DOI:
10.1039/D4QI01234H
(Research Article)
Inorg. Chem. Front., 2024,
11, 5286-5298
Efficient electrochemical coupling of nitrate and biomass-derived acetone to acetoxime at a high current density over a Zn/Cu hexagonal nanosheet catalyst†
Received
16th May 2024
, Accepted 19th June 2024
First published on 20th June 2024
Abstract
Acetoxime, as an important type of organic compound containing a C
N bond, is commonly employed as a boiler chemical deoxygenation agent and boiler acid pickling passivator due to its low toxicity and high reducibility characteristics. The conventional synthesis of acetoxime involves the initial catalysis of NOx and H2 under harsh conditions by noble metal catalysts to generate unstable NH2OH, which further reacts with acetone to form acetoxime. Herein, we report a Zn/Cu/CF hexagonal ultrathin nanosheet catalyst capable of efficiently catalyzing the coupling reaction between NH2OH produced in situ from electrocatalytic NO3− reduction and CH3COCH3 to generate an oxime under ambient conditions. The presence of Cu facilitates the conversion of NO3−, while the introduction of Zn is favourable to accelerate the electron transfer rate, enhance the number of active sites, and suppress the reduction hydrogenation of *CH3COCH3, thereby significantly improving the activity of oxime. Through catalyst screening and the optimization of electrolysis conditions, a faradaic efficiency of 44.5% with a yield rate of 415.5 μmol cm−2 h−1 is achieved at an industrially relevant current density of −150 mA cm−2 in the electrolyte containing 0.2 M NO3− + 0.2 M CH3COCH3 over the Zn/Cu-CF-6 electrocatalyst, corresponding to a C selectivity approaching 100%. A series of control experiments and in situ characterization studies indicate that *NH2OH and *CH3COCH3 are crucial intermediates for the synthesis of acetoxime, proposing a plausible reaction pathway. This work presents a facile synthesis method for Zn/Cu/CF hexagonal nanosheet catalysts and proposes a green strategy for the one-pot efficient electrochemical synthesis of acetoxime from pollutants NO3− and biomass-derived acetone, offering a green pathway for high-value-added C
N product synthesis.
10th Anniversary Statement
The launch of this journal a decade ago has not only provided an exceptional platform for inorganic chemists to exchange cutting-edge research results, but also greatly promoted the rapid development of inorganic chemistry based interdisciplinary research worldwide. Looking to the years ahead, it is my hope that this journal will become a promising reservoir of knowledge for demonstrating how inorganic chemistry can contribute to sustainable development and continue a great success story too.
|
Introduction
Acetoxime, a significant chemical raw material, is employed in a multitude of industrial applications.1–3 (1) Due to its low toxicity and high reducibility, it is commonly employed as a boiler chemical deoxygenation agent, replacing the more toxic hydrazine;4,5 (2) as a passivator after boiler acid pickling, it effectively prevents secondary corrosion of metals, and avoids the use of toxic passivators such as ammonia and sodium nitrite;6 (3) as an organic synthesis intermediate, it belongs to a crucial class of organonitrogen compounds used in pharmaceuticals, pesticides, fuels, and organosilane coupling agent synthesis;7,8 (4) it is also used as an analytical reagent for measuring Ni and Co metal elements.9,10 Currently, the traditional synthesis of acetoxime mainly involves two steps. The initial step is to utilize a reducing agent such as H2 under the catalysis of the noble metal catalyst Pd to reduce nitrogen oxides (NOx) to NH2OH (route 1 in Fig. 1a).11,12 Due to its easy decomposition and explosive properties,13 hydroxylamine requires to be further neutralized with H2SO4 or HCl to obtain hydroxylamine hydrochloride or hydroxylamine sulfate for easy storage and transportation, alternatively, the Raschig process can be employed to reduce bisulfite and nitrite to hydroxylamine sulfate. The above strategies lead to low yield, high energy consumption and more waste emission.14–16 The second step involves the reaction of acetone with hydroxylamine hydrochloride or hydroxylamine sulfate under alkaline conditions to produce acetoxime.17,18 Apparently, these methods involve complex procedures, the use of toxic reducing agents or noble metal catalysts, and the generation of large amounts of chloride or sulfate by-products.19 Recently, researchers have developed alternative strategies, and acetone ammoxidation is a commonly used method in the industry,20 where H2 and O2 are first synthesized into oxidant H2O2 using a Pd catalyst under high temperature and pressure, and then, NH3 is oxidized by H2O2 to generate NH2OH in situ over a titanium silicalite molecular sieve (TS-1) catalyst, which further nucleophilically attacks acetone to produce acetoxime21–23 (route 2 in Fig. 1a). However, this strategy requires excess NH3 and H2O2 and suffers from slow kinetics which needs to be compensated by raising the temperature and pressure, leading to more energy consumption.24 Moreover, NH3 is usually produced by energy-intensive industries,25–27 making the strategy of synthesizing acetoxime from NH3 and acetone less environmentally friendly. Therefore, there is an urgent need to establish a green synthesis pathway for acetoxime and develop low-cost, high-performance electrocatalysts to achieve energy-saving processes for one-pot synthesis of acetoxime from environmental waste NOx and biomass-derived acetone.
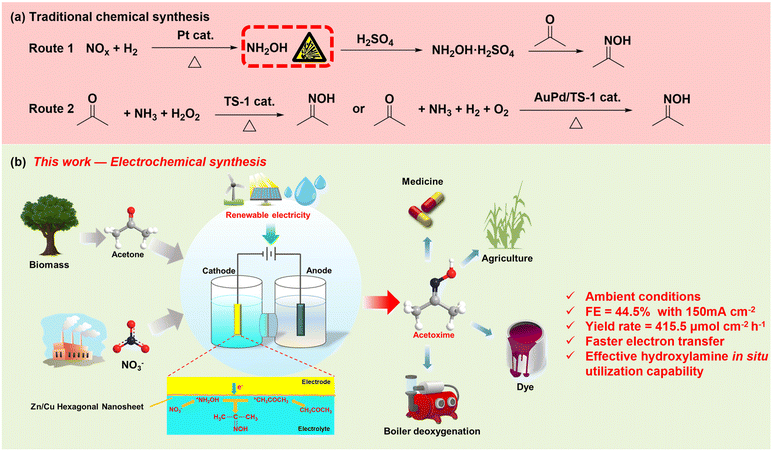 |
| Fig. 1 (a) Schematic diagram of two traditional routes for the synthesis of acetoxime. (b) Schematic diagram of direct electrosynthesis of acetoxime from waste nitrate and biomass-derived acetone driven by renewable energy sources. | |
Global warming and energy crises, exacerbated by fossil fuel consumption, as well as environmental pollution, necessitate a transition in modern industry from fossil fuel dependency to using renewable energy sources.28,29 Electrocatalytic technology, considered as an important means to alleviate energy and environmental issues, driving green innovations in the synthesis of high-value compounds,30,31 has been extensively researched in recent years.32,33 Among the compounds, organonitrogen compounds driven by renewable energy have attracted widespread attention due to their significant academic research and economic application value.34–42 Currently, the top 200 pharmaceuticals and the top ten agricultural chemicals globally are organonitrogen compounds.43 Since Jiao successfully constructed acetamide from CO and NH3 using an electrocatalytic method over Cu-based catalysts,44 the green synthesis of organonitrogen compounds using readily available carbon/nitrogen-containing small molecules under mild conditions has become an emerging field.8,45–48 In the field of electrosynthesis of oximes, researchers have successfully synthesized cyclohexanone oxime,49,50 benzaldoxime,51 formaldoxime,52 cyclopentanoxime,53 and pralidoxime42 from C-containing (cyclohexanone, benzaldehyde, formaldehyde, acetone, pyridine aldehyde) and N-containing (NO3−, NO2−, NO) small molecules as reactants. Although there has been some progress in the electrocatalytic C–N/C
N field, this reaction typically entails multi-step elementary reactions, rendering it a complex catalytic system. For instance, challenges such as preventing the individual reduction of the N or C source to produce separate N/C-containing products, regulating the formation of high-value C
N bond oximes from unstable key intermediates, and inhibiting the further reduction of oximes to secondary amines under a high current density all need to be addressed.38,54 Hence, establishing a suitable electrocatalytic system and comprehending the mechanism steps involved in the co-catalysis of NO3− and CH3COCH3 through screening and designing efficient electrocatalysts are crucial for enhancing the electrochemical activity of the desired product.
