DOI:
10.1039/D4TB00887A
(Review Article)
J. Mater. Chem. B, 2024,
12, 8007-8032
Hydrogels: a promising therapeutic platform for inflammatory skin diseases treatment
Received
25th April 2024
, Accepted 13th July 2024
First published on 24th July 2024
Abstract
Inflammatory skin diseases, such as psoriasis and atopic dermatitis, pose significant health challenges due to their long-lasting nature, potential for serious complications, and significant health risks, which requires treatments that are both effective and exhibit minimal side effects. Hydrogels offer an innovative solution due to their biocompatibility, tunability, controlled drug delivery capabilities, enhanced treatment adherence and minimized side effects risk. This review explores the mechanisms that guide the design of hydrogel therapeutic platforms from multiple perspectives, focusing on the components of hydrogels, their adjustable physical and chemical properties, and their interactions with cells and drugs to underscore their clinical potential. We also examine various therapeutic agents for psoriasis and atopic dermatitis that can be integrated into hydrogels, including traditional drugs, novel compounds targeting oxidative stress, small molecule drugs, biologics, and emerging therapies, offering insights into their mechanisms and advantages. Additionally, we review clinical trial data to evaluate the effectiveness and safety of hydrogel-based treatments in managing psoriasis and atopic dermatitis under complex disease conditions. Lastly, we discuss the current challenges and future opportunities for hydrogel therapeutics in treating psoriasis and atopic dermatitis, such as improving skin barrier penetration and developing multifunctional hydrogels, and highlight emerging opportunities to enhance long-term safety and stability.
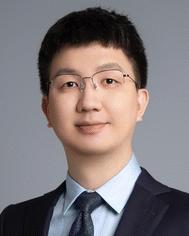
Yiliang Lin
| Dr Yiliang Lin currently holds the position of Assistant Professor in the Department of Chemical and Biomolecular Engineering at the National University of Singapore. He completed his PhD in Chemical Engineering at North Carolina State University in 2018, followed by a postdoctoral research stint at the University of Chicago. In November 2023, Dr Lin joined the National University of Singapore and founded his research group. His team is dedicated to the innovative design and development of soft materials and living materials, with a focus on applications in human–machine interfaces, biomedical therapies, and sustainability initiatives. |
1 Introduction
Human skin is a complex, multi-functional organ that is essential for maintaining systemic homeostasis. Primarily, the epidermal barrier serves as the first line of defense against exogenous threats, such as microbial and physical agents, and it also regulates water loss. Additionally, the skin plays an active role in immune responses by releasing cytokines and chemokines, acting as a sentinel organ to alert immune cells in the event of skin damage or infection.1 Skin and subcutaneous disorders have become significant health challenges, ranking as the fourth most prevalent cause of non-fatal health issues.2 In 2013, skin conditions accounted for 1.79% of the global burden of disease, measured in disability-adjusted life years from 306 diseases and injuries.2 The increasing prevalence of dermatological conditions and the associated healthcare costs for treatment, along with the impact on patients' quality of life, have become increasingly significant issues for global health.3,4
Among various skin conditions, inflammatory skin diseases, such as psoriasis5 and atopic dermatitis (AD),6 have garnered significant attention due to their prolonged course, serious complications, and major health risks. These critical conditions profoundly impact both individual and public health, significantly influencing clinical practices and the focus of biomedical research.3 The chronic visibility and potential stigma associated with these diseases often lead to substantial psychological distress, increasing the likelihood of depression and anxiety. The continuous itch and pain typical of these conditions can severely disrupt daily life, affecting work performance and social interactions. The consequences also impact the families of patients, who may experience considerable emotional and financial stress due to ongoing treatment and care responsibilities. Moreover, psoriasis and AD are associated with numerous comorbidities, including cardiovascular issues in psoriasis and the increased infection risk in AD, further intensifying the overall health burden.7,8
The primary goal of treating psoriasis and AD is to reduce the presence of skin lesions, minimize symptom flare-ups, and alleviate the overall burden of symptoms. For mild to moderate cases, topical treatments are typically employed, and for more severe cases, systemic therapies are considered due to their potential for greater adverse events.9 Consequently, topical therapy remains a cornerstone in managing these conditions due to its benefits, including ease of application and a lower risk of gastrointestinal irritation or systemic side effects.10 Nonetheless, conventional topical treatments, like vitamin D derivatives for psoriasis, corticosteroids, and calcineurin inhibitors for both conditions,11–13 may not always provide optimal effectiveness, due to the limited drug penetration14 and safety concerns related to prolonged use.15,16
Hydrogels are showing significant promise in improving psoriasis and AD topical therapy by minimizing systemic adverse effects. Their high biocompatibility, capability to encapsulate and release therapeutic agents, and high water content similar to natural tissues make them particularly suitable for this use.12–14 Hydrogels consist of water-rich, crosslinked hydrophilic polymer networks, and can be engineered specifically for topical treatment of psoriasis and AD, incorporating several key characteristics (Fig. 1): (1) excellent biocompatibility with extracellular matrix mimicking properties; (2) tunable porosity and degradability to modulate cellular behavior and control drug delivery; (3) adjustable stiffness and viscoelasticity to regulate cell functionalities; (4) customizable adhesiveness through specific physical interactions and chemical linking; (5) effective moisture retention with inherent therapeutic activity, essential for skin barrier repair – a fundamental non-pharmacological approach for psoriasis and AD;17 (6) enhanced drug-loading capacity and efficacy with reduced toxicity, attributed to their ability to prevent drug leakage and degradation, thereby ensuring controlled release of a wide range of therapeutic compounds, including chemodrugs, proteins, siRNA, and plasmids under mild conditions.18,19
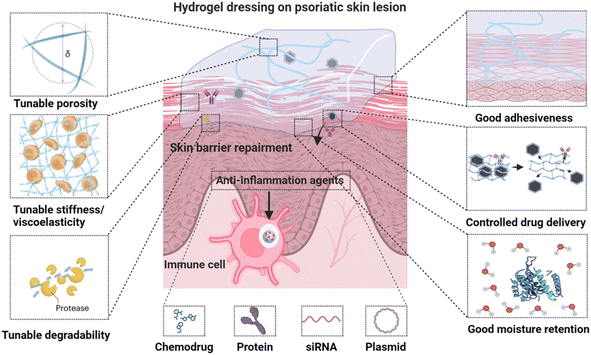 |
| Fig. 1 Distinctive characteristics of hydrogels as a topical therapeutic platform for inflammatory skin diseases. Hydrogels feature tunable porosity, tunable stiffness/viscoelasticity, tunable degradability, excellent adhesiveness, controlled drug delivery ability, and moisturizing capability, collectively to achieve skin barrier repair. Additionally, hydrogels can be utilized as drug delivery platforms, particularly as nanocarriers, to deliver therapeutic agents directly to inflammatory sites, either extracellularly or intracellularly. These features make hydrogels a highly effective option for the localized treatment of conditions like psoriasis and atopic dermatitis. The figure is made with BioRender (https://biorender.com/). | |
This review examines the components of hydrogels, their adjustable properties, the therapeutic agents that can be loaded into them, and the challenges associated with their application. It delves into how these elements combine to make hydrogels a versatile and promising option for targeted treatment strategies in medical applications, particularly for skin-related therapies.
2 Overview of psoriasis and atopic dermatitis
2.1 Pathogenesis and treatment goals of psoriasis and atopic dermatitis
Psoriasis is a prevalent chronic inflammatory skin disease characterized by scaly, erythematous plaques, often accompanied by systemic symptoms, which affects approximately 2–4% of the global population.5,20 Psoriasis is typically associated with multiple comorbidities, significantly affecting patients’ quality of life. Specifically, individuals with psoriasis are more than three times as likely to suffer from depression and anxiety, primarily due to the pruritic, painful, and socially stigmatizing nature of the skin lesions.21 Chronic plaque or psoriasis vulgaris, results from a combination of genetic susceptibility and environmental triggers such as streptococcal infection, stress, smoking, obesity, and alcohol consumption.
Histologically, psoriasis vulgaris is characterized by several distinctive features: the thickening of the epidermis, known as acanthosis, with downward elongation of the rete ridges; a thinned or absent granular layer; elongated and dilated capillaries; and suprapapillary thinning. Additionally, there is a notable presence of T cells infiltrating in both dermis and epidermis, and sometimes clusters of neutrophil in the parakeratotic scale.22 The central mechanisms of psoriasis involve the crosstalk between the innate and adaptive immune systems (known as feed-forward amplification of psoriasis inflammation), the critical role played by interleukin (IL)-23 and the responses of helper T cell type 17 (Th17),23 and the contribution from the tumor necrosis factor (TNF)-α through keratinocyte activation (Fig. 2).24 Treatment strategies for plaque psoriasis are typically tailored based on the severity of the psoriasis, psoriatic arthritis, other related health condition, and patient's preference and satisfaction.
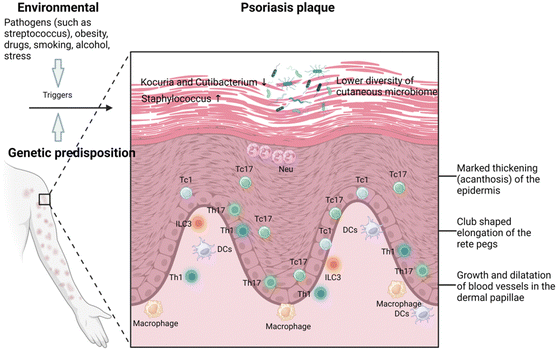 |
| Fig. 2 Pathogenesis and pathophysiology of psoriasis plaques. The development of a psoriasis plaque involves the participation of plasmacytoid dendritic cells and type I interferons, which lead to a marked thickening of the epidermis, known as acanthosis, club-shaped elongation of the rete pegs, and growth and dilatation of blood vessels in the dermal papillae. Various immune cell subsets, including IL-17 secreting group 3 innate lymphoid and Th17 and Tc17 cells, IFN-γ secreting Th1 and Tc1 cells, and neutrophils are drawn into the skin, along with activated macrophages and dendritic cells. Through the involvement of skin draining lymph nodes, the initial immune response creates a self-sustaining cycle of inflammation, maintaining disease activity. DC: dendritic cell. ILC: innate lymphoid cell. Neu: neutrophil. Tc: cytotoxic T cell. Th: helper T cell. The figure is made with BioRender (https://biorender.com/). | |
Atopic dermatitis (AD) is another common chronic pruritic inflammatory skin disease25 affecting around 15–30% of children and approximately 2–10% of adults.26,27 It is characterized by inflammation, impaired skin barrier function, and dysbiosis, leading to pruritus and the formation of eczematous areas.28,29 AD is generally influenced by both environmental and genetic factors. The impaired terminal differentiation of keratinocytes leads to barrier dysfunction, allowing the penetration of cutaneous antigens that initiate AD.30 AD significantly impacts the quality of life for both patients and their families and increases the risk of developing allergic comorbidities.31
In AD, epidermal barrier dysfunction is characterized by the reduced expression of terminal differentiation markers, an increased permeability defect caused by skin lipid film impairment, and the increased trans-epidermal water loss.32 This dysfunction makes AD-affected skin more susceptible to the invasion of external agents that are harmful to keratinocytes.33 When these agents invade, damaged keratinocytes release epidermal alarmins, such as IL-33, IL-25, and thymic stromal lymphopoietin (TSLP), which subsequently activates dendritic cells (DCs) and type 2 innate lymphoid cells (ILC2s).34,35 These cells, in turn, produce IL-5 and IL-13, thereby leading to the activation of eosinophils and Th2 cells. This cycle of local Th2 polarization further impairs the skin barrier and sustains itching, thereby exacerbating skin barrier dysfunction and promoting dysbiosis.36 Targeting these pathways by blocking IL-4, IL-13,37 IL-3138 and inhibiting Janus kinase activity39 has been effectively shown to improve the outcome of AD patients.
In the skin affected by AD, microbial diversity typically decreases significantly. Predominantly, pathogenic Staphylococcus aureus, together with Candida and Trichophyton, become prevalent and play a key role in the progression of AD.40,41 An associated complication is the deficiency of the sphingolipids and antimicrobial peptides in diseased skins, as these two components constitute the epidermal permeability barrier (EPB), providing a broad-spectrum defense against multiple pathogens.42 The complexity of this disease (as illustrated in Fig. 3) suggests that a multifaceted approach targeting various aspects of the immune response and skin microbiome dysbiosis may be beneficial.43
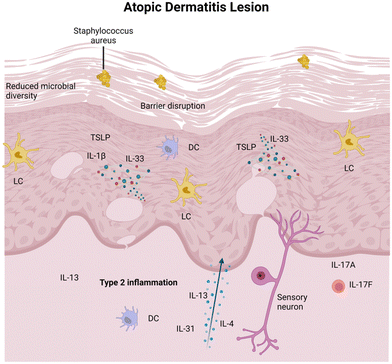 |
| Fig. 3 Pathogenesis and pathophysiology of atopic dermatitis. In lesional AD skin, key immune cells such as Langerhans cells, dermal dendritic cells, and inflammatory epidermal dendritic cells (bearing specific IgE bound to the high-affinity receptor for IgE), play a crucial role by taking up allergens and antigens. The type-2 cytokines IL-4, IL-13, and IL-31 directly activate sensory nerves, which promotes pruritus, a hallmark symptom of AD. As the condition becomes more chronic, there is a progressive increase of keratinocyte-derived and Th-cell-derived cytokines, further exacerbating the inflammatory response and symptom severity in affected skin areas. DC = dendritic cell. IL = interleukin. TSLP = thymic stromal lymphopoietin. The figure is made with BioRender (https://biorender.com/). | |
2.2 Rationale for topical hydrogels in psoriasis and AD
All psoriasis and AD patients benefit from the liberal application of emollients. Hydrogel, as an emollient, offers several advantages: it improves localized drug delivery, enhances skin barrier repair, and reduces inflammation, specifically targeting the pathology of inflammatory skin lesions. By creating a moist environment, hydrogels promote healing, reduce trans-epidermal water loss, and prevent the penetration of harmful agents. They can deliver anti-inflammatory and immunomodulatory drugs directly to affected areas, minimizing the release of alarmins and subsequent inflammation. Moreover, hydrogels can restore microbial diversity and reduce pathogenic microorganisms by incorporating antimicrobial peptides or sphingolipids. They also provide immediate relief from itching and discomfort, helping to break the cycle of scratching and further skin damage.
3 The properties of hydrogels and their effects on topical therapy
Hydrogels, synthesized through physical or chemical crosslinking of polymers, exhibit a remarkable ability to take up and retain fluids, with absorption capacities typically ranging from 70–99%. This notable feature is largely attributed to their porous matrix and hydrophilic 3D network, which allows hydrogels to closely mimic the physical attributes of biological tissues.44 Emulating the biomimetic characteristics of the natural extracellular matrix (ECM), hydrogels play a critical role in skin barrier repair.45 They replicate the soft, hydrated environment of the ECM through their 3D porous hydrophilic polymer networks and significant hydrophilic capacity. This structural similarity enhances cellular activities and creates an optimal microenvironment for the physiological processes of resident skin cells, highlighting their significance in dermatological interventions.46 The versatility of hydrogels extends to a wide range of tunable properties that are critical for therapeutic applications in inflammatory skin diseases. These properties include the sources of the polymers used, adhesive properties, occlusivity, mechanical stiffness, viscoelasticity, porosity, micro/nanostructure and degradation kinetics. Additionally, the interactions between hydrogels and skin or hydrogels and drugs play a critical role in the therapeutic effect of hydrogels in inflammatory skin disease treatment. By exploring and optimizing these properties, hydrogels can be tailored to effectively address the complex needs for inflammatory skin diseases treatment, making them a powerful and adaptable tool in dermatological therapeutics.
3.1 Hydrogels components
Hydrogels for inflammatory skin diseases can be generally classified into three main types based on their origins: natural, synthetic and hybrid hydrogels (Fig. 4).47 Natural biopolymeric hydrogels, typically composed of natural polymers like alginate and gelatin, are valued for their biocompatibility and biodegradability, aligning with the principles of regenerative medicine. Meanwhile, synthetic polymeric hydrogels, made from materials such as polyvinyl alcohol and polyacrylic acid, offer enhanced mechanical strength and adjustable drug release mechanisms, fulfilling a wide range of pharmacotherapeutic demands. Hybrid hydrogels, which incorporate the advantage of natural and synthetic polymers, exhibit a diverse compositional framework that highlights their transformative potential in the therapeutic management of inflammatory skin disorders.48
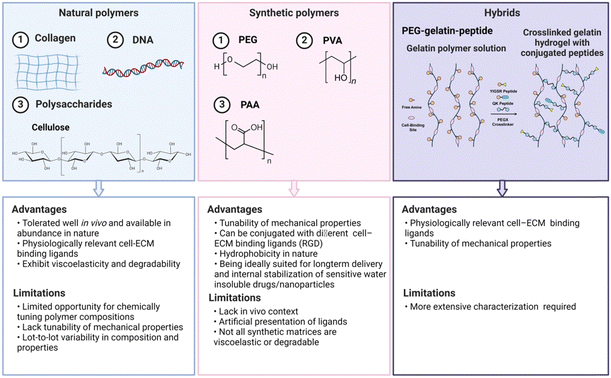 |
| Fig. 4 Classification, advantages, and limitations of hydrogels from different polymer sources, including natural polymers, synthetic polymers and hybrids. The figure is made with BioRender (https://biorender.com/). | |
3.1.1 Hydrogels from natural polymer.
Hydrogels synthesized from natural polymers, including collagen, gelatin, polypeptides, DNA, alginate,49 hyaluronic acid, cellulose,50 and chitosan,51 are widely recognized for their high biocompatibility and biodegradability. These natural materials are preferred for many biomedical uses, including tissue engineering and drug delivery systems, because they are derived from substances that are naturally occurring in the body or the environment. This compatibility makes them ideal for integration into biological systems, as they can be broken down by the body's own processes without causing harmful side effects. Moreover, their natural origin often means they interact very well with biological tissues, facilitating effective and safe therapeutic applications.
