have shown a significant association between the presence of Cd and problems getting pregnant, preeclampsia, kidney damage, alteration in essential element levels or lower bone mineral density, among other adverse effects.41 Likewise, there has been a direct correlation described between the presence of Cd in the urine of pregnant women and a reduction of the birth weight, height and cranial circumference of the newborn. Similarly, the exposure to Cd in the middle and late pregnancy increases the concentration of this metal in the blood of the pregnant women and is linked to fetal growth retardation (FGR)42–45 due to the functional and/or structural abnormalities induced by this metal.46 In addition, the toxic effects of Cd have been also detected at the molecular level, where characteristic DNA methylations have been identified.47,48
Regarding the lactation period, studies conducted in suckling rats fed with breast milk containing Cd, revealed brain alterations. These included changes in reflexes and physical maturation, delay in neuromotor development and hyperactivity.49
The aim of this work is to review what is known so far about the relationship between the Cd exposure of pregnant and nursing mothers in mammals, the presence of Cd(II) in specific tissues, organs or fluids, and the synthesis of MTs and their repercussions.
2. Metal selectivity of MTs
MTs can be classified depending on their origin and structure. However, this classification does not easily reflect the possible functions in which MTs are involved. According to Palacios et al., the identification of the metal-binding features contributes to characterizing the physiological functions of these metalloproteins. So, in vitro metal-replacement studies of MTs treated with Zn(II), Cd(II) or Cu(I) have allowed identification of genuine Zn–, Cd– or Cu–thioneins (i.e. formation of homometallic species) as well as MTs that bind a mixture of two metals (i.e. formation of heterometallic species). Thus, depending on the behaviour of the MTs forming well-structured species with a single metal, or low-structured species containing a mixture of metals (Zn(II)/Cd(II) or Cu(I)/Cd(II)), a gradation can be established according to the preference of the MTs for the metals. Likewise, the coordination environment induces the protein to establish a particular metal–MT folding which will result in a specific structure and function of this particular complex.5
Focusing on these features of mammalian MTs, MT1/MT2 are considered typical Zn–thioneins, whereas MT4 presents a strong Cu–thionein feature, and shows a weak ability to bind Zn or Cd.5,50 The analysis of the binding abilities of the mammalian MT1 and MT2 also revealed that they differ significantly from each other. Despite the fact they share a very close degree of coincidence in their metal binding behaviour. According to Artells et al., MT1 exhibits a typical metal binding Cd(II) detoxifying behaviour, whereas MT2 displays a closer Zn–thionein behaviour for Zn(II) handling and homeostasis.39 However, according to Vallee and Palmiter, it possibly cannot be considered that the Cd detoxification is a function gained through evolution, but a mere property that these proteins can take advantage of, when Cd is present.51,52
In particular, recombinant synthesis of MT1 and MT2, in the presence of Zn(II) or Cd(II), yields Zn7– and Cd7–MT1/2, respectively. More precisely, in vitro titration analysis of Zn7–MT1/2 with Cd(II) shows Zn/Cd replacement as a progressive, non-cooperative, incorporation of Cd(II) into the MT1 and MT2 complexes, passing from a homonuclear state of Zn7–MT to a heteronuclear state of ZnxCdy–MT and lastly obtaining a new homonuclear state with Cd7–MT complexes. Focusing on the β and α domains and due to the number of cysteines as well as their distribution in each domain, the α domain binds up to four Cd ions, whereas the β domain only has three in stable complexes.39,53 According to this, it is feasible to think of a situation in which the presence of Cd(II) will progressively replace Zn–MT complexes in the tissues where Cd(II) is accumulated until practically all the free ions will be bound to the MT complexes. However, there is much consensus following the idea that this will occur only in vitro with a fully enriched Cd medium, but not in vivo, where it seems that MTs form mostly partially metallated species under basal conditions. It may be expected that when the concentration of Cd exceeds the capacity of the free thiols to collect the metal, then Zn displacement will occur.
That said, if Cd(II) can escape from being bound, not only will it act as a toxic element on its own, but may also be mobilized to other tissues where the same situation would be repeated.
3. Materno-fetal transport of Zn and Cd
3.1. General considerations of Zn in mammals
Zn is an essential heavy metal in living beings taking part in different cellular processes. The main functions in which Zn is involved in proteins are: structural, regulatory and catalytic, among others.54 In addition, Zn in mammals is necessary for the successful functioning of the immune and nervous system and endocrine function.55 Under biological conditions, Zn does not undergo redox changes and as a consequence, it cannot be involved in electron transfer reactions, which is why the cellular toxicity of Zn is lower – but not negligible – than that of other physiological metals like Cu or Fe. Nonetheless, Zn can be toxic to many cell types, which is why the regulation of the bound ions is required to maintain appropriate cell conditions.56 To ensure a correct amount of this metal with the proteins that require it, the cells have developed a complex homeostatic system to mobilize, exchange or bind Zn ions. The system comprises Zn-transporters, low affinity ligands, metallochaperones and chelators such as MTs; the latter are considered some of the most significant Zn-binding proteins, which participate in intracellular homeostasis by binding Zn ions.57,58 Hence, the responsibility of the input and the output of Zn ions from cells involves different Zn transporters, including those from the ZIP, ZnT families or the divalent transporter DMT1.59,60
3.2. Zn deficiency during pregnancy and lactation
Zn is an indispensable metal during embryo/fetal development and in the first years of the newborn's life. Under normal conditions (i.e. in the absence of Cd or other situations that may affect the Zn homeostasis), the metal is transferred from the mother to the embryo/fetus through the placenta or to the newborn through breast milk.61 When the required Zn levels are not met due to gestational Zn deficiency, complications may occur in the embryo/fetus. These complications can range from alterations in Zn homeostasis, epigenetic changes in the fetal MTs to FGR, newborn delivery complications, low weight, low height or low cranial perimeter of the newborn.62,63 Likewise, early years of the postnatal stage are characterized by a period of a rapid growth and development, requiring high amounts of Zn. When the newborn is exclusively fed by breastfeeding, the provision of adequate Zn levels in the breast milk is essential in order to meet the newborn's needs. If these needs cannot be met, complications may arise, ranging from growth delay to cognitive impairments in humans.49
It has been demonstrated that Cd exposure affects the essential elements metabolism in which Zn homeostasis is included. Cd exposure reduces the bioavailability of Zn by means of changes in the normal expression of Zn transporters, altering the transfer of this metal from the maternal blood to the embryo/fetus or from the breast milk to the newborn.49,64 As a consequence, the required levels of Zn, in the embryo/fetus or newborn, may be insufficient to maintain suitable physiological functions, affecting their optimal health, as stated above.
3.3. Zn and Cd transport in pregnancy and lactation related tissues
3.3.1. Placenta.
Zn arrives in the placental syncytiotrophoblast, from the maternal blood as a result of ZIP proteins and then it is delivered to the cord blood, reaching the fetus, mainly by means of the ZnT2, ZIP14 and DMT1. Likewise, the transport of Zn to the fetus through the placenta can be influenced by different factors, among them: gestational age, Zn levels in the maternal blood or changes in Zn transporter synthesis.65 So, for example, in the case of low dietary Zn intake, the expression of the ZnT in this tissue may decrease, reducing the Zn transport to the fetus, in an attempt to avoid an imbalance in the maternal Zn homeostasis.66,67
Furthermore, the placenta is regarded as the principal tissue that acts as a natural barrier to avoid the transfer of harmful substances from the pregnant woman to the embryo or fetus. However, it is considered as a partial barrier, due to the ability of some molecules to cross it.68 Cd is one of them, as it is able to pass from the mother to the fetus, in addition to being accumulated in the placental tissue.42,43,69 Placental Cd concentrations in pregnant women are usually high despite some researchers detecting lower levels than others. This is probably due to specific conditions and measurement techniques.70,71 The pathway that allows Cd to cross the placenta and enter the fetus remains unclear, nevertheless studies performed in rats by Nakamura et al. suggest that DMT1 is the protein involved in the transport of Cd from the placenta to the fetus. On the other hand, it seems that the presence of placental MTs would contribute to avoiding Cd transfer, although for the time being, the mechanism remains not well understood. According to Nakamura et al., different levels of Cd in the placenta regulate the induction of the metal transporters and also the expression of MT genes, indicating that the whole mechanism of mobilisation and transport of Cd in the placenta depends on different metal-proteins including the MTs.59,72 Then, Wang et al. demonstrated also in rats, that in the presence of Cd the Zn transporters, ZnT1 and ZnT2 are down-regulated, thus decreasing the probability of Cd being transported to the fetus64 (Table 1). As a consequence, Zn transfer from the placenta to the fetus will also be modified, accumulating Zn in the placental tissues and decreasing its levels in the fetus.73,74 Therefore, the presence of Zn and Cd in the fetus is determined by a set of players, in which the concentration of maternal Zn and Cd seems to play the main role.
Table 1 Summary table of the repercussions of cadmium exposure, in different tissues and fluids and over metallothioneins, during pregnancy and lactation. Cd: cadmium; Zn: zinc; Cu: copper; MTs: metallothioneins
|
Cd(II) |
MTs |
Pregnancy |
Placenta and uterus |
• Transport to cord blood by means of DMT1.59,72 |
• Cd–MT complex formation.29,63,93–96,101,102 |
• Down-regulation of Zn transporters.64 |
• Induction of MT1 and upregulation of MT2.32 |
• Reduced transport of maternal Zn to fetus.73,74 |
• Significant expression of MT3 in uterus.72 |
Maternal blood |
• Down-regulation of Zn and Cu transporters64,72 |
• Higher concentrations of Cd in the case of MT2-5A/G heterozygosis vs. homozygosis.103,104 |
Cord blood |
• Lower concentrations than in maternal blood.44,46,59,82 |
• (Not enough significant data found) |
Fetus |
• Accumulation in the kidneys and liver.41,59 |
• Role of MT1 and MT2 unclear but it is suspected to be similar to those in adults.104,107,108 |
|
Lactation |
Mammary gland |
• Down-regulation of Zn exporters (ZnT2, ZnT4) and overexpression of Zn importers (ZIP3, ZIP4 and ZIP8).85 |
• Cd–MT complex formation.44 |
Breast milk |
• Presence of Cd(II) not totally retained by mammary glands.66,72,83 |
• Lower amounts of MTs than in Cd absence.111 |
• Cd(II) bound to intestinal MT.59 |
3.3.2. Maternal and cord blood.
Concentrations of Zn in the maternal blood seem to be different depending on the gestational trimester due to the requirements in the Zn homeostasis during fetal development.75,76 However, despite the variability existing in pregnant women, it is difficult to speak of an adequate amount of Zn in the maternal blood. In fact, there are several studies in the literature showing different concentrations of plasma Zn in pregnant women according to diverse variables: age, parity, pregnancy pathologies or even place of origin.77–80
Zn is transported from enterocytes into the maternal blood thanks to ZnT1 and from there it reaches and enters the placenta by means of ZIP proteins, as stated above.66 Once Zn is in the placenta, it can be bound to MTs or transported to the cord blood through Zn transporters which include ZIP14, ZnT2 and DMT1.59,72,81
In the case of Cd exposure, the presence of this toxic metal in the maternal plasma is negatively related with the Zn and Cu levels in the placenta due to down-regulation of Zn and Cu transporters, which therefore proves that the toxic metal modifies the concentration of the physiological ones.64,72 Similarly, studies analysing the presence of Cd in the maternal and cord blood of exposed pregnant women, find the concentrations of this metal to be at least one and a half times higher in the maternal blood rather than the cord blood,46 corroborating the low rate of Cd transfer from the mother to the fetus via the placenta, most likely as a result of the MT role, avoiding its transport.44,59,82 However, Cd reaches the cord blood and subsequently, the fetus.