In this study, we report an efficient electrochemical approach for the one-pot synthesis of acetoxime from nitrate and acetone by employing foam copper-based hexagonal Zn/Cu nanosheets (Zn/Cu/CF) as the working electrode (Fig. 1b). This process utilizes water as the proton source and achieves the green electrosynthesis of acetoxime under mild conditions. Electrochemical characterization studies indicate that Zn/Cu/CF nanosheets possess a larger electrochemically active surface area and a faster electron transfer rate, enabling the efficient electrochemical synthesis of acetoxime by capturing *NH2OH generated in situ from NO3− electroreduction by *CH3COCH3. Through a series of condition optimizations, a faradaic efficiency of 44.5% and a yield rate of 415.5 μmol cm−2 h−1 are achieved at a current density of −150 mA cm−2. Additionally, under such an industrial-relevant current density (>100 mA cm−2), oximes are not further reduced to secondary amines, and the C selectivity of acetoxime in the carbon-containing liquid products approaches 100% (with minimal isopropanol production, FE < 1%). A continuous 10-cyclic test and catalyst characterization results after the reaction demonstrate high catalyst durability. Results of a series of control experiments, in situ electrochemical attenuated total reflectance-infrared absorption spectroscopy (ATR-IRAS), and online differential electrochemical mass spectrometry (DEMS) indicate that *NH2OH generated in situ from NO3− nucleophilically attacks *CH3COCH3 to produce the target product, oxime. The introduction of Zn not only transforms the morphology of Cu nanowires into Zn/Cu hexagonal nanosheets, but also boosts the electrochemically active surface area, accelerates electron transfer, enhances *NH2OH generation, diminishes *CH3COCH3 hydrogenation, and thus improves the electrosynthesis performance of acetoxime.
Results and discussion
Materials synthesis and characterization
Electrocatalytic co-reduction of acetone and NO3− for C
N coupling selective formation of oximes (e-NARR) was tested in an H-type cell containing 30 mL of 0.5 M KOH electrolyte, where both acetone and NO3− were added at a concentration of 0.2 M. At the initial stage of our investigation, we discovered that acetoxime possessed two chemical environments for its methyl groups under strong alkaline conditions, corresponding to the appearance of double peaks (∼1.72, 1.67 ppm) of the 1H nuclear magnetic resonance (1H NMR) spectrum. However, as the pH of the electrolyte gradually decreased, the proton promoted the cis–trans isomerization of the C
N double bond and the chemical environment of the two methyl groups of acetoxime tended to be the same, and thus, only one peak (∼1.78 ppm) could be observed from the 1H NMR spectra (Fig. S1†). In view of this, to standardize the quantitative results, the electrolyte was bubbled with CO2 to a pH of approximately 8 at the end of each test. At a constant current density of −100 mA cm−2, Co, Fe, Ag, Zn, Pb, Sn, Pt, Ni, and Cu catalysts were screened via a 2 h electrochemical performance test. Acetoxime and isopropanol were the only two categories of liquid C-containing products identified by 1H NMR. Therefore, standard curves of isopropanol and acetoxime at different concentrations were obtained by 400M 1H NMR for the qualitative analysis of carbon-containing liquid products (Fig. S2†). Among the screened metal catalysts, Zn (FE = 19.2%, yield rate = 119.5 μmol cm−2 h−1) and Cu (FE = 13.9%, yield rate = 86.6 μmol cm−2 h−1) exhibited the most favourable performance, with a C selectivity approaching 100% (Fig. S3 and 4†). Although the Zn catalyst exhibits optimal performance in catalyzing the synthesis of acetoxime from acetone and NO3− undergoing C
N coupling, Zn is an amphoteric metal which reacts with OH− in alkaline systems to produce metazincate (ZnO22−) dissolved in the electrolyte, diminishing the durability of the catalyst (<4 h at −100 mA cm−2) (Fig. S5†).
Accordingly, this work envisages well-designed Zn/Cu-based nanocatalysts that are expected to accelerate the electron transfer rate, enhance the reaction kinetics, increase the electrochemically active sites of the catalyst to improve the performance of acetoxime electrosynthesis and prolong the lifetime of the catalyst. Consequently, a combination procedure of alkaline etching, wet impregnation, calcination, and in situ electrochemical conversion was employed to prepare Zn/Cu hexagonal nanosheet electrocatalysts (Fig. 2a), which was achieved by first utilizing porous copper foam (CF) with a larger specific surface area as the substrate, followed by treatment of the CF with an alkaline oxidative etching solution (AOES) until a light blue color appeared on the surface55 (Fig. S6–8†). X-ray diffraction (XRD) and scanning electron microscopy (SEM) results demonstrated that Cu(OH)2 nanowires were obtained after etching (Fig. S9 and S10†). Cu(OH)2 nanowires were then immersed in a Zn2+ solution for different periods (3, 6, 12 h) to facilitate the attachment and deposition of Zn ions. The results of SEM images revealed that a transformation was observed in the structural arrangement of Cu(OH)2/CF nanowires, undergoing a transition to that of Zn2+/Cu(OH)2/CF hexagonal ultrathin nanosheets (Fig. S11†), which may be more favourable for the adsorption of reactants and electron transfer, and the XRD results indicated the presence of Zn(OH)2/Cu(OH)2 over the catalyst surface (Fig. S12†), possibly attributed to a partial hydrolysis reaction of Zn2+ during the process. The samples were then named according to the different impregnation times, respectively, Zn2+/Cu(OH)2/CF-3, Zn2+/Cu(OH)2/CF-6, and Zn2+/Cu(OH)2/CF-12. In order to stabilize Zn2+ on the surface of Zn2+/Cu(OH)2/CF, calcination was carried out at 220 °C under an air atmosphere for a period of two hours. The XRD results demonstrated that the phases on the surface of the catalysts had undergone a transformation into ZnO and CuO (Fig. S13†), and the SEM images showed that the catalysts retained their hexagonal nanosheet morphology and the stacked agglomerations of multiple nanosheets transformed into nanoflowers (Fig. 2b and S14†). The materials obtained at this juncture were named ZnO/CuO/CF-3, ZnO/CuO/CF-6, and ZnO/CuO/CF-12, respectively. Subsequently, the obtained materials were in situ reduced with 0.25 M Na2SO4 as the electrolyte at a current density of −36 mA cm−2 for 45 minutes. The SEM results demonstrated that their morphology retained the hexagonal nanosheet structure (Fig. 2c and S15†), with the nanoflower structure of the clusters unfolding, possibly exposing an increased number of electrochemically active sites. XRD analysis revealed that the phases of catalysts had transformed into Zn and Cu metallic states at this stage (Fig. 2d). The Zn/Cu/CF catalysts were finally obtained, which were designated as Zn/Cu/CF-3, Zn/Cu/CF-6, and Zn/Cu/CF-12.