3.1.1.1 Collagen.
Collagen is a fibrous protein consisting of three polypeptide chains twined around one another, acting as a natural scaffolding in the human body.52 Primarily sourced from animal tissues like skin and cartilage, collagen is renowned for its excellent biocompatibility and biodegradability, making it highly suitable for biomedical applications.53 In the context of skin diseases, hydrogels incorporating collagen are particularly valuable due to their robust mechanical properties, essential for accommodating movement and stretching. Moreover, the abundant functional groups on collagen's side chains allow for easy modification and multifunctionalization, enhancing its utility in various therapeutic applications.54 Additionally, collagen is pivotal in wound healing as a hemostatic agent, being a primary activator for platelet response immediately after an injury.55
Collagen can spontaneously transform into hydrogels via pH and temperature-dependent self-fibrogenesis processes under physiological conditions, which is beneficial for ease of use in clinical settings. Moreover, collagen fibrils contain cell adherence peptide ligands such as collagen triple-helical ligands and arginine-glycine-aspartic acid (RGD), which can bind to cell membrane receptors, such as α1β1 and α2β1 integrins. This binding promotes rapid cellular growth and migration within the hydrogel matrices, and ensures sustained drug release, offering a comprehensive approach to skin therapy.56
In specific applications like wound healing, collagen-enriched hydrogels provide significant benefits. For instance, studies on antibiotic-loaded silica nanoparticles/collagen composite hydrogels have shown that collagen helps maintain optimal hydration levels in the wound bed, crucial for effective healing. It also facilitates cell colonization and remodeling into neotissue due to its biocompatible, biodegradable, and hemostatic properties. Additionally, the fibrillar structure of collagen hydrogels supports keratinocyte and fibroblast migration, essential for effective wound repair. These multifunctional properties make collagen a cornerstone material in the design of hydrogels for dermatological and broader biomedical applications.57
3.1.1.2 Gelatin.
Gelatin, a natural polymer derived from Collagen I found in animal skins, bones, and tendons, stands out due to its ready availability and cost-effectiveness, positioning it as a polymer with significant commercial potential. Its high biocompatibility and biodegradability, coupled with non-toxic byproducts upon degradation, make gelatin an excellent candidate for hydrogel for medical applications.58,59 Specifically, Gelatin can be biodegraded by cell-derived enzymes (e.g., matrix metalloproteinases (MMPs)), facilitating cell-mediated stroma remodeling via ECM protein deposition and cell contraction force-induced network rearrangement. Furthermore, in the realm of biomedical hydrogels, where multifunctionality is crucial, the amino acid residues on the gelatin chain provide abundant sites for chemical modification.60 With molecular composition identical to collagen, gelatin is a preferred substitute for various applications due to its biocompatible, biodegradable, non-immunogenic properties, and its natural abundance.61 In addition, gelatin contains the cell-binding motif arginylglycylaspartic acid, enhancing cell encapsulation and growth factor delivery within gelatin-based hydrogels.62 This is particularly beneficial for the treating skin inflammatory diseases, where growth factors and antibodies are proving to be effective therapeutic agents.63
Moreover, gelatin improves the hydrogel's mechanical properties, increasing their elasticity and durability, which is critical for maintaining the integrity of the wound dressing during the healing process. The biodegradability of gelatin allows the hydrogel to gradually degrade, reducing the frequency of dressing changes and minimizing disturbance to the healing wound. An example of its application is the use of a gelatin-based hydrogel film for treating AD, where the gelatin composite reduces the hydrophilicity of the film, enhancing the stability of the hydrogel and improving drug entrapment efficiency through interactions with other compounds, such as 1,4-anhydro-4-seleno-D-talitol.64 This multifaceted functionality makes gelatin a valuable component in the design and implementation of advanced therapeutic hydrogels.
3.1.1.3 Polypeptides and DNA.
Polypeptides are a class of polymers composed of amino acid monomers and their derivatives linked together by peptide bonds. Similar to proteins, peptides possess stable secondary structures (α-helix or β-sheet),65 which for the design of responsive hydrogels with novel structures and functionalities, offering advantages over synthetic polymers.66 Additionally, amino acids, such as glutamic acid and lysine, which constitute polypeptides, can be readily functionalized to achieve specific functionalities to the hydrogels.67 Polypeptide-based hydrogels are characterized by their nontoxicity, biodegradability, and low immunogenicity as well as easy modification,68 making them extremely suitable for biomedical applications.69 For example, a polypeptide-based hydrogel crosslinked by 6-arm polyethylene glycol-amide succinimidyl glutarate has demonstrated antibacterial properties and cell adhesive behaviors, making it a promising option for facilitating wound healing processes. This is particularly relevant for AD patients, who often suffer from increased susceptibility to microbial skin infections due to impaired expression of antimicrobial peptides (AMP).70
Similarly, DNA (deoxyribonucleic acid) is a fundamental biomolecule serving as a block copolymer and polyanion that harbors essential genetic information for nearly all organisms. Its structural units consist of nucleotides, each comprising three molecular components: a nucleobase (cytosine [C], guanine [G], adenine [A], or thymine [T]), a deoxyribose sugar, and a phosphate group,71 linked together by phosphodiester bonds. DNA's susceptibility to specific enzymatic cleavage by restriction endonucleases and DNA ligases makes it uniquely amenable to precise modifications and functionalization compared to synthetic polymers.72 Utilizing these specific enzyme cleavage sites within DNA polymers has led to the development of various enzyme-assisted or enzyme-triggered DNA hydrogels. For instance, DNA hydrogels have been utilized for the co-delivery of small-molecule drugs and macromolecular biologics in cancer photoimmunotherapy.73,74 By incorporating photosensitizers directly onto the DNA backbone via predesigned phosphorothioate modification sites, it's possible to assemble a DNA tetrahedron framework efficiently. This framework can encapsulate programmed death ligand-1 (PD-L1) small interfering RNA (siRNA), creating a synergistic nanogel that enhances the efficacy of therapeutic interventions.
3.1.1.4 Alginate.
Alginic acid is a linear polymer composed of D-mannuronic acid and L-guluronic acid residues, arranged in blocks within the polymer chain, and can be cross-linked by divalent cations like Ca2+.75 Alginates, derived from brown seaweed, are natural polysaccharide polymers known for their exceptional biocompatibility, hydrophilicity, and low immunogenicity, making them highly suitable for treating skin diseases.76 Moreover, the gentle gelling behavior is beneficial for encapsulating a variety of substances without causing significant tissue trauma.77 Hydrogels based on alginates are effectively used in designing skin drug delivery systems. For example, an alginate solution was mixed with ceria nanoparticles (CeNPs), CaCO3 microparticles, and glucono-δ-lactone (GDL) to form CeNP-loaded, Ca2+-cross-linked hydrogel for the treatment of AD. This therapeutic hydrogel, embedded with reactive oxygen species (ROS)-scavenging CeNPs, exhibited cytoprotective effects in highly oxidative conditions.49 Mouse model studies of AD showed this approach resulted in reduced epidermal thickness and lowered levels of AD-associated immunological biomarkers, pointing to enhanced therapeutic outcomes. In another study, incorporating alginate into hydrogels significantly boosted skin moisturization by aiding water retention, which is vital for the dry and compromised skin characteristic of AD. Alginate formed a semi-interpenetrating network with polyvinyl alcohol within the hydrogel, ensuring its prolonged adherence to the skin. Moreover, the mildly crosslinked nature of alginate facilitated the controlled release of prednisolone, a corticosteroid, thereby providing sustained anti-inflammatory effects.78 This versatility and efficacy underline the potential of alginate-based hydrogels in advanced dermatological treatments.
3.1.1.5 Hyaluronic acid (HA).
Hyaluronic acid (HA) is a type of glycosaminoglycan composed of alternating units of N-acetyl-D-glucosamine and glucuronic acid.79 Hyaluronic acid is primarily extracted from animal tissues,80 and it can be crosslinked through various physical and chemical methods to create hydrogels.81 Hyaluronic acid molecules are rich in carboxyl and hydroxyl groups that form intra- and intermolecular hydrogen bonds in aqueous solutions, providing strong hydrating properties. These characteristics make hyaluronic acid a popular choice in the treatment of skin diseases, particularly for its ability to facilitate tissue repair by promoting the migration and differentiation of mesenchymal and epithelial cells.82 Furthermore, hyaluronic acid hydrogel also form exhibits collagen-stimulating properties, thereby promoting collagen synthesis in the skin and supporting tissue regeneration.83 The efficacy of HA is augmented by its interaction with cells that express the CD44 receptor, which plays a significant role in cell proliferation, migration, and differentiation. This interaction is fundamental to the development of HA-based hydrogels, which have demonstrated potential in modulating cell behavior, including inducing the spontaneous polarization of M2-like monocyte/macrophage through CD44-mediated STAT3 activation in THP-1 cells.84
In topical drug delivery, HA-based hydrogels offer significant benefits, particularly for treating inflamed psoriatic skin. For instance, HA-modified ethosomes (HA-ES) are an effective delivery vehicle for curcumin, a poorly water-soluble drug. HA enhances the formation of a gel network on the surface of HA-ES, minimizing drug leakage and controlling the release of curcumin.85 In another study, HA is employed as a matrix in nanocrystal-based hydrogel (NC-gel) for the delivery of therapeutic agents like indirubin. These formulations target the overexpressed CD44 protein in psoriatic inflamed tissues, thereby improving the efficacy of topical treatments.86 The utilization of HA not only enhances the bioavailability of drugs but also leverages its intrinsic properties to mitigate inflammatory responses, exemplifying its unique advantages in hydrogel-based therapeutic applications.
3.1.1.6 Cellulose.
Cellulose is a linear polysaccharide polymer composed of D-anhydroglucopyranose units (AGU) linkded by β-1,4 glycosidic bonds, forming a chain with a 180° alternating steering structure.87 Within the cellulose macromolecular chain, each AGU unit contains three reactive hydroxyl groups (–OH), making them prime sites for various chemical modifications. As the most abundant biomass resource on Earth, cellulose is derived from a wide range of sources, including wood, bamboo, cotton, hemp, agricultural by-products as well as sea squirts, periphyton, algae and bacteria.88 Its renewability, biodegradability, biocompatibility, high strength, non-toxicity and low-cost make cellulose an invaluable natural polymer for hydrogel networks.89 Furthermore, cellulose hydrogels stand out for their multi-scale design (macro, micron, nano and molecular sizes), customizable high mechanical strength, excellent thermal stability, and efficient water retention. However, cellulose itself is poorly soluble due to inter- and intra-molecular hydrogen bonding and van der Waals forces, so it is mostly used in derivative forms in hydrogels. These hydrogels play a crucial role as excipients in pharmaceutical formulations, helping to modulate drug release kinetics to maintain optimal drug concentrations.90
In particular, one type of cellulose, nanocellulose (NC), offers significant enhancements in mechanical strength and surface area due to its nanometric scale, promoting interaction with biological tissues. The abundance of hydroxyl groups and a negatively charged surface on NC facilitate tissue adhesion through electrostatic interactions. Its natural abundance and modifiability make NC an excellent candidate for developing bespoke hydrogels for managing inflammatory skin diseases.91 For instance, hydrogels based on cellulose nanocrystals (CNCs) provide a strategy for treating AD by forming film-like hydrogel barriers that conform directly to the skin, mitigating the compromised barrier function inherent in AD and retaining the moisture. This is critical for relieving the common symptoms and dryness associated with AD. Incorporating CNCs with natural polymers like alginate or pectin enables the encapsulation of therapeutic agents such as betamethasone dipropionate, enhancing the hydrogel's functionality as a drug delivery system, demonstrated effectively in vitro on both synthetic and porcine skin models.92 Additionally, hydrogels based on cellulose nanofiber (CNFs) are used for the controlled release or topical delivery of drugs. These hydrogels can also be labeled with technetium-99m for tracking and monitoring drug delivery in vivo over time, while the CNFs can facilitate the local application of metabolic enzymes to break down into nutrients such as glucose.93
Moreover, cellulose, particularly bacterial cellulose (BC), when integrated into hydrogels for skin wound treatment, offers additional advantages. BC-based hydrogels loaded with silver nanoparticles (AgNP) produced via a green synthesis method show significant antimicrobial activity against common pathogens, including Staphylococcus aureus, Pseudomonas aeruginosa, and Candida auris, which are often implicated in chronic wound infections. The incorporation of cellulose into hydrogels not only enhances their suitability for wound dressing by creating a moist healing environment and delivering antimicrobial agents directly to the wound site but also allows for non-invasive monitoring of the healing process due to their transparent nature.94
3.1.2 Synthetic hydrogels.
Unlike natural hydrogels, which often have properties limited by their biological origins such as a weak mechanical strength, synthetic hydrogels offer unparalleled tunability in both chemical and physical dimensions. This flexibility is achieved through the selection of monomers, precise adjustment of cross-linking agents' types and ratios, and manipulation of polymer chain lengths during the fabrication process.95,96 These steps enable the engineering of hydrogels with specific, tailored functionalities. For example, the mechanical properties of synthetic hydrogels, such as tensile strength and elasticity, can be finely controlled by managing the cross-link density within the polymer matrix. This allows for the creation of hydrogels that can precisely match the mechanical properties of different types of tissue, accommodating various moduli. This level of control makes synthetic hydrogels particularly valuable for diverse applications, including those in biomedical fields where matching the mechanical properties to the surrounding or interfacing tissues is crucial.97,98
3.1.2.1 Polyethylene glycol (PEG).
Polyethylene glycol (PEG) is an inexpensive water-soluble linear ether polymer composed of repeating glycol units [–(CH2CH2O)n]. It is synthesized through the ring-opening polymerization of ethylene oxide reactive anions with a hydroxyl initiator and can be produced in molecular weights ranging from 0.4 to 100 kDa.99 Despite PEG's simple linear structure with only two terminal hydroxyl groups, which limits direct functional modification, it has spurred the development of a variety of derivative products with varying degrees of polymerization and activated functional groups to enhance its utility.
PEG shows high hydration capacity, structural flexibility, biocompatibility, and amphiphilicity, and devoid of any steric hindrances at the molecular level.100 These properties make it highly suitable for forming hydrogels through methods like radiation cross-linking,101 free radical polymerization of its acrylate derivatives,102 and specific chemical reactions such as Michael-type addition and click chemistry and condensation.103 PEG-based hydrogels are known for their non-toxic properties, biocompatibility, low immune response, tailored degradation rates, and ability to sustain drug release, making them ideal for biomedical applications, especially in drug delivery systems that require precise release kinetics104,105 Their effectiveness and tolerance in dermatological contexts highlight their value, offering substantial benefits for managing sensitive skin conditions and enabling extended treatment regimens. The unique properties of PEG allow it to form hydrogels that can efficiently deliver drugs at controlled rates, which is essential for treating chronic skin diseases effectively and minimizing the frequency of application, thereby enhancing patient compliance and overall treatment success.106
Incorporation of PEG into hydrogels significantly enhances the treatment of psoriasis by improving the solubilization of tacrolimus (TAC), a hydrophobic immunosuppressive drug. This is achieved using nanocarriers made from methoxy poly(ethylene glycol)-hexyl substituted poly(lactic acid) (mPEGhexPLA) as the nanocarriers. This formulation approach not only improves the drug's topical delivery to psoriatic lesions, but also enhances patient-friendly application and ensures superior local bioavailability, compared to traditional paraffin-based ointments. The presence of PEG in the hydrogel facilitates the controlled release of TAC, maintaining a steady anti-inflammatory effect crucial for managing psoriasis. Additionally, this composite hydrogel demonstrates good tolerance and safety profiles, even with repeated topical administration in animal models, demonstrating no signs of immediate toxicity.107 Moreover, the inclusion of PEG in hydrogels has been found to improve the appearance and symptoms of psoriasis by reducing epidermal thickness and suppressing keratinocyte proliferation. These effects are achieved through the down-regulation of Th17 cells and myeloid-derived suppressor cells (MDSCs), crucial in the inflammatory pathways that exacerbate psoriasis.108 PEG moderates these cells' activity, reducing pro-inflammatory cytokines and mediators that contribute to epidermal thickening. Consequently, these actions collectively enhance the therapeutic efficacy of PEG-based treatments in controlling psoriasis progression, establishing it as a valuable component in advanced dermatological therapies.