3.3.3. Fetus.
Zn reaches the fetus via the cord blood. If the concentration of Zn in the placenta is not adequate or there is no bioavailability of the metal because it is bound to other molecules, its transfer through the cord blood can be altered. This will result in alterations of fetal homeostasis of Zn, FGR or changes in anthropometric parameters of the newborn with lower than expected values, among other changes.60,79
When Cd is present in the placenta, it reaches the fetus, although at low concentrations.72 Studies conducted by Nakamura et al., revealed that rats on the first day of life, present higher concentrations of Cd in their kidneys and liver, indicating that in the fetal life, Cd has been present at least in the last intrauterine period. Evenly, the Cd levels in these organs will depend on its concentration in the mother.59 Likewise, the presence of Cd during fetal development is related with a long list of alterations which include modifications in the central nervous system, kidney or liver development, imbalance in the homeostasis of essential elements or FGR83 (for more detailed information, please refer to ref. 41).
3.3.4. Lactation (mammary glands and breast milk) and the newborn.
In the same way as the placenta, the mammary glands act as a barrier between the maternal blood and the breast milk. In fact, it seems that the mammary glands can activate or inhibit the transport of essential elements. Since breast milk has to meet all nutritional needs of the newborn, there is an intense transport of essential elements, and Zn is among them.84
According to Rossipal et al., the concentration of Zn in the human colostrum can be higher than in the maternal blood. However, despite the modulation of essential elements carried out by this gland, it seems that the Cd transport is not completely inhibited and Cd has been found in breast milk.66,72,83 At the same time, the presence of Cd in the mammary glands causes a down-regulation of ZnT2 and ZnT4 and an overexpression of ZIP3, ZIP4 and ZIP8, which indicates that the Zn-transporters play a significant role in the Zn transport and Cd retention in the mammary glands85 (Table 1).
In turn, the formation of specific Cd–MT complexes in this tissue has also been described,86 these formations would reduce the transfer of this toxic metal from the breast milk to the newborn, and thus diminish possible damage. So, one might affirm that the changes in the expression of ZnT and ZIP transporter families, together with the up-regulation of MTs in the mammary glands respond to a dual need: on one hand, binding essential metals that need to be mobilized from milk to the breastfed infant in order to meet the higher requirements during breastfeeding;87 and on the other hand, minimising the transport of toxic metals, such as Cd, from milk to the newborn through its retention in the tissue.44
Furthermore, recent studies also demonstrated that low concentrations of Cd contained in the breast milk and transferred to the newborn are able to reach their digestive system where it will bind to the intestinal MTs.59 Similarly, studies performed on lactating rats show that in early postnatal days, the presence of Cd in the breast milk also induces the down-regulation of ZnT and the overexpression of ZIP protein families in the intestines of the newborn, as occurred in the mammary gland,44 corroborating the same role that these proteins play in the mother exposed to Cd.
4. Role of MTs in materno-fetal transport of Zn and Cd
Even though this mini-review aims to explore the role of Cd(II) and MTs in materno-fetal tissues in Cd exposure, it is worth noting that other organs also experience changes as a consequence of the presence of Cd, this being the case for the liver and kidneys. So, specifically in the case of MTs at this stage, Cd accumulates from the highest to the lowest concentrations in the liver, serum, kidneys and placenta. In particular, the maternal liver Cd induces MT1 and MT2 mRNA, which suggests a crucial role of MT1 and MT2 binding Cd in this organ and thus avoiding its presence in the placenta.64 Similarly, it has been described that Cd in the liver may be mobilized as a Cd–MT complex to other organs like the kidneys.88
When the MT concentrations increase in the maternal serum during pregnancy, the Cd–MT complexes formed accumulate in the kidneys.88 Since the complexes are more nephrotoxic than Cd alone, the complications caused by the presence of Cd–MT complexes in these organs may become irreversible89 (Table 1).
The role of MTs in the materno-fetal organs and tissues related to Zn and Cd transport are detailed below.
4.1. Placenta and uterus
In human placental structures, MTs have been identified in the decidua, the syncytiotrophoblasts and the amniotic cells, suggesting that MTs play an important role in the metal (i.e. Zn, Cu, and Cd) transport from mother to fetus.90 Therefore, it is well known that MT1 and MT2 bind Zn(II) and the Zn–MT complexes formed participate in the transport of Zn from mother to fetus.91
In Cd exposure, it has been demonstrated that MT concentrations in the trophoblast cells are higher than in other placental regions, which indicates that Cd accumulation in the trophoblastic structures carries an increased risk for fetal development, and Cd needs to be bound to avoid cell apoptosis.9,92 In fact, it has long been known that the presence of Cd(II) induces the expression of MT1 and MT2 genes in the uterine and placental tissues in a dose-dependent relation.32 Likewise, it has been described that nearly all free Cd ions are sequestered by proteins in the placenta, therefore MT1 and MT2 are responsible for binding the metal. So, the presence of Cd displaces the Zn during the metal–MT complex formation, due to the higher affinity of MTs for Cd than for Zn, thus reducing the toxicity of Cd(II) in the placenta.23,69,93–96 However, for the time being, the mechanism by which MT1 and MT2 avoid Cd transfer but deliver essential metals such as Zn to the embryo and fetus59 remains unclear.