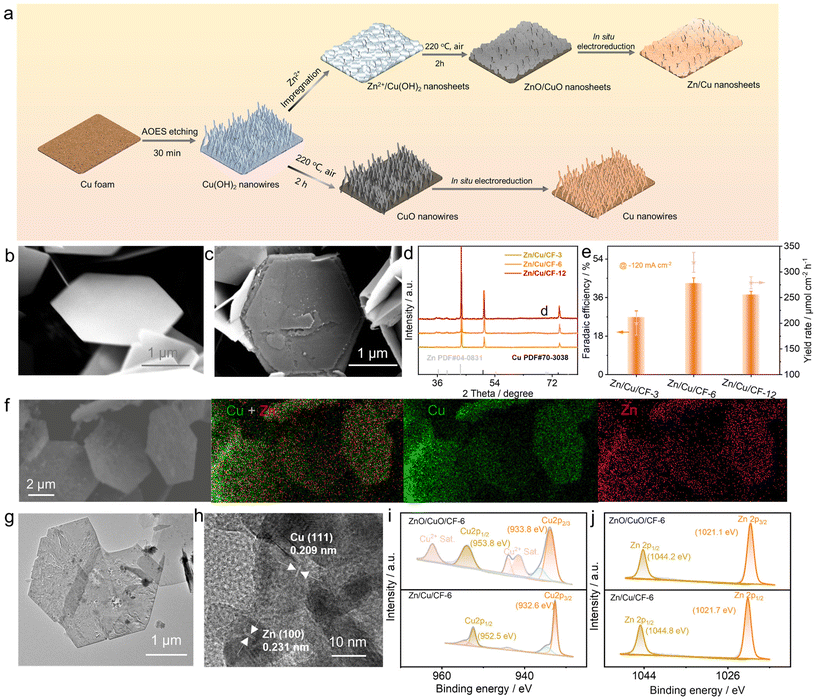 |
| Fig. 2 (a) Schematic diagram of the synthesis of CuNWs/CF and Zn/Cu/CF catalysts. SEM images of (b) ZnO/CuO/CF-6 and (c) Zn/Cu/CF-6. (d) XRD patterns of different Zn/Cu/CF electrocatalysts. (e) Performance of acetoxime electrosynthesis using different Zn/Cu/CF catalysts. (f) SEM and the corresponding EDS images of Zn/Cu/CF-6. (g) TEM and (h) HRTEM images of Zn/Cu/CF-6. (i) Cu 2p and (j) Zn2p XPS results of ZnO/CuO/CF-6 and Zn/Cu/CF-6. | |
Then, the electrochemical performance of Zn/Cu/CF catalysts impregnated for different times was investigated under a constant current of −120 mA cm−2. Zn/Cu/CF-6 exhibited the optimal acetoxime electrosynthesis performance, with a faradaic efficiency of 42.69% (Fig. 2e). Furthermore, the results of performance characterization studies at different current densities, linear scanning voltammetry (LSV), cyclic voltammetry (CV), and electrochemical impedance spectroscopy (EIS) all indicated that Zn/Cu/CF-6 exhibited significant electrochemical advantages, which will be discussed subsequently in this article. Consequently, more infinite characterization studies of Zn/Cu/CF-6 were conducted. SEM and the corresponding energy dispersive X-ray spectroscopy (EDS) revealed an ultrathin nanosheet morphology of the catalyst, with Zn and Cu elements distributed uniformly on the surface (Fig. 2f g and S16†). The TEM images also demonstrated the nanosheet structure of the catalyst. The corresponding EDS showed that the Zn content accounted for 19.5% (Fig. S17†), which was considerably higher than the inductively coupled plasma (ICP) result of 2.7% (Table S1†), further showing Zn was primarily located on the catalyst surface and uniformly distributed with Cu to form nanosheets. High-resolution transmission electron microscopy (HRTEM) revealed the presence of Cu (111) and Zn (100) lattice planes, confirming that Zn and Cu were distributed uniformly in their metallic state (Fig. 2h and S18†). Furthermore, X-ray photoelectron spectroscopy (XPS) was employed to analyze ZnO/CuO/CF-6 and Zn/Cu/CF-6, and compared with ZnO/CuO/CF-6, the binding energy of Cu2p shifted negatively by 1.2 eV and the characteristic satellite peak of Cu2+ disappeared (Fig. 2i), while a 0.6 eV positive shift appeared for the Zn2p binding energy (Fig. 2j) which indicated that Zn and Cu mainly existed in the metallic state. However, a small number of high-valence peaks of Cu2p still appeared after electroreduction, possibly due to the limited oxidation of Zn/Cu/CF-6 exposed to air. Additionally, the Cu LMM spectra showed that the kinetic energy was found to have a positive shift of 0.5 eV after electroreduction, which also demonstrated that Cu predominantly existed as the metal phase (Fig. S19†). In addition, control samples were prepared under identical synthesis conditions for the calcination of Cu(OH)2NWs/CF to acquire CuO NWs/CF (Fig. S20 and S21†) and in situ electrochemical treatment to obtain CuNWs/CF (Fig. S22–24†).
Target product identification
Subsequently, Zn/Cu/CF-6, Hg/HgO, and Pt mesh were employed as working, reference and counter electrodes, respectively. The electrolyte environment consisted of a solution of 0.5 M potassium hydroxide, 0.2 M acetone, and 0.2 M nitrate ions. Electrolysis was performed for two hours at a current density of −150 mA cm−2 and the resulting solution was analyzed via1H NMR (Fig. 3a), gas chromatography (GC) (Fig. 3b), and 13C nuclear magnetic resonance (13C NMR) (Fig. 3c) to ascertain the successful synthesis of acetoxime. Furthermore, when 15N-labeled NO3− was used as the nitrogen source, gas chromatography–mass spectrometry (GC–MS) results revealed the presence of 15N-labelled acetoxime (m/z = 74.05), thereby indicating that the nitrogen in acetoxime originated from NO3− (Fig. S25†).
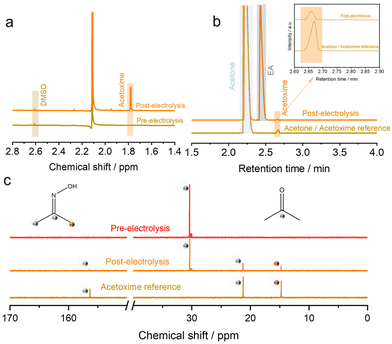 |
| Fig. 3 (a) 1H NMR results before and after electrolysis of acetone and NO3−. (b) GC diagram of the electrolyte after electrolysis and the standard acetoxime sample. (c) 13C NMR spectra before and after electrolysis and the standard sample. | |
Performance optimization and electrochemical characterization
To optimize the performance of acetoxime from acetone and nitrate, the electrolyte pH and the concentration of NO3− were investigated. The electrolyte was initially switched from an alkaline system (0.5 M KOH) to a neutral (0.25 M K2SO4) or acidic system (0.1 M H2SO4), and it was observed that the alkaline system was the most conducive to the formation of acetoxime with an FE(acetoxime) of 19.7%, followed by the neutral system of 11.0%. However, only isopropanol was detected as the liquid carbon-containing product under acidic conditions which may be attributed to the protonation of the key intermediate NH2OH to NH3OH+, thereby diminishing its nucleophilic attack capability.56 Moreover, the oxime cannot remain stable under acidic conditions and will decompose into ketone and NH2OH spontaneously.51 Additionally, a higher adsorption of protons *H on the catalyst surface under acidic conditions facilitates the hydrogenation reduction of *CH3COCH3, resulting in the detection of only isopropanol (Fig. 4a). Subsequently, with the acetone concentration held constant at 0.2 M, the NO3− concentration was gradually increased from 0 to 500 mM. It was found that the FE of acetoxime increased as the NO3− concentration increased, while the performance of isopropanol was significantly inhibited. However, until reaching a NO3− concentration of 0.2 M, further increments showed minimal change (from FEacetoxime = 19.7% at 0.2 M to FEacetoxime = 21.2% at 0.5 M) (Fig. 4b). Thus, a NO3− concentration of 0.2 M was adopted for subsequent tests.
