DOI:
10.1039/D3MH01292A
(Review Article)
Mater. Horiz., 2024,
11, 102-112
The emergence and prospects of carbon dots with solid-state photoluminescence for light-emitting diodes
Received
15th August 2023
, Accepted 26th September 2023
First published on 27th September 2023
Abstract
The significant features of carbon dots (CDs), such as bright and tunable photoluminescence, high thermal stability, and low toxicity, endow them with tremendous potential for application in next generation optoelectronics. Despite great progress achieved in the design of high-performance CDs so far, the practical applications in solid-state lighting and displays have been retarded by the aggregation-caused quenching (ACQ) effect ascribed to direct π–π interactions. This review provides a comprehensive overview of the recent progress made in solid-state CD emitters, including their synthesis, optical properties and applications in light-emitting diodes (LEDs). Their triplet-excited-state-involved properties, as well as their recent advances in phosphor-converted LEDs and electroluminescent LEDs, are mainly reviewed here. Finally, the prospects and challenges of solid-state CD-based LEDs are discussed with an eye on future development. We hope that this review will provide critical insights to inspire new exciting discoveries on solid-state CDs from both fundamental and practical standpoints so that the realization of their potential in optoelectronic areas can be facilitated.
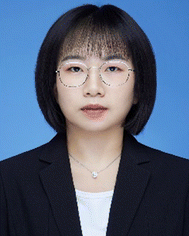
Ting Yuan
| Ting Yuan received her PhD degree in physical chemistry from Beijing Normal University (BNU) in 2022. She is currently a postdoctoral researcher with research interests focused on the synthesis and optoelectronic applications of luminescent carbon nanomaterials. |
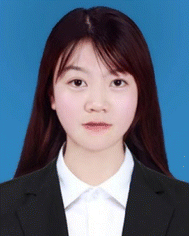
Qian Teng
| Qian Teng is currently a graduate student majoring in physical chemistry at BNU. Her research interest is focused on the design and synthesis of organic/inorganic functional materials for light-emitting diodes. |
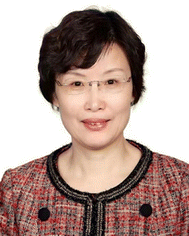
Louzhen Fan
| Louzhen Fan is now a chemistry professor in the College of Chemistry, BNU. She received her PhD in physical chemistry in 1998 under the supervision of Prof. Daoben Zhu and Prof. Yongfang Li from the Institute of Chemistry, Chinese Academy of Sciences, Beijing. Her research interests are focused on the synthesis of novel carbon and metal nanomaterials and their applications in biology and energy. |
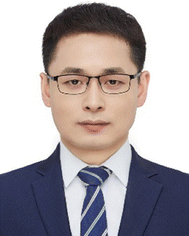
Fanglong Yuan
| Fanglong Yuan obtained his PhD degree at BNU in 2018, and carried out his postdoctoral research at Linköping University, and the University of Toronto. Now he is a full professor at the College of Chemistry, BNU. His research interest is focused on organic/inorganic functional materials chemistry, light-emitting materials, optical and photophysical properties, and optoelectronic devices. |
Wider impact
This paper primarily introduces the latest significant development in solid-state CDs (SSCDs), including synthesis, classification such as fluorescent SSCDs, phosphorescent SSCDs, delay fluorescent SSCDs, and their wide application prospect in the field of light-emitting diodes (LEDs). At present, due to resonance energy transfer and direct π–π interactions between CDs in the aggregate state, SSCDs usually exhibit severe fluorescence self-quenching, which severely hinders their practical applications in solid-state lighting and displays. Accordingly, SSCDs have received extensive attention recently. We predict SSCDs will accelerate the realization of their potential in optoelectronic areas. This review provides a comprehensive overview of the recent progress made in solid-state CD emitters, including their synthesis, optical properties, and applications in LEDs. Their triplet-excited-state-involved properties, as well as their recent advances in phosphor-converted LEDs and electroluminescent LEDs, are mainly reviewed here. Finally, the prospects and challenges of solid-state CD-based LEDs are discussed with an eye on future development.