The protective role that MT1 and MT2 play against the Cd toxicity has been established. Moreover, the ratio of MT1/MT2 in the placenta changes due to the presence of Cd, MT2 being up-regulated against MT1. This makes the concentrations of MT1 and MT2 more similar, which could suggest that either the up-regulation of MT2 happens to ensure adequate Zn handling in the presence of Cd–MT1 complexes, or alternatively, it would presuppose a more important role of MT2 in the detoxification of Cd.64,72,97 If the latter is true, it would be in contrast to what is known so far about the behaviour of MT2, considering that its role is more close to Zn homeostasis, in contrast to MT1 which plays a more important role in Cd detoxification.39
Despite the up-regulation of the MT2 gene compared to MT1, Adebambo et al. demonstrated in placental JEG-3 cells, that when Cd exposure occurs together with arsenic (iAs), the general expression of MTs and in particular of the MT1A, MT1F and MT1G sub-isoforms is larger than in the presence of Cd alone.98 Since MT expression depends on the induction of the metal regulatory transcription factor (MTF1), which once activated binds the metal response elements (MRE),99,100 it is suggested that the induction of MTF1 by the synergy between Cd and iAs allows expression of more MTs than Cd by itself.98 Since the induction of MTF1 is given by stimulation of one or more kinases, one may hypothesize that the number of stimulated kinases is larger in the presence of Cd and iAs, than in the case of Cd alone.99
As a consequence of the upregulation of MTs by the presence of Cd, they will form not only Cd–MT complexes, but also more Zn–MT than in physiological conditions (Fig. 1). This will decrease the availability of Zn in the placenta to be transferred to the embryo/fetus, therefore explaining in part, the low concentrations of Zn in the cord blood, which contributes to low fetal weight, as a result of the deficiency of this element.101,102
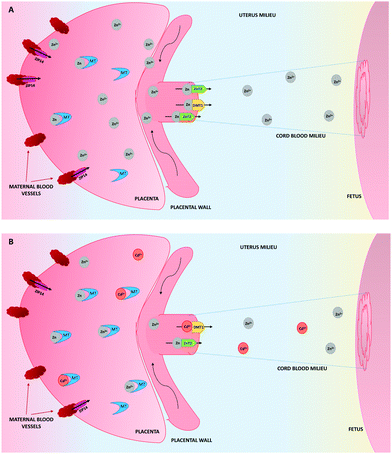 |
| Fig. 1 Zinc (Zn) transport from the placenta to the cord blood, in the absence (A) or presence (B) of cadmium (Cd). (A) Zn is imported to the placenta thanks to ZIP14. Once inside the placenta, Zn can be bound to metallothioneins (MTs) or transported to the fetus by means of metal-transporters (ZnT2 and DMT1). (B) The presence of Cd upregulates the synthesis of the MT genes, which implies an increase in the formation of Zn–MT and Cd–MT complexes in the placenta. At the same time, the transport of Zn to the fetus decreases, due to its low availability and this also decreases the number of Zn transporters. The Cd transport at low doses to the fetus is mediated by DMT1. | |
By contrast, although the synthesis of MT3 is not Cd-dependent, it is significantly expressed in the uterus, even though the ratio of MT3 concentrations to those of MT1 and MT2, suggests that MT3 does not play a significant role in Cd detoxification in this tissue72 (Table 1).
4.2. Maternal and cord blood
Several studies conducted in humans, analysed the maternal and the cord blood of pregnant women exposed to Cd. They showed that MT levels in the maternal plasma increase on Cd exposure. However, there exist significant differences between pregnant women depending on their MT2-5A/G single nucleotide polymorphism. Thus, those mothers presenting the MT2-heterozygote genotype have higher Cd concentration in maternal blood, while the levels are lower in those with MT2-homozygosis.103,104
Concerning the cord blood of pregnant smokers, whose Cd levels are higher than those of non-smokers, the concentration in the cord blood is positively correlated with the increase in cord jelly, whereas it is negatively associated with the arterial and the umbilical cord diameters (Table 1). On the other hand, it has also been demonstrated that in pregnancies diagnosed with FGR, the MT levels in the cord blood are higher than in those diagnosed with normal fetal growth.105 Likewise, a negative relation between the Cd levels of heterozygote MT2 gene and the Zn levels in cord blood103 has been established.
Meanwhile, comparing what happens in a non Cd-exposure pregnancy where MTs in the cord blood are low, regarding the Cd-exposure, no significant data are available to establish a clear association between Cd-exposure and the increase or decrease of MT concentrations in the cord blood, which could indicate a possible dysregulation of MT function at this level.106
4.3. Fetus
The role of MT1 and MT2 in the fetus remains unclear. However, it is known that overexpression of these MTs, and more precisely MT2, in the placenta, protects against FGR induced by Cd.70 At the same time, the placental MT synthesis allows the formation of Cd–MT complexes, thus reducing Cd transport to the fetus.59,72
Despite the low levels of Cd detected in the fetus, MT concentrations in the fetal liver are important.104 Likewise, it has also been established that there is a comparable expression of fetal MTs in the liver relative to those in the kidneys, because of the similarity in the Cd concentrations found in both organs.107 Moreover, the analogy of the pattern of MT synthesis between the fetus and adult mammals, suggests the same distribution of Cd in the organism.108 Compiling all this information seems to indicate that although Cd reaches the fetus at low concentrations, fetal MTs behave in a comparable manner to those of the adult, thus contributing to the binding of Cd by MTs and as a consequence, would reduce the toxicity of the metal during the development and growth process to the maximum.
