DOI:
10.1039/D4MH90010C
(Commentary)
Mater. Horiz., 2024,
11, 1608-1610
A reflection on ‘A shape-memory scaffold for macroscale assembly of functional nanoscale building blocks’
First published on 11th March 2024
Abstract
Controllable and precise fabrication of macroscopic assembly of nanoscale building blocks is a key step towards practical application of nanotechnology. In 2014, Yu et al. presented a novel approach to fabricate a series of functional macroscale assemblies using a shape-memory chitosan scaffold (H.-L. Gao, Y. Lu, L.-B. Mao, D. An, L. Xu, J.-T. Gu, F. Long and S.-H. Yu, Mater. Horiz., 2014, 1, 69, https://doi.org/10.1039/c3mh00040k). Here, they briefly review the development process of the assembly strategy directed by the pre-designed scaffold to further explore the potential of the strategy in constructing the artificial macroscopic assemblies with versatile properties.
Overview
In the past decades, numerous nanoscale materials featuring diverse functions have been designed and synthesized.1 To further broaden their application in materials science, the assembly of these tiny nanoparticles into macroscopic three-dimensional (3D) structures is a crucial step. However, achieving controlled assembly and macroscopic integration while preserving functionality remains a formidable challenge. In 2014, Yu et al. (https://doi.org/10.1039/c3mh00040k) reported a strategy for the macroscale integration of functional nanoscale building blocks (NSBBs) using a shape-memorable scaffold. First, they used ice templating to fabricate a three-dimensional chitosan scaffold (CSS) with oriented pore structures, which were then stabilized via chemical treatment. Thanks to their shape-memory capability, these scaffolds could be cyclically compressed in a suspension of NSBBs, facilitating their assembly onto the scaffold walls. Various macroscale assemblies with shape memory or distinct functional properties were successfully prepared. This work demonstrated the potential of cross-scale integration of macroscopic nanostructures and inspired further research on shape-memorable scaffold-directed assembly.
After its publication in the first issue of Materials Horizons in 2014, macroscale assembly with NSBBs has seen considerable advancement, in which this work had a strong impact.2 In reviewing such progress, we find that the use of scaffolds in the assembly of NSBBs has been attracting increasing interest.3 Moreover, macroscale assemblies with various functional properties have been achieved by designing4,5 or directly employing processed natural biological scaffolds.6 Researchers have constructed an array of remarkable structural materials with excellent mechanical properties, such as reboundability7 and fatigue resistance,8 as well as diverse functional properties, including solar desalination,9 adsorption,10 biomedicine,11 electromagnetic interference shielding12 and integrated functions.13 Considering the pivotal role of the scaffold-directed assembly strategy to build macroscopic structured materials, there is immense value in reflecting on the progress since the publication of this work and revisiting its significance and impact—insights crucial for advancing scaffold-directed multilevel macroscale assembly research.
Discussion
The outstanding properties of a macroscale assembly composed of functional NSBBs stem from the effective combination of the properties of assembled nanocomponents with those of the scaffold. Such functional integration leads to a synergistic effect, such that the combined assembly exhibits capabilities more significant than the sum of its parts. In the study in 2014 (https://doi.org/10.1039/c3mh00040k), the shape-memory function of the CSS was derived from its microstructure with aligned pores achieved through unidirectional freeze-casting; the inherent elasticity of the pore walls enables them to revert to their original form once the external force is removed. The oriented pore structure not only promotes mass transport but also significantly influences the assembly process of the NSBBs. The effectiveness of the scaffold-directed assembly is contingent upon matching electrostatic interactions between the building blocks and the scaffold interface, which requires a specific interfacial design to achieve an oriented and induced assembly. Given the universal applicability of this assembly strategy, it can be employed in various material systems to facilitate the preparation of macroscopic assemblies composed of diverse NSBBs.