|
1. Introduction
Carbon dots (CDs) are an emerging subclass of photoluminescent (PL) carbon nanomaterials, characterized by an atomically thin graphitic plane (typically 1 or 2 layers, <2 nm thick) with lateral dimensions typically <10 nm.1–3 Fluorescent carbon was discovered in 2004, during the electrophoretic purification of arc-synthesized single-walled carbon nanotubes.4 The nanoscale carbon particles were first named “carbon dots” in 2006, and CDs with bright and colorful PL were obtained by laser ablation of graphite powder.5 Generally, the tunable PL of CDs ranging from deep ultraviolet to near-infrared (NIR) can be tuned by their size, shape, surface functional groups and heteroatom doping.6–8 More importantly, CDs are mainly composed of abundant carbon elements in nature, with almost no toxicity, environmental friendliness, and low cost.3,9,10 These intriguing properties open up a new possibility for next-generation lighting and displays compared to fluorescent dyes and semiconductor quantum dots (QDs).11,12 Immense efforts have been made to develop CD-based light-emitting diodes (LEDs) with a high photoluminescence quantum yield (PLQY) from deep-blue to NIR in the last few years.13,14 In particular, the characteristic broadband emission and narrow bandwidth can be used for white LEDs (WLEDs) and high color-purity multicolored LEDs, respectively, which opens up great prospects for CDs in next-generation lighting and displays.15,16
Despite the remarkable progress made in designing high-performance LEDs, it is noteworthy that, thus far, nearly all the reported CDs have only demonstrated efficient light emission in solution.17,18 Due to the resonance energy transfer and direct π–π interactions between CDs in the aggregate state, solid-state CDs (SSCDs) usually exhibit severe fluorescence self-quenching.19–21 As a result, for the fabrication of the emissive layer, a common approach is to utilize a suitable host matrix such as a polymer to mitigate the energy loss caused by concentration quenching. Typically, the host materials dominate (up to 99%) the host/guest emitters. Therefore, the selection of the host material is an additional concern.22–24 Precise control of the host to guest ratio plays a major role, and is the foremost challenge. Moreover, the host–guest blend system can experience phase separation and crystallization at high driving voltages, which can adversely affect the overall device performance.17,25,26 In order to circumvent these demerits, undoubtedly, it is highly desirable to develop SSCD-based LEDs consisting of non-doped emissive layers. Specifically, employing single SSCDs as the emissive layer not only simplifies the device fabrication process but also maintains a homogeneous surface film morphology during device operation. This characteristic enhances the device stability and contributes to making LEDs more commercially viable.27,28
Most SSCDs are achieved by embedding CDs into various matrices, including inorganic salts,29,30 polymers,21,31–33 and silica gel.26,34 However, this strategy using CD composites to achieve solid-state PL is more vulnerable to phase segregation, poor thermal stability and conductivity, which severely impedes their practical applications. Therefore, single-component CDs with intrinsic solid-state photoluminescence properties are highly desired. Extensive research has been conducted and documented on SSCDs,35,36 which demonstrates a compelling solution as non-doped emitters for the development of highly efficient LED devices.
There have been already a number of excellent reviews focusing on different aspects of photoluminescent CDs, such as their synthesis, luminescence mechanism, optical property regulation and optoelectronic applications.13,37–41 However, only a very limited number of review articles have focused on SSCDs and their related applications. In this review, with the aim of promoting better SSCD engineering and their further development, we primarily update the latest significant developments on SSCDs, including synthesis, classification such as fluorescent SSCDs, phosphorescent SSCDs, delay fluorescent SSCDs, and their recent advances in LEDs, as illustrated in Fig. 1. In particular, the current challenges and future prospects of SSCDs in lighting and displays are highlighted. Finally, we hope that this review will further stimulate the development of high-performance SSCD-based LEDs with a combined effort from different disciplines.
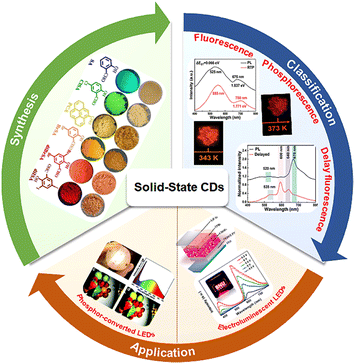 |
| Fig. 1 Illustration of the overview of solid-state photoluminescent CDs (main topics of this review). Reprinted with permission from ref. 51,71,73,82. Copyright 2023 John Wiley and Sons. Copyright 2019 the Royal Society of Chemistry. Copyright 2021 American Chemical Society. | |
2. Synthesis
Synthetic approaches for CDs are generally classified into two categories–‘‘top-down’’ and ‘‘bottom-up’’. Generally, the CDs prepared by the former are prone to π–π stacking due to the lack of functional groups. We here mainly focus on the bottom-up methods that are realized by the fusion of organic molecules under thermal42/solvothermal11,43,44 conditions to synthesize SSCDs. The precursors chosen often determine the optical properties of the resulting CDs such as the PLQY,45,46 solubility,47 charge transport mobility,48 structural characteristics49 including size, shape, and crystallinity. This method offers relatively high yields and the convenience of introducing multiple heteroatom doping by readