DOI:
10.1039/C6RA14932D
(Paper)
RSC Adv., 2016,
6, 84990-84998
Supranutritional dietary selenium induced hyperinsulinemia and dyslipidemia via affected expression of selenoprotein genes and insulin signal-related genes in broiler†
Received
8th June 2016
, Accepted 25th August 2016
First published on 26th August 2016
Abstract
The purpose of this study was to investigate the effects of supranutritional selenium (Se) on the mRNA expression of selenoprotein genes and insulin signal-related genes in the liver, muscle and pancreas of the broiler. A total of 160 one-day-old Cobb male broilers (n = 80 per group) were fed a Se-deficient corn–soybean basal diet supplemented with 0.3 (adequate), and 3.0 (excess) mg kg−1 sodium selenite for 6 weeks. Pancreas, liver and muscle were collected, and the effects of supranutritional Se on mRNA abundance of 23 selenoprotein genes and 17 insulin-related genes were compared at day 42. Also, enzyme activities and plasma biochemical measurements were measured at days 14, 28, and 42. The results showed that a high-Se diet depressed the growth performance of broilers and elevated (P < 0.05) the activities of superoxidase dismutase (SOD), total antioxidant capacity (T-AOC) and glutathione peroxidase (GSH-Px), mainly in the early stages. A high-Se diet up-regulated 12 selenoprotein genes in muscle, 7 genes in liver and Sephs2 in the pancreas, and down-regulated 3 selenoprotein genes in muscle, 4 genes in liver and 7 genes in the pancreas. Meanwhile, 4, 3 and 2 insulin signal-related genes were up-regulated in muscle, liver and the pancreas, respectively, 3 and 5 genes were down-regulated in muscle and the pancreas. Accompany the aberrant expression of selenoprotein and insulin signal-related genes, the birds exhibited a higher plasma insulin, total triglyceride (TG) and total cholesterol (TC) concentrations by high Se. In conclusion, the high Se diet induced hyperinsulinemia and dyslipidemia in birds via a mediating aberrant expression of selenoprotein genes associated with insulin signal-related genes, which indicated potential roles of those selenoprotein genes in the lipid and carbohydrate metabolism regulation in birds.
Introduction
Selenium (Se) is identified as an essential nutrient and plays an important role in the health of humans and animals.1,2 While its importance for the immune,3 fertility,4 and thyroid functions,5 and for cancer prevention,6 is well established, the association between Se and glucose metabolism is conflicting. Previous studies have shown that Se is incorporated into selenoproteins that have antioxidant and anti-inflammatory effects,7 and as an insulin mimetic, Se can activate key proteins involved in the insulin signaling pathway and has an anti-diabetic effect.8 However, researchers have reported that supranutritional Se intake is associated with the development of insulin resistance, advising blood lipid profiles and increasing the risk of type 2 diabetes in humans.9–11 Prolonged feeding of high dietary Se (3.0 mg kg−1) induces gestational diabetes in rat dams and insulin resistance in their offsprings,12 and pigs fed 3.0 mg Se per kg become hyperinsulinemic compared with those fed 0.3 mg Se per kg.13
Both insulin secretion and insulin signaling are linked to the cellular redox state,14,15 providing a rationale for an interference of Se and antioxidant selenoproteins with insulin-regulated metabolic pathways. Supranutritional Se may dys-regulate insulin synthesis and secretion, and suppress insulin signaling. Meanwhile, it may also affect the activities or production of selenoproteins and the intracellular redox state and eventually induce hyperinsulinemia, insulin resistance and lipid metabolism disorder.8 However, the resulting mechanism of how supranutritional Se affects insulin signaling and selenoproteins remains unclear.
The pancreas is the organ responsible for the secretion of insulin. Synthesis and secretion of insulin in pancreatic islets are regulated by transcriptional factors, signal molecules, and functional proteins encoded by genes such as pancreatic and duodenal homeobox 1 (Pdx1), neurogenic differentiation 1 (Neurod1), hnf1 homeobox A (Hnf1a), forkhead box A2 (Foxa2) and glucose transporter 2 (Glut2).16–19 The main targets of insulin are muscle and liver, and the insulin signaling components in those tissues may be associated with insulin resistance caused by high Se.12 Some key proteins relating to the insulin signaling cascade include insulin receptor (Ir), insulin substrate 1 (Irs1), insulin substrate 2 (Irs2), serine/threonine protein kinase 1 (Akt1), forkhead box O1 (Foxo1), and phosphoinositide 3-kinase (Pi3k). Evidence has indicated that selenate is able to initiate the transportation of glucose transporters to the membrane surface,20 and then induce phosphorylation of the Ir.21 A number of studies have illustrated that deviant expression or activation of any of these protein may lead to disruption of the carbohydrate and lipid metabolism.22 Some evidence have indicated the presence of correlations between the expression of insulin signal proteins, or other key proteins relating to insulin metabolism, and Se through the redox system or in other ways.23
Previous research mainly focused on the effects of high Se on potential insulin resistance in mammals.9–13 Chicken is very susceptible to the status of dietary Se.24 It is now well established that Se plays important biological roles, mostly through its incorporation in a family of proteins called selenoproteins, and the incorporated amino acid selenocysteine (Sec) is considered to be the 21st amino acid.25 However, the effects of supranutritional Se on the expression of selenoprotein genes and insulin signaling genes in chickens remain unclear, and systematic data are needed to link selenoprotein gene expression profiles to metabolic impacts of excess dietary Se on insulin metabolism tissues. Therefore, we used broilers and conducted a 6 week feeding experiment to: (1) determine if the high Se intake can induce dys-regulation of plasma glucose, lipid, and insulin; (2) compare the effects of dietary high Se (3.0 mg Se per kg) on the mRNA expression profiles of 23 selenoproteins in the pancreas, liver and muscle; and (3) explore the effects of dietary high Se on the mRNA expression profiles of 17 insulin signal-related genes in those three tissues.