3.1.2.2 Polyvinyl alcohol (PVA).
Polyvinyl alcohol (PVA) is characterized by its relatively simple chemical structure, which includes multiple pendant hydroxyl groups on a carbon–carbon linked polymer chain.109 Its monomer, vinyl alcohol, is unstable for free-radical polymerization, so PVA is synthesized by free-radical polymerization of vinyl acetate in an alcohol solution instead, followed by partial hydrolysis of the resulting poly(vinyl acetate).110
PVA hydrogels are generally prepared through various crosslinking methods, such as physical, chemical and radiation crosslinking, or by advanced processing techniques like directional freezing, stretching, and spinning. One common physical method involves repeated freezing–thawing cycles, which is widely used to prepare PVA hydrogels due to its effectiveness in enhancing the gel's properties.109 PVA hydrogels are particularly suitable for dermatological applications due to their excellent water solubility, biodegradability, and biocompatibility.111 The freeze–thaw method results in a semi-crystalline structure within the PVA hydrogel, creating a balance between crystalline and amorphous domains that can be finely tuned by adjusting process parameters.112 This balance is crucial for optimizing the chemical stability and mechanical properties of the hydrogels, making them valuable in medical applications where these qualities are essential.113 An example of an innovative application involves enhancing PVA hydrogels with vanillin (V) and fungal-derived carboxymethyl chitosan (FC) through freeze–thawing. This method improves the properties of the hydrogel, making it suitable for skin wound dressing applications. The enhancements include strengthened bonding due to Schiff's base reactions between V and FC, and hydrogen bonding among PVA, FC, and V, which contribute to increased thermal stability.114
PVA-based hydrogels have been engineered to modulate the ROS-mediated inflammatory microenvironment, particularly for treating AD.115 PVA not only shows an efficient ROS scavenging capacity but also forms aryl borate polymers through quaternization reactions with ROS-responsive linkers. This structure is susceptible to cleavage under ROS influence, yielding phenols that actively reduces the concentration of ROS in the tissue. This reduction in ROS is vital for alleviating the oxidative stress that can exacerbate AD conditions. Additionally, the PVA network can be integrated with antibacterial Zn–metal organic framework materials, which release Zn2+ to disrupt bacterial integrity, therefore demonstrating sustained antibacterial activity. These properties offer a promising therapeutic strategy for AD by providing localized control of the inflammatory cutaneous microenvironment to improve the condition effectively.115
3.1.2.3 Polyacrylic acid (PAA).
Polyacrylic acid (PAA) is a polymer synthesized from acrylic acid monomers, featuring a carboxyl group attached to the vinyl group at the end of each repeating unit. PAA hydrogels are typically synthesized by free radical polymerization of acrylic monomers in aqueous solution. The abundance of ionizable carboxyl groups in the PAA molecular chain endows it with super-absorbency and pH-sensitivity and thermal responsiveness.116–118 Additionally, the carboxyl groups facilitate bio-mucosal adhesion and various chemical interactions (e.g., hydrogen bonding, ion–ion, electrostatic, and dipole–ion interactions), enhancing the hydrogel's multi-functionality.119,120 For instance, the formation of hydrogen bonds of PAA with the sialic acid –COOH groups of mucus glycoproteins leads to an increase in viscosity, thereby improving the drug's residence time and bioavailability at the site of application.121
Recognized for their biodegradability, biocompatibility and low toxicity, PAA hydrogels find extensive use in biomedical applications, including products like Carbopol, medical-grade acrylic adhesives. PAA hydrogels, especially in the forms of Carbopol, plays a crucial role in creating hydrogels for topical drug delivery systems. Their significant bioadhesiveness ensures prolonged contact with biological tissues, enhancing local drug concentration at the target site and thereby improving the efficacy of treatments for skin conditions by increasing drug bioavailability and reducing the frequency of application.122 One specific application of Carbopol® 980 is in microemulsion (ME) gel and nanoparticle (NP) gel formulations to deliver oat-derived phytoceramides into the skin's stratum corneum. The incorporation of Carbopol® 980 enhances the formulation's ability to localize ceramides into the upper epidermal layers, concentrating the therapeutic agents in the stratum corneum (SC) where they are most needed, thus improving the skin barrier function and offering targeted delivery of oat ceramides deep into the lipid matrix of the skin.48 Carbopol is also versatile in its ability to integrate various pharmacologically active agents. For instance, in the development of Celastrol Noisome hydrogel (Cel Nio gel) for psoriasis treatment, Carbopol serves as the foundational gel matrix, optimizing the delivery and cutaneous adherence of therapeutic agents.98 Moreover, a variant of PAA polymer, Carbopol 940, has been employed in the design of a self-nano emulsifying drug delivery system, incorporating azelaic acid as a gelling agent. This selection is based on its mechanical properties, such as viscosity and thixotropy, crucial for maintaining the stability and effectiveness of the nano-emulsion within the hydrogel, making it an ideal choice for topical treatments of conditions like atopic dermatitis.123
3.1.3 Hybrid hydrogels.
Hybrid hydrogels represent a unique category that blends the characteristics of both natural and synthetic hydrogels, creating formulations that surpass the limitations of traditional hydrogels. This classification encompasses various combinations, including natural polymer-synthetic polymer hydrogels, gel-inorganic material hybrids, gel-organic material hybrids, and gel-bioactive ingredient hybrids. These hydrogels are synthesized by integrating different building blocks with diverse chemistry (such as natural and synthetic polymers), functionality (like conductive or magnetic properties), and morphology (ranging from nano- to micron-sized particles).124 The primary aim is to engineer hydrogels that meet the demands for high-performance applications by leveraging the strengths of each component to enhance overall multifunctionality, including improved mechanical strength, responsive behaviors, and smart properties.90,95,96
Hybrid hydrogels address the limitations of conventional hydrogels in terms of drug loading and controlled release, as well as mechanical properties, by integrating various materials.125 For example, an alginate–polyacrylamide tough hydrogel was developed as a drug delivery system for the delivery of the corticosteroid triamcinolone acetonide, commonly used for inflammatory skin diseases. The integration of LAPONITE® nanoparticles or poly(lactic-co-glycolic acid) (PLGA) microparticles within this system allows for refined control over the release of triamcinolone acetonide, enhancing the hydrogel's effectiveness.126 In another study, a hybrid hydrogel specifically designed for treating skin wounds incorporates glycyrrhizic acid (GA) to harness its immunoregulatory properties. This GA-based hydrogel comprises interpenetrating polymer networks of self-assembled GA induced by Zn2+ and photo-crosslinked methyl acrylate silk fibroin (SF), resulting in a scaffold that exhibits both superb injectability and mechanical strength. The immunomodulatory capabilities of the hybrid hydrogel directly modulate macrophage responses within the inflammatory microenvironment, reducing the need for additional additives.127
Additionally, a hybrid hydrogel combining gelatin methacryloyl (GelMA) with polydopamine-modified fullerene nanocomposites (C60@PDA) leverages the ROS-scavenging properties for wound healing. The GelMA provides a biocompatible matrix, while C60@PDA introduces antioxidant capabilities. This synergistic combination results in a hydrogel that not only demonstrates enhanced mechanical properties but also exhibits favorable cytocompatibility, hemocompatibility, and antibacterial abilities, crucial for effective wound healing.128 These examples highlight the versatility and potential of hybrid hydrogels in advanced biomedical applications, particularly in improving therapeutic outcomes for various skin conditions.
3.2 Bioadhesion of hydrogel
The effectiveness of topical hydrogels is heavily influenced by their ability to adhere well to the skin, particularly to its complex outer layer, the stratum corneum. Bioadhesive hydrogels emerge as a potent tool in drug delivery and topical therapy, primarily due to their capacity to maintain prolonged and intimate contact with biological tissues, which enhances drug absorption and retention.129 This increased contact time allows for better permeation through the stratum corneum, overcoming the skin's barrier properties and facilitating deeper penetration of active ingredients. Consequently, bioadhesive hydrogels improve the efficacy of topical treatments by ensuring more consistent and targeted delivery of medications to affected areas, thereby enhancing therapeutic outcomes for conditions such as psoriasis and atopic dermatitis. However, the challenge lies in achieving efficient bioadhesion on such intricate and varied surfaces.
Bioadhesion in hydrogels is generally facilitated through two main types of interactions: physical and chemical. Physical interactions include van der Waals forces, hydrogen bonding, and interpenetration, which may offer limited diffusion and entanglement with the tissue, sometimes resulting in less-than-optimal adhesion. On the other hand, chemical interactions, such as covalent bonding, provide stronger adhesion but typically lack the flexibility and reversibility offered by physical interactions. Therefore, designing bioadhesive hydrogels often involves finding a balance between these interactions to ensure both robust and reversible adhesion.130
Several innovative strategies have been explored to enhance the bioadhesive properties of hydrogels. For instance, dopamine has been grafted onto hyaluronic acid to enhance its skin tissue adhesion properties through imide formation or Michael-type reactions between catechol and quinone groups on the hydrogels and amino or thiol groups on proteins.131 Furthermore, ultrasound-mediated strategies have been employed to improve the adhesion of hydrogels, enabling not only stronger attachment but also on-demand detachment for applications, which is particularly useful in transdermal drug delivery. In these system, chitosan, a polymer abundant in amine groups, acts as a bridging agent to enhance both physical and chemical bonding with tissue substrates (Fig. 5A).132 Additionally, the integration of chitosan with alginate acrylamide hydrogel has demonstrated a significant increase in bioadhesion, utilizing a unidirectional manner to optimize contact and bonding (Fig. 5B).133 Another notable development involves a double-network material that comprises PAA grafted with N-hydroxysuccinimide (NHS) ester and chitosan (Fig. 5C).134 This bioadhesive employs a duel mechanism: it initially rapidly forms physical bonds (e.g., hydrogen bonds) upon contacting with wet tissue surfaces, and subsequently develop covalent bonds forms between NHS ester groups and primary amine groups on the tissue. Upon hydration, this bioadhesive layer transitions into a hydrogel, exhibiting mechanical properties akin to soft tissues, thus enhancing adhesion strength and stability.135 Such advancements highlight the potential of bioadhesive hydrogels in enhancing the efficacy of topical treatments through improved delivery and retention of therapeutic agents.
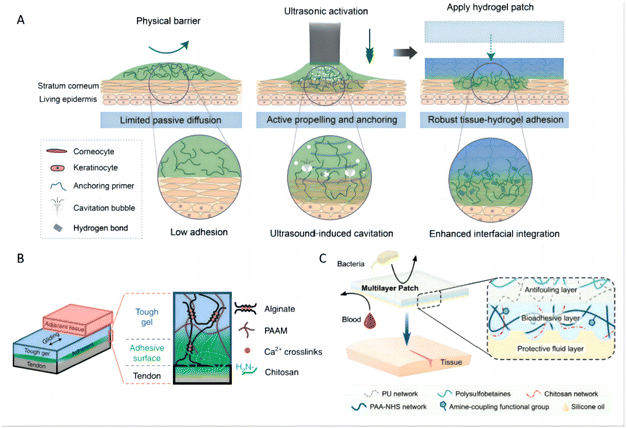 |
| Fig. 5 Bioadhesion of hydrogels. (A) Schematic of skin with barrier effects limiting passive diffusion and impairing bioadhesion. Ultrasound actively propels and anchors primer agents into a tissue substrate, causing spatially confined tough adhesion between hydrogel and tissue. Reproduced with permission from ref. 132 Copyright 2022 American Association for the Advancement of Science. (B) The adhesive surface of a tough hydrogel was achieved by the unilateral coupling of the amine-rich bridging polymer chitosan. Reproduced with permission from ref. 133 Copyright 2022 Springer Nature. (C) The illustrated schematic of the multilayer composition of the bioadhesive patch. The patch comprises a textured bioadhesive fused with an antifouling polymer layer on the non-adherent side and is wetted with a hydrophobic fluid layer on the adherent side to repel body fluids. Reproduced with permission from ref. 134 Copyright 2021 Wiley-VCH. | |
3.3 Occlusive effect of hydrogel
Occlusivity in dermatology refers to the ability of a substance to form a protective barrier over the skin, which helps to retain moisture by preventing transepidermal water loss (TEWL). This barrier mimics the function of the skin's natural lipid layer, providing a seal that slows water evaporation and maintains hydration levels within the stratum corneum (SC). The efficacy of topical formulations in maintaining skin hydration is intimately connected to their occlusivity, which is essential for regulating water loss from the stratum corneum.136 This property is particularly important in treating conditions like psoriasis and atopic dermatitis (AD), where maintaining skin hydration can significantly mitigate symptoms and improve skin aesthetics, impacting the pathology of these conditions directly. The effect of occlusivity on skin hydration and drug delivery is quantitatively evaluated through the measurement of TEWL.137 Under occlusive conditions, which ensure the SC is fully hydrated, the permeation of drugs through the skin can vary. This variation is influenced by changes in the skin's hydrophilic/hydrophobic balance, affecting the resistance to diffusion depending on the drug's properties.138
Hydrogels offer several distinct advantages compared to highly occlusive products like Vaseline that primarily protects against dehydration without adding moisture. They maintain the skin's respiratory capacity while providing additional moisture to affected areas. Due to their hydrophilic nature, hydrogels are easily removed from the skin, provide a cooling effect, and are effective at absorbing excess exudate often present during the acute phases of psoriasis and AD (Fig. 6). Compared to creams or lotions, hydrogels designed with strategic consideration can achieve prolonged contact with the skin, resulting in enhanced occlusivity and improved drug absorption. For example, a randomized study involving 20 participants with mild-to-moderate atopic dermatitis revealed that a hydrogel vehicle enhanced skin hydration more effectively than a moisturizing lotion, without further impairing the epidermal barrier function.139 In another comparison, a hydrogel formulation containing 0.1% mometasone furoate demonstrated bioequivalence to a lotion form but provided superior moisturization.140 It significantly reduced TEWL by 43% within 2 hours, maintaining a 29% reduction after 24 hours, and also increased skin hydration by 38% above baseline after 24 hours. Given its benefits, the hydrogel formulation was anticipated to improve patient adherence to the regimen, echoing findings from prior preference studies. Further comparative analysis of different galenic formulations revealed that hydrogels provided higher tissue breathability and hydration compared to semi-occlusive ointments and petrolatum-based products. Unlike these, hydrogels preserved tissue breathability and effectively moistened the skin, attributed to their composition of water, carbomer, and propylene glycol.141 These properties make hydrogels a preferred option for delivering therapeutic agents in dermatological treatments, offering efficient hydration and enhancing the overall treatment efficacy.
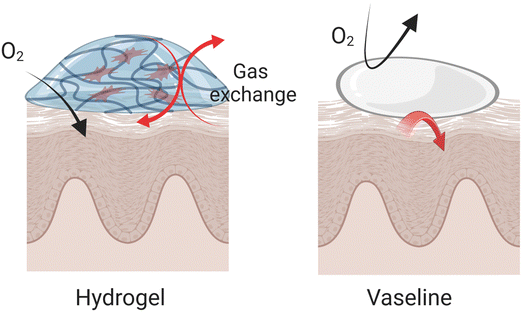 |
| Fig. 6 Occlusive effect of hydrogels. Hydrogels provide skin lesions with additional moisture while allowing gas exchange to maintain occlusive effects. The figure is made with BioRender (https://biorender.com/). | |
3.4 Tunable stiffness of hydrogel
The tunable stiffness of hydrogels plays a critical role in modulating the mechanical environment around skin cells, particularly in the context of inflammatory skin diseases. This property is essential in inflamed skin, where increased tissue stiffness can significantly influence cellular behavior and exacerbate disease progression.142 The stiffness of a hydrogel, often quantified by its elastic modulus, acts as a crucial physical determinant that influences how tissues respond to mechanical stress. This is especially relevant as different tissues naturally exhibit varying stiffness levels, from the softness of brain tissue to the rigidity of bone tissue. (Fig. 7A).142
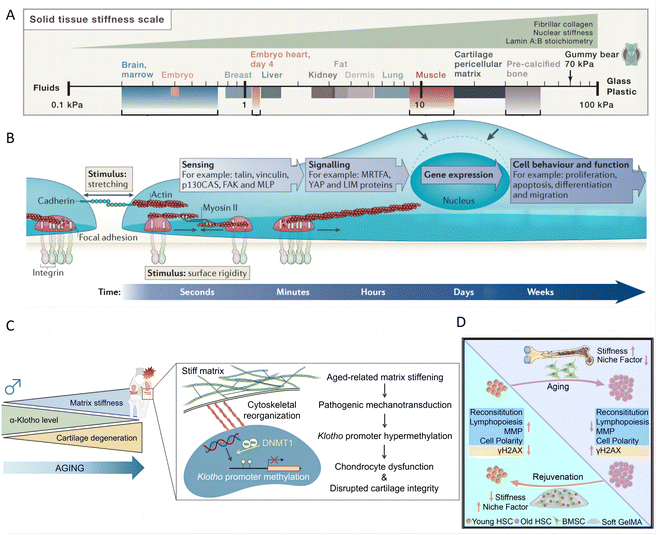 |
| Fig. 7 The impact of matrix stiffness on cell behavior and function for inflammatory skin diseases treatment. (A) Different tissues have varying levels of stiffness, ranging from soft brain tissue to rigid bone tissue. Reproduced with permission from ref. 142 Copyright 2016 Elsevier Inc. (B) Mechanotransduction converts mechanical stimuli-such as substrate rigidity (through contractile units or mature integrin adhesions), stretching (through cell–cell contacts or integrin adhesions) or shear stress (not shown) – into chemical signals to regulate cell behavior and function. Reproduced with permission from ref. 143 Copyright 2014 Springer Nature. (C) Time-dependent matrix stiffening in articular cartilage initiates pathogenic mechanotransductive signaling pathways. This process leads to dysfunction in chondrocytes and compromised integrity of the cartilage, primarily through hypermethylation of Klotho promoter. Reproduced with permission from ref. 144 Copyright 2023 Springer Nature. (D) Matrix stiffness negatively regulates the HSC niche factor expression by BMSCs. Reproduced with permission from ref. 145 Copyright 2023 Elsevier Inc. | |
Topically applied hydrogels directly interact with the stratum corneum of the skin. Keratinocytes, the primary cell type in the stratum corneum, not only form the skin's protective barrier but also proliferate and differentiate to repair damaged tissue upon injury. Additionally, keratinocytes can sense external stimuli, release inflammatory mediators, and play pivotal roles in skin inflammation and immune regulation, which are critical in the pathogenesis of psoriasis and AD.146
Hydrogels can be strategically designed to influence cellular behaviors by mimicking or counteracting changes in tissue rigidity. Cells can sense the stiffness of an external hydrogel matrix and translate these mechanical cues into biochemical signals, which in turn influence their biological activities and homeostasis through mechanotransduction signaling.147 Such hydrogels can profoundly influence cell behaviors,143 such as cell proliferation,148 migration,149 maintenance of stemness,150 and differentiation (Fig. 7B).151 Consequently, hydrogels play a pivotal role in various tissue pathological processes, including inflammation, matrix deposition, and fibrosis.152 In inflammatory conditions, elevated tissue stiffness can intensify cellular strain, triggering altered intracellular signaling pathways. In one study, PAA hydrogels with varying substrate stiffness were used to incubate bone marrow-derived macrophages (BMM). Hydrogel with low stiffness increase CD86 expression and promote IL-1β and TNF-α secretion, indicating a shift toward M1 macrophages. Conversely, medium stiffness hydrogels enhanced CD206 expression and increase IL-4 and TGF-β secretion, suggesting a transition toward M2 macrophages, which is influenced by the ROS-initiated NF-κB pathway.153 A specific example involves time-dependent matrix stiffening in chondrocyte physiology through epigenetically regulating the expression of α-Klotho, a protein associated with aging. Specifically, increased matrix stiffness leads to the methylation of the Klotho promoter, which in turn downregulates Klotho gene expression and accelerates chondrocyte senescence (Fig. 7C).144 Additionally, another study demonstrated that matrix stiffness modulates hematopoietic stem cell (HSC) niche factor expression in bone marrow stromal cells (BMSCs). The increased stiffness activated Yap/Taz signaling and promoting BMSC expansion in 2D culture. However, this effect is reversed in 3D culture using soft gelatin methacrylate hydrogels, highlighting the importance of three-dimensional context in cellular responses to mechanical cues (Fig. 7D).145 Based on the observed effects of hydrogel stiffness on immune cells,153 chondrocytes,144 and stem cells,145 it is inferred that hydrogel stiffness could similarly affect keratinocytes through mechanotransduction mechanisms. Given the critical roles of keratinocytes in skin barrier function, repair, and inflammation, modulating hydrogel stiffness might influence keratinocyte proliferation, differentiation, and release of inflammatory mediators, thereby playing a significant role in the treatment of psoriasis and AD. These examples underline the critical role of hydrogel stiffness in designing materials that can effectively mimic or modulate the cellular microenvironment, thereby guiding cell function and fate. This capability is crucial for developing advanced therapies for inflammation, aging, and tissue regeneration, leveraging the physical properties of hydrogels to achieve desired biological outcomes.