Over the last ten years, a wave of related research efforts has been inspired by the proposed practical assembly strategy.6–9,14–17 Some have focused on further expanding the compositions and types of scaffolds to build multifunctional macroscopic assemblies (Fig. 1). For example, Yu and co-workers designed a super-elastic and fatigue-resistant carbon–graphene composite with multi-arch microstructures by applying a bidirectional freezing process of the slurry containing chitosan and graphene oxide.7 Due to the difference in thermal stability of the components during the heat treatment in nitrogen, the flat lamellas of scaffold translate into a waved multi-arch morphology. Furthermore, they incorporated magnetic particles into the carbon scaffold, which introduce magnetic response and enhance sensing capabilities of the carbon macroscopic assembly under extreme conditions.8 Lu et al. developed an anti-biofouling shape-memory CSS with unidirectional channels and tannic acid coating, which could maintain high solar-driven water evaporation efficiency even after exposure to a microbe-rich environment.9 Recently, Yu and co-workers successfully integrated a long-chain fatty acid macromolecule into the rigid one-dimensional pore walls of a resin wood-like scaffold fabricated by unidirectional freeze casting, which endows the artificial wood with super-flexibility.14
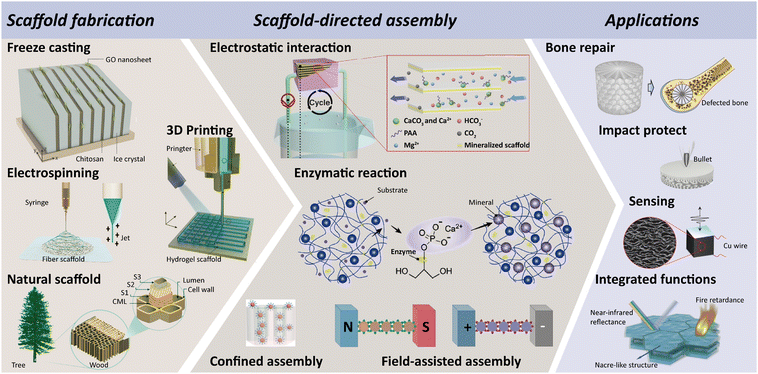 |
| Fig. 1 Typical examples of the scaffold fabricated technologies, scaffold-directed assembly strategies and future applications of artificial macroscopic assemblies.5,6,8,11,13,15,17–19 | |
On the other hand, researchers have been dedicated to optimize the preparation strategy of the scaffolds or to utilize the inherent properties of the scaffolds to affect the assembly process (Fig. 1), eventually the structures or the compositions of the macroscopic assemblies can be adjusted.15–19 In 2016, Yu and co-workers achieved the fabrication of artificial nacre in the laboratory for the first time using an assembly-and-mineralization approach.15 Layered CSS was constructed by introducing a bidirectional temperature gradient during the freezing process. Subsequently, the CSS were further acetylated to promote the mineralized growth of aragonite. Remarkably, this synthetic nacre exhibits a structure and properties highly analogous to natural nacre. Moreover, they successfully introduced the incorporation of Fe3O4 nanoparticles within the macroscopic assemblies through the fine regulation of the scaffold-directed mineralization process.16 The nanoscale incorporation can engender the residual stresses in the mineral layer, which have great enhancement of the fracture toughness of the nacre-mimetic structural materials. Recently, Liu et al. integrated a 3D printed enzyme-incorporated hydrogel scaffold with biomineralization to develop functional materials.17 More sophisticated and multicomponent scaffolds can endow macroscopic assemblies with a wider array of properties. These works have significantly expanded the understanding and practical applications of macroscopic assemblies (Fig. 1).
Outlook
Although numerous efforts have been made to design scaffold structures and control scaffold-directed assembly processes, there remains an urgent need for innovative approaches to further enhance the properties of artificial macroscopic assemblies. Fortunately, numerous natural biomineral macroscopic assemblies with remarkable properties, such as nacre,20 cuttlebone,21 and the hinge ligament of bivalves,22 provide a natural paradigm for the further development of artificial composites. Advanced observation techniques have revealed that the complexity and precision in the natural control of structure and composition in these biominerals far exceed those of any artificial macroscopic assemblies.20 The assembly and growth processes of building blocks in biological systems are precisely regulated by constructing a microenvironment generated from the pre-designed scaffold, which facilitate the formation of dense composite materials, as opposed to a mere adsorption assembly or crystallization growth process.23 In addition, the step-by-step biological assembly strategy achieves the optimization of the mass transfer process. While the fabrication of artificial assemblies still has many shortages that need to be addressed, more and more new technologies are being developed to optimize the scaffold structures and the precise control of scaffold composition, which will provide a promising outlook for precise and efficient fabrication of future macroscale assemblies.23–26 It is anticipated that the versatile macroscopic assemblies directed by pre-designed scaffolds will find greater utility across a broader range of fields like bioengineering, sensing and protection.
Author contributions
X. S. M., L. B. M. and S. H. Y. wrote the manuscript.
Conflicts of interest
There are no conflicts to declare.
Acknowledgements
The authors acknowledge the support from the National Natural Science Foundation of China (Grants 22293044).
Notes and references
- S.-H. Yu, R. O’Reilly, L. Jiang and N. A. Kotov, Acc. Chem. Res., 2022, 55, 1783–1784 CrossRef CAS PubMed.
- J. Yin, Y. Huang, S. Hameed, R. Zhou, L. Xie and Y. Ying, Nanoscale, 2020, 12, 17571–17589 RSC.
- J. S. Kahn and O. Gang, Angew. Chem., Int. Ed., 2022, 61, e202105678 CrossRef CAS PubMed.
- M. Li, X. Dai, W. Gao and H. Bai, Acc. Mater. Res., 2022, 3, 1173–1185 CrossRef CAS.
- N. A. Norzain and W. C. Lin, J. Ind. Text., 2022, 51, 6728S–6752S CrossRef CAS.
- C. Montanari, P. Olsén and L. A. Berglund, Front. Chem., 2021, 9, 682883 CrossRef CAS PubMed.