Materials and methods
Broilers, diet, and experimental design
The animal protocol was approved by the Animal Care Office of Sichuan Agricultural University, Chengdu, China, and the animal experimental procedure was performed according to the guidelines for the care and use of experimental animals established by the Ministry of Agriculture of People's Republic of China. Informed consent was obtained for any experimentation with human subjects. A total of 160 one-day-old Cobb male broilers of average body weight (44.30 ± 0.49) g were randomly allocated into two groups, each of 4 replicates with 20 birds. The birds were fed a Se-deficient corn–soybean basal diet (ESI Table 1†) supplemented with 0.3 and 3.0 mg kg−1 sodium selenite for 6 weeks, respectively. The basal diet (BD) was formulated to best meet the nutrient requirements of the broiler. The analyzed Se concentration (mg kg−1) in BD + 0.3 mg Se (adequate) and BD + 3.0 mg Se (excess) diets were 0.31 and 3.04 mg Se per kg, respectively. Broilers were housed in wire-mesh cages and the temperature was maintained at 32, 28, and 25 °C for the first, second and subsequence weeks, respectively. Birds had free access to water and were fed ad libitum. The experiment lasted for 6 weeks. Birds were weighted at biweekly intervals and feed intakes were recorded to calculate body weight gain and feed intake (g per days). Daily observations were made to record general health, clinical symptoms of Se excess diseases and mortality.
Sample collection and preparation
At the end of the 2nd, 4th and 6th weeks, birds were fasted for 8 h overnight, and then four birds with the average body weight per group were selected and killed by decapitation to collect blood, liver, pectoral muscle and pancreas. Plasma samples were prepared by centrifugation of the whole blood (sodium EDTA as anticoagulant, 3000g for 10 min, 5804R centrifuge, F45-30-11 rotor; Eppendorf, Hamburg, Germany) and stored at −80 °C. Tissue samples, including muscle, liver, and pancreas, were immediately removed, minced and rinsed with ice-cold isotonic saline, frozen in liquid nitrogen, and stored at −80 °C until used.
Biochemical measurements
Se concentration of feed and tissues were measured using the hybrid generation-atomic fluorescence spectrometer (AFS-3200, Titan instrument) against the standard Se reference [GBW (E) 08044, National Research Centre for Certified Reference Materials, Beijing, China]. Fasting plasma glucose, insulin, TG and TC concentrations were determined by using the corresponding assay kit (Jiancheng Bioengineering, Nanjing, China) according to the manufacturers' instructions. Tissue samples (around 0.5 g) were thawed on ice and homogenized in ice-cold isotonic saline (0.9% NaCl) using Bullet Blender (Storm BBY24M, Next Advance, USA) for 25 s three times, and then centrifuged at 1000g for 10 min at 4 °C, and the supernatant was collected for further analysis. Activities of the superoxide dismutase (SOD), glutathione peroxidase (GSH-Px), total antioxidant capability (T-AOC) and concentration of malondialdehyde (MDA) were determined by using the corresponding assay kits (Jiancheng Bioengineering, Nanjing, China) according to the manufacturers' instructions. Concentrations of protein were determined with the bicinchoninic acid (BCA) method by using a commercial BCA protein assay kit (Jiancheng Bioengineering, Nanjing, China).
Q-PCR analyses of mRNA abundance
RNA extraction and quality control were conducted as described previously by our group13,26,27 with the exception that the total RNA of the pancreas was extracted using RNeasy Tissue Mini Kit (Takara, Dalian, China). qPCRs were performed on a ABI 7900HT system (Applied Biosystems, Foster City, CA, USA) in a final volume of 10 μL using a Qiagen one-step RT-PCR kit (Qiagen, Duesseldorf, Germany). A total RNA of 100 ng was applied to each sample. Melting curve and cycle threshold analyses were performed to confirm the specificity of amplification. The primers for the 23 selenoprotein genes, 17 insulin signal-related genes, and two reference genes, β-actin (Actb) and glyceraldehyde 3-phosphate dehydrogenase (Gapdh), were designed using Primer Express 3.0 (Applied 156 Biosystems, Foster City, CA) and are presented in ESI Table 2.† The relative mRNA abundance quantification was the same as previously described by our group using the ΔCt method.26,27
Statistical analysis
Independent t-test (SPSS for Windows 13.0; Chicago, IL, USA) was used to analyze the effects of the supranutritional dietary Se on plasma biochemical measurements and mRNA levels of each gene within the same tissue. Data are presented as means ± SE and the significance level was set at P ≤ 0.05 unless indicated otherwise.
Results
Growth performance and tissue Se concentration
A dietary high-Se supplement negatively affected the growth performance of broilers (Table 1). Compared to the control group (0.3 mg Se per kg), a high-Se diet intake decreased body weight gains of the broilers during 2–4 week and 4–6 week (P < 0.05), and also the feed intake during 2–4 week was significantly decreased (P < 0.05). Feed intake/body gain was not affected by the high Se diet in any period of the experiment (data not shown). No clinical symptoms of Se excess diseases were found in broilers fed on 3.0 Se mg kg−1, and also there was no difference in mortality between the two groups (data not shown). Supranutritional Se significantly increased Se concentration in the plasma and liver of broilers in each period (P < 0.01), and improved by 1.2 to 2.6 fold in broilers fed on 3.0 mg Se per kg compared with those fed on 0.3 mg Se per kg at the same growth stage (Table 1).