3.5 Tunable porosity of hydrogels
The structure of hydrogels is characterized by a crosslinked polymeric network that encapsulates water within its matrix at the nanometer scale. This structure forms open spaces known as mesh size or porosity, which are crucial for facilitating the diffusion of liquids and small molecular solutes. The porosity of a hydrogel is a key factor in understanding how cells behave within the hydrogel environment and how drugs diffuse through it.154
Recent research highlights how the porosity of hydrogels, through the design of 3D microniches with controlled geometries, significantly affects human mesenchymal stem cell (hMSC) behavior. Studies have demonstrated that the cell volume and shape within these microniches can affect various cellular components and functions, such as actin filaments, focal adhesions, nuclear shape, YAP/TAZ localization, cell contractility, nuclear accumulation of histone deacetylase 3, and lineage selection. These findings illustrate that cellular responses are highly sensitive to changes in their physical environment, especially the spatial configuration offered by the hydrogel (Fig. 8A).155 Another study further exemplifies the influence of hydrogel porosity on cellular behavior, particularly in the context of regenerative wound healing. Specifically, microporous annealed particle scaffolds, particularly those with larger pores, showed that such structures promote regenerative wound healing by inducing a balanced pro-regenerative macrophage response. This response facilitates mature collagen regeneration and reduces inflammation, leading to improved healing outcomes (Fig. 8B).156 In drug delivery applications, the porosity of hydrogels plays a critical role in determining the diffusion pathway of drugs, thereby influencing the interactions between the drugs and the polymer network. This property is harnessed to design hydrogels as controllable drug release platforms, which can be tailored to meet specific therapeutic needs. The relationship between the mesh scale features and the molecular interaction within the hydrogel is crucial for achieving controlled drug release (Fig. 8C). An illustrative example of this principle is observed in triblock copolymer hydrogels, where the release of proteins (BSA or IgG) was prolonged from 6 to 14 days as the polymer concentration was increased from 20% to 35%.157 This ability to decouple hydrogel porosity from its macroscopic properties allows for the independent customization at different length scales, thereby catering to specific application requirements and enhancing the functionality of hydrogels in biomedical contexts.
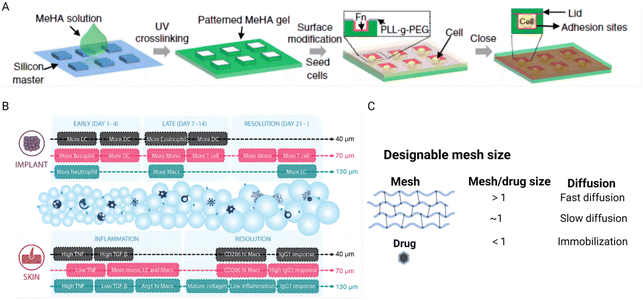 |
| Fig. 8 The effect of hydrogel porosity on tuning cellular behavior and controlling drug delivery. (A) Schematic of the method to encapsulate single cells in a 3D microniche. Reproduced with permission from ref. 155 Copyright 2017 Springer Nature. (B) Schematic illustrations of the immune responses to 40 μm, 70 μm, and 130 μm MAP scaffolds in the subcutaneous implantation model and the wound healing model. Reproduced with permission from ref. 156 Copyright 2023 Wiley-VCH. (C) Porosity mediates drug diffusion at different scales. The figure is made with BioRender (https://biorender.com/). | |
3.6 Tunable viscoelasticity of hydrogels
The viscoelasticity of topical hydrogels may have a significant impact on keratinocytes in the stratum corneum of the skin, with mechanisms similar to how the viscoelasticity of the extracellular matrix (ECM) regulates behaviors of other cell types. Keratinocytes play a crucial role in the pathogenesis of psoriasis and atopic dermatitis, making the modulation of their behavior particularly relevant in the treatment of these conditions. The viscoelastic properties of hydrogels can mimic those of the ECM, including stress relaxation and creep, are fundamental in regulating cell behaviors such as proliferation, migration, and spreading.158 These behaviors are regulated through the activation of various proteins (FAK, ROCK, MLCK), pathways (phosphatidylinositol 3-kinase (PI3K), ERK, and Rho), and transcription regulators (YAP, p27, Sp1, RUNX2, and EGR1).159 The ability to tune the stress relaxation of hydrogels, independently from their initial stiffness, plays a crucial role in influencing mesenchymal stem cell (MSC) differentiation and activity. Studies have shown that hydrogels with faster stress relaxation rates promote enhanced cell spreading, proliferation, and osteogenic differentiation, underscoring the importance of a hydrogel's mechanical properties in guiding stem cell fate decisions. This suggests that the ability of a hydrogel to mimic the dynamic nature of the ECM through stress relaxation is significant in stem cell biology and tissue engineering (Fig. 9A).160 For instance, hydrogels with dynamic crosslinks that have a high dissociation rate constant allow for cell-induced network alteration, leading to accelerated stellate spreading, assembly, mechanosensing, and differentiation of the encapsulated stem cells compared to hydrogels with slower dissociating dynamic crosslinks. The swift and precise attachment of cell adhesive ligands facilitated by these quick-dissociating crosslinks is vital for extremely rapid stellate spreading (within 18 hours post-encapsulation) and enhanced stem cell mechanosensing in a 3D setting (Fig. 9B).161 The hydrogel's viscoelasticity can also induce symmetry breaking of spheroidal tissues, subsequently leading to the formation of invading finger-like protrusions and epithelial-to-mesenchymal transition. Through computational modeling, it is found that the morphological changes are closely linked to matrix viscoelasticity, tissue viscosity, cell motility, and cell division rate. This highlights how the viscoelastic nature of the matrix influences tissue proliferation and disrupts spheroidal symmetry, promoting invasive growth and YAP nuclear translocation dependent on the Arp2/3 complex (Fig. 9C).162 Furthermore, the viscoelasticity of the ECM can also regulate immune responses. For instance, functionally distinct T-cell populations can be generated from T cells receiving the same stimulation by altering the viscoelasticity of their surrounding ECM. By employing a model ECM based on norbornene-modified collagen type I, whose viscoelasticity can be adjusted independently from its bulk stiffness via bioorthogonal click reactions, researchers found that ECM viscoelasticity influences T-cell phenotype and function through the activator-protein-1 signaling pathway, a key regulator of T-cell activation and fate.163 These examples highlight the profound impact of hydrogel viscoelasticity on cellular behavior and tissue dynamics, offering promising strategies for designing materials that effectively influence biological processes for therapeutic applications.
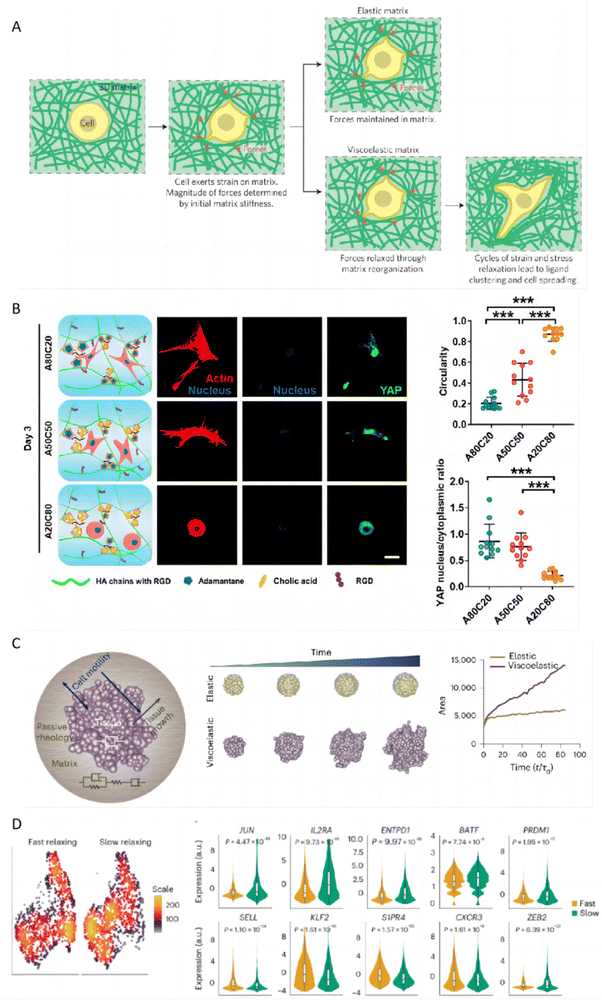 |
| Fig. 9 The impact of hydrogel viscoelasticity on cellular behaviors. (A) Hypothesis for how initial elastic modulus and stress relaxation properties of matrix regulate cellular behaviors. Reproduced with permission from ref. 160 Copyright 2015 Springer Nature. (B) Immunofluorescence staining revealed differences in F-actin, nuclei, and YAP expression in hMSCs encapsulated in hydrogels with varying HA-ADA and HA-CA ratios after 3 days, alongside analyses of cell shape and nuclear YAP intensity. Reproduced with permission from ref. 161 Copyright 2021 Springer Nature. (C) A schematic illustrates a theoretical model for tissue growth within a passive viscoelastic matrix, highlighting the adjustability of tissue and matrix viscosities alongside matrix elasticity. Reproduced with permission from ref. 162 Copyright 2023 Springer Nature. (D) The scRNA-seq UMAP density plots revealed distinct localization of CD8+ T cells cultured in fast-relaxing or slow-relaxing collagen matrices. Violin plots were utilized to compare the expression levels of the selected genes. Reproduced with permission from ref. 163 Copyright 2023 Springer Nature. | |
3.7 Tunable degradation of hydrogels
The tunable degradation of hydrogels is a critical feature that significantly impacts their effectiveness in treating inflammatory skin conditions, by directly affecting cellular activities essential for tissue healing and disease control, such as cell proliferation, apoptosis, migration,164 and stemness.89 The degradation process of hydrogels can influence the local microenvironment, modulating the behavior of keratinocytes and immune cells, which are pivotal in the pathogenesis of these skin diseases. On the other hand, as hydrogels degrade, their mesh size expands, which facilitates the diffusion of drugs out of hydrogel. This degradation can occur either in the polymer backbone or at crosslink junctions, predominantly through mechanisms such as hydrolysis or enzymatic activity. For example, employing ester bonds, known for their hydrolytic susceptibility, allows for the development of biodegradable polyethylene glycol (PEG) hydrogels that result in prolonged protein release. Such hydrogels have been engineered to have protein release half-lives extending up to 17 days, showcasing their ability to provide sustained therapeutic effects.165 Moreover, the hydrogels can be designed with controlled degradability, offering an advantage in protecting labile drugs from premature breakdown. This characteristic makes them excellent platforms for facilitating a myriad of physiochemical interactions that determine drug release dynamics. A notable example is the design and synthesis of a series of ester linkers with hydrolytic half-lives ranging from 4 hours to 4 months. By incorporating these linkers into hydrogels, it is possible to precisely control the degradation rate of the hydrogel, thereby tailoring the subsequent release profile of encapsulated proteins. The release duration and kinetics in these hydrogels are thus determined by the cleavage rate of the ester linkers, allowing for fine-tuned control over the therapeutic payload.166 This tunable degradation of hydrogels offers targeted and controlled treatment options that align closely with the physiological needs of inflamed or damaged tissue for advanced drug delivery and regenerative medicine.
3.8 Tunable interactions between hydrogels and drug/tissues
The tunability of hydrogel-tissue and hydrogel-drug interactions play a vital role in the development of hydrogels for treating inflammatory skin diseases. These interactions, governed by the choice and functionalization of ligands, are crucial for modulating cellular responses and optimizing drug delivery effectiveness.
One significant example of this is the exploration of engineered peptide assemblies to control cell migration. By precisely regulating the presentation of integrin ligands derived from the ECM, bidirectional control of cell migration was achieved. The self-assembled nanofibers exhibited an ultra-high ligand density, inhibiting cell migration by preventing the disassembly of integrin/actin at the cell rear, which offers innovative strategies for tackling issues like tumor metastasis and provides valuable insights into creating hydrogels that can precisely regulate cellular functions (Fig. 10A).167 Similarly, another study elucidated how the density of RGD ligands in alginate hydrogels affects interactions between cells and polymers, influencing the morphogenesis of human induced pluripotent stem cells (hiPSCs) in 3D cultures. A high RGD density alongside rapid stress relaxation fosters hiPSC viability, proliferation, polarization, and lumen formation. In contrast, a reduced RGD density paired with slow stress relaxation leads to hiPSC apoptosis (Fig. 10B).168
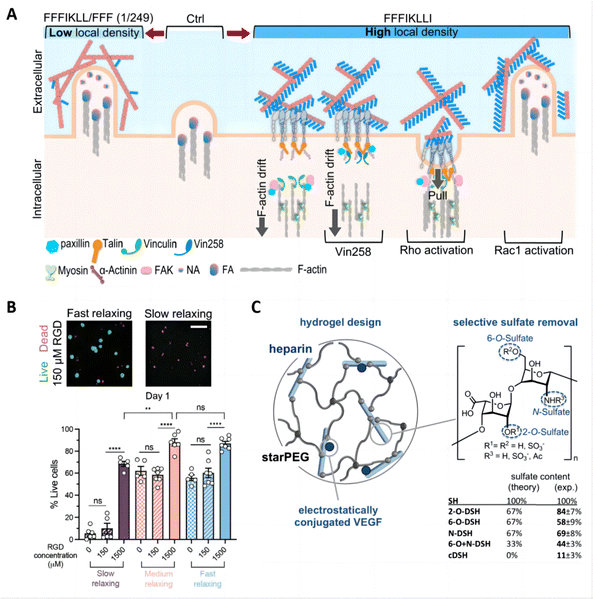 |
| Fig. 10 Tunable interactions between hydrogels and drugs/tissues for skin therapy and drug delivery. (A) Schematic summary of outside-in regulation of HuH-7 cell motility via precise control of ligand density on nanofilaments, and the restoration of cell motility via intracellular signaling. Reproduced with permission from ref. 167 Copyright 2022 Springer Nature. (B) Hydrogel ligand density and stress relaxation regulate hiPSC viability. Reproduced with permission from ref. 168 Copyright 2021 Wiley-VCH. (C) Hydrogel design using selectively desulfated heparin derivatives as hydrogel building blocks. Reproduced with permission from ref. 169 Copyright 2015 Elsevier. | |
In drug delivery applications, the chemical composition of hydrogels drives interactions between the drug and the polymer chains, and these interactions can be made specific by incorporating multiple binding sites for targeted drug–polymer interactions. An effective strategy involves forming chemical bonds between drug molecules and polymer chains, utilizing medium- to high-affinity interactions to control drug release rates and minimize uncontrolled diffusion through the hydrogel matrix.154 For example, covalent binding methods have been employed to attach engineered variants of vascular endothelial growth factor (VEGF) to fibrin hydrogels. This approach prevents the rapid depletion of growth factors and fosters localized, controlled angiogenesis.170 Additionally, electrostatic interactions are frequently utilized to strengthen the affinity between drugs and polymer chains. An example of this is the controlled release of heparin-binding proteins achieved by integrating sulfated heparin into the hydrogel, optimizing therapeutic efficacy (Fig. 10C).169 These studies highlight the sophisticated engineering behind hydrogel formulations, demonstrating how molecular control over interactions within the hydrogel matrix can lead to improved outcomes in skin disease therapy and drug delivery applications.
4. Therapeutic agents loaded in hydrogels for inflammatory skin diseases
Hydrogels, owing to their specialized physicochemical properties, are highly effective for targeted drug delivery in the treatment of inflammatory skin diseases such as psoriasis and atopic dermatitis.171 Their utility stems from several key features: (1) polymer composition and structure: hydrogel's polymer composition, degree of cross-linking, and adjustable pore sizes are conducive to encapsulating therapeutic agents of varying sizes, from small molecule drugs to large biomolecules such as proteins, and even whole cells, accommodating their diverse sizes and biological activities.172,173 (2) Controlled release capabilities: the mesh size of hydrogels, combined with tunable degradation rates and the introduction of specific functional groups, allows for the effective encapsulation and controlled release of therapeutic agents. This controlled release is crucial for maintaining effective therapeutic levels over extended periods, which is particularly beneficial for chronic conditions like psoriasis and atopic dermatitis.174 (3) Biocompatibility and stability: the predominantly aqueous environment within hydrogels minimizes the risk of drug denaturation—a common issue with organic solvents—thus preserving the biological activity of the therapeutic agents. This aspect is especially important for protein-based drugs and other sensitive molecules. (4) Drug loading for diverse molecule types: hydrogels are capable of being loaded with various types of drugs, including hydrophilic and hydrophobic drugs.175–177 Hydrophilic drugs can be released directly through the hydrogel's porous structure, while hydrophobic drugs can be effectively loaded and released by modifying the hydrogel's hydrophilic/hydrophobic balance or by using nanoparticles to encapsulate the drugs before incorporating them into the hydrogel matrix.178 (5) Smart hydrogels with responsiveness: smart hydrogels can dynamically adjust their mechanical properties—such as swelling, hydrophilicity, and permeability—in response to specific triggers like temperature, pH, and proteases, which are commonly elevated in inflammatory conditions.179 Integrating smart hydrogels with wearable sensors and AI-driven models enhances treatment outcomes. These sensors monitor hydrogel states like swelling and permeability, transmitting data wirelessly for analysis.180 AI models analyze this data to predict patient responses, optimizing treatment protocols. This technology improves treatment precision, personalizes care, and provides real-time feedback on disease progression and therapy responses.