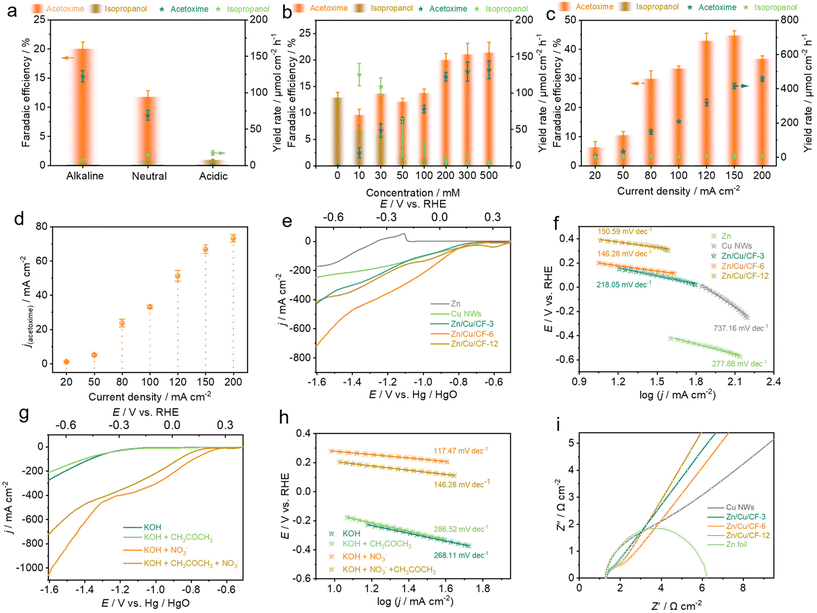 |
| Fig. 4 (a) Performance of carbon-containing liquids under different pH electrolyte conditions. (b) Performance of carbon-containing liquids with different NO3− concentrations. (c) The current density-dependent faradaic efficiency and the yield rate of products under the optimized conditions (0.5 M KOH + 0.2 M CH3COCH3 + 0.2 M NO3−) over the Zn/Cu/CF-6 catalyst. (d) The partial current density of acetoxime (j(acetoxime)) under different applied current densities. (e) LSV curves and the corresponding (f) Tafel slopes for different catalysts. (g) LSV and the corresponding (h) Tafel slopes for different electrolytes using Zn/Cu/CF-6. (i) Nyquist plots of different catalysts. | |
Performance tests were conducted over different catalysts: CuNWs/CF, Zn/Cu/CF-3, Zn/Cu/CF-6, Zn/Cu/CF-12, and Zn, under different current densities (−20, −50, −80, −120, −150, −200 mA cm−2). Zn/Cu/CF-6 exhibited the optimal performance, achieving a faradaic efficiency of 44.5% with a productivity of 415.5 μmol cm−2 h−1 at −150 mA cm−2, corresponding to a partial current density of −66.8 mA cm−2 for acetoxime (Fig. 4c and d), outperforming the results of most of the reported articles in aspects of FE and current density (Table S2†), while CuNWs/CF exhibited the least favourable performance, with an optimal FE of 24.8% and a corresponding yield rate of 123.4 μmol cm−2 h−1 at an applied current density of −80 mA cm−2, and the incorporation of Zn resulted in a notable enhancement in the electrosynthesis performance of acetoxime (Fig. S26†).
As shown in Fig. 4e, LSV tests were conducted under optimal conditions (0.5 M KOH + 0.2 M CH3COCH3 + 0.2 M NO3−) over different catalysts. Compared to CuNWs/CF and Zn, Zn/Cu nanosheets significantly improved the current density. At −100 mA cm−2, the corresponding potentials were −0.52 V vs. RHE (Zn), −0.11 V vs. RHE (CuNWs/CF), −0.10 V vs. RHE (Zn/Cu/CF-3), 0.04 V vs. RHE (Zn/Cu/CF-6), and −0.01 V vs. RHE (Zn/Cu/CF-12), respectively, with Tafel slopes of 277.88 mV dec−1 (Zn), 737.16 mV dec−1 (CuNWs/CF), 218.05 mV dec−1 (Zn/Cu/CF-3), 146.28 mV dec−1 (Zn/Cu/CF-6), and 150.59 mV dec−1 (Zn/Cu/CF-12) (Fig. 4f). Zn/Cu/CF-6 exhibited the highest current density and the lowest Tafel slope, implying the fastest electron transfer rate, which is likely more favorable for promoting the formation of acetoxime, consistent with the performance test results mentioned above. Similarly, LSV tests were conducted using Zn/Cu/CF-6 as the working electrode under different electrolyte conditions (Fig. 4g). In the absence of acetone and NO3− in the electrolyte, the HER occurred with a current density of −100 mA cm−2 and a corresponding potential of −0.46 V vs. RHE. The introduction of nitrate resulted in a decrease in the potential by 580 mV, indicating that Zn/Cu/CF-6 is more conducive to the electrochemical nitrate reduction reaction (e-NO3RR) kinetically. However, when sole acetone was added, the potential shifted negatively by 40 mV compared to the HER at −100 mA cm−2, showing that the absorbed *CH3COCH3 was less susceptible to individual acetone electroreduction (e-ARR) to isopropanol, which facilitated subsequent C
N coupling reactions. The addition of both nitrate and acetone into the reaction system resulted in a decrease in current density compared to e-NO3RR alone which demonstrated that acetone adsorbed on the catalyst surface may have occupied some of the active sites for e-NO3RR, thereby reducing its reaction rate. Despite a decrease in current density after acetone addition, it remained significantly higher than individual ARR and HER, suggesting that coupling reactions between acetone and nitrate still occurred. The Tafel slope under different electrolyte conditions indicates the kinetic order to be e-NO3RR > HER > e-ARR (Fig. 4h), further confirming that NO3− is more easily electro-reduced to the key nitrogen-containing intermediate, while acetone adsorbed on the catalyst surface hinders further hydrogenation to the by-product isopropanol, thus improving the electrosynthesis performance of acetoxime.
In addition, electrochemical impedance spectroscopy (EIS) of different catalysts was carried out to analyze the kinetics during the co-reduction process (Fig. 4i). The results showed that Zn/Cu/CF exhibited a smaller semicircular radius compared to CuNWs/CF and Zn electrodes, also indicating that the introduction of Zn significantly reduced the electron transfer impedance and increased the electron transfer rate. Furthermore, cyclic voltammetry (CV) tests were carried out in the non-faradaic region at different scan rates using different catalysts. The results showed that Zn/Cu nanosheets significantly increased the double layer capacitance (Cdl) and the electrochemically active surface area compared to CuNWs/CF and Zn. Specifically, Zn/Cu/CF-6 exhibited the highest electrochemically active surface area, approximately 9.5 times higher than CuNWs/CF and 4.8 times higher than Zn, signifying that more active sites existed on the catalyst surface, thereby increasing the probability of C
N coupling to form acetoxime (Fig. S27 and Table S3†).
Nitrogen/carbon-containing liquid by-products analysis
Understanding the by-products of the C
N coupling reaction is beneficial for assuming a plausible reaction mechanism and guiding the subsequent catalyst design. Therefore, we first analyzed the nitrogen-containing liquid by-products by carrying out UV-vis absorption spectroscopy colorimetric tests for different concentrations of NH4+, NO2−, and NH2OH using sodium salicylate, p-amino benzenesulfonamide, and 8-quinolinol method,57 respectively (Fig. 5a–c). Then the absorbance values at specific wavelengths (NH4+∼650 nm, NO2−∼540 nm, and NH2OH∼705 nm) and the concentrations were linearly fitted for qualification (Fig. S28†). Results showed that NH4+ was the main by-product at different current densities over various catalysts, and the faradaic efficiency of NO2− by-products was about 10% (Fig. 5d and e and S29†). Notably, the presence of NH2OH was barely discernible after the reaction (Fig. S30†), which may be attributed to two factors, one is that NH2OH is unstable in strongly alkaline systems, undergoing spontaneous decomposition into N2 and NH3,58 and the other is that NH2OH may be predominantly present on the catalyst surface in an adsorbed state, a hypothesis that will be discussed subsequently. In addition, the FE of isopropanol was less than 1% in the C-containing liquid by-products and the presence of isopropylamine was not detected in all the tests, indicating that acetoxime would hardly undergo further hydrogenation reduction. The ratios for the performance of acetone oxime as well as the main nitrogenous by-products NH3 and NO2− were then compared between Zn/Cu/CF-6 and CuNWs (Fig. 5f). It was found that the introduction of Zn with a hexagonal nanosheet structure was able to achieve acetoxime electrosynthesis with remarkable selectivity and inhibit the generation of NH3 from the e-NO3RR, which was conducive to the reduction of subsequent purification and isolation costs.
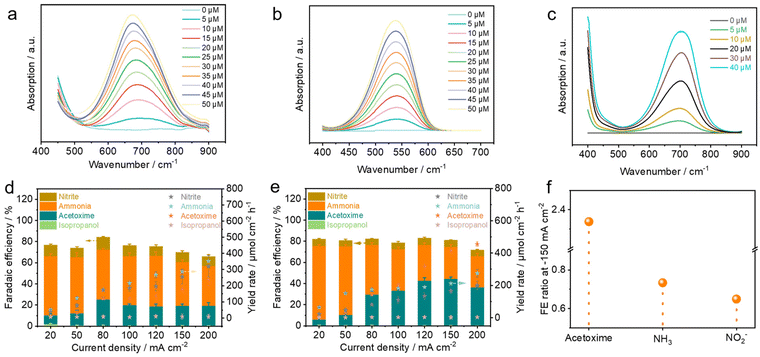 |
| Fig. 5 UV-vis absorption spectroscopy of different concentrations of (a) NH3, (b) NO2−, and (c) NH2OH. The current density-dependent faradaic efficiency and the yield rate of carbon/nitrogen-containing liquid products using (d) CuNWs/CF and (e) Zn/Cu/CF-6. (f) Performance ratios of acetoxime, NH3, and NO2− of Zn/Cu/CF-6 relative to CuNWs/CF at a current density of −150 mA cm−2. | |
Stability test
To assess the stability of the electrocatalyst, Zn/Cu/CF-6 was employed as the working electrode to conduct 10 continuous cyclic stability tests under the conditions of 0.2 M CH3COCH3 + 0.2 M KNO3, where each cyclic test lasted for 2 hours. As shown in Fig. 6a, the potential–time curve did not exhibit significant changes after 10 cycles. The 1H NMR spectra of the liquid-phase carbon-containing products after each reaction cycle are presented in Fig. 6b. The faradaic efficiency and yield rate of acetoxime showed no noticeable variation (Fig. 6c). Subsequent to stability tests, a series of characterization studies were performed. ICP results indicated that the Zn content was 2.4 wt% (Fig. 6d), which was consistent with the pre-reaction content, suggesting that Zn remained stable after 10 cyclic tests. SEM, TEM, HRTEM, and EDS images of the catalyst showed no obvious changes in the morphology and both Zn and Cu were uniformly distributed on the catalyst surface (Fig. 6e and S31, 32†). The XRD results demonstrated that the catalyst structure remained unchanged (Fig. 6f), and post-reaction XPS analysis showed that Zn and Cu also persisted mainly in the metallic form on the catalyst surface (Fig. 6g and h). All the aforementioned results indicated that the structure and morphology of the catalyst remained almost unchanged before and after the stability tests (Fig. S33†), demonstrating excellent durability of Zn/Cu/CF-6 for the electrosynthesis of acetoxime from acetone and nitrate.
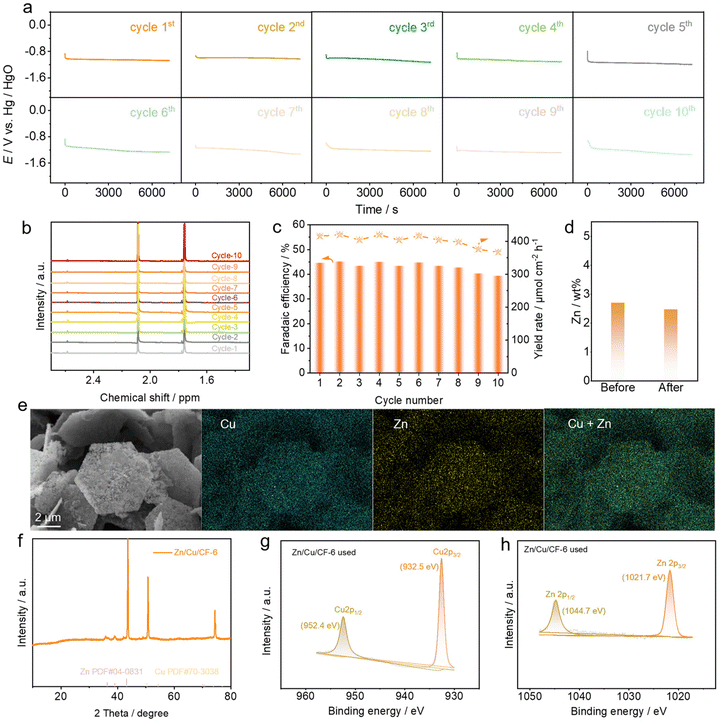 |
| Fig. 6 (a) The potential–time curves of Zn/Cu/CF-6 from 1st to 10th cyclic stability test. (b) 1H NMR spectra of ten cyclic tests. (c) The faradaic efficiency and the yield rate of acetoxime. (d) ICP results of Zn content of Zn/Cu/CF-6 before and after the stability tests. (e) SEM and the corresponding EDS results of Zn/Cu/CF-6 after the stability test. (f) XRD pattern of Zn/Cu/CF-6 after the stability test. Cu2p (g) and Zn2p (h) XPS results of Zn/Cu/CF-6 after the stability test. | |
Mechanistic analysis
To elucidate the mechanistic pathways involved in the electrosynthesis of acetoxime from acetone and nitrate, a series of control experiments were conducted to identify the key intermediates of both the carbon and nitrogen feedstocks. As shown in Table S4 and Fig. S34,† experiments were performed using 0.5M KOH as the electrolyte at −150 mA cm−2. Initially, with acetone as the C source and NO3− as the N source, subsequent replacement of NO3− with NO2−, N2, NH2OH, and NH3 (entries 1–5 in Table S4†) revealed that no acetoxime was produced when NH3 was used as the N source, suggesting NH2OH was the crucial nitrogen-containing intermediate. However, acetoxime was undetectable when N2 was employed as the nitrogen reactant, likely due to its low solubility in the electrolyte and the high dissociation energy of the N
N bond (941 kJ mol−1), making its activation challenging. Upon the introduction of hydroxylamine (NH2OH) as the N source, a substantial amount of acetoxime was generated. However, the nucleophilic addition–elimination reaction between NH2OH and acetone does not involve electron transfer, and thus, the yield rate was utilized to represent the performance of acetoxime (entry 4 in Table S4†). Further experiments with NO3− as the N source and isopropanol as the C source showed no acetoxime production, suggesting that *CH3COCH3 could be a key carbon intermediate (entry 6 in Table S4†). The cessation of the current application also resulted in no acetoxime production, showing acetoxime was formed driven by electricity (entry 7 in Table S4†). In order to ascertain whether C
N coupling occurs at the electrode surface or in the electrolyte due to the desorption of key intermediates, we conducted an examination to evaluate the presence of NH2OH. It is worth noting that NH2OH was almost undetectable in the post-reaction solution (Fig. S35†) and NH2OH was unstable under alkaline conditions which decomposed into N2 and NH3 spontaneously,51 suggesting that C
N coupling occurred between the adsorbed *NH2OH and *CH3COCH3 on the electrode surface, rather than *NH2OH desorbing into the solution to couple with acetone. To elucidate the cumulative effect of Zn introduction on *CH3COCH3, electrochemical studies were conducted using Zn/Cu/CF-6, CuNWs/CF, and Zn as working electrodes to evaluate the individual activity of e-ARR (Fig. S36†). The results demonstrated that the incorporation of Zn inhibited the formation of isopropanol. Concurrently, considering the results from Fig. 4c and Fig. S26a,† it can be inferred that, compared to CuNWs/CF, the introduction of Zn was detrimental to the hydrogenation of *CH3COCH3, and thus, it is more prone to undergo a coupling reaction with surface-generated *NH2OH, thereby promoting the formation of acetoxime.