Table 1 Growth performances and tissue Se concentration of broiler fed diets containing 0.3 and 3.0 mg Se per kg for 6 weeka
Measures |
0.3 mg Se per kg |
3.0 mg Se per kg |
P value |
Values are mean ± SE, n = 4.*P < 0.05; **P < 0.01, compared with the control group (0.3 mg Se per kg). |
Body weight gain (g d−1) |
0–2 week |
19.11 ± 0.56 |
18.48 ± 0.37 |
0.39 |
2–4 week |
42.63 ± 1.20 |
37.10 ± 0.48** |
<0.01 |
4–6 week |
66.38 ± 1.87 |
59.35 ± 1.46* |
0.03 |
![[thin space (1/6-em)]](https://www.rsc.org/images/entities/char_2009.gif) |
Feed intake (g d−1) |
0–2 week |
24.02 ± 1.04 |
22.51 ± 1.13 |
0.37 |
2–4 week |
81.61 ± 2.88 |
72.10 ± 0.92* |
0.02 |
4–6 week |
129.1 ± 6.8 |
118.6 ± 1.8 |
0.19 |
![[thin space (1/6-em)]](https://www.rsc.org/images/entities/char_2009.gif) |
Plasma Se (μmol L−1) |
2nd week |
127.8 ± 1.4 |
152.9 ± 4.5** |
<0.01 |
4th week |
152.2 ± 10.9 |
400.7 ± 12.9** |
<0.01 |
6th week |
236.1 ± 1.5 |
388.1 ± 19.1** |
<0.01 |
![[thin space (1/6-em)]](https://www.rsc.org/images/entities/char_2009.gif) |
Liver Se (μg kg−1) |
2nd week |
408.4 ± 13.1 |
984.4 ± 44.6** |
<0.01 |
4th week |
537.3 ± 19.8 |
1386.5 ± 56.8** |
<0.01 |
6th week |
616.9 ± 25.4 |
1319.7 ± 53.6** |
<0.01 |
Antioxidant measurements in plasma and tissues
The effects of excess dietary Se on the activities of GSH-Px, SOD, T-AOC and the concentration of MDA in the plasma and tissues of broilers were investigated; the results are shown in Table 2. Compared to the 0.3 mg Se per kg group, a high Se diet improved plasma GSH-Px by 37% (P < 0.01) and decreased plasma MDA concentration by about 35% (P < 0.01) at the 4th week. SOD and T-AOC exhibited no difference between the two groups during the experiment period (data not shown). In liver, the activities of SOD and concentration of MDA had increased in the high-Se group by the 4th week (P < 0.05), whereas T-AOC decreased by 8% in the high-Se group at 4 week compared to those in the 0.3 mg Se per kg group (P < 0.05). A high Se intake increased GSH-Px activities by about 1.8, 2.2 and 2.8 fold in muscle at the 2nd, 4th and 6th week (P < 0.01), respectively. The SOD activities in muscle also increased in broilers fed on the higher Se diet compared with the control group at the 2nd week (P < 0.05). There were no changes of T-AOC and MDA in muscle between the two groups in each period (data not shown). A high-Se diet exhibited impacts on antioxidant measurements in the pancreas mainly in early period (Table 2), which resulted in 38, 28 and 27% higher (P < 0.05) GSH-Px, SOD and T-AOC in the pancreas compared to that in the 0.3 mg Se per kg group at the 2nd week. The effect of high-Se on the pancreas SOD lasted to the 4th week, which showed a 2.7-fold increase (P < 0.05) compared to the 0.3 mg Se per kg group, whereas no difference was found between the two groups at the 6th week.
Table 2 Effect of supranutritional Se on the antioxidant measurements in liver, muscle, pancreas and seruma
Parameters |
2nd week |
4th week |
6th week |
0.3 mg Se per kg |
3.0 mg Se per kg |
0.3 mg Se per kg |
3.0 mg Se per kg |
0.3 mg Se per kg |
3.0 mg Se per kg |
Values are mean ± SE, n = 4.*P < 0.05; **P < 0.01, compared with the control group (0.3 mg Se per kg). |
Serum |
GSH-Px (U ml−1) |
411.91 ± 144.62 |
459.25 ± 61.4 |
608.16 ± 10.60 |
833.12 ± 51.21** |
804.60 ± 185.10 |
870.81 ± 263.50 |
MDA (nmol ml−1) |
4.64 ± 0.27 |
5.36 ± 0.47 |
1.45 ± 0.32 |
0.94 ± 0.30* |
2.62 ± 0.64 |
2.14 ± 0.58 |
![[thin space (1/6-em)]](https://www.rsc.org/images/entities/char_2009.gif) |
Liver |
SOD (U mg−1 prot.) |
116.95 ± 2.01 |
114.92 ± 2.62 |
111.51 ± 1.05 |
115.02 ± 1.61* |
111.98 ± 1.10 |
113.89 ± 2.36 |
T-AOC (U mg−1 prot.) |
1.66 ± 0.19 |
1.40 ± 0.30 |
1.47 ± 0.072 |
1.35 ± 0.05* |
1.457 ± 0.05 |
1.687 ± 0.20 |
MDA (nmol mg−1 prot.) |
0.93 ± 0.20 |
0.88 ± 0.25 |
0.63 ± 0.07 |
0.85 ± 0.09* |
1.06 ± 0.25 |
0.81 ± 0.17 |
![[thin space (1/6-em)]](https://www.rsc.org/images/entities/char_2009.gif) |
Muscle |
GSH-Px (U mg−1 prot.) |
5.50 ± 1.34 |
9.81 ± 1.26** |
7.36 ± 1.04 |
16.36 ± 3.76** |
8.09 ± 1.02 |
22.64 ± 2.48** |
SOD (U mg−1 prot.) |
53.56 ± 12.75 |
75.78 ± 25.52 |
49.92 ± 7.02 |
80.74 ± 16.99* |
81.04 ± 17.27 |
75.40 ± 25.51 |
![[thin space (1/6-em)]](https://www.rsc.org/images/entities/char_2009.gif) |
Pancreas |
GSH-Px (U mg−1 prot.) |
15.20 ± 0.42 |
20.96 ± 4.57* |
13.15 ± 1.43 |
12.95 ± 2.43 |
11.48 ± 2.42 |
11.21 ± 1.17 |
SOD (U mg−1 prot.) |
11.55 ± 0.86 |
14.76 ± 0.52** |
22.54 ± 6.48 |
61.39 ± 12.56* |
33.39 ± 6.22 |
35.47 ± 1.84 |
T-AOC (U mg−1 prot.) |
0.82 ± 0.04 |
1.04 ± 0.01** |
0.68 ± 0.05 |
0.66 ± 0.16 |
0.73 ± 0.17 |
0.72 ± 0.17 |
Biochemical measurements in plasma
Broilers fed 3.0 mg kg−1 Se (high Se group) exhibited a lower fasting plasma glucose concentration in each period compared to the control group (0.3 mg kg−1 Se), especially at the 2nd week when the plasma glucose was significantly lower (P < 0.05) than that of control (Fig. 1A). A correspondingly high-Se diet resulted in a higher plasma insulin concentration in each period compared to that of the control group, and the difference between the two groups attained a significant level (P < 0.05) at the 2nd week (Fig. 1B). The results show that the concentrations of plasma TC and TG were higher in broilers fed on high-Se diet compared to the control group during each period. As the age increased, the effect of high-Se on TC increased and at the 6th week the concentration of plasma TC in the 3.0 mg Se per kg group was significantly higher than that of the control group (P < 0.05) (Fig. 1C). A high-Se diet resulted in a higher plasma TG concentration at the 2nd and 4th week (P < 0.05), while it showed no difference compared to that of the control group at the 6th week (Fig. 1D).