Here, we provide a comprehensive overview of therapeutic agents that have been successfully encapsulated in hydrogels for the topical treatment of inflammatory skin diseases. The therapeutic agents include conventional therapeutic agents,181,182 novel compounds targeting oxidative stress pathways, small molecule drugs, and biologics such as antibodies (Fig. 11). Each of these categories represents a crucial component of the current therapeutic landscape, offering unique mechanisms of action that are central to managing the complex pathology of these skin conditions. Furthermore, we will explore the therapeutic agents not previously incorporated into hydrogels, such as emerging therapeutic modalities like small interfering RNA (siRNA), to offer insights for delivering a more precise approach to treatments with potentially fewer side effects.
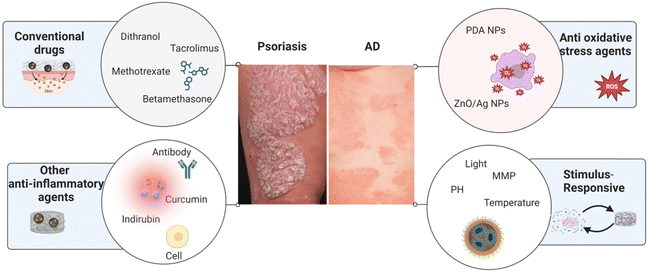 |
| Fig. 11 A summary of therapeutic agents loaded in hydrogels for inflammatory skin diseases. The figure is made with BioRender (https://biorender.com/). | |
4.1 Conventional drugs
A range of commercial topical formulations, including corticosteroids,13 vitamin D3 analogues,183 calcineurin inhibitors,107 keratolytics, and combination topical agents (e.g., corticosteroid with vitamin D3), which are available in forms such as creams, ointments, foams, or gels, are utilized to treat inflammatory skin diseases.184 However, these traditional methods often fall short in providing the desired drug release kinetics and distribution profiles. To address this, recent research has focused on developing hydrogel-based delivery systems for conventional drugs such as dithranol, tacrolimus (TAC), methotrexate (MTX), and betamethasone. These hydrogel systems are engineered to deliver precise drug dosages with controlled release rates, thereby improving therapeutic efficacy and enhancing patient compliance.
A notable example from early 1992 involved the development of a dithranol-impregnated hydrogel for psoriasis treatment, which utilized a slow-release mechanism through integration into a polyurethane urea film, showing significant improvements in clinical efficacy.185 Additionally, Carbomer hydrogels incorporating nanostructured lipid carriers (NLCs) have been used for delivering MTX, a low-concentration anti-inflammatory immunosuppressant. This formulation reduces skin irritation commonly associated with topical MTX applications by entrapping the drug within NLC and solid–lipid nanoparticle (SLN)-containing hydrogels, significantly decreasing irritation compared to traditional MTX hydrogels.97 Moreover, a betamethasone-loaded hydrogel (B-Gel) was effectively developed to entrap steroids and sustain drug release.19 The hydrogel, synthesized by heating and cooling a mixture of betamethasone and A6 gelator, effectively addressed challenges such as inefficient steroid entrapment, burst drug release, poor skin permeability, and high toxicity, thereby alleviating symptoms associated with psoriasis.
In addition to pre-clinical research, clinical trials also underscore the potential of hydrogel formulations in treatment settings (Table 1). For example, a double-blind, single-center controlled trial involving 30 patients observed that occlusive hydrogels had an increased therapeutic effect on 0.005% calcipotriene-0.064% betamethasone malonate ointment without causing skin irritation. Moreover, patients with palmoplantar lesions exhibited high tolerance to topical MTX (0.25%) preparations in a hydrogel base, suggesting that formulations with higher concentrations and enhanced penetration may yield better outcomes.186 A proof-of-concept, open-label clinical study of a novel doxycycline topical formulation for treating dermatologic conditions in AD patients confirmed that the application of NanoDOX® Hydrogel 1% led to significant clinical improvements and pruritus relief without adverse effects, emphasizing the potential of hydrogel formulations in revolutionizing dermatological treatments.187
Table 1 Clinical trials involving hydrogels for the treatment of inflammatory skin diseases
Hydrogels |
NCT number |
Payload(s) |
Features |
Disease |
Status |
Topical desonide hydrogel 0.05% |
NCT00690833 |
Desonide 0.05% |
Corticosteroids |
AD |
Completed |
Nanodox 1% (doxycycline monohydrate hydrogel) |
NCT02910011 |
1% doxycycline monohydrate |
Antibiotics |
AD |
Completed |
Hydrogel vehicle |
NCT01065714 |
Hydrogel vehicle |
Maintaining epidermal barrier |
AD |
Completed |
Aurstat anti-itch hydrogel |
NCT01905631 |
Unknown |
Unknown |
AD |
Completed |
PH-10 |
NCT01247818 |
Rose bengal disodium |
Unknown |
Psoriasis |
Completed |
DLX105 Hydrogel |
NCT01936337 |
Unknown |
Unknown |
Psoriasis |
Completed |
Topical 1% ZL-1102 hydrogel |
ACTRN12620000700932 |
Anti-interleukin-17A antibody fragment |
Antibody |
Psoriasis |
Completed |
DLX105 hydrogel |
NCT01595997 and NCT01936337 |
Single-chain anti-TNF-α-PENTRA®-antibody |
Antibody |
Psoriasis |
Completed |
Hydrogel patch |
NCT00924508 |
Non |
Hydrogel patch as occlusion |
Eczema |
Terminated |
4.2 Anti-oxidative stress agents
ROS-induced oxidative stress plays a critical role in the pathogenesis of inflammatory skin diseases, such as psoriasis and atopic dermatitis (AD). A high level of ROS promotes the release of pro-inflammatory cytokines and T-cell differentiation, resulting in the onset and deterioration of psoriasis188 and AD.189 Hydrogels, loaded with a variety of antioxidant drugs, offer a promising therapeutic approach. These antioxidants include ceria nanoparticles, MnO2 nanosheets, fullerenol, and organic compounds with phenol groups, each selected for their ability to counteract oxidative stress (Fig. 12A).190
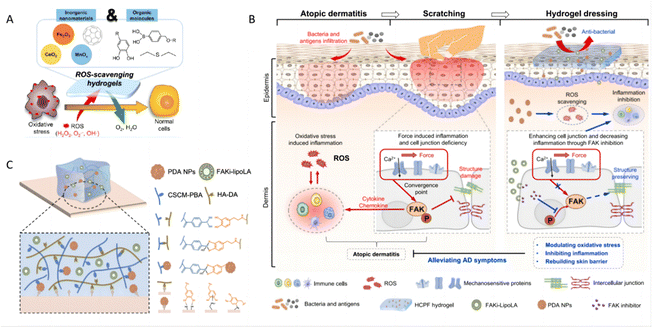 |
| Fig. 12 Hydrogels regulating oxidative stress. (A) Major strategies of ROS-scavenging hydrogels and therapeutic mechanisms to suppress inflammatory responses. Reproduced with permission.190 Copyright (2021) American Chemical Society. (B) Schematic of hydrogel for AD treatment. The left schematic depicts the inflammatory response and scratching-induced exacerbation of AD, while the right shows the hydrogel dressing treating AD by scavenging reactive oxygen species and inhibiting FAK phosphorylation. (C) Schematic illustration of crosslinking mechanisms and adhesive mechanisms of hydrogels. Reproduced with permission.51 Copyright (2023) Springer Nature. | |
Numerous studies have focused on therapeutic hydrogels designed to suppress the elevated oxidative stress observed in skin lesions. For example, a hydrogel incorporating polydopamine nanoparticles (PDA NPs) has proven effective ROS scavenging capability. This hydrogel also includes a liposome-encapsulated hydrophobic focal adhesion kinase inhibitor (FAKi-lipo), which not only possesses adhesive, stretchable, and self-healing properties but also synergistically reduces AD symptoms, restores the skin barrier, and decreases inflammation (Fig. 12B and C).51 In addition, the hydrogel can be integrated with other functional materials as composites for psoriasis treatment. In one specific composite hydrogel, ZnO nanoparticles anchored with silver (Ag) particles (ZnO/Ag) are integrated into the hydrogel matrix to enhance immunoregulatory properties. Experimental results have demonstrated that ZnO/Ag nanoparticles exert a self-therapeutic effect by inactivating the p65 subunit in pro-inflammatory macrophages and reducing adaptive cytokine secretion in keratinocytes. This effect is mediated through the downregulation of ROS-induced STAT3-cyclin D1 signaling, which demonstrates the potential of these nanoparticles to directly target the inflammatory pathways implicated in skin diseases.191 The multi-functional approach highlights the versatility and efficacy of hydrogels in delivering targeted treatments that address both the symptoms and underlying mechanisms of inflammatory skin conditions.
4.3 Hydrogels incorporating other anti-inflammatory agents
Hydrogels are being increasingly explored for their capability to encapsulate and deliver a wide range of therapeutic agents, including natural products with anti-inflammatory properties (such as curcumin and indirubin),18,192 small molecule drugs193 and biologics,194 in treating psoriasis and AD. One specific strategy involves hydrogels incorporating hydrophobic drug-loaded (e.g., curcumin) PLGA nanoparticles to improve their transdermal delivery. This hydrogel was employed to topically treat imiquimod-induced psoriasis-like mice, for promotion of drug permeability across skin and enhancement of anti-psoriatic activity.18 Similarly, a hydrogel-based microemulsion drug delivery system for indirubin demonstrated improved moisture retention, drug penetration, and retention in the skin, thereby achieving better therapeutic effects.192 For more severe cases of psoriasis, the systemic administration of biologics targeting TNF-α,195 IL-17,196,197 the p40 subunit of IL12 and IL-23, or p19 subunit of IL-23 significantly ameliorated skin lesions.23,198,199 However, long-term systemic administration of these biologics poses a greater risk of adverse effects compared to topical treatments.200 To mitigate these risks, embedding these biologics in a hydrogel matrix creates a localized drug reservoir that can release the therapeutic agent directly to the affected skin areas in a controlled manner.194,201 Additionally, the hydrophilic nature of hydrogels facilitates the encapsulation of large, complex molecules like proteins without compromising their structural integrity or biological activity. This feature is crucial for protein-based biologics, whose therapeutic efficacy depends heavily on maintaining their three-dimensional conformation. For example, a single-chain anti-TNF-α-PENTRA® antibody (DLX105) was incorporated into hydrogels for topical psoriasis treatment. Clinical studies indicated significant reductions in proinflammatory cytokine mRNA levels compared to placebo. Enhanced effects were observed following weekly tape stripping of plaques to increase drug penetration.194 Another example involved the integration of an anti-interleukin-17A antibody fragment (ZL-1102) into hydrogels for topical psoriasis treatment. A randomized, double-blind, placebo-controlled phase Ib study showed greater improvements in local PASI scores with ZL-1102 hydrogel compared to the vehicle, highlighting its efficacy and tolerability.201 With further understanding of psoriasis and AD pathogenesis, new molecules such as ruxolitinib,202 delgocitinib,203 roflumilast,204 tapinarof,200,205 benvitimod206 and retinoic acid receptor-related orphan receptor-γ agonists207 are emerging as new, safer, and effective topical agents for inflammatory skin diseases, which can be integrated into hydrogel platform systems. Ongoing preclinical studies are also exploring the potential of integrating hydrogels with new topical anti-inflammatory drugs, including interleukin-2 inhibitors, RNA modulators, and amygdalin analogues.200 Additionally, small interfering RNA (siRNA), plasmid DNA, and bacteriophages have shown effectiveness as a topical therapy for inflammatory skin diseases, suggesting that hydrogels could also be adapted to load and deliver them, leveraging their versatile delivery capabilities to enhance treatment outcomes for these challenging conditions.208,209
4.4 Design of hydrogels for cell encapsulation, expansion and delivery
Hydrogels, due to their tissue stroma matrix-mimicking properties, serve as excellent platforms for cell encapsulation and expansion, both in vitro and in vivo, enabling high-efficient tissue regeneration.159 Various cells, including stem cells210 and endothelial cells (ECs),211 can be encapsulated in hydrogels where they can proliferate while maintaining their functional characteristics. This technology holds significant potential for the treatment of psoriasis and atopic dermatitis, where localized delivery of therapeutic cells or bioactive molecules can enhance skin repair and reduce inflammation. For instance, hydrogels can be designed to encapsulate keratinocytes or fibroblasts, which play crucial roles in skin homeostasis and wound healing. Once cultured, these cells can be transferred to specific disease sites, acting as localized factories producing proteins and factors that sustainably promote tissue regeneration and repair. A notable application of this technology is the one-step method used to fabricate cell sheets integrated with a biomimetic hydrogel for skin wound healing. In this process, cell sheets composed of human skin fibroblasts (HSFs) or human umbilical vein endothelial cells (HUVECs) are combined with a biomimetic hydrogel to create a cell sheet-laden hydrogel (CSH). The hydrogel is fabricated using UV crosslinking and ionic crosslinking, which allows for easy release of the gel and cell sheet film from the substrate. When implanted into full-thickness skin defects, the CSH promotes enhanced wound healing by accelerating the formation of granulation tissue and the development of a new dermis complete with skin appendages (Fig. 13A).212 In another study, calcium-crosslinked alginate hydrogel dressings were designed to deliver murine macrophages or their secretome to enhance impaired wound healing in diabetic mice. The design of these alginate hydrogel dressing features a microporous structure that not only facilitates even cell loading but also supports prolonged cell survival and controlled cell release after the dressings are placed on wounds. (Fig. 13B).213
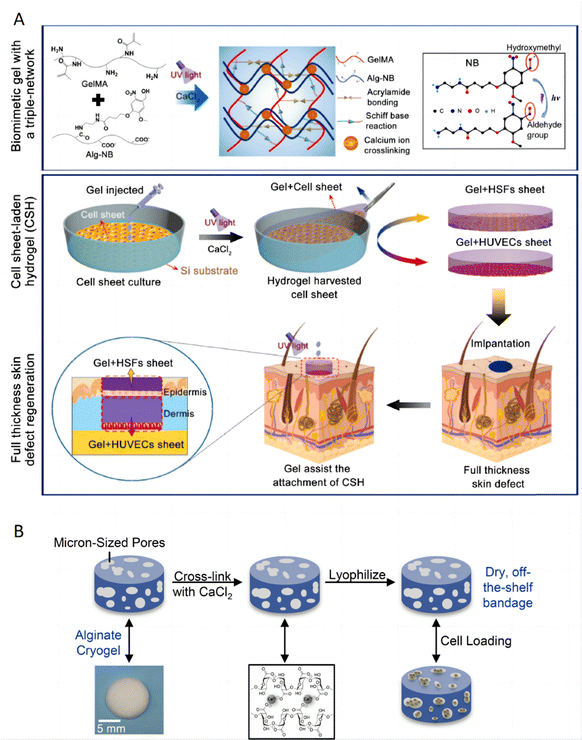 |
| Fig. 13 Hydrogels for cell encapsulation, expansion and delivery. (A) Schematic illustration of the formation of the biomimetic gel with a triple-network, the preparation of the cell sheet-laden hydrogel (CSH), and application of the CSH for full-thickness skin regeneration. Reproduced with permission from ref. 212 Copyright 2023 Elsevier B.V. (B) Descriptive schematic of the dressing fabrication showing cross-linking, lyophilization and cell loading. Reproduced with permission from ref. 213 Copyright 2022 Elsevier B.V. | |
5. Challenges, opportunities, and open questions
The innovative utilization of hydrogels as platforms for topical therapeutic systems in inflammatory skin diseases highlights their significant potential and the complexities involved in their application. With over half a century of industrial use, a wide array of hydrogel formulations are currently available on the market for transdermal drug delivery, serving various therapeutic purposes.214 These hydrogels enhance the skin permeation of drugs primarily through skin hydration, leveraging their moisturizing effects, which makes them particularly suitable for topical applications. Notable commercial examples include products from the Neutrogena® family by Johnson & Johnson, which utilize hydrogels to control the delivery of active ingredients through the skin. Another example is the Collagen Hydrogel Mask by Skin Republic, a serum-infused mask designed to restore skin moisture, elasticity, and radiance, enriched with sea minerals to nourish and hydrate the skin. These products highlight the practical applications of hydrogels in both therapeutic and cosmetic contexts.
Despite their proven ability to enhance drug permeation, control release, and provide targeted treatment for inflammatory conditions, several challenges and opportunities remain to be addressed to fully exploit their capabilities, especially for hydrogel therapeutics in inflammatory skin diseases. Several challenges and opportunities are discussed below.