4.4. Lactation (mammary glands and breast milk) and newborns
Studies performed in rats showed that during the lactation period, the synthesis of MTs increases in the kidneys, liver and duodenum of the breastfeeding mother, and decreases a few days after weaning.109 This rise in MT concentration is described as a physiological process, since it does not depend on the presence of toxic heavy metals. This determines the relevant role of MTs in binding heavy metals, including low levels of Cd from dietary intake on the mother's side.110 Furthermore, formation of Cd–MT complexes has also been established in the mammary glands. Evenly, comparing non-smokers with smokers, lower levels of MTs in the breast milk of smoking mothers have been detected. This would seem to be beneficial to the newborn, since the Cd–MT complex has proven to be toxic.111,112
In addition, studies conducted in rats by Mimouna et al., revealed an association between the presence of Cd in the newborn brain during lactation, a significant increase of MT synthesis in this tissue and its negative correlation with the brain development113,114 (Table 1). In addition, it has also been demonstrated that increased MT levels in the liver of lactating dams corroborate the possible role of MTs retaining Cd to avoid toxicity.44
According to this, and knowing that Cd–MT complexes cause toxicity in kidneys,44 future studies should determine the role of free Cd ions, as well as Cd–MT complexes in the brain, and also in the central nervous system, kidneys, liver and bones of the breastfed infant.
5. Conclusions
The exponentially rising presence of Cd is a threat for the general population, and in the case of pregnancy and lactation it poses a greater risk not only for the mother but also for the fetus and the newborn. Cd can induce and regulate the synthesis of a set of proteins in the tissues or organs where it is present among which are included transporters like ZnT, ZIP and DMT1 proteins, as well as metal-binding proteins like MTs. Similarly, the synthesis of MT genes in the placenta is induced as a consequence of exposure to Cd, which plays an important role in avoiding the transfer of the toxic metal to the fetus. However, the formation of Cd–MT complexes does not completely prevent this transfer, because some Cd(II) ions can cross the placenta with the help of the DMT1 divalent metal transporter. As an indirect effect, the high concentrations of MTs facilitate the binding of Zn, reducing the availability of this metal to be transferred through the placenta and cord blood. In conclusion, the Cd exposure during pregnancy results in a combination of factors, which will cause changes in the Zn availability that will eventually affect the mother and the embryo/fetus or the newborn to a greater or lesser extent.
Conflicts of interest
There is nothing to declare.
Acknowledgements
Anna Espart would like to thank Dr Sílvia Atrian for all the knowledge and instruction received during her PhD training. Sebastián Artime also would like to thank all the knowledge received from Dr Sílvia Atrian. Anna Espart and Edinson Yara-Varón would also like to thank Nicolau Yara and Gael Yara for their patience during the preparation of this review.
References
-
ATSDR, Toxicological profile for cadmium, Agency for toxics Substances and Disease Registry, Atlanta, 2012 Search PubMed
.
-
UNEP, Chemicals Branch, DTIE, Final review of scientific information on cadmium, United Nations Environment Programme, Geneva, 2010 Search PubMed
.
-
WHO, Exposure to cadmium: a major public health concern, World Health Organization, Geneva, 2010 Search PubMed
.
-
IARC, Cadmium and cadmium compounds, International Agency for Cancer Research, Geneva, 2012 Search PubMed
.
- O. Palacios, S. Atrian and M. Capdevila, J. Biol. Inorg. Chem., 2011, 16, 991–1009 CrossRef PubMed
.
- L. Järup, Br. Med. Bull., 2003, 68, 167–182 CrossRef
.
- M. Fagioni, G. M. D'Amici, A. M. Timperio and L. Zolla, J. Proteome Res., 2009, 8, 310–326 CrossRef PubMed
.
- E. Galano, A. Arciello, R. Piccoli, D. M. Monti and A. Amoresano, Metallomics, 2014, 6, 587–597 RSC
.
- M. F. McAleer and R. S. Tuan, In Vitro Mol. Toxicol., 2001, 14, 25–42 CrossRef PubMed
.
- M. F. McAleer and R. S. Tuan, In Vitro Mol. Toxicol., 2001, 14, 219–231 CrossRef PubMed
.
- F. Thévenod and W. K. Lee, Met. Ions Life Sci., 2013, 11, 415–490 Search PubMed
.
- W. Lee, S. Lee, J. Roh, J. U. Won and J. H. Yoon, J. Korean Med. Sci., 2017, 32, 568–575 CrossRef PubMed
.
- G. F. Nordberg, Toxicol. Appl. Pharmacol., 2009, 238, 192–200 CrossRef PubMed
.
- C. Ding, J. Yin, E. M. M. Tovar, D. A. Fitzpatrick, D. G. Higgins and D. J. Thiele, Mol. Microbiol., 2011, 81, 1560–1576 CrossRef PubMed
.
- P. Iturbe-Espinoza, S. Gil-Moreno, W. Lin, S. Calatayud, O. Palacios, M. Capdevila and S. Atrian, PLoS One, 2016, 16, e0148651 CrossRef PubMed
.
- J. H. R. Kägi and B. L. Vallee, J. Biol. Chem., 1960, 235, 3460–3465 Search PubMed
.
- M. Capdevila and S. Atrian, J. Biol. Inorg. Chem., 2011, 16, 977–989 CrossRef PubMed
.
- C. A. Blindauer and O. I. Leszczyszyn, Nat. Prod. Rep., 2010, 27, 720–741 RSC
.
- M. Vasák, J. Trace Elem. Med. Biol., 2005, 19, 13–17 CrossRef PubMed
.
- N. Romero-Isart and M. Vasák, J. Inorg. Biochem., 2002, 88, 388–396 CrossRef PubMed
.
- M. Vasák, J. Trace Elem. Med. Biol., 2005, 19, 13–17 CrossRef PubMed
.
- A. Moleirinho, J. Carneiro, R. Matthiensen, R. M. Silva, A. Amorim and L. Azevedo, PLoS One, 2011, e18487 CrossRef PubMed
.
-
C. A. Blindauer, Metallothioneins, in Binding, Transport and Storage of Metal Ions in Biological Cells, ed. M. Marett, A. Wedd, Royal Society of Chemistry, London, 2014, ch. 21 Search PubMed
.