Moreover, the Zn/Cu/CF-6 catalyst was employed as the working electrode for in situ ATR-IRAS and DEMS investigations to monitor the intermediates of e-NO3RR and e-NARR. When only e-NO3RR occurred, the peaks of *NH2OH, *NO2−, and *NH4+ were detected at 1112 cm−1,59,60 1229 cm−1,61 and 1458 cm−1,25,62,63 respectively (Fig. 7a), with 1880 cm−1 and 2670 cm−1 corresponding to the CaF2 substrate.64 Upon the introduction of CH3COCH3 into the system, the *NH2OH signal significantly weakened (Fig. S37 and S38†), accompanied by the appearance of signals located at 1683 cm−1 of the C
N bond,40,53,65 1027 cm−1 for the N–O stretching of C
N–OH,41 and 1227 cm−1 attributed to the C–C–C asymmetric stretch peak of adsorbed *CH3COCH366,67 (Fig. 7b). To further verify the production of *NH2OH with *CH3COCH3, quasi in situ electron paramagnetic resonance (EPR) trapping experiments of hydrogen and carbon radicals were conducted over Zn/Cu/CF-6 (Fig. S39†). During the e-NO3RR test, only H radicals were detected, which were attributed to the protonation of NO3−. With the addition of acetone, significant C radicals were observed, confirming the adsorption and activation of *CH3COCH3 on the electrode surface, which provides additional evidence for the production of *NH2OH with *CH3COCH3. The results of the DEMS data indicated that when only NO3− was present in the solution, a significant signal rise/fall of m/z = 17 and m/z = 33 (corresponding to NH3 and NH2OH, respectively) was observed with the application/withdrawal of the current (Fig. 7c). Upon the introduction of acetone to the reaction system, the signals of m/z = 33 and m/z = 17 were significantly weakened (Fig. 7d), suggesting that acetone was able to capture the in situ formed *NH2OH, which generated the target product oxime and inhibited the formation of the by-product NH4+. Furthermore, CuNWs/CF was also tested for e-NO3RR and e-NARR DEMS. The NH2OH intensity was found to be weaker than that of Zn/Cu/CF-6 when only e-NO3RR occurred (Fig. S40a†). This indicated that the introduction of Zn was conducive to the generation of NH2OH. As for the introduction of acetone, the intensity of m/z = 33 also exhibited a significant attenuation (Fig. S40b†), meaning further reduction of *NH2OH was diminished. The in situ ATR-IRAS and DEMS findings collectively suggested that Zn/Cu/CF-6 facilitated *NH2OH generation and *CH3COCH3 accumulation. It can be concluded that the hydrogenation of *NH2OH and *CH3COCH3 is less facile than the spontaneous C
N coupling reaction, enabling acetoxime electrosynthesis with high faradaic efficiency at large current densities. Based on the aforementioned conclusions and existing literature reports, we propose a plausible reaction pathway for the synthesis of acetoxime, as illustrated in Fig. 7e: NO3− → *NO2 → *NO → *HNO → *HNHO → *NH2OH, followed by C
N coupling between *NH2OH and *CH3COCH3 to yield acetoxime.
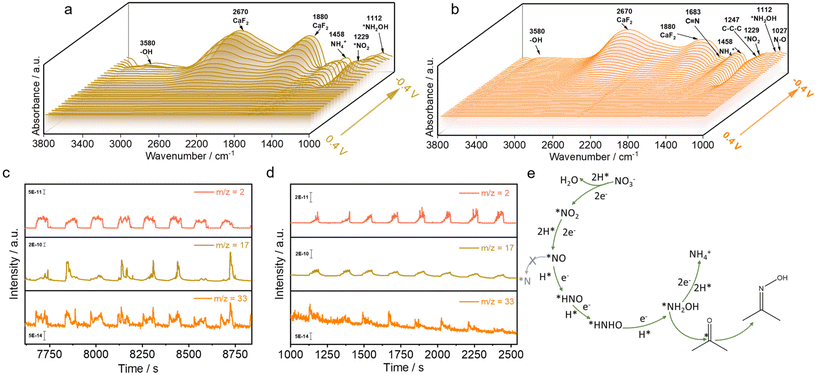 |
| Fig. 7
In situ ATR-IRAS spectra of Zn/Cu/CF-6 for (a) e-NO3RR and (b) e-NARR. DEMS results of Zn/Cu/CF-6 for (c) e-NO3RR and (d) e-NARR. (e) Proposed plausible reaction pathways of acetoxime from nitrate and acetone. | |
Conclusions
In summary, this work reports a method for the synthesis of hexagonal Zn/Cu/CF nanosheets through wet-impregnation, calcination, and in situ electroreduction. Through optimization of the electrolyte pH, precursor concentration, and current densities, a faradaic efficiency of 44.5% for acetoxime production was achieved at a near-industrial current density of −150 mA cm−2, corresponding to a yield rate of 415.5 μmol cm−2 h−1 with a C selectivity approaching 100%. A series of electrochemical characterization studies revealed that the introduction of Zn leads to a faster electron transfer and a larger ECSA with more active sites and promotes the generation of *NH2OH while inhibiting further hydrogenation of *CH3COCH3, thereby improving the performance of acetoxime electrosynthesis. Results of a series of control experiments, ATR-IRAS and DEMS indicate that the C
N bond arises from the coupling of key intermediates *NH2OH and *CH3COCH3, and a reasonable pathway, NO3− → *NO2 → *NO → *HNO → *HNHO → *NH2OH → CH3C=NOHCH3, was proposed. This work not only provides a facile method for the synthesis of hexagonal Zn/Cu ultrathin nanosheets, but also elucidates the mechanism by which Zn/Cu synergistically promotes the electrosynthesis of acetoxime. Furthermore, a green transformation of the inorganic pollutant NO3− and biomass-derived acetone into a high-value organonitrogen chemical is achieved, which expands the research scope of electrocatalytic C–N coupling reactions and offers a new pathway for the one-step green synthesis of acetoxime from inorganic nitrogen sources.
Data availability
The datasets supporting this article have been uploaded as part of the ESI† (QI-RES-05-2024-001234).
Author contributions
Ya-Wen Zhang conceived the idea and directed the project. Jiang Shao designed the experiments and carried out the materials synthesis and characterization. Zi-Yuan Li and Xin-Li helped in the TEM data collection, Bin Liang contributed to the SEM image capturing, and Sheng-Zhi Xue contributed to the drawing of the schematic diagram and result discussion. Ting-Zhou Li assisted in the XPS test. Yi-Fei Zhang helped in the electrochemical tests and mechanism analysis. Jiang Shao wrote the paper. Ya-Wen Zhang and Hao Dong revised the manuscript. All authors discussed the results and commented on the manuscript.
Conflicts of interest
The authors declare no competing interests.
Acknowledgements
This work was supported by the National Key R&D Program of China (No. 2023YFA1506800 and 2021YFA1501100), the National Natural Science Foundation of China (No. 22293042), and the Beijing National Laboratory for Molecular Sciences (BNLMSCXXM-202104). We greatly acknowledge Prof. Jie Zheng from Peking University for the help on the in situ ATR-IRAS and DEMS test and sincerely thank Dr Shuai Ma for his assistance with GC-MS.
References
- N. B. Johnson, I. C. Lennon, P. H. Moran and J. A. Ramsden, Industrial-scale synthesis and applications of asymmetric hydrogenation catalysts, Acc. Chem. Res., 2007, 40, 1291–1299 CrossRef CAS PubMed.
- P. Debnath, Recent advances in the synthesis of amides via oxime rearrangements and its applications, Curr. Org. Synth., 2018, 15, 666–706 CrossRef CAS.
-
U. Romano and M. Ricci, Industrial applications, Liquid Phase Oxidation via Heterogeneous Catalysis: Organic Synthesis and Industrial Applications, John Wiley & Sons, Hoboken, 2013 Search PubMed.
- P. Su, H.-L. Guo, L. Tian and S.-K. Ning, An efficient method of producing stable graphene suspensions with less toxicity using dimethyl ketoxime, Carbon, 2012, 50, 5351–5358 CrossRef CAS.
- C. K. Chua and M. Pumera, Chemical reduction of graphene oxide: a synthetic chemistry viewpoint, Chem. Soc. Rev., 2014, 43, 291–312 RSC.
-
E.-S. M. Sherif, Chapter 20-The role of corrosion inhibitors in protecting metallic structures against corrosion in harsh environments, The role of colloidal systems in environmental protection, 2014, pp. 509–526 Search PubMed.
- Z.-Z. Yang, L.-N. He, J. Gao, A.-H. Liu and B. Yu, Carbon dioxide utilization with C–N bond formation: carbon dioxide capture and subsequent conversion, Energy Environ. Sci., 2012, 5, 6602–6639 RSC.
- P. Liao, J. Kang, R. Xiang, S. Wang and G. Li, Electrocatalytic systems for NOx valorization in organonitrogen synthesis, Angew. Chem., 2024, 136, e202311752 CrossRef.
- P. Beaupre and W. Holland, Pyridine ketoximes as analytical reagents. XV: The spectrophotometric determination of ultra-trace amounts of cobalt in natural waters with 2-pyridyl-2-thienyl-β-ketoxime, Microchim. Acta, 1978, 70, 95–104 CrossRef.