5.1. How to improve skin barrier penetration of hydrogels?
Improving the skin barrier penetration of hydrogels is crucial for enhancing the efficacy of topical treatments, particularly for conditions like psoriasis where deep skin layers must be reached.215 However, achieving effective penetration without compromising skin integrity or causing irritation remains a challenge. Here are some potential advanced strategies and considerations for enhancing the penetration of hydrogels: (1) chemical penetration enhancers (CPEs): the use of CPEs216 such as fatty acids, urea, and surfactants can disrupt the lipid matrix of the stratum corneum, facilitating the deeper penetration of therapeutic agents into the skin. These enhancers work by altering the skin's barrier properties, increasing the permeability and thereby allowing more drug to reach its target. (2) Keratolytic agents: incorporating keratolytic agents like salicylic acid into hydrogels can help by removing scales and reducing the thickness of hyperkeratotic plaques, which are common in psoriasis. This not only aids in drug penetration but also helps in the symptomatic relief of scaling.217 One study reports the incorporation of betamethasone dipropionate and salicylic acid (SA) into cubosomes, unique nanoparticulate systems known for their biocompatibility and penetration efficacy, to enhance the management of scalp psoriasis.218 (3) Nanocarrier systems: encapsulating active ingredients in nanocarriers123,219 such as liposomes, niosomes, and solid lipid nanoparticles is a promising approach. These nanocarriers can protect the drug during penetration, enhance absorption by altering the interaction between the drug and the skin surface, and provide controlled release properties. (4) Other innovative technologies: exploring new technologies such as microneedles,220 integrated with hydrogel systems, or iontophoresis where a mild electrical charge is used to drive drugs into the skin, can also be considered to enhance delivery efficiency.
5.2. How to better design multifunctional hydrogels?
Ideally, the hydrogel itself should possess inherent anti-inflammatory properties, potentially through the incorporation of bioactive molecules or the engineering of the polymer network to interact beneficially with the skin's biochemistry. Hydrogels that are endowed with these properties offer a dual function in both drug delivery and therapeutic action, making them highly advantageous.
Looking forward, the trajectory of hydrogel therapeutics in inflammatory skin diseases is set to include the exploration of smart hydrogels that can respond to biological stimuli, as well as the integration of diagnostic features for monitoring treatment response, and the development of more robust and patient-friendly application systems. Notably, the incorporation of nanoparticles within hydrogels has been shown to improve drug delivery capabilities and enable responsiveness to environmental stimuli such as pH, temperature, or enzymes present in inflammatory lesions, thus adding a new dimension to their functionality.221 The versatility of hydrogels enables the tailoring of patient-specific treatments, which can be precisely customized according to the severity and type of inflammatory skin condition. Given the dynamic nature of inflammatory skin diseases, designing the ideal topical medication is complex, requiring a formulation that can adapt to the evolving requirements of lesion resolution throughout the treatment course. This approach ensures that hydrogel therapeutics remain effective and relevant in managing diverse and changing skin conditions.
5.3. How to achieve long-term safety?
Although hydrogels are recognized for their biocompatibility, the long-term safety of their degradation products on both skin and systemic health requires further investigation. While many hydrogels show promising rapid results in vitro or in animal models, their effectiveness and safety must be rigorously evaluated in human trials. The safety assessment of hydrogels involves a comprehensive approach that incorporates both in vitro and in vivo methods. This process may begin with cytotoxicity tests on human skin cell lines and a 3D cultured skin model to evaluate potential skin irritation and toxic effects. Skin permeation studies may be conducted using diffusion systems with micropig skin tissues and artificial membranes to assess the extent of hydrogel penetration through the skin.222 Hydrogels designed for prolonged use in treating inflammatory skin diseases must adhere to international standards and regulations through comprehensive testing. This includes conducting repeated insult patch testing (RIPT) to assess allergenicity, along with evaluations for potential irritation and sensitization. Additionally, regulatory bodies such as the FDA or EMA require thorough reviews that cover manufacturing consistency and the stability of hydrogels over time. Such regulatory approval processes are critical to ensure that hydrogels are not only effective but also safe for long-term use in a clinical setting. Moreover, it would be advantageous to underscore the imperative for conducting multi-center trials or long-term studies to gain deeper insights into the chronic impacts associated with these treatments, thereby enhancing our understanding of their efficacy and safety profiles.
5.4. How to better store and maintain the stability of hydrogels?
Storing and maintaining the stability of hydrogels designed for topical therapeutic systems, especially for the treatment of inflammatory skin diseases, is a complex task that demands a multifaceted approach to ensure the hydrogel's therapeutic efficacy and structural integrity over time. Given their high-water content, hydrogels face unique storage challenges compared to dry biodegradable polymers, such as PLGA microspheres. The hydrated nature of hydrogels can make terminal sterilization difficult, and sterility must therefore be typically validated for all source materials and fabrication processes. Improving the way of production is also a strategy, and one study demonstrated the enhanced physical stability of starch-based hydrogels via high-pressure processing.223
It's also crucial to maintain a controlled environment with optimal temperature (often between 2 °C and 8 °C for many formulations) and humidity conditions to prevent the premature swelling or dehydration of the hydrogel. Additionally, packaging plays a significant role; the use of airtight, light-resistant containers can protect photosensitive components and reduce the risk of oxidation. Hydrogels should also be buffered to maintain a pH that is compatible with both the stability of the API and the skin's natural pH. A meticulous design of the hydrogel network to facilitate reversible physical interactions can also aid in self-healing properties, contributing to the resilience of the gel during storage. Lastly, rigorous in-process quality checks and real-time stability studies should be conducted to determine the shelf-life and to establish appropriate storage guidelines that ensure the hydrogel will perform as intended for the duration of its use.
6. Conclusions
Among the various pharmaceutical formulations used for the topical administration of drugs, hydrogels stand out due to their excellent physical properties and high patient acceptability. Their close resemblance to physiological tissue, along with adjustable physicochemical properties, biocompatibility, and hydrophilicity, makes hydrogels excellent platforms for both anti-inflammatory action and drug delivery. This review concisely summarized the recent advances in hydrogels involved in topical anti-inflammatory therapy for psoriasis and AD, and highlighted the critical parameters necessary to understand, master, and control hydrogel systems effectively. Hydrogels can be synthesized from a variety of different natural, synthetic, and hybrid materials and can be engineered to include many desirable properties. Advancing this field will require collaborative efforts among material scientists, pharmacologists, and clinicians, promising new therapeutic options for patients suffering from inflammatory skin conditions.
Data availability
As this is a review paper, no new data were generated or analyzed during this study. All data discussed in this review are derived from previously published studies, which are cited appropriately in the manuscript. Readers interested in the original data can refer to the cited articles for more information.
Conflicts of interest
The authors declare no conflict of interest.
Acknowledgements
The authors acknowledge financial support from the NUS Start-up Grant, Zhejiang Provincial Natural Science Foundation of China under Grant No. LY19H110001 and the National Natural Science Foundation of China Youth Foundation under Grant No. 81602739.
References
- K. Kabashima, T. Honda, F. Ginhoux and G. Egawa, Nat. Rev. Immunol., 2019, 19, 19–30 CrossRef CAS PubMed.
- C. Karimkhani, R. P. Dellavalle, L. E. Coffeng, C. Flohr, R. J. Hay, S. M. Langan, E. O. Nsoesie, A. J. Ferrari, H. E. Erskine, J. I. Silverberg, T. Vos and M. Naghavi, JAMA Dermatol., 2017, 153, 406–412 CrossRef PubMed.
- E. Pezzolo and L. Naldi, Expert Rev. Clin. Immunol., 2020, 16, 155–166 CrossRef CAS PubMed.
- L. Eicher, M. Knop, N. Aszodi, S. Senner, L. E. French and A. Wollenberg, J. Eur. Acad. Dermatol. Venereol., 2019, 33, 2253–2263 CrossRef CAS PubMed.
- C. E. M. Griffiths, A. W. Armstrong, J. E. Gudjonsson and J. Barker, Lancet, 2021, 397, 1301–1315 CrossRef CAS PubMed.
- M. Mennini, G. Di Nardo and A. G. Fiocchi, Lancet, 2022, 400, 252–253 CrossRef PubMed.
- J. Takeshita, S. Grewal, S. M. Langan, N. N. Mehta, A. Ogdie, A. S. Van Voorhees and J. M. Gelfand, J. Am. Acad. Dermatol., 2017, 76, 377–390 CrossRef PubMed.
- P. Y. Ong and D. Y. Leung, Clin. Rev. Allergy Immunol., 2016, 51, 329–337 CrossRef CAS PubMed.
- A. W. Armstrong and C. Read, JAMA, 2020, 323, 1945–1960 CrossRef CAS PubMed.
- H. Chen, D. Mou, D. Du, X. Chang, D. Zhu, J. Liu, H. Xu and X. Yang, Int. J. Pharm., 2007, 341, 78–84 CrossRef CAS PubMed.
- J. I. Silverberg and E. L. Simpson, Dermatitis, 2014, 25, 107–114 CrossRef PubMed.
- E. L. M. M. Simpson, A. D. M. Irvine, L. F. M. Eichenfield and S. F. M. Friedlander, Semin. Cutaneous Med. Surg., 2016, 35, S84–88 CrossRef PubMed.
- T. Czarnowicki, D. Malajian, S. Khattri, J. Correa da Rosa, R. Dutt, R. Finney, N. Dhingra, P. Xiangyu, H. Xu, Y. D. Estrada, X. Zheng, P. Gilleaudeau, M. Sullivan-Whalen, M. Suarez-Farinas, A. Shemer, J. G. Krueger and E. Guttman-Yassky, J. Allergy Clin. Immunol.: Pract., 2016, 137, 1091–1102e1097 CrossRef CAS PubMed.
- S. S. Pukale, S. Sharma, M. Dalela, A. K. Singh, S. Mohanty, A. Mittal and D. Chitkara, Acta Biomater., 2020, 115, 393–409 CrossRef CAS PubMed.
- E. C. Siegfried, J. C. Jaworski, J. D. Kaiser and A. A. Hebert, BMC Pediatr., 2016, 16, 75 CrossRef PubMed.
- J. Callen, S. Chamlin, L. F. Eichenfield, C. Ellis, M. Girardi, M. Goldfarb, J. Hanifin, P. Lee, D. Margolis, A. S. Paller, D. Piacquadio, W. Peterson, K. Kaulback, M. Fennerty and B. U. Wintroub, Br. J. Dermatol., 2007, 156, 203–221 CrossRef CAS PubMed.
- P. M. Elias and J. S. Wakefield, Clin. Rev. Allergy Immunol., 2011, 41, 282–295 CrossRef CAS PubMed.
- L. Sun, Z. Liu, L. Wang, D. Cun, H. H. Y. Tong, R. Yan, X. Chen, R. Wang and Y. Zheng, J. Controlled Release, 2017, 254, 44–54 CrossRef CAS PubMed.
- K. Rana, T. Pani, S. K. Jha, D. Mehta, P. Yadav, D. Jain, M. K. Pradhan, S. Mishra, R. Kar, B. R. G
, A. Srivastava, U. Dasgupta, V. S. Patil and A. Bajaj, Nanoscale, 2022, 14, 3834–3848 RSC.
- I. M. Michalek, B. Loring and S. M. John, J. Eur. Acad. Dermatol. Venereol., 2017, 31, 205–212 CrossRef CAS PubMed.
- F. J. Dalgard, U. Gieler, L. Tomas-Aragones, L. Lien, F. Poot, G. B. E. Jemec, L. Misery, C. Szabo, D. Linder, F. Sampogna, A. W. M. Evers, J. A. Halvorsen, F. Balieva, J. Szepietowski, D. Romanov, S. E. Marron, I. K. Altunay, A. Y. Finlay, S. S. Salek and J. Kupfer, J. Invest. Dermatol., 2015, 135, 984–991 CrossRef CAS PubMed.
- M. Pasparakis, I. Haase and F. O. Nestle, Nat. Rev. Immunol., 2014, 14, 289–301 CrossRef CAS PubMed.
- K. Ghoreschi, A. Balato, C. Enerbäck and R. Sabat, Lancet, 2021, 397, 754–766 CrossRef CAS PubMed.
- A. Chiricozzi, E. Guttman-Yassky, M. Suarez-Farinas, K. E. Nograles, S. Tian, I. Cardinale, S. Chimenti and J. G. Krueger, J. Invest. Dermatol., 2011, 131, 677–687 CrossRef CAS PubMed.
- S. M. Langan, A. D. Irvine and S. Weidinger, Lancet, 2020, 396, 345–360 CrossRef CAS PubMed.
- A. Wollenberg, S. Barbarot, T. Bieber, S. Christen-Zaech, M. Deleuran, A. Fink-Wagner, U. Gieler, G. Girolomoni, S. Lau, A. Muraro, M. Czarnecka-Operacz, T. Schafer, P. Schmid-Grendelmeier, D. Simon, Z. Szalai, J. C. Szepietowski, A. Taieb, A. Torrelo, T. Werfel, J. Ring, t. E. A. o. D. European Dermatology Forum, t. E. A. o. A. Venereology, t. E. T. F. o. A. D. E. F. o. A. Clinical Immunology, t. E. S. f. D. Airways Diseases Patients' Associations, t. E. S. o. P. D. G. A. Psychiatry, N. Asthma European and S. the European Union of Medical, J. Eur. Acad. Dermatol. Venereol., 2018, 32, 657–682 CrossRef CAS PubMed.
- J. I. Silverberg, S. Barbarot, A. Gadkari, E. L. Simpson, S. Weidinger, P. Mina-Osorio, A. B. Rossi, L. Brignoli, G. Saba, I. Guillemin, M. C. Fenton, S. Auziere and L. Eckert, Ann. Allergy, Asthma, Immunol., 2021, 126, 417–428e412 CrossRef PubMed.
- T. Czarnowicki, H. He, J. G. Krueger and E. Guttman-Yassky, J. Allergy Clin. Immunol.: Pract., 2019, 143, 1–11 CrossRef PubMed.
- S. Ständer, N. Engl. J. Med., 2021, 384, 1136–1143 CrossRef PubMed.
- E. Goleva, E. Berdyshev and D. Y. Leung, J. Clin. Invest., 2019, 129, 1463–1474 CrossRef PubMed.
- L. F. Eichenfield, W. L. Tom, S. L. Chamlin, S. R. Feldman, J. M. Hanifin, E. L. Simpson, T. G. Berger, J. N. Bergman, D. E. Cohen, K. D. Cooper, K. M. Cordoro, D. M. Davis, A. Krol, D. J. Margolis, A. S. Paller, K. Schwarzenberger, R. A. Silverman, H. C. Williams, C. A. Elmets, J. Block, C. G. Harrod, W. Smith Begolka and R. Sidbury, J. Am. Acad. Dermatol., 2014, 70, 338–351 CrossRef PubMed.
- T. Tsakok, R. Woolf, C. H. Smith, S. Weidinger and C. Flohr, Br. J. Dermatol., 2019, 180, 464–474 CrossRef CAS PubMed.
- C. N. Palmer, A. D. Irvine, A. Terron-Kwiatkowski, Y. Zhao, H. Liao, S. P. Lee, D. R. Goudie, A. Sandilands, L. E. Campbell and F. J. Smith, Nat. Genet., 2006, 38, 441–446 CrossRef CAS PubMed.
- V. Soumelis, P. A. Reche, H. Kanzler, W. Yuan, G. Edward, B. Homey, M. Gilliet, S. Ho, S. Antonenko and A. Lauerma, Nat. Immunol., 2002, 3, 673–680 CrossRef CAS PubMed.
- J. Schmitz, A. Owyang, E. Oldham, Y. Song, E. Murphy, T. K. McClanahan, G. Zurawski, M. Moshrefi, J. Qin and X. Li, Immunity, 2005, 23, 479–490 CrossRef CAS PubMed.
- E. B. Brandt and U. Sivaprasad, J. Clin. Cell. Immunol., 2011, 2, 110–125 Search PubMed.
- Y. Renert-Yuval and E. Guttman-Yassky, Ann. Allergy, Asthma, Immunol., 2020, 124, 28–35 CrossRef CAS PubMed.
- J. P. Thyssen and P. Schmid-Grendelmeier, Lancet, 2023, 401, 172–173 CrossRef CAS PubMed.
- J. P. Thyssen and S. F. Thomsen, Lancet, 2021, 397, 2126–2128 CrossRef PubMed.
- R. Castillo-González, I. Fernández-Delgado and P. Comberiati, Allergy, 2022, 77, 1331–1333 CrossRef PubMed.
- S. J. Nolan and N. K. Archer, Cell Host Microbe, 2023, 31, 573–575 CrossRef CAS PubMed.
- T. Nakatsuji, T. R. Hata, Y. Tong, J. Y. Cheng, F. Shafiq, A. M. Butcher, S. S. Salem, S. L. Brinton, A. K. Rudman Spergel, K. Johnson, B. Jepson, A. Calatroni, G. David, M. Ramirez-Gama, P. Taylor, D. Y. M. Leung and R. L. Gallo, Nat. Med., 2021, 27, 700–709 CrossRef CAS PubMed.
- X. Liu, Y. Qin, L. Dong, Z. Han, T. Liu, Y. Tang, Y. Yu, J. Ye, J. Tao, X. Zeng, J. Feng and X. Z. Zhang, Bioact. Mater., 2023, 21, 253–266 CAS.
- T.-C. Ho, C.-C. Chang, H.-P. Chan, T.-W. Chung, C.-W. Shu, K.-P. Chuang, T.-H. Duh, M.-H. Yang and Y.-C. Tyan, Molecules, 2022, 27, 2902 CrossRef CAS PubMed.
- T. Liu, Y. Wang, J. Liu, X. Han, Y. Zou, P. Wang, R. Xu, L. Tong, J. Liu, J. Liang, Y. Sun, Y. Fan and X. Zhang, J. Mater. Chem. B, 2024, 12, 2282–2293 RSC.
- H. Cheng, M. A. A. Newton, M. Rajib, Q. Zhang, W. Gao, Z. Lu, Y. Zheng, Z. Dai and J. Zhu, J. Mater. Chem. B, 2024, 12, 2042–2053 RSC.
- P. C. Pires, F. Damiri, E. N. Zare, A. Hasan, R. E. Neisiany, F. Veiga, P. Makvandi and A. C. Paiva-Santos, Int. J. Biol. Macromol., 2024, 130296, DOI:10.1016/j.ijbiomac.2024.130296.
- E. N. Tessema, T. Gebre-Mariam, G. Paulos, J. Wohlrab and R. H. H. Neubert, Eur. J. Pharm. Biopharm., 2018, 127, 260–269 CrossRef CAS PubMed.