- H. L. Gao, Y. B. Zhu, L. B. Mao, F. C. Wang, X. S. Luo, Y. Y. Liu, Y. Lu, Z. Pan, J. Ge, W. Shen, Y. R. Zheng, L. Xu, L. J. Wang, W. H. Xu, H. A. Wu and S. H. Yu, Nat. Commun., 2016, 7, 12920 CrossRef CAS PubMed.
- H.-L. Gao, Z.-Y. Wang, C. Cui, J.-Z. Bao, Y.-B. Zhu, J. Xia, S.-M. Wen, H.-A. Wu and S.-H. Yu, Adv. Mater., 2021, 33, 2102724 CrossRef CAS PubMed.
- H. Xu, H. Xing, S. Chen, Q. Wang, L. Dong, K.-D. Hu, B. Wang, J. Xue and Y. Lu, Nanoscale, 2022, 14, 7493–7501 RSC.
- A. A. Chavan, H. Li, A. Scarpellini, S. Marras, L. Manna, A. Athanassiou and D. Fragouli, ACS Appl. Mater. Interfaces, 2015, 7, 14778–14784 CrossRef CAS PubMed.
- S.-J. Jiang, M.-H. Wang, Z.-Y. Wang, H.-L. Gao, S.-M. Chen, Y.-H. Cong, L. Yang, S.-M. Wen, D.-D. Cheng, J.-C. He and S.-H. Yu, Adv. Funct. Mater., 2022, 32, 2110931 CrossRef CAS.
- Z. Fan, D. Wang, Y. Yuan, Y. Wang, Z. Cheng, Y. Liu and Z. Xie, Chem. Eng. J., 2020, 381, 122696 CrossRef CAS.
- Q.-F. Guan, H.-B. Yang, C.-H. Yin, Z.-M. Han, K.-P. Yang, Z.-C. Ling and S.-H. Yu, ACS Mater. Lett., 2021, 3, 243–248 CrossRef CAS.
- Y.-C. Gao, Z.-L. Yu, B. Qin, C. Chen, Z.-Y. Ma and S.-H. Yu, Adv. Mater., 2023, 35, 2303518 CrossRef CAS PubMed.
- L.-B. Mao, H.-L. Gao, H.-B. Yao, L. Liu, H. Cölfen, G. Liu, S.-M. Chen, S.-K. Li, Y.-X. Yan, Y.-Y. Liu and S.-H. Yu, Science, 2016, 354, 107–110 CrossRef CAS PubMed.
- Y.-F. Meng, Y.-B. Zhu, L.-C. Zhou, X.-S. Meng, Y.-L. Yang, R. Zhao, J. Xia, B. Yang, Y.-J. Lu, H.-A. Wu, L.-B. Mao and S.-H. Yu, Adv. Mater., 2022, 34, 2108267 CrossRef CAS PubMed.
- G. Chen, X. Liang, P. Zhang, S. Lin, C. Cai, Z. Yu and J. Liu, Adv. Funct. Mater., 2022, 32, 2113262 CrossRef CAS.
- S.-M. Wen, S.-M. Chen, W. Gao, Z. Zheng, J.-Z. Bao, C. Cui, S. Liu, H.-L. Gao and S.-H. Yu, Adv. Mater., 2023, 35, 2211175 CrossRef CAS PubMed.
- R. Liang, J. Xu, R. Deng, K. Wang, S. Liu, J. Li and J. Zhu, ACS Macro Lett., 2014, 3, 486–490 CrossRef CAS PubMed.
- W. Huang, D. Restrepo, J.-Y. Jung, F. Y. Su, Z. Liu, R. O. Ritchie, J. McKittrick, P. Zavattieri and D. Kisailus, Adv. Mater., 2019, 31, 1901561 CrossRef CAS PubMed.
- E. Lee, Z. Jia, T. Yang and L. Li, Acta Biomater., 2022, 154, 312–323 CrossRef PubMed.
- X.-S. Meng, L.-C. Zhou, L. Liu, Y.-B. Zhu, Y.-F. Meng, D.-C. Zheng, B. Yang, Q.-Z. Rao, L.-B. Mao, H.-A. Wu and S.-H. Yu, Science, 2023, 380, 1252–1257 CrossRef CAS PubMed.
- L.-B. Mao, Y.-F. Meng, X.-S. Meng, B. Yang, Y.-L. Yang, Y.-J. Lu, Z.-Y. Yang, L.-M. Shang and S.-H. Yu, J. Am. Chem. Soc., 2022, 144, 18175–18194 CrossRef CAS PubMed.
- H. Zhao, Y. Yue, L. Guo, J. Wu, Y. Zhang, X. Li, S. Mao and X. Han, Adv. Mater., 2016, 28, 5099–5105 CrossRef CAS PubMed.
- M. T. I. Mredha and I. Jeon, Prog. Mater. Sci., 2022, 124, 100870 CrossRef CAS.
- T. Xiang, J. Hou, H. Xie, X. Liu, T. Gong and S. Zhou, Nano Today, 2020, 35, 100980 CrossRef CAS.
|
This journal is © The Royal Society of Chemistry 2024 |
Click here to see how this site uses Cookies. View our privacy policy here.