- M. P. Waalkes and C. D. Klaassen, Fundam. Appl. Toxicol., 1985, 5, 473–477 CrossRef PubMed
.
- C. D. Klaassen and L. D. Lehman-McKeeman, Biol. Trace Elem. Res., 1989, 21, 119–129 CrossRef PubMed
.
- M. K. Yagle and R. D. Palmiter, Mol. Cell. Biol., 1985, 5, 291–294 CrossRef PubMed
.
- R. D. Palmiter, S. D. Findley, T. E. Whitmore and D. M. Durnam, Proc. Natl. Acad. Sci. U. S. A., 1992, 89, 6333–6633 CrossRef
.
-
P. Dziegiel, B. Pula, C. Kobierzycki, M. Stasiolek and M. Podhorska-Okolow, Metallothionein-3, in Metallothioneins in
Normal and Cancer Cells, ed. P. Dzięgiel, 2016, pp. 21–27 Search PubMed
.
- L. Liang, K. Fu, D. K. Lee, R. J. Sobieski, T. Dalton and G. K. Andrews, Mol. Reprod. Dev., 1996, 43, 25–37 CrossRef PubMed
.
- G. K. Andrews, Biochem. Pharmacol., 2000, 59, 95–104 CrossRef PubMed
.
- F. Haq, M. Mahoney and J. Koropatnick, Mutat. Res., 2003, 533, 211–226 CrossRef PubMed
.
- L. D. Lehman and A. M. Poisner, J. Toxicol. Environ. Health, 1984, 14, 419–432 CrossRef PubMed
.
- E. Tokuda, S. I. Ono, K. Ishige, A. Naganumad, Y. Ito and T. Suzuki, Toxicology, 2007, 229, 33–34 CrossRef PubMed
.
- S. H. Garrett, V. Phillips, S. Somji, M. A. Sens, R. Dutta, S. Park, D. Kim and D. A. Sens, Toxicol. Lett., 2002, 126, 69–80 CrossRef PubMed
.
- J. P. Bourdineadu, M. Baudrimont, P. González and J. L. Moureau, Biochimie, 2006, 88, 1787–1792 CrossRef PubMed
, (review).
- D. J. Mazzatti, M. Malavolta, A. J. White, L. Costarelli, R. Giacconi, E. Muti, C. Cipriano, J. R. Powell and E. Mocchegiani, Exp. Gerontol., 2008, 43, 423–432 CrossRef PubMed
.
- S. Astbury, A. Mostyn, M. E. Symonds and R. C. Bell, Appl. Physiol., Nutr., Metab., 2015, 40, 1100–1106 CrossRef PubMed
.
- J. Moya, L. Phillips, J. Sanford, M. Wooton, A. Gregg and L. Schuda, J. Exposure Sci. Environ. Epidemiol., 2014, 24, 449–458 CrossRef PubMed
.
- E. Artells, O. Palacios, M. Capdevila and S. Atrian, Metallomics, 2013, 5, 1397–1410 RSC
.
- A. E. Funk, F. A. Day and F. O. Brady, Comp. Biochem. Physiol., 1987, 86, 1–6 CrossRef
.
- T. Jacobo-Estrada, M. Santoyo-Sánchez, F. Thévenod and O. Barbier, Int. J. Mol. Sci., 2017, 18, E1590 CrossRef PubMed
, (review).
- J. L. Blum, J. R. Edwards, W. C. Prozialeck, J. Q. Xiong and J. T. Zelikoff, J. Toxicol. Environ. Health, 2015, 78, 711–724 CrossRef PubMed
.
- M. Kippler, F. Tofail, R. Gardner, A. Rahman, J. D. Hamadani, M. Bottai and M. Vahter, Environ. Health Perspect., 2012, 120, 284–289 CrossRef PubMed
.
- Y. L. Zhang, Y. C. Zhao, J. X. Wang, H. D. Zhu, Q. F. Liu, Y. G. Fan and T. Q. Fan, J. Environ. Sci. Health, Part A: Toxic/Hazard. Subst. Environ. Eng., 2004, 39, 2507–2515 CrossRef
.
- M. Menai, B. Heude, R. Slama, A. Forhan, J. Sahuquillo, M. A. Charles and C. Yazbeck, Reprod. Toxicol., 2012, 34, 622–627 CrossRef PubMed
.
- C. P. Sibley, M. A. Turner, I. Cetin, P. Ayuk, C. A. Boyd, S. W. D'Souza, J. D. Glazier, S. L. Greenwood, T. Jansson and T. Powell, Pediatr. Res., 2005, 58, 827–832 CrossRef PubMed
.
- A. P. Sanders, L. Smeester, D. Rojas, T. DeBussycher, M. C. Wu, F. A. Wright, Y. H. Zhou, J. E. Laine, J. E. Rager, G. K. Swamy, A. Ashley-Koch, M. L. Miranda and R. C. Fry, Epigenetics, 2014, 9, 212–221 CrossRef PubMed
.
- P. D. Ray, A. Yosim and R. C. Fry, Front. Genet., 2014, 5, 1–26 Search PubMed
.
- S. B. Mimouna, M. Chemek, S. Boughammoura, M. Banni and I. Messaoudi, Biol. Trace Elem. Res., 2018, 184, 409–421 CrossRef PubMed
.
- L. Tió, L. Villarreal, S. Atrian and M. Capdevila, J. Biol. Chem., 2004, 279, 24403–24413 CrossRef PubMed
.
-
B. L. Vallee, Implications and Inferences of Metallothionein Structure, in Metallothionein II. Experientia Supplementum, ed. Kägi J. H. R. and Kojima Y., Birkhäuser, Basel, 1987, vol. 52 Search PubMed
.