- Y. E. Kim, S. W. Choi, M. K. Kim, T. L. Nguyen and J. Kim, Nano Lett., 2022, 22, 2038–2047 CrossRef CAS PubMed.
- H. B. Eral, V. Lopez-Mejias, M. O'Mahony, B. L. Trout, A. S. Myerson and P. S. Doyle, Cryst. Growth Des., 2014, 14, 2073–2082 CrossRef CAS.
- Y. Jia, J. Hu, K. An, Q. Zhao, Y. Dang, H. Liu, Z. Wei, S. Geng and F. Xu, Nat. Commun., 2023, 14, 2478 CrossRef CAS PubMed.
- R. Khan and M. H. Khan, J. Ind. Soc. Periodontol., 2013, 17, 539–542 CrossRef PubMed.
- R. Naomi, H. Bahari, P. M. Ridzuan and F. Othman, Polymers, 2021, 13, 2319 CrossRef CAS PubMed.
- K. Panduranga Rao, J. Biomater. Sci., Polym. Ed., 1996, 7, 623–645 CrossRef PubMed.
- R. Naomi, H. Bahari, P. M. Ridzuan and F. Othman, Polymers, 2021, 13, 2319 CrossRef CAS PubMed.
- F. Valipour, E. Z. Rahimabadi and H. Rostamzad, Int. J. Biol. Macromol., 2023, 126704, DOI:10.1016/j.ijbiomac.2023.126704.
- G. S. Alvarez, C. Hélary, A. M. Mebert, X. Wang, T. Coradin and M. F. Desimone, J. Mater. Chem. B, 2014, 2, 4660–4670 RSC.
- K. Su and C. Wang, Biotechnol. Lett., 2015, 37, 2139–2145 CrossRef CAS PubMed.
- J. Mobika, M. Rajkumar, S. L. Sibi and V. N. Priya, Mater. Chem. Phys., 2020, 250, 123187 CrossRef CAS.
- T. Huang, Z.-C. Tu, X. Shangguan, X. Sha, H. Wang, L. Zhang and N. Bansal, Trends Food Sci. Technol., 2019, 86, 260–269 CrossRef CAS.
- A. B. Bello, D. Kim, D. Kim, H. Park and S. H. Lee, Tissue Eng., Part B, 2020, 26, 164–180 CrossRef CAS PubMed.
- Q. Chai, Y. Jiao and X. Yu, Gels, 2017, 3, 6 CrossRef PubMed.
- S. S. Ng, K. Su, C. Li, M. B. Chan-Park, D.-A. Wang and V. Chan, Acta Biomater., 2012, 8, 244–252 CrossRef CAS PubMed.
- G. T. Voss, M. J. Davies, C. H. Schiesser, R. L. de Oliveira, A. B. Nornberg, V. R. Soares, A. M. Barcellos, C. Luchese, A. R. Fajardo and E. A. Wilhelm, Int. J. Pharm., 2023, 123174, DOI:10.1016/j.ijpharm.2023.123174.
- C. Bonduelle, Polym. Chem., 2018, 9, 1517–1529 RSC.
- A. Dasgupta, J. H. Mondal and D. Das, RSC Adv., 2013, 3, 9117–9149 RSC.
- K. Bauri, M. Nandi and P. De, Polym. Chem., 2018, 9, 1257–1287 RSC.
- X. Li, J. Liu, H. Chen, Y. Chen, Y. Wang, C. Y. Zhang and X.-H. Xing, Green Chem. Eng., 2023, 4, 173–188 CrossRef.
- L. Cai, S. Liu, J. Guo and Y. G. Jia, Acta Biomater., 2020, 113, 84–100 CrossRef CAS PubMed.
- A. Copling, M. Akantibila, R. Kumaresan, G. Fleischer, D. Cortes, R. S. Tripathi, V. J. Carabetta and S. L. Vega, Int. J. Mol. Sci., 2023, 24, 7563 CrossRef CAS PubMed.
- F. Li, J. Tang, J. Geng, D. Luo and D. Yang, Prog. Polym. Sci., 2019, 98, 101163 CrossRef CAS.
- Y. Dong, C. Yao, Y. Zhu, L. Yang, D. Luo and D. Yang, Chem. Rev., 2020, 120, 9420–9481 CrossRef CAS PubMed.
- D. Wang, J. Duan, J. Liu, H. Yi, Z. Zhang, H. Song, Y. Li and K. Zhang, Adv. Healthcare Mater., 2023, e2203031, DOI:10.1002/adhm.202203031.
- D. Wang, Y. Hu, P. Liu and D. Luo, Acc. Chem. Res., 2017, 50, 733–739 CrossRef CAS PubMed.
- K. Nahar, K. Hossain and T. A. Khan, Res. J. Pharm. Technol., 2017, 10, 3195–3204 CrossRef.
- D. Kothale, U. Verma, N. Dewangan, P. Jana, A. Jain and D. Jain, Curr. Drug Delivery, 2020, 17, 755–775 CrossRef CAS PubMed.
- J. Sun and H. Tan, Materials, 2013, 6, 1285–1309 CrossRef CAS PubMed.
- H. R. Lee, T. H. Kim, S. H. Oh and J. H. Lee, J. Biomater. Sci., Polym. Ed., 2018, 29, 1612–1624 CrossRef CAS PubMed.
- A. Sionkowska, M. Gadomska, K. Musiał and J. Piątek, Molecules, 2020, 25, 4035 CrossRef CAS PubMed.
-
V. D. Prajapati and P. M. Maheriya, Functional polysaccharides for biomedical applications, Elsevier, 2019, pp. 213–265 Search PubMed.
- J. A. Burdick and G. D. Prestwich, Adv. Mater., 2011, 23, H41–H56 CrossRef CAS PubMed.
- M. Hemshekhar, R. M. Thushara, S. Chandranayaka, L. S. Sherman, K. Kemparaju and K. S. Girish, Int. J. Biol. Macromol., 2016, 86, 917–928 CrossRef CAS PubMed.
- S. N. A. Bukhari, N. L. Roswandi, M. Waqas, H. Habib, F. Hussain, S. Khan, M. Sohail, N. A. Ramli, H. E. Thu and Z. Hussain, Int. J. Biol. Macromol., 2018, 120, 1682–1695 CrossRef CAS PubMed.
- H. Kim, J. Cha, M. Jang and P. Kim, Biomater. Sci., 2019, 7, 2264–2271 RSC.
- Y. Zhang, Q. Xia, Y. Li, Z. He, Z. Li, T. Guo, Z. Wu and N. Feng, Theranostics, 2019, 9, 48–64 CrossRef CAS.
- L. Li, C. Liu, J. Fu, Y. Wang, D. Yang, B. Peng, X. Liu, X. Han, Y. Meng, F. Feng, X. Hu, C. Qi, Y. Wang, Y. Zheng and P. Li, Int. J. Biol. Macromol., 2023, 125239, DOI:10.1016/j.ijbiomac.2023.125239.
-
T. Heinze, Cellulose chemistry and properties: fibers, nanocelluloses and advanced materials, 2016, pp. 1–52 Search PubMed.
- B. Thomas, M. C. Raj, J. Joy, A. Moores, G. L. Drisko and C. Sanchez, Chem. Rev., 2018, 118, 11575–11625 CrossRef CAS.
- S. Bhaladhare and D. Das, J. Mater. Chem. B, 2022, 10, 1923–1945 RSC.
- M. Wang, R. Li, X. Feng, C. Dang, F. Dai, X. Yin, M. He, D. Liu and H. Qi, ACS Appl. Mater. Interfaces, 2020, 12, 27545–27554 CrossRef CAS PubMed.
- M. Oprea and S. I. Voicu, Carbohydr. Polym., 2020, 247, 116683 CrossRef CAS PubMed.
- K. Bolko Seljak, B. Sterle Zorec and M. Gosenca Matjaž, Pharmaceutics, 2023, 15, 1918 CrossRef CAS PubMed.
- P. Laurén, Y.-R. Lou, M. Raki, A. Urtti, K. Bergström and M. Yliperttula, Eur. J. Pharm. Sci., 2014, 65, 79–88 CrossRef PubMed.
- A. Gupta, S. M. Briffa, S. Swingler, H. Gibson, V. Kannappan, G. Adamus, M. Kowalczuk, C. Martin and I. Radecka, Biomacromolecules, 2020, 21, 1802–1811 CrossRef CAS PubMed.
- M. Wang, O. J. Rojas, L. Ning, Y. Li, X. Niu, X. Shi and H. Qi, Carbohydr. Polym., 2023, 301, 120330 CrossRef CAS PubMed.
- M. Wang, X. Feng, X. Wang, S. Hu, C. Zhang and H. Qi, J. Mater. Chem. A, 2021, 9, 24539–24547 RSC.
- P. Tripathi, A. Kumar, P. K. Jain and J. R. Patel, Int. J. Biol. Macromol., 2018, 120, 1322–1334 CrossRef CAS PubMed.
- F. Qiu, L. Xi, S. Chen, Y. Zhao, Z. Wang and Y. Zheng, Int. J. Nanomed., 2021, 16, 6171–6182 CrossRef PubMed.
- X. Lu, T. H. Perera, A. B. Aria and L. A. S. Callahan, J. Exp. Pharmacol., 2018, 10, 37–49 CrossRef CAS PubMed.
- J. M. Harris and R. B. Chess, Nat. Rev. Drug Discovery, 2003, 2, 214–221 CrossRef CAS PubMed.
- N. A. Peppas, K. B. Keys, M. Torres-Lugo and A. M. Lowman, J. Controlled Release, 1999, 62, 81–87 CrossRef CAS.
- M. S. Hahn, M. K. McHale, E. Wang, R. H. Schmedlen and J. L. West, Ann. Biomed. Eng., 2007, 35, 190–200 CrossRef PubMed.
- M. Malkoch, R. Vestberg, N. Gupta, L. Mespouille, P. Dubois, A. F. Mason, J. L. Hedrick, Q. Liao, C. W. Frank and K. Kingsbury, Chem. Commun., 2006, 2774–2776 RSC.
- J. Shi, L. Yu and J. Ding, Acta Biomater., 2021, 128, 42–59 CrossRef CAS PubMed.
- J. M. Harris and R. B. Chess, Nat. Rev. Drug Discovery, 2003, 2, 214–221 CrossRef CAS PubMed.
- C. Gong, Q. Wu, Y. Wang, D. Zhang, F. Luo, X. Zhao, Y. Wei and Z. Qian, Biomaterials, 2013, 34, 6377–6387 CrossRef CAS PubMed.
- D. Gabriel, T. Mugnier, H. Courthion, K. Kranidioti, N. Karagianni, M. C. Denis, M. Lapteva, Y. Kalia, M. Möller and R. Gurny, J. Controlled Release, 2016, 242, 16–24 CrossRef CAS PubMed.
- Y. Lu, Y. Xiao, M. Z. Yin, X. C. Zhou, L. S. Wu, W. Q. Chen, Y. Luo, Y. H. Kuang and W. Zhu, Front. Med., 2020, 7, 560579 CrossRef PubMed.
-
C. M. Hassan and N. A. Peppas, Biopolymers· PVA hydrogels, anionic polymerisation nanocomposites, 2000, pp. 37–65 Search PubMed.
- B. V. Slaughter, S. S. Khurshid, O. Z. Fisher, A. Khademhosseini and N. A. Peppas, Adv. Mater., 2009, 21, 3307–3329 CrossRef CAS PubMed.
- A. Kumar and S. S. Han, Int. J. Polym. Mater. Polym. Biomater., 2017, 66, 159–182 CrossRef CAS.
- R. Ricciardi, F. Auriemma, C. Gaillet, C. De Rosa and F. Lauprêtre, Macromolecules, 2004, 37, 9510–9516 CrossRef CAS.
- T. H. Kim, D. B. An, S. H. Oh, M. K. Kang, H. H. Song and J. H. Lee, Biomaterials, 2015, 40, 51–60 CrossRef CAS PubMed.
- M. Suneetha, D. Hemalatha, H. Kim, K. Rao and S. S. Han, Int. J. Biol. Macromol., 2024, 130910, DOI:10.1016/j.ijbiomac.2024.130910.
- L. Qiu, C. Ouyang, W. Zhang, J. Liu, L. Yu, G. Chen and L. Ren, J. Nanobiotechnol., 2023, 21, 163 CrossRef CAS PubMed.
- Z. Ge, W. Guo, Y. Tao, H. Sun, X. Meng, L. Cao, S. Zhang, W. Liu, M. L. Akhtar and Y. Li, Adv. Mater., 2023, 35, 2304005 CrossRef CAS PubMed.
- S. Thakur and O. A. Arotiba, Polym. Bull., 2018, 75, 4587–4606 CrossRef CAS.
- J. Fei, Z. Zhang, L. Zhong and L. Gu, J. Appl. Polym. Sci., 2002, 85, 2423–2430 CrossRef CAS.
- Y.-C. Nho, J.-S. Park and Y.-M. Lim, Polymers, 2014, 6, 890–898 CrossRef.
- M. Wang, Z. Lai, X. Jin, T. Sun, H. Liu and H. Qi, Adv. Funct. Mater., 2021, 31, 2101957 CrossRef CAS.
- V. D. Wagh, B. Inamdar and M. Samanta, Asian J. Pharm., 2008, 2, 12–17 CrossRef.
- G. Calixto, A. C. Yoshii, H. Rocha e Silva, B. Stringhetti Ferreira Cury and M. Chorilli, Pharm. Dev. Technol., 2015, 20, 490–496 CrossRef CAS PubMed.
- N. Parveen, A. Sheikh, N. Molugulu, S. Annadurai, S. Wahab and P. Kesharwani, Environ. Res., 2023, 116850, DOI:10.1016/j.envres.2023.116850.
- L. L. Palmese, R. K. Thapa, M. O. Sullivan and K. L. Kiick, Curr. Opin. Chem. Eng., 2019, 24, 143–157 CrossRef PubMed.
- F. Wu, Y. Pang and J. Liu, Nat. Commun., 2020, 11, 4502 CrossRef CAS PubMed.
- E. Koh, B. R. Freedman, F. Ramazani, J. Gross, A. Graham, A. Kuttler, E. Weber and D. J. Mooney, Adv. Healthcare Mater., 2022, e2201000, DOI:10.1002/adhm.202201000.
- Y. Qian, Y. Zheng, J. Jin, X. Wu, K. Xu, M. Dai, Q. Niu, H. Zheng, X. He and J. Shen, Adv. Mater., 2022, 34, e2200521 CrossRef.
- X. Chen, Y. Zhang, W. Yu, W. Zhang, H. Tang and W. E. Yuan, J. Nanobiotechnol., 2023, 21, 129 CrossRef CAS PubMed.
- S. Nam and D. Mooney, Chem. Rev., 2021, 121, 11336–11384 CrossRef CAS PubMed.
- J. Yang, R. Bai and Z. Suo, Adv. Mater., 2018, 30, e1800671 CrossRef PubMed.
- Y. Liang, X. Zhao, T. Hu, B. Chen, Z. Yin, P. X. Ma and B. Guo, Small, 2019, 15, e1900046 CrossRef PubMed.
- Z. Ma, C. Bourquard, Q. Gao, S. Jiang, T. De Iure-Grimmel, R. Huo, X. Li, Z. He, Z. Yang, G. Yang, Y. Wang, E. Lam, Z. H. Gao, O. Supponen and J. Li, Science, 2022, 377, 751–755 CrossRef CAS PubMed.
- B. R. Freedman, A. Kuttler, N. Beckmann, S. Nam, D. Kent, M. Schuleit, F. Ramazani, N. Accart, A. Rock, J. Li, M. Kurz, A. Fisch, T. Ullrich, M. W. Hast, Y. Tinguely, E. Weber and D. J. Mooney, Nat. Biomed. Eng., 2022, 6, 1167–1179 CrossRef CAS PubMed.
- S. J. Wu, H. Yuk, J. Wu, C. S. Nabzdyk and X. Zhao, Adv. Mater., 2021, 33, e2007667 CrossRef.
- H. Yuk, C. E. Varela, C. S. Nabzdyk, X. Mao, R. F. Padera, E. T. Roche and X. Zhao, Nature, 2019, 575, 169–174 CrossRef CAS.
- S. G. Danby, Z. D. Draelos, L. F. S. Gold, A. Cha, B. Vlahos, L. Aikman, P. Sanders, D. Wu-Linhares and M. J. Cork, J. Dermatol. Treat., 2022, 33, 685–698 CrossRef CAS PubMed.
- S. G. Danby, P. V. Andrew, L. J. Kay, A. Pinnock, J. Chittock, K. Brown, S. F. Williams and M. J. Cork, Br. J. Dermatol., 2022, 186, 875–886 CrossRef CAS PubMed.
- J. Chen, J. Bian, B. M. Hantash, L. Albakr, D. E. Hibbs, X. Xiang, P. Xie, C. Wu and L. Kang, Int. J. Pharm., 2021, 606, 120868 CrossRef CAS.
- L. H. Kircik, J. Drugs Dermatol., 2012, 11, 180–184 CAS.
- K. A. Greive and T. M. Barnes, Australas. J. Dermatol., 2016, 57, e39–45 CrossRef PubMed.
- L. Rüther and W. Voss, Heliyon, 2021, 7, e06071 CrossRef PubMed.
- J. Irianto, C. R. Pfeifer, Y. Xia and D. E. Discher, Cell, 2016, 165, 1820–1820.e1821 CrossRef CAS.
- T. Iskratsch, H. Wolfenson and M. P. Sheetz, Nat. Rev. Mol. Cell Biol., 2014, 15, 825–833 CrossRef CAS PubMed.
- H. Iijima, G. Gilmer, K. Wang, A. C. Bean, Y. He, H. Lin, W. Y. Tang, D. Lamont, C. Tai, A. Ito, J. J. Jones, C. Evans and F. Ambrosio, Nat. Commun., 2023, 14, 18 CrossRef CAS PubMed.
- X. Zhang, D. Cao, L. Xu, Y. Xu, Z. Gao, Y. Pan, M. Jiang, Y. Wei, L. Wang, Y. Liao, Q. Wang, L. Yang, X. Xu, Y. Gao, S. Gao, J. Wang and R. Yue, Cell Stem Cell, 2023, 30, 378–395.e378 CrossRef CAS PubMed.