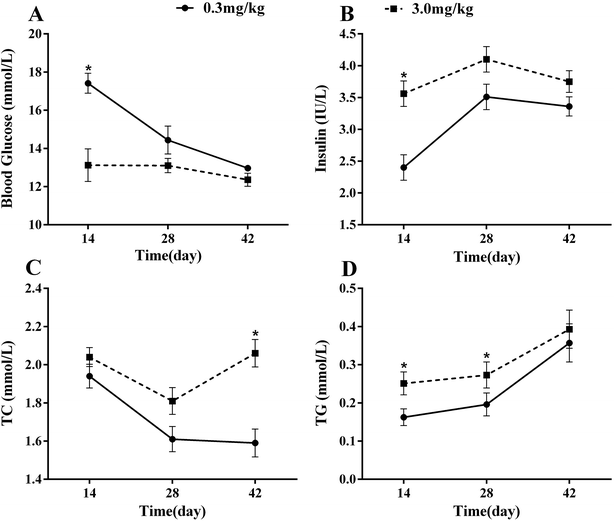 |
| Fig. 1 Effects of supranutritional Se on biochemical measurements in plasma. Data are presented as means ± SE (n = 4). | |
Abundances of selenoprotein mRNA
The expression of selenoprotein genes in various tissues at 6 week responded to dietary Se concentrations in 3 patterns. The first was that dietary high-Se (3.0 mg Se per kg) resulted in an increase (P < 0.05 or as indicated) of tissue mRNA levels compared with those fed on Se at 0.3 mg kg−1 (Fig. 2). Those up-regulated genes included 7 selenoprotein genes (Txnrd1, Txnrd3, Selm, Seli, Selx, Sepn1 and Sephs2) in liver (Fig. 2A), 12 selenoprotein genes (Gpx1, Gpx2, Txnrd1, Txnrd3, Dio2, Selk, Selo, Selu, Seli, Sepn1, Sepw1, and Sephs2) in muscle (Fig. 2C), and Sephs2 in the pancreas (Fig. 2B). The second pattern was manifested as lower (P < 0.05 or as indicated) mRNA levels in the high-Se group than those in the 0.3 mg kg−1 dietary Se group (Fig. 3). Those down-regulations included 3 genes (Dio1, Selm and Sepp1) in muscle (Fig. 3A), 4 selenoprotein genes (Gpx1, Selk, Selu and Sepw1) in liver (Fig. 3B), and 7 selenoprotein genes (Gpx1, Gpx3, Gpx4, Dio3, Selm, Selk and Sepp1) in the pancreas (Fig. 3C). Thirdly, dietary Se excess had no significant effects on the expression of selenoprotein genes in the three tissues investigated (ESI Table 3†).
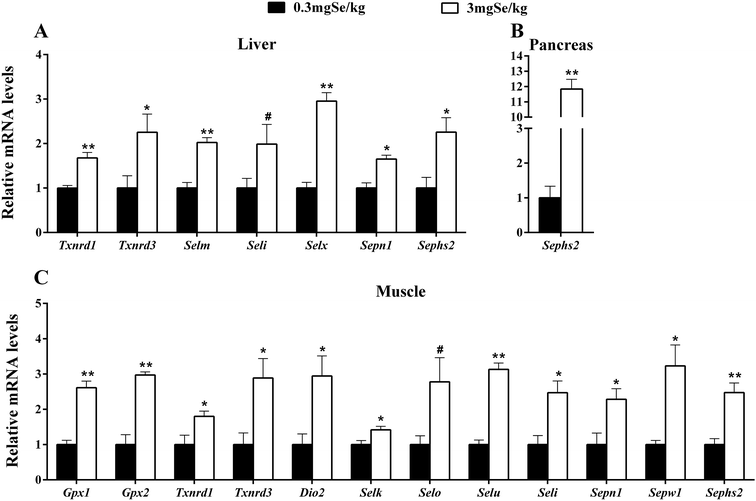 |
| Fig. 2 Effects at the 6th week of supranutritional dietary Se on relative mRNA levels of the up-regulated selenoprotein genes in liver (A), pancreas (B) and muscle (C) of chickens compared with those fed the control diet. Data are presented as means ± SE (n = 4). Asterisks indicate difference from control: #P < 0.1,*P < 0.05, **P < 0.01. | |
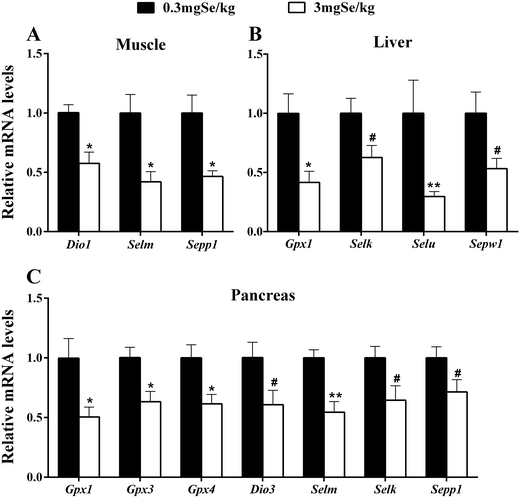 |
| Fig. 3 Effects at the 6th week of supranutritional dietary Se on relative mRNA levels of the down-regulated selenoprotein genes in muscle (A), liver (B) and pancreas (C) of chickens compared with those fed the control diet. Data are presented as means ± SE (n = 4). Asterisks indicate difference from control: #P < 0.1,*P < 0.05, **P < 0.01. | |
mRNA levels of insulin signal-related genes
Among the 17 insulin signal-related genes assayed, 6 genes in three tissues were up-regulated by the high-Se diet at the 6th week (P < 0.05) (Fig. 4). These included Foxo1, Hnf4A, Irs2 and Pi3k in muscle (Fig. 4A), Gcg, Hnf4A and Slc2A2 in liver (Fig. 4B), and Hnf4A and Irs2 in the pancreas (Fig. 4C). Hnf4A was up-regulated in the three tissues investigated and Irs2 was up-regulated in 2 tissues (pancreas and muscle). In contrast, 7 genes were down-regulated (P < 0.05 or as indicated) in 2 tissues (Fig. 5). These changes included 5 genes (Akt1, Foxa2, Ins, Pi3k and Ucp) in the pancreas (Fig. 5A), and three genes (Akt1, Gcg and Ir) in muscle (Fig. 5B). The unaffected insulin signal-related genes are shown in ESI Table 4.†
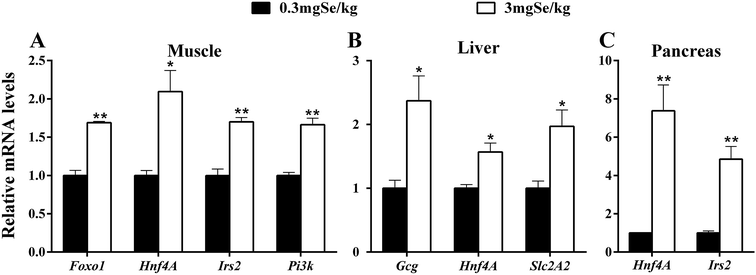 |
| Fig. 4 Effects at the 6th week of supranutritional dietary Se on relative mRNA levels of the up-regulated insulin signal-related genes in muscle (A), liver (B) and pancreas (C) of chickens compared with those fed the control diet. Data are presented as means ± SE (n = 4). Asterisks indicate difference from control: #P < 0.1,*P < 0.05, **P < 0.01. | |
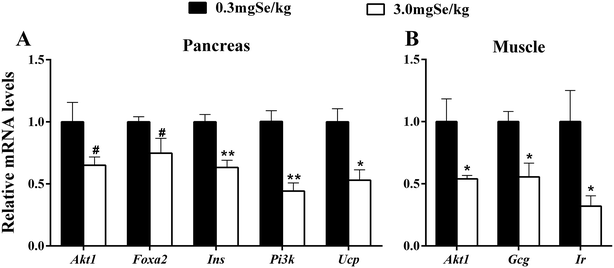 |
| Fig. 5 Effects of supranutritional dietary Se on relative mRNA levels of the down-regulated insulin signal-related genes in pancreas (A) and muscle (B) of chickens compared with those fed the control diet at 6th week. Data are presented as means ± SE (n = 4). Asterisks indicate difference from control: #P < 0.1,*P < 0.05, **P < 0.01. | |