- D. Reiner and D. P. Poe, Removal of iron, copper, cadmium, cobalt, and nickel from sodium hydroxide by precipitation and extraction with phenyl-2-pyridyl ketoxime, Anal. Chem., 1977, 49, 889–891 CrossRef CAS.
- H. Yu, F. Shang, Q. Chu, P. Wang, M. Wang, H. Zhu, F. Song, H. Yang and T. Diao, Cleaner and atomic economy production of hydroxylamine hydrochloride under solvent-free conditions through process intensification, J. Cleaner Prod., 2020, 269, 122187 CrossRef CAS.
- G. R. Tauszik and P. Crocetta, Production of hydroxylamine from nitrogen oxide: A short review, Appl. Catal., 1985, 17, 1–21 CrossRef CAS.
- L. O. Cisneros, W. J. Rogers and M. S. Mannan, Comparison of the thermal decomposition behavior for members of the hydroxylamine family, Thermochim. Acta, 2004, 414, 177–183 CrossRef CAS.
- H.-Y. He, H. Niikura, Y.-L. Du and K. S. Ryan, Synthetic and biosynthetic routes to nitrogen–nitrogen bonds, Chem. Soc. Rev., 2022, 51, 2991–3046 RSC.
- R. T. Driessen, P. Kamphuis, L. Mathijssen, R. Zhang, L. G. van der Ham, H. van den Berg and A. J. Zeeuw, Industrial process design for the production of aniline by direct amination, Chem. Eng. Technol., 2017, 40, 838–846 CrossRef CAS.
- A. Soler-Jofra, J. Pérez and M. C. Van Loosdrecht, Hydroxylamine and the nitrogen cycle: a review, Water Res., 2021, 190, 116723 CrossRef CAS PubMed.
- F. Guan, Y. Chen, Y. Zhang and R. Yu, A coupling process of electrodialysis with oxime hydrolysis reaction for preparation of hydroxylamine sulfate, RSC Adv., 2021, 11, 19238–19247 RSC.
- R. Adams and O. Kamm, ORGANIC CHEMICAL REAGENTS. I. DIMETHYLGLYOXIME, J. Am. Chem. Soc., 1918, 40, 1281–1289 CrossRef CAS.
- X. Pan, Synthesis of acetone oxime and solid hydroxylamine, Technol. Dev. Chem. Ind., 2006, 35, 1–3 CAS.
- X. Liang, Z. Mi, Y. Wang, L. Wang and X. Zhang, Synthesis of acetone oxime through acetone ammoximation over TS-1, React. Kinet. Catal. Lett., 2004, 82, 333–337 CrossRef CAS.
- J. Ding, L. Xu, Y. Yu, H. Wu, S. Huang, Y. Yang, J. Wu and P. Wu, Clean synthesis of acetaldehyde oxime through ammoximation on titanosilicate catalysts, Catal. Sci. Technol., 2013, 3, 2587–2595 RSC.
- Z. Li, R. Chen, W. Jin and W. Xing, Catalytic mechanism and reaction pathway of acetone ammoximation to acetone oxime over TS-1, Korean J. Chem. Eng., 2010, 27, 1423–1427 CrossRef CAS.
- A. Thangaraj, S. Sivasanker and P. Ratnasamy, Catalytic properties of crystalline titanium silicalites III. Ammoximation of cyclohexanone, J. Catal., 1991, 131, 394–400 CrossRef CAS.
- J. Ding and P. Wu, Selective synthesis of dimethyl ketone oxime through ammoximation over Ti-MOR catalyst, Appl. Catal., A, 2014, 488, 86–95 CrossRef CAS.
- K. Yang, S.-H. Han, C. Cheng, C. Guo, T. Li and Y. Yu, Unveiling the Reaction Mechanism of Nitrate Reduction to Ammonia Over Cobalt-Based Electrocatalysts, J. Am. Chem. Soc., 2024, 146, 12976–12983 CrossRef CAS.
- T.-N. Ye, S.-W. Park, Y. Lu, J. Li, M. Sasase, M. Kitano, T. Tada and H. Hosono, Vacancy-enabled N2 activation for ammonia synthesis on an Ni-loaded catalyst, Nature, 2020, 583, 391–395 CrossRef CAS PubMed.
- Y. Wang, C. Wang, M. Li, Y. Yu and B. Zhang, Nitrate electroreduction: mechanism insight, in situ characterization, performance evaluation, and challenges, Chem. Soc. Rev., 2021, 50, 6720–6733 RSC.
-
S. Singh, Energy crisis and climate change: Global concerns and their solutions, Energy: Crises, Challenges and Solutions, 2021, pp. 1–17 Search PubMed.
- J. L. Holechek, H. M. Geli, M. N. Sawalhah and R. Valdez, A global assessment: can renewable energy replace fossil fuels by 2050?, Sustainability, 2022, 14, 4792 CrossRef.
- X. Xiao, L. Yang, W. Sun, Y. Chen, H. Yu, K. Li, B. Jia, L. Zhang and T. Ma, Electrocatalytic water splitting: from harsh and mild conditions to natural seawater, Small, 2022, 18, 2105830 CrossRef CAS PubMed.
- C. Choi, X. Wang, S. Kwon, J. L. Hart, C. L. Rooney, N. J. Harmon, Q. P. Sam, J. J. Cha, W. A. Goddard III and M. Elimelech, Efficient electrocatalytic valorization of chlorinated organic water pollutant to ethylene, Nat. Nanotechnol., 2023, 18, 160–167 CrossRef CAS.
- J. Liu, J. Ma, Z. Zhang, Y. Qin, Y.-J. Wang, Y. Wang, R. Tan, X. Duan, T. Z. Tian and C. H. Zhang, 2021 Roadmap: electrocatalysts for green catalytic processes, JPhys Mater., 2021, 4, 022004 CrossRef CAS.
- S. M. Jordaan and C. Wang, Electrocatalytic conversion of carbon dioxide for the Paris goals, Nat. Catal., 2021, 4, 915–920 CrossRef.
- S. Chu and A. Majumdar, Opportunities and challenges for a sustainable energy future, Nature, 2012, 488, 294–303 CrossRef CAS PubMed.
- J. E. Kim, S. Choi, M. Balamurugan, J. H. Jang and K. T. Nam, Electrochemical C–N bond formation for sustainable amine synthesis, Trends Chem., 2020, 2, 1004–1019 CrossRef CAS.
- J. Li, Y. Zhang, K. Kuruvinashetti and N. Kornienko, Construction of C–N bonds from small-molecule precursors through heterogeneous electrocatalysis, Nat. Rev. Chem., 2022, 6, 303–319 CrossRef CAS PubMed.
- Z. Tao, C. L. Rooney, Y. Liang and H. Wang, Accessing organonitrogen compounds via C–N coupling in electrocatalytic CO2 reduction, J. Am. Chem. Soc., 2021, 143, 19630–19642 CrossRef CAS.
- Y. Huang, Y. Wang, Y. Wu, Y. Yu and B. Zhang, Electrocatalytic construction of the C-N bond from the derivates of CO2 and N2, Sci. China: Chem., 2022, 65, 204–206 CrossRef CAS.
- Y. Zhong, H. Xiong, J. Low, R. Long and Y. Xiong, Recent progress in electrochemical C–N coupling reactions, eScience, 2023, 3, 100086 CrossRef.
- M. Li, Y. Wu, B.-H. Zhao, C. Cheng, J. Zhao, C. Liu and B. Zhang, Electrosynthesis of amino acids from NO and α-keto acids using two decoupled flow reactors, Nat. Catal., 2023, 6, 906–915 CrossRef CAS.
- Y. Cheng, S. Liu, J. Jiao, M. Zhou, Y. Wang, X. Xing, Z. Chen, X. Sun, Q. Zhu and Q. Qian, Highly Efficient Electrosynthesis of Glycine over an Atomically Dispersed Iron Catalyst, J. Am. Chem. Soc., 2024, 146, 10084–10092 CrossRef CAS PubMed.