- Y. Jiang, L. C. Tsoi, A. C. Billi, N. L. Ward, P. W. Harms, C. Zeng, E. Maverakis, J. M. Kahlenberg and J. E. Gudjonsson, JCI Insight, 2020, 5, e142067 CrossRef PubMed.
- S. E. Reid, E. J. Kay, L. J. Neilson, A. T. Henze, J. Serneels, E. J. McGhee, S. Dhayade, C. Nixon, J. B. Mackey, A. Santi, K. Swaminathan, D. Athineos, V. Papalazarou, F. Patella, Á. Román-Fernández, Y. ElMaghloob, J. R. Hernandez-Fernaud, R. H. Adams, S. Ismail, D. M. Bryant, M. Salmeron-Sanchez, L. M. Machesky, L. M. Carlin, K. Blyth, M. Mazzone and S. Zanivan, EMBO J., 2017, 36, 2373–2389 CrossRef CAS PubMed.
- Q. Wei, J. Young, A. Holle, J. Li, K. Bieback, G. Inman, J. P. Spatz and E. A. Cavalcanti-Adam, ACS Biomater. Sci. Eng., 2020, 6, 4687–4701 CrossRef CAS PubMed.
- W. W. Chen, M. S. Tjin, A. W. C. Chua, S. T. Lee, C. Y. Tay and E. Fong, ACS Appl. Mater. Interfaces, 2017, 9, 36483–36492 CrossRef CAS PubMed.
- C. M. Madl, B. L. LeSavage, R. E. Dewi, C. B. Dinh, R. S. Stowers, M. Khariton, K. J. Lampe, D. Nguyen, O. Chaudhuri, A. Enejder and S. C. Heilshorn, Nat. Mater., 2017, 16, 1233–1242 CrossRef CAS PubMed.
- P. A. Parmar, S. C. Skaalure, L. W. Chow, J. P. St-Pierre, V. Stoichevska, Y. Y. Peng, J. A. Werkmeister, J. A. Ramshaw and M. M. Stevens, Biomaterials, 2016, 99, 56–71 CrossRef CAS PubMed.
- D. T. Wu, N. Jeffreys, M. Diba and D. J. Mooney, Tissue Eng., Part C, 2022, 28, 289–300 CrossRef CAS PubMed.
- M. Chen, Y. Zhang, P. Zhou, X. Liu, H. Zhao, X. Zhou, Q. Gu, B. Li, X. Zhu and Q. Shi, Bioact. Mater., 2020, 5, 880–890 Search PubMed.
- J. Li and D. J. Mooney, Nat. Rev. Mater., 2016, 1, 16071 CrossRef CAS PubMed.
- M. Bao, J. Xie, A. Piruska and W. T. S. Huck, Nat. Commun., 2017, 8, 1962 CrossRef PubMed.
- Y. Liu, A. Suarez-Arnedo, E. L. P. Caston, L. Riley, M. Schneider and T. Segura, Adv. Mater., 2023, e2304049, DOI:10.1002/adma.202304049.
- R. Censi, T. Vermonden, M. J. van Steenbergen, H. Deschout, K. Braeckmans, S. C. De Smedt, C. F. van Nostrum, P. di Martino and W. E. Hennink, J. Controlled Release, 2009, 140, 230–236 CrossRef CAS PubMed.
- O. Chaudhuri, L. Gu, M. Darnell, D. Klumpers, S. A. Bencherif, J. C. Weaver, N. Huebsch and D. J. Mooney, Nat. Commun., 2015, 6, 6364 CrossRef PubMed.
- A. Saraswathibhatla, D. Indana and O. Chaudhuri, Nat. Rev. Mol. Cell Biol., 2023, 24, 495–516 CrossRef CAS PubMed.
- O. Chaudhuri, L. Gu, D. Klumpers, M. Darnell, S. A. Bencherif, J. C. Weaver, N. Huebsch, H. P. Lee, E. Lippens, G. N. Duda and D. J. Mooney, Nat. Mater., 2016, 15, 326–334 CrossRef CAS PubMed.
- B. Yang, K. Wei, C. Loebel, K. Zhang, Q. Feng, R. Li, S. H. D. Wong, X. Xu, C. Lau, X. Chen, P. Zhao, C. Yin, J. A. Burdick, Y. Wang and L. Bian, Nat. Commun., 2021, 12, 3514 CrossRef CAS PubMed.
- A. Elosegui-Artola, A. Gupta, A. J. Najibi, B. R. Seo, R. Garry, C. M. Tringides, I. de Lázaro, M. Darnell, W. Gu, Q. Zhou, D. A. Weitz, L. Mahadevan and D. J. Mooney, Nat. Mater., 2022, 22, 117–127 CrossRef PubMed.
- K. Adu-Berchie, Y. Liu, D. K. Y. Zhang, B. R. Freedman, J. M. Brockman, K. H. Vining, B. A. Nerger, A. Garmilla and D. J. Mooney, Nat. Biomed. Eng., 2023, 7, 1374–1391 CrossRef CAS PubMed.
- B. Trappmann, B. M. Baker, W. J. Polacheck, C. K. Choi, J. A. Burdick and C. S. Chen, Nat. Commun., 2017, 8, 371 CrossRef PubMed.
- T. M. O'Shea, A. A. Aimetti, E. Kim, V. Yesilyurt and R. Langer, Adv. Mater., 2015, 27, 65–72 CrossRef PubMed.
- C. M. L. Lau, G. Jahanmir, Y. Yu and Y. Chau, J. Controlled Release, 2021, 335, 75–85 CrossRef CAS PubMed.
- X. Hu, S. R. Roy, C. Jin, G. Li, Q. Zhang, N. Asano, S. Asahina, T. Kajiwara, A. Takahara, B. Feng, K. Aoki, C. Xu and Y. Zhang, Nat. Commun., 2022, 13, 5002 CrossRef CAS PubMed.
- D. Indana, P. Agarwal, N. Bhutani and O. Chaudhuri, Adv. Mater., 2021, 33, e2101966 CrossRef PubMed.
- U. Freudenberg, A. Zieris, K. Chwalek, M. V. Tsurkan, M. F. Maitz, P. Atallah, K. R. Levental, S. A. Eming and C. Werner, J. Controlled Release, 2015, 220, 79–88 CrossRef CAS PubMed.
- S. Traub, J. Morgner, M. M. Martino, S. Höning, M. A. Swartz, S. A. Wickström, J. A. Hubbell and S. A. Eming, Biomaterials, 2013, 34, 5958–5968 CrossRef CAS PubMed.
- S. Yu, C. Wang, J. Yu, J. Wang, Y. Lu, Y. Zhang, X. Zhang, Q. Hu, W. Sun, C. He, X. Chen and Z. Gu, Adv. Mater., 2018, 30, e1801527 CrossRef PubMed.
- J. Kim, D. M. Francis, L. F. Sestito, P. A. Archer, M. P. Manspeaker, M. J. O’Melia and S. N. Thomas, Nat. Commun., 2022, 13, 1497 CrossRef PubMed.
- J. Kim, D. M. Francis and S. N. Thomas, Nanomaterials, 2021, 11, 471 CrossRef CAS PubMed.
- X. Du, J. Zhou, J. Shi and B. Xu, Chem. Rev., 2015, 115, 13165–13307 CrossRef CAS PubMed.
- M. E. Wechsler, R. E. Stephenson, A. C. Murphy, H. F. Oldenkamp, A. Singh and N. A. Peppas, Biomed. Microdevices, 2019, 21, 31 CrossRef PubMed.
- A. Erfani, J. Seaberg, C. P. Aichele and J. D. Ramsey, Biomacromolecules, 2020, 21, 2557–2573 CrossRef CAS PubMed.
- Z. Y. Wei, S. Q. Wang, J. Hirvonen, H. A. Santos and W. Li, Adv. Healthcare Mater., 2022, 11, 2200846 CrossRef CAS PubMed.
- H. Labie and M. Blanzat, Biomater. Sci., 2023, 11, 4073–4093 RSC.
- M. K. Gupta, J. R. Martin, B. R. Dollinger, M. E. Hattaway and C. L. Duvall, Adv. Funct. Mater., 2017, 27, 1704107 CrossRef PubMed.
- J. Li, Q. Ding, H. Wang, Z. Wu, X. Gui, C. Li, N. Hu, K. Tao and J. Wu, Nano-Micro Lett., 2023, 15, 105 CrossRef CAS PubMed.
- G. Gallo, L. Mastorino, D. Barilà, F. Cattel, M. Panzone, P. Quaglino, S. Ribero and P. Dapavo, Dermatol. Ther., 2022, e15917, DOI:10.1111/dth.15917.
- S. Del Río-Sancho, M. Lapteva, K. Sonaje, C. Böhler, V. Ling, W. H. Boehncke and Y. N. Kalia, Int. J. Pharm., 2020, 580, 119234 CrossRef PubMed.
- T. Patel, T. Bhutani, K. L. Busse and J. Koo, Cutis, 2011, 88, 149–154 Search PubMed.
- A. Menter, N. J. Korman, C. A. Elmets, S. R. Feldman, J. M. Gelfand, K. B. Gordon, A. Gottlieb, J. Y. Koo, M. Lebwohl, H. W. Lim, A. S. Van Voorhees, K. R. Beutner, R. Bhushan and D. American Academy of, J. Am. Acad. Dermatol., 2009, 60, 643–659 CrossRef PubMed.
- A. Ormerod, A. Winfield, A. Priprem, R. Moody, N. Graham and C. Moran, J. Dermatol. Treat., 1992, 2, 133–136 CrossRef PubMed.
- B. Kumar, K. Sandhu and I. Kaur, J. Dermatol., 2004, 31, 798–801 CrossRef CAS PubMed.
- M. Bohannon, M. Liu, P. Nadeau, J. Talton, D. Gibson, S. Datta, G. Schultz, J. Talton and A. De Benedetto, Exp. Dermatol., 2020, 29, 1171–1175 CrossRef CAS PubMed.
- E. C. Dobrică, M. A. Cozma, M. A. Găman, V. M. Voiculescu and A. M. Găman, Antioxidants, 2022, 11, 282 CrossRef PubMed.
- S. Galiniak, M. Mołoń, M. Biesiadecki, A. Bożek and M. Rachel, Antioxidants, 2022, 11, 1590 CrossRef CAS PubMed.
- Y. E. Kim and J. Kim, ACS Appl. Mater. Interfaces, 2022, 14, 23002–23021 CrossRef CAS PubMed.
- J. Xu, H. Chen, Z. Chu, Z. Li, B. Chen, J. Sun, W. Lai, Y. Ma, Y. He, H. Qian, F. Wang and Y. Xu, J. Nanobiotechnol., 2022, 20, 155 CrossRef CAS PubMed.
- E. He, H. Li, X. Li, X. Wu, K. Lei and Y. Diao, Int. J. Mol. Sci., 2022, 23, 3798 CrossRef CAS PubMed.
- H. K. Noddeland, M. Lind, L. B. Jensen, K. Petersson, T. Skak-Nielsen, F. H. Larsen, M. Malmsten and A. Heinz, Acta Biomater., 2023, 157, 149–161 CrossRef CAS PubMed.
- A. Tsianakas, P. M. Brunner, K. Ghoreschi, C. Berger, K. Loser, M. Röcken, G. Stingl, T. Luger and T. Jung, Exp. Dermatol., 2016, 25, 428–433 CrossRef CAS PubMed.
- A. Menter, B. E. Strober, D. H. Kaplan, D. Kivelevitch, E. F. Prater, B. Stoff, A. W. Armstrong, C. Connor, K. M. Cordoro, D. M. R. Davis, B. E. Elewski, J. M. Gelfand, K. B. Gordon, A. B. Gottlieb, A. Kavanaugh, M. Kiselica, N. J. Korman, D. Kroshinsky, M. Lebwohl, C. L. Leonardi, J. Lichten, H. W. Lim, N. N. Mehta, A. S. Paller, S. L. Parra, A. L. Pathy, R. N. Rupani, M. Siegel, E. B. Wong, J. J. Wu, V. Hariharan and C. A. Elmets, J. Am. Acad. Dermatol., 2019, 80, 1029–1072 CrossRef PubMed.
- C. E. Griffiths, K. Reich, M. Lebwohl, P. van de Kerkhof, C. Paul, A. Menter, G. S. Cameron, J. Erickson, L. Zhang, R. J. Secrest, S. Ball, D. K. Braun, O. O. Osuntokun, M. P. Heffernan, B. J. Nickoloff, K. Papp, Uncover and U. investigators, Lancet, 2015, 386, 541–551 CrossRef CAS PubMed.
- K. A. Papp, M. A. Weinberg, A. Morris and K. Reich, Lancet, 2021, 397, 1564–1575 CrossRef CAS PubMed.
- P. Gisondi, D. Geat, M. Pizzolato and G. Girolomoni, Curr. Opin. Pharmacol., 2019, 46, 90–99 CrossRef CAS PubMed.
- J. E. Hawkes, T. C. Chan and J. G. Krueger, J. Allergy Clin. Immunol.: Pract., 2017, 140, 645–653 CrossRef CAS PubMed.
- A. M. Lé and T. Torres, Am. J. Clin. Dermatol., 2022, 23, 13–24 CrossRef PubMed.
- R. Sinclair, S. Sharifeh, S. Thackwray, J. Lickliter, J. Wu, J. Li, B. Qi, P. Bland-Ward and H. Reinhart, Exp. Dermatol., 2023, 32, 1538–1545 CrossRef CAS PubMed.
- K. Papp, J. C. Szepietowski, L. Kircik, D. Toth, L. F. Eichenfield, D. Y. M. Leung, S. B. Forman, M. E. Venturanza, K. Sun, M. E. Kuligowski and E. L. Simpson, J. Am. Acad. Dermatol., 2021, 85, 863–872 CrossRef CAS PubMed.
- S. Dhillon, Drugs, 2020, 80, 609–615 CrossRef CAS PubMed.
- D. Kwiatkowska and A. Reich, Br. J. Dermatol., 2023, 189, 359–360 CrossRef PubMed.
- T. Bieber, N. Engl. J. Med., 2021, 385, 2295–2296 CrossRef PubMed.
- J. Zhang, L. Cai and M. Zheng, J. Am. Acad. Dermatol., 2022, 86, e137–e138 CrossRef CAS PubMed.
- E. G. Kang, S. Wu, A. Gupta, Y. L. von Mackensen, H. Siemetzki, J. M. Freudenberg, W. Wigger-Alberti and Y. Yamaguchi, Br. J. Dermatol., 2018, 178, 1427–1429 CrossRef CAS PubMed.
- A. Mandal, N. Kumbhojkar, C. Reilly, V. Dharamdasani, A. Ukidve, D. E. Ingber and S. Mitragotri, Sci. Adv., 2020, 6, eabb6049 CrossRef CAS PubMed.
- A. Vitória Pupo Silvestrini, F. Garcia Praça, M. Nani Leite, M. Carvalho de Abreu Fantini, M. Andrey Cipriani Frade and M. Vitória Lopes Badra Bentley, Int. J. Pharm., 2023, 123019, DOI:10.1016/j.ijpharm.2023.123019.
- W. Farhat, A. Hasan, L. Lucia, F. Becquart, A. Ayoub and F. Kobeissy, IEEE Rev. Biomed. Eng., 2019, 12, 333–351 Search PubMed.
- U. Blache and M. Ehrbar, Adv. Wound Care, 2018, 7, 232–246 CrossRef PubMed.
- H. Wang, D. Sun, W. Lin, C. Fang, K. Cheng, Z. Pan, D. Wang, Z. Song and X. Long, Bioact. Mater., 2023, 28, 420–431 CAS.
- G. Theocharidis, S. Rahmani, S. Lee, Z. Li, A. Lobao, K. Kounas, X. L. Katopodi, P. Wang, S. Moon, I. S. Vlachos, M. Niewczas, D. Mooney and A. Veves, Biomaterials, 2022, 288, 121692 CrossRef CAS PubMed.
- S. Cascone and G. Lamberti, Int. J. Pharm., 2020, 573, 118803 CrossRef CAS PubMed.
- R. Saka, H. Jain, N. Kommineni, N. Chella and W. Khan, J. Drug Delivery Sci. Technol., 2020, 58, 101691 CrossRef CAS.
- E. P. Vasyuchenko, P. S. Orekhov, G. A. Armeev and M. E. Bozdaganyan, Pharmaceutics, 2021, 13, 66 CrossRef CAS PubMed.
- L. Kircik, A. F. Alexis, A. Andriessen, C. Blattner, B. P. Glick, C. W. Lynde and L. S. Gold, J. Drugs Dermatol., 2023, 22, 773–778 CrossRef PubMed.
- R. A. Shalaby, O. El-Gazayerly and M. Abdallah, Int. J. Nanomed., 2022, 17, 1659–1677 CrossRef CAS PubMed.
- A. Farjami, S. Salatin, S. Jafari, M. Mahmoudian and M. Jelvehgari, Curr. Pharm. Des., 2021, 27, 4315–4329 CrossRef CAS PubMed.
- H. Du, J. Yang, M. Li, Y. Xia, Y. Li, J. Zhu, L. Zhang and J. Tao, J. Mater. Chem. B, 2024, 12, 2618–2627 RSC.
- Y. Jiang, N. Krishnan, J. Heo, R. H. Fang and L. Zhang, J. Controlled Release, 2020, 324, 505–521 CrossRef CAS PubMed.
- S. Y. Kim, H. Y. Shin, J. Y. Kim and S. J. Park, Molecules, 2023, 28, 806 CrossRef CAS PubMed.
- D. Larrea-Wachtendorff, V. Del Grosso and G. Ferrari, Gels, 2022, 8, 152 CrossRef CAS PubMed.
Footnote |
† These authors contributed equally to this work. |
|
This journal is © The Royal Society of Chemistry 2024 |
Click here to see how this site uses Cookies. View our privacy policy here.