Discussion
The present study has clearly demonstrated that the supranutritional dietary Se feeding elevated fasting plasma insulin concentrations of chicken and affected the mRNA abundances of 17 insulin metabolism-related genes in three tissues. The essential mineral element Se can supply antioxidants and is especially active in maintaining the production performance for poultry.28 In the present study, supranutritional dietary Se increased tissue Se deposition and depressed the growth performance of chickens (Table 1). The results were consistent with previous research that broilers fed with supranutritional Se (2.30 mg Se per kg) have lower feed intake and body weight gain29 and Se intake beyond nutritional requirements can trigger adverse side-effects in chickens.30 However, supranutritional Se (3.0 mg Se per kg) exhibited no growth performance depression effects in pig13,27,31 and rat dam12 models, indicating chicken is more sensitive to Se status than mammals. Previous studies have shown adverse effects of supranutritional Se on carbohydrate and lipid metabolisms, such as hyperglycemia, dyslipidemia and even type 2 diabetes.8,32 The overall metabolic responses (Fig. 1) of chicken to the excess dietary Se were very similar to those of rodents12,33 and pigs.13 Furthermore, the present study has also shown that higher plasma TG and TC concentrations in chickens fed on supranutritional Se during each period (Fig. 1). Apparently, high Se exposure is associated with increased plasma lipid levels and the risk of dyslipidemia.34 Therefore, the observed changes in plasma insulin, lipid, and insulin metabolism-related gene expressions in the present study indicate the experimental suitability and physiological relevance of this chicken model for studying interactions and mechanisms between insulin metabolism and supranutritional dietary Se via selenoprotein expression.
To reveal the interference of selenoproteins with the insulin-regulated carbohydrate and lipid metabolisms, we investigated the effects of supranutritional dietary Se on the mRNA abundance of 23 selenoprotein genes in three main insulin metabolism organs of the broiler. The results show that supranutritional dietary Se up-regulated 12 genes in muscle, 7 genes in liver and Sephs2 in the pancreas (Fig. 2). Muscle and liver are the important insulin target organs, and in the present study the two organs had more up-regulated selenoprotein genes compared with the pancreas when subjected to excess dietary Se. Among the affected genes, Gpx1 is considered a primary mediator in insulin resistance12 and plays a key role in glucose homoeostasis in myocytes35 and mice.36 The up-regulation of Gpx1 and Gpx2 was consistent with the increased GSH-Px activity in muscle and might be correlated with insulin resistance caused by high Se.9 Enhanced Txnrd1 and Txnrd3 expressions, through mediating the thioredoxin activity, have the potential to control the redox state.37 Dio1, Dio2 and Seli might be involved in the lipid metabolism26,38 and the increased expression of those genes in liver and muscle may partially contribute to the higher plasma TG and TC induced by high Se supplementation. Our results show that Sepn1 was up-regulated by high Se in muscle and liver, which was very consistent with previous studies on broilers.39 Moreover, Selk, Selo, Slem, Sepn1 and Sepw1 all participate in redox in cells40–44 and up-regulation of those genes indicates that oxidation stress may be induced by excess dietary Se. Sephs2 is responsible for selenoprotein biosynthesis,45 and thus it may be easy to explain why it was up-regulated in three organs under sufficient Se supplementation in the present study.