- R. D. Palmiter, Proc. Natl. Acad. Sci. U. S. A., 1998, 95, 8428–8430 CrossRef
.
- K. E. Rigby Duncan, D. W. Kirby and M. J. Stillman, FEBS J., 2008, 275, 2227–2239 CrossRef PubMed
.
- B. L. Vallee and K. H. Falchuk, Physiol. Rev., 1993, 73, 79–118 CrossRef PubMed
.
- J. C. King, Am. J. Clin. Nutr., 2011, 94, 679S–684S CrossRef PubMed
.
- R. A. Bozym, F. Chimienti, L. J. Giblin, G. W. Gross, I. Korichneva, Y. Li, S. Libert, W. Maret, M. Parviz, C. J. Frederickson and R. B. Thompson, Exp. Biol. Med., 2010, 235, 741–750 CrossRef PubMed
.
- J. P. Liuzzi and R. J. Cousins, Annu. Rev. Nutr., 2004, 24, 151–172 CrossRef PubMed
.
- S. A. Sinclair and U. Krämer, Biochim. Biophys. Acta, 2012, 1823, 1553–1567 CrossRef PubMed
.
- Y. Nakamura, K. Ohba, K. Suzuki and H. Ohta, J. Toxicol. Sci., 2012, 37, 149–156 CrossRef PubMed
.
- T. M. Leazer, Y. Liu and C. D. Klaassen, Toxicol. Appl. Pharmacol., 2002, 185, 18–24 CrossRef PubMed
.
- N. Wellinghausen, Br. J. Nutr., 2001, 85, S81–S86 CrossRef PubMed
.
- C. L. Fischer Walker, M. Ezzati and R. E. Black, Eur. J. Clin. Nutr., 2009, 63, 591–597 CrossRef PubMed
.
- H. Kurita, S. Ohsako, S. Hashimoto, J. Yoshinaga and C. Tohyama, J. Nutr. Biochem., 2013, 24, 256–266 CrossRef PubMed
.
- H. Wang, Y. Wang, Q. L. Bo, Y. L. Ji, L. Liu, Y. F. Hu, Y. H. Chen, J. Zhang, L. L. Zhao and D. X. Xu, Reprod. Toxicol., 2016, 63, 174–182 CrossRef PubMed
.
- P. G. Paterson, A. Mas, A. Sarkar and S. H. Zlotkin, J. Nutr., 1991, 121, 338–344 CrossRef PubMed
.
- R. J. Cousins, J. P. Liuzzi and L. A. Lichten, J. Biol. Chem., 2006, 281, 24085–24089 CrossRef PubMed
, (review).
- D. Ford, Proc. Nutr. Soc., 2004, 63, 21–29 CrossRef PubMed
.
- C. Gundacker and M. Hengstschläger, Wien. Med. Wochenschr., 2012, 162, 201 CrossRef PubMed
.
- G. V. Iyengar and A. Rapp, Sci. Total Environ, 2001, 280, 221–238 CrossRef PubMed
.
- J. Selvaratnam, H. Guan, J. Koropatnick and K. Yang, Am. J. Physiol.: Endocrinol. Metab., 2013, 305, 727–735 CrossRef PubMed
.
- M. Piasek, M. Blanusa, K. Kostial and J. Laskey, Reprod. Toxicol., 2001, 15, 673–681 CrossRef PubMed
.
- Y. Nakamura, K. Ohba, K. Suzuki and H. Ohta, J. Toxicol. Sci., 2012, 37, 1035–1044 CrossRef PubMed
.
- P. J. Wier, R. K. Miller and P. A. DiSant’Angese, Toxicol. Appl. Pharmacol., 1990, 105, 156–171 CrossRef PubMed
.
- A. Torreblanca, J. Del Ramo and B. Sarkar, Toxicology, 1992, 72, 167–174 CrossRef PubMed
.
- R. M. Helston, S. R. Phillips, J. A. McKay, K. A. Jackson, J. C. Mathers and D. Ford, Placenta, 2007, 28, 437–444 CrossRef PubMed
.
- S. Izquierdo-Álvarez, S. García-Castañón, M. L. Calvo-Ruata, E. Fuertes-Aragüés, P. Bocos-Terraz, Y. González-Irazabal, E. García-González and B. García-Rodríguez, J. Trace Elem. Med. Biol., 2007, 21, 49–52 CrossRef PubMed
.
- M. Lima de Moraes, R. De Faria-Barbosa, R. Espírito-Santo, F. da Silva-Santos, E. F. Oliveira de Jesus, F. L. de Carvalho-Sardinha and M. G. Tavares do Carmon, Biol. Trace Elem. Res., 2011, 139, 126–136 CrossRef PubMed
.
- M. L. Jobarteh, H. J. McArdle, G. Holtrop, E. A. Sise, A. M. Prentice and S. E. Moore, J. Nutr., 2017, 147, 401–1409 CrossRef PubMed
.
- A. L. Youssof, N. L. Kassim, S. A. Rashid, M. De Ley and M. T. Rahman, Biol. Trace Elem. Res., 2017, 175, 17–26 CrossRef PubMed
.
- O. Katz, O. Paz-Tal, T. Lazer, B. Aricha-Tamir, M. Mazor, A. Wiznitzer and E. Sheiner, J. Matern.-Fetal Neonat. Med., 2012, 25, 1127–1130 CrossRef PubMed
.
- C. J. Ferguson, M. Wareing, D. T. Ward, R. Green, C. P. Smith and D. Riccardi, Am. J. Physiol. Renal. Physiol., 2001, 280, 803–814 CrossRef PubMed
.
- E. E. Brako, A. K. Wilson, M. M. Jonah, C. A. Blum, E. A. Cerny, K. L. Williams and M. H. Bhattacharyya, Toxicol. Sci, 2003, 71, 154–163 CrossRef PubMed
.