- R. Xiang, S. Wang, P. Liao, F. Xie, J. Kang, S. Li, J. Xian, L. Guo and G. Li, Electrocatalytic Synthesis of Pyridine Oximes using in Situ Generated NH2OH from NO species on Nanofiber Membranes Derived from NH2-MIL-53 (Al), Angew. Chem., Int. Ed., 2023, 62, e202312239 CrossRef CAS.
- X. Chen, S. Song, H. Li, G. k. Gözaydın and N. Yan, Expanding the boundary of biorefinery: organonitrogen chemicals from biomass, Acc. Chem. Res., 2021, 54, 1711–1722 CrossRef CAS.
- M. Jouny, J.-J. Lv, T. Cheng, B. H. Ko, J.-J. Zhu, W. A. Goddard III and F. Jiao, Formation of carbon–nitrogen bonds in carbon monoxide electrolysis, Nat. Chem., 2019, 11, 846–851 CrossRef CAS PubMed.
- X. Peng, L. Zeng, D. Wang, Z. Liu, Y. Li, Z. Li, B. Yang, L. Lei, L. Dai and Y. Hou, Electrochemical C–N coupling of CO2 and nitrogenous small molecules for the electrosynthesis of organonitrogen compounds, Chem. Soc. Rev., 2023, 52, 2193–2237 RSC.
- J. Wu, L. Xu, Z. Kong, K. Gu, Y. Lu, X. Wu, Y. Zou and S. Wang, Integrated tandem electrochemica-chemical-electrochemical coupling of biomass and nitrate to sustainable alanine, Angew. Chem., 2023, 135, e202311196 CrossRef.
- J. Xian, S. Li, H. Su, P. Liao, S. Wang, R. Xiang, Y. Zhang, Q. Liu and G. Li, Electrosynthesis of α-Amino Acids from NO and other NOx species over CoFe alloy-decorated Self-standing Carbon Fiber Membranes, Angew. Chem., 2023, 135, e202306726 CrossRef.
- J. Xian, S. Li, H. Su, P. Liao, S. Wang, Y. Zhang, W. Yang, J. Yang, Y. Sun and Y. Jia, Electrocatalytic Synthesis of Essential Amino Acids from Nitric Oxide Using Atomically Dispersed Fe on N-doped Carbon, Angew. Chem., Int. Ed., 2023, 62, e202304007 CrossRef CAS PubMed.
- Y. Wu, J. Zhao, C. Wang, T. Li, B.-H. Zhao, Z. Song, C. Liu and B. Zhang, Electrosynthesis of a nylon-6 precursor from cyclohexanone and nitrite under ambient conditions, Nat. Commun., 2023, 14, 3057 CrossRef CAS PubMed.
- Y. Wu, W. Chen, Y. Jiang, Y. Xu, B. Zhou, L. Xu, C. Xie, M. Yang, M. Qiu and D. Wang, Electrocatalytic synthesis of nylon–6 precursor at almost 100% yield, Angew. Chem., Int. Ed., 2023, 62, e202305491 CrossRef CAS.
- W. Chen, Y. Wu, Y. Jiang, G. Yang, Y. Li, L. Xu, M. Yang, B. Wu, Y. Pan and Y. Xu, Catalyst Selection over an Electrochemical Reductive Coupling Reaction toward Direct Electrosynthesis of Oxime from NOx and Aldehyde, J. Am. Chem. Soc., 2024, 146, 6294–6306 CrossRef CAS.
- X. Lan, C. Cheng, C. Guo, M. Guo, T. Li, Y. Wu, Y. Yu and B. Zhang, Electrosynthesis of hydroxylamine from nitrate reduction in water, Sci. China: Chem., 2023, 66, 1758–1762 CrossRef CAS.
- S. Jia, L. Wu, X. Tan, J. Feng, X. Ma, L. Zhang, X. Song, L. Xu, Q. Zhu and X. Kang, Synthesis of Hydroxylamine via Ketone-Mediated Nitrate Electroreduction, J. Am. Chem. Soc., 2024, 146, 10934–10942 CrossRef CAS PubMed.
- J. Fu, Y. Yang and J.-S. Hu, Dual-sites tandem catalysts for C–N bond formation via electrocatalytic coupling of CO2 and nitrogenous small molecules, ACS Mater. Lett., 2021, 3, 1468–1476 CrossRef CAS.
- F.-Y. Chen, Z.-Y. Wu, S. Gupta, D. J. Rivera, S. V. Lambeets, S. Pecaut, J. Y. T. Kim, P. Zhu, Y. Z. Finfrock and D. M. Meira, Efficient conversion of low-concentration nitrate sources into ammonia on a Ru-dispersed Cu nanowire electrocatalyst, Nat. Nanotechnol., 2022, 17, 759–767 CrossRef CAS.
- X. Zhang, H. Jing, S. Chen, B. Liu, L. Yu, J. Xiao and D. Deng, Direct electro-synthesis of valuable C=N compound from NO, Chem. Catal., 2022, 2, 1807–1818 CrossRef CAS.
- T. Muthusamy, S. S. Markandaraj and S. Shanmugam, Nickel nanoparticles wrapped in N-doped carbon nanostructures for efficient electrochemical reduction of NO to NH3, J. Mater. Chem. A, 2022, 10, 6470–6474 RSC.
- G. Pio, P. Mocellin, C. Vianello and E. Salzano, A detailed kinetic model for the thermal decomposition of hydroxylamine, J. Hazard. Mater., 2021, 416, 125641 CrossRef CAS.
- Y. Xiong, Y. Wang, J. Zhou, F. Liu, F. Hao and Z. Fan, Electrochemical nitrate reduction: ammonia synthesis and the beyond, Adv. Mater., 2023, 2304021 Search PubMed.
- J.-Y. Fang, Q.-Z. Zheng, Y.-Y. Lou, K.-M. Zhao, S.-N. Hu, G. Li, O. Akdim, X.-Y. Huang and S.-G. Sun, Ampere-level current density ammonia electrochemical synthesis using CuCo nanosheets simulating nitrite reductase bifunctional nature, Nat. Commun., 2022, 13, 7899 CrossRef CAS.
- S. Han, H. Li, T. Li, F. Chen, R. Yang, Y. Yu and B. Zhang, Ultralow overpotential nitrate reduction to ammonia via a three-step relay mechanism, Nat. Catal., 2023, 6, 402–414 CrossRef CAS.
- J. Meng, C. Cheng, Y. Wang, Y. Yu and B. Zhang, Carbon Support Enhanced Mass Transfer and Metal–Support Interaction Promoted Activation for Low-Concentrated Nitric Oxide Electroreduction to Ammonia, J. Am. Chem. Soc., 2024, 146, 10044–10051 CrossRef CAS PubMed.
- Y. Yao, S. Zhu, H. Wang, H. Li and M. Shao, A spectroscopic study on the nitrogen electrochemical reduction reaction on gold and platinum surfaces, J. Am. Chem. Soc., 2018, 140, 1496–1501 CrossRef CAS PubMed.
- X. Li, J. H. Wang, C. Y. Yuan, Q. W. Sun, J. Shao, X. C. Li, Z. L. Feng, H. Dong, C. Li and Y. W. Zhang, A Unique Amorphous Porous BiSbOx Nanotube with Abundant Unsaturated Sb–Stabilized BiO8−x Sites for Efficient CO2 Electroreduction in a Wide Potential Window, Adv. Funct. Mater., 2024, 2402220 CrossRef.
- S. Jia, X. Tan, L. Wu, X. Ma, L. Zhang, J. Feng, L. Xu, X. Song, Q. Zhu and X. Kang, Integration of plasma and electrocatalysis to synthesize cyclohexanone oxime under ambient conditions using air as a nitrogen source, Chem. Sci., 2023, 14, 13198–13204 RSC.
- R. L. Hudson, P. A. Gerakines and R. F. Ferrante, IR spectra and properties of solid acetone, an interstellar and cometary molecule, Spectrochim. Acta, Part A, 2018, 193, 33–39 CrossRef CAS PubMed.
- M. El-Maazawi, A. Finken, A. Nair and V. Grassian, Adsorption and photocatalytic oxidation of acetone on TiO2: an in situ transmission FT-IR study, J. Catal., 2000, 191, 138–146 CrossRef CAS.
|
This journal is © the Partner Organisations 2024 |