The down-regulation of 14 selenoprotein genes was found in three organs affected by supranutritional dietary Se. The pancreas had the most altered genes (7 genes), followed by 4 genes in liver and 3 genes in muscle (Fig. 3). These down regulations may reflect a general adverse oxidative effect of the high Se on tissue redox control or lipid metabolism as most of these genes encode redox-associated selenoproteins.46 This notion is supported by the number and types of selenoprotein genes (for example Gpx1, Gpx3, Gpx4) affected in the pancreas, while the pancreas is the organ with a high redox level and low amounts of the major antioxidant enzymes in maintaining its insulin secretion function.47,48 Studies have shown that there is abundant Dio3 in islet B,32,49 and the number and gene expression of β cell or GSIS are influenced when Dio3 is damaged.50 Sepp1 was down-regulated in the pancreas and muscle (Fig. 3), and the down-regulation of Sepp1 might be a feedback regulation by the increased insulin concentration caused by high Se.51
As mentioned above, chicken muscle had the most up-regulated selenoprotein genes, while the pancreas had the most down-regulated selenoprotein genes among all the three tissue assayed. These differential regulations by dietary high Se may be partially explained by “tissue specific expression” and “hierarchy of selenoprotein expression”.52 That the major changes in selenoprotein gene expression resulted from feeding the high dietary Se may reflect crucial roles of the respective selenoproteins in the affected tissues. Also, more selenoprotein genes were affected in liver and muscle by dietary high Se in the present study than in our previous studies in the pig13 and rodent models.12 The pancreas is the only organ that produces insulin, while liver and muscle are the main insulin target tissues. The unique responses of selenoprotein genes to dietary high Se in the insulin metabolism tissues may be associated with their metabolic interactions between relevant hormones and Se.
The dietary high Se also exerted impacts on the 17 insulin signal-related genes assayed. These included up-regulations of 6 genes (Gcg, Foxo1, Hnf4A, Slc2A2, Irs2 and Pi3k) in three tissues (Fig. 4), and down-regulations of 7 genes in two tissues (Fig. 5). These divergent patterns of response may reflect crucial roles of each gene in different tissues. In the pancreas, a total of 5 genes (Akt1, Foxa2, Ins, Pi3k and Ucp) were down-regulated and 2 genes (Irs2 and Hnf4A) were up-regulated by excess Se. High-Se affected some key regulators, such as Foxa2, Pdx1, Ucp and Hnf4A, which exert important roles of secretion and synthesis of insulin in B cells. Previous research has shown that Akt1 and Foxo2 play an important role in the regulation of pancreas cell differentiation and function, and the deletion of Foxa2 in the pancreas results in hyperinsulinemic hypoglycemia.53,54 In B cells, Irs2 regulates the growth and proliferation of B cells by the Pi3k/Akt and MAPK signal pathway.55 Therefore, the lower expression of Foxa2 and higher expression of Irs2 in the pancreas might partly explain the higher plasma insulin by high dietary Se. Se acts as an insulin-mimetic molecule56 and high Se may promote the synthesis and secrete of insulin in the pancreas. In the present study, high-Se decreased Pi3k and Akt1 expression in the pancreas, and this may reflect a feedback to a weak insulin signal. Liver plays an important role in nutrient homeostasis through regulating carbohydrate, protein, and fat metabolism,57 and liver and muscle are important target organs in which insulin exerts its biological function through the Pi3k/Akt signal pathway. In liver, a high-Se diet up-regulated Slc2A2, Hnf4A and Gcg. Slc2A2 is responsible for encoding Glut2, an important glucose transporter.58 The up-regulated expression of Slc2A2 indicates that high Se might promote the uptake of glucose in liver. There is evidence that Gcg plays key roles in the regulation of fat and glucose metabolism in liver,59 and the up-regulated expression of Gcg in liver may reflect a need to keep plasma glucose homeostasis under a high Se status. In muscle, high-Se diet up-regulated 4 genes (Irs2, Hnf4A, Pi3k and Foxo1) and down-regulated 3 genes (Ir, Gcg and Akt1). Insulin could induce the Akt-mediated phosphorylation of Foxo1 to suppress the expression of gluconeogenic genes.60 The damaged Ir or Akt phosphorylation would cause insulin resistance in the liver and muscle of mice.9 In muscle, Foxo1 was up-regulated while Akt1 and Ins were down-regulated by a high-Se diet in the present study, which may indicate a decreasing sensitivity of muscle to plasma insulin by high dietary Se. Hnf4A as a transcription factor can regulate the expression of protein involving the insulin signal pathway, such as Glut2.61 After entering the necleus, Hnf4A interacts with Foxo1 and induces the expression of SelP in mammals.62 In the present study high-Se diet up-regulated Hnf4A in the three organs investigated, which indicates that Hnf4A plays an important role in the insulin signal pathway mediated by high Se.
We also compared the effects of supranutritional dietary Se on antioxidant capacity in tissues of the chicken and the results showed the increased (P < 0.05) activities of GSH-Px, T-AOC and SOD mainly occurred in the early stages (2nd and 4th week) (Table 2). These are consistent with previous research.63,64 GSH-Px is an important Se-containing enzyme in the blood and tissues of animals. Abundant expression of antioxidant selenoproteins due to dietary Se oversupply was reported to result in hyperinsulinemia and decreased insulin sensitivity.9,32 In the present study we observed an improving effect of oversupply of Se on GSH-Px in muscle that lasted at the 6th week (Table 2) and an up-regulation of Gpx1 in muscle by high Se (Fig. 2), which indicated a potential impaired sensitivity of muscle to insulin. Therefore, this might partly explain the potential relationship between abundant antioxidant enzymes and the symptoms of hyperinsulinemia and dyslipidemia by an overdose of Se supply.
Conclusions
This study indicated that a dietary excess of Se depressed the growth performance of broilers, affected tissue antioxidant activity, and caused hyperinsulinemia and dyslipidemia. Also, the expressions of selenoproteins and insulin signal-related genes in the pancreas, liver and muscle were greatly affected. The present study provides some compensated data about the roles of Se in the regulation of insulin metabolism and the results may imply a potential link between selenoprotein genes and insulin metabolism by using a chicken model.
Acknowledgements
This work was supported partly by the National Natural Science Foundation of China (No. 31272468 and 31072043) and by a Research Funding provided by the Sichuan Longda Animal Husbandry Science and Technology Co., Ltd. (No. 2015SCLD001). We would like to thank the staff at our laboratory for their ongoing assistance.
References
- R. F. Burk and K. E. Hill, Annu. Rev. Nutr., 2005, 25, 215–235 CrossRef CAS PubMed.