- F. Salvatori, C. B. Talassi, S. A. Salzgeber, H. S. Spinosa and M. M. Bernardi, Neurotoxicol. Teratol., 2004, 26, 673–680 CrossRef PubMed
.
- E. Rossipal, M. Krachler, F. Li and D. Micetic-Turk, Acta Paediatr., 2000, 89, 1190–1195 CrossRef PubMed
.
- M. Chemek, S. Boughammoura, S. B. Mimouna, L. Chouchene, M. Banni and I. Messaoudi, Biol. Trace Elem. Res., 2015, 165, 173–182 CrossRef PubMed
.
- K. Peterrson Grawé and A. Oskarsson, Arch. Toxicol., 2000, 73, 519–527 CrossRef
.
- N. H. McCormick, J. King, N. Krebs, D. I. Soybel and S. L. Kelleher, J. Trace Elem. Med. Biol., 2015, 29, 170–175 CrossRef PubMed
.
- H. M. Chan, Y. Tamura, M. G. Cherian and R. A. Goyer, Proc. Soc. Exp. Biol. Med., 1993, 202, 420–427 CrossRef PubMed
.
- M. G. Cherian, In Vitro Cell. Dev. Biol., 1985, 21, 505–508 CrossRef
.
- R. A. Goyer, M. D. Haust and M. G. Cherian, Placenta, 1992, 13, 349–355 CrossRef PubMed
.
- L. C. Carey, P. Coyle, J. C. Philcox and A. M. Rofe, Alcohol.: Clin. Exp.
Res., 2000, 24, 1236–1240 CrossRef
.
- J. G. Breen, C. Eisenmann, S. Horowitz and R. K. Miller, Reprod. Toxicol., 1994, 8, 297–306 CrossRef PubMed
.
- S. K. De, M. T. McMaster, S. K. Dey and G. K. Andrews, Development, 1989, 107, 611–621 Search PubMed
.
- K. B. Nielson, C. L. Atkin and D. R. Winge, J. Biol. Chem., 1985, 260, 5342–5350 Search PubMed
.
- M. Nordberg and G. F. Nordberg, Cell. Mol. Biol., 2000, 46, 451–463 Search PubMed
.
- Y. Fukase, H. Tsugami, Y. Nakamura, K. Ohba and H. Ohta, Yakugaku Zasshi, 2014, 134, 801–804 CrossRef
.
- W. Y. Boadi, S. Yannai, J. Urbach, J. M. Brandes and K. H. Summer, Arch. Toxicol., 1991, 65, 318–323 CrossRef PubMed
.
- O. A. Adebambo, P. D. Ray, D. Shea and R. C. Fry, Toxicol. Appl. Pharmacol., 2015, 289, 534–541 CrossRef PubMed
.
- O. LaRochelle, V. Gagne, J. Charron, J. W. Soh and C. Séguin, J. Biol. Chem., 2001, 276, 41879–41888 CrossRef PubMed
.
- M. Waisberg, P. Joseph, B. Hale and D. Beyersmann, Toxicology, 2003, 192, 95–117 CrossRef PubMed
.
- A. M. Ronco, G. Arguello, M. Suazo and M. N. Llanos, Toxicology, 2005, 208, 133–139 CrossRef PubMed
.
- A. M. Ronco, G. Arguello, L. Muñoz, N. Gras and M. N. Llanos, Biometals, 2005, 18, 233–241 CrossRef PubMed
.
- D. Tekin, Z. Kayaalt, V. Aliyev and T. Söylemezoğlu, J. Appl. Toxicol., 2012, 32, 270–275 CrossRef PubMed
.
- A. Sekovanić, J. Jurasović, M. Piasek, D. Pašalić, T. Orct, A. S. Grgec, S. Stasenko, K. B. Čakanić and A. Jazbec, J. Trace Elem. Med. Biol., 2018, 45, 163–170 CrossRef PubMed
.
-
E. Milnerowicz-Nabzdyka and A. Bizoń, Reprod. Toxicol., 2014, 50, 27–35 Search PubMed
.
-
E. Milnerowicz-Nabzdyka and A. Bizoń, Reprod. Toxicol., 2015, 58, 79–84 Search PubMed
.
- G. Jacquillet, O. Barbier, I. Rubera, M. Tauc, A. Borderie, M. C. Namorado, D. Martin, G. Sierra, J. L. Reyes, P. Poujeol, M. Cougnon and Am. J. Physiol, Ren. Physiol., 2007, 293, F1450–F1460 CrossRef PubMed
.
- M. Webb and K. Cain, Biochem. Pharmacol., 1982, 31, 137–142 CrossRef PubMed
.
- K. Patersson Grawé and A. Oskarsson, Arch. Toxicol., 2000, 73, 519–527 CrossRef
.
- D. Solaiman, M. M. Jonah, W. Miyazaki, G. Ho and M. H. Bhattacharyya, Toxicol. Sci, 2001, 60, 184–192 CrossRef PubMed
.
- H. Milnerowicz and M. Chmarek, Acta Paediatr., 2005, 94, 402–406 CrossRef PubMed
.
- J. P. Groten, E. J. Sinkeldam, J. B. Luten and P. J. Van Bladeren, Toxocol. Appl. Pharmacol., 1991, 111, 504–513 CrossRef
.
- S. Ben Mimouna, M. Chemek, S. Boughammoura, M. Banni and I. Messaoudi, Biol. Trace Elem. Res., 2017, 184, 1–13 Search PubMed
.
- S. Ben Mimouna, M. Chemek, S. Boughammoura, Z. Haouas and I. Messaoudi, Drug Chem. Toxicol., 2018, 3, 1–10 CrossRef PubMed
.
|
This journal is © The Royal Society of Chemistry 2018 |
Click here to see how this site uses Cookies. View our privacy policy here.