- H. Tapiero, D. Townsend and K. Tew, Biomed. Pharmacother., 2003, 57, 134–144 CrossRef CAS.
- Z. Huang, A. H. Rose and P. R. Hoffmann, Antioxid. Redox Signaling, 2012, 16, 705–743 CrossRef CAS PubMed.
- C. Boitani and R. Puglisi, Adv. Exp. Med. Biol., 2008, 636, 65–73 CrossRef CAS PubMed.
- L. Schomburg, Nat. Rev. Endocrinol., 2011, 83, 160–171 CrossRef PubMed.
- H. Zeng and G. J. Combs, J. Nutr. Biochem., 2008, 19, 1–7 CrossRef CAS PubMed.
- M. P. Rayman, Lancet, 2012, 379, 1256–1268 CrossRef CAS.
- J. Zhou, K. Huang and X. G. Lei, Free Radicals Biol. Med., 2013, 65, 1548–1556 CrossRef CAS PubMed.
- J. P. McClung, C. A. Roneker, W. Mu, D. J. Lisk, P. Langlais, F. Liu and X. G. Lei, Proc. Natl. Acad. Sci. U. S. A., 2004, 101, 8852–8857 CrossRef CAS PubMed.
- S. Stranges, M. Laclaustra, C. Ji, F. P. Cappuccio, A. Navas-Acien, J. M. Ordovas, M. Rayman and E. Guallar, J. Nutr., 2010, 140, 81–87 CrossRef CAS PubMed.
- S. Stranges, S. Sieri, M. Vinceti, S. Grioni, E. Guallar, M. Laclaustra, P. Muti, F. Berrino and V. Krogh, BMC Public Health, 2010, 10, 564–572 CrossRef PubMed.
- M. S. Zeng, X. Li, Y. Liu, H. Zhao, J. C. Zhou, K. Li, J. Q. Huang, L. H. Sun, J. Y. Tang and X. J. Xia, Free Radicals Biol. Med., 2012, 52, 1335–1342 CrossRef CAS PubMed.
- Y. Liu, H. Zhao, Q. Zhang, J. Tang, K. Li, X. J. Xia, K. N. Wang, K. Li and X. G. Lei, J. Nutr., 2012, 142, 1410–1416 CrossRef CAS PubMed.
- X. G. Lei and M. Z. Vatamaniuk, Antioxid. Redox Signaling, 2011, 14, 489–503 CrossRef CAS PubMed.
- A. A. Szypowska and B. M. Burgering, Antioxid. Redox Signaling, 2011, 15, 219–232 CrossRef CAS PubMed.
- U. Ahlgren, J. Jonsson, L. Jonsson, K. Simu and H. Edlund, Genes Dev., 1998, 12, 1763–1768 CrossRef CAS PubMed.
- C. Gu, G. H. Stein, N. Pan, S. Goebbels, H. Hörnberg, K. A. Nave, P. Herrera, P. White, K. H. Kaestner and L. Sussel, Cell Metab., 2010, 11, 298–310 CrossRef CAS PubMed.
- K. A. Lantz, M. Z. Vatamaniuk, J. E. Brestelli, J. R. Friedman, F. M. Matschinsky and K. H. Kaestner, J. Clin. Invest., 2004, 114, 512–520 CrossRef CAS PubMed.
- D. Q. Shih, S. Screenan, K. N. Munoz, L. Philipson, M. Pontoglio, M. Yaniv, K. S. Polonsky and M. Stoffel, Diabetes, 2001, 50, 2472–2480 CrossRef CAS PubMed.
- C. Fürnsinn, R. Englisch, K. Ebner, P. Nowotny, C. Vogl and W. Waldhäusl, Life Sci., 1996, 59, 1989–2000 CrossRef.
- G. L. Brown, L. Curtsinger, J. R. Brightwell, D. M. Ackerman, G. R. Tobin, H. Polk, C. George-Nascimento, P. Valenzuela and G. Schultz, J. Exp. Med., 1986, 163, 1319–1324 CrossRef CAS PubMed.
- S. F. Previs, D. J. Withers, J. M. Ren, M. F. White and G. I. Shulman, J. Biol. Chem., 2000, 275, 38990–38994 CrossRef CAS PubMed.
- B. J. Goldstein, K. Mahadev and X. Wu, Diabetes, 2005, 54, 311–321 CrossRef CAS PubMed.
- J. Q. Huang, D. L. Li, H. Zhao, L. H. Sun, X. J. Xia, K. N. Wang, X. Luo and X. G. Lei, J. Nutr., 2011, 141, 1605–1610 CrossRef CAS PubMed.
- A. V. Lobanov, D. L. Hatfield and V. N. Gladyshev, Biochim. Biophys. Acta, 2009, 1790, 1424–1428 CrossRef CAS PubMed.
- H. Zhao, K. Li, J. Y. Tang, J. C. Zhou, K. N. Wang, X. J. Xia and X. G. Lei, J. Nutr., 2015, 145, 1394–1401 CrossRef CAS PubMed.
- J. C. Zhou, H. Zhao, J. G. Li, X. J. Xia, K. N. Wang, Y. J. Zhang, Y. Liu, Y. Zhao and X. G. Lei, J. Nutr., 2009, 139, 1061–1066 CrossRef CAS PubMed.
- M. Briens, Y. Mercier, F. Rouffineau, V. Vacchina and P. A. Geraert, Br. J. Nutr., 2013, 110, 617–624 CrossRef CAS PubMed.
- K. Balogh, M. Weber, M. Erdélyi and M. Mezes, Acta Vet. Hung., 2004, 52, 403–411 CrossRef CAS PubMed.
- X. Peng, Y. Cui, W. Cui, J. Deng and H. Cui, Biol. Trace Elem. Res., 2009, 131, 33–42 CrossRef CAS PubMed.
- J. G. Li, J. C. Zhou, H. Zhao, X. G. Lei, X. J. Xia, G. Gao and K. N. Wang, Meat Sci., 2011, 87, 95–100 CrossRef CAS PubMed.
- H. Steinbrenner, Free Radicals Biol. Med., 2013, 65, 1538–1547 CrossRef CAS PubMed.
- C. Wang, S. Yang, N. Zhang, Y. Mu, H. Ren, Y. Wang and K. Li, PLoS One, 2014, 9, e101315 Search PubMed.
- M. Laclaustra, S. Stranges, A. Navas-Acien, J. M. Ordovas and E. Guallar, Atherosclerosis, 2010, 210, 643–648 CrossRef CAS PubMed.
- B. Speckmann, H. J. Bidmon, A. Pinto, M. Anlauf, H. Sies and H. Steinbrenner, J. Biol. Chem., 2011, 286, 10764–10772 CrossRef CAS PubMed.
- V. M. Labunskyy, B. C. Lee, D. E. Handy, J. Loscalzo, D. L. Hatfield and V. N. Gladyshev, Antioxid. Redox Signaling, 2011, 14, 2327–2336 CrossRef CAS PubMed.
- Q. A. Sun, Y. Wu, F. Zappacosta, K. T. Jeang, B. J. Lee, D. L. Hatfield and V. N. Gladyshev, J. Biol. Chem., 1999, 274, 24522–24530 CrossRef CAS PubMed.
- Y. Horibata and Y. Hirabayashi, J. Lipid Res., 2007, 48, 503–508 CrossRef CAS PubMed.
- J. Zhang, Z. W. Zhang, A. S. Shan and S. W. Xu, Biol. Trace Elem. Res., 2014, 157, 234–241 CrossRef CAS PubMed.
- P. Castets, A. Lescure, P. Guicheney and V. Allamand, J. Mol. Med., 2012, 90, 1095–1107 CrossRef CAS PubMed.
- M. Dudkiewicz, T. Szczepinska, M. Grynberg and K. Pawlowski, PloS One, 2012, 7(2), e32138 CAS.
- D. W. Jeong, T. S. Kim, Y. W. Chung, B. J. Lee and I. Y. Kim, FEBS Lett., 2002, 517, 225–228 CrossRef CAS PubMed.
- C. Lu, F. Qiu, H. Zhou, Y. Peng, W. Hao, J. Xu, J. Yuan, S. Wang, B. Qiang and C. Xu, FEBS Lett., 2006, 580, 5189–5197 CrossRef CAS PubMed.
- M. A. Reeves, F. P. Bellinger and M. J. Berry, Antioxid. Redox Signaling, 2010, 12, 809–818 CrossRef CAS PubMed.
- X. Xu, B. Carlson, R. Irons, H. Mix, N. Zhong, V. Gladyshev and D. Hatfield, J. Biol. Chem., 2007, 404, 115–120 CAS.
- M. Roman, P. Jitaru and C. Barbante, Metallomics, 2014, 6, 25–54 RSC.
- S. Lenzen, J. Drinkgern and M. Tiedge, Free Radicals Biol. Med., 1996, 20, 463–466 CrossRef CAS PubMed.
- M. Tiedge, S. Lortz, J. Drinkgern and S. Lenzen, Diabetes, 1997, 46, 1733–1742 CrossRef CAS PubMed.
- A. Bast, G. Wolf, I. Oberbäumer and R. Walther, Diabetologia, 2002, 45, 867–876 CrossRef CAS PubMed.
- M. C. Medina, J. Molina, Y. Gadea, A. Fachado, M. Murillo, G. Simovic, A. Pileggi, A. Hernández, H. Edlund and A. C. Bianco, Endocrinology, 2011, 152, 3717–3727 CrossRef CAS PubMed.
- J. Mao and W. Teng, Nutrients, 2013, 5, 1937–1948 CrossRef CAS PubMed.
- R. A. Sunde and A. M. Raines, Adv. Nutr., 2011, 2, 138–150 CrossRef CAS PubMed.
- N. J. Sund, M. Z. Vatamaniuk, M. Casey, S. L. Ang, M. A. Magnuson, D. A. Stoffers, F. M. Matschinsky and K. H. Kaestner, Genes Dev., 2001, 15, 1706–1715 CrossRef CAS PubMed.
- C. S. Lee, N. J. Sund, M. Z. Vatamaniuk, F. M. Matschinsky, D. A. Stoffers and K. H. Kaestner, Diabetes, 2002, 51, 2546–2551 CrossRef CAS PubMed.
- E. P. Araujo, M. E. Amaral, C. T. Souza, S. Bordin, F. Ferreira, M. J. Saad, A. C. Boschero, E. C. Magalhães and L. A. Velloso, FEBS Lett., 2002, 531, 437–442 CrossRef CAS PubMed.
- S. R. Stapleton, Cell. Mol. Life Sci., 2000, 57, 1874–1879 CrossRef CAS PubMed.
- R. F. Burk, K. E. Hill and A. K. Motley, J. Nutr., 2003, 133, 1517S–1520S CAS.
- S. S. Im, S. Y. Kim, H. I. Kim and Y. H. Ahn, Curr. Diabetes Rev., 2006, 2, 11–18 CrossRef CAS PubMed.
- G. Q. Jiang and B. B. Zhang, Am. J. Physiol.: Endocrinol. Metab., 2003, 284, E671–E678 CrossRef CAS PubMed.
- H. Steinbrenner, A. L. Hotze, B. Speckmann, A. Pinto, H. Sies, M. Schott, M. Ehlers, W. A. Scherbaum and S. Schinner, J. Mol. Endocrinol., 2013, 50, 31–42 CrossRef CAS PubMed.
- M. Osanai, H. Chiba, T. Kojima, M. Fujibe, K. Kuwahara, H. Kimura, M. Satoh and N. Sawada, Jpn. J. Cancer Res., 2002, 93, 532–541 CrossRef CAS PubMed.
- B. Speckmann, P. L. Walter, L. Alili, R. Reinehr, H. Sies, L. O. Klotz and H. Steinbrenner, Hepatology, 2008, 48, 1998–2006 CrossRef CAS PubMed.
- S. MilinkovićTur, J. Aladrović, B. B. Ljubić and N. PoljičakMilas, Vet. Arh., 2009, 79, 481–489 Search PubMed.
- S. Ghazi Harsini, M. Habibiyan, M. M. Moeini and A. R. Abdolmohammadi, Biol. Trace Elem. Res., 2012, 148, 322–330 CrossRef CAS PubMed.
Footnote |
† Electronic supplementary information (ESI) available. See DOI: 10.1039/c6ra14932d |
|
This journal is © The Royal Society of Chemistry 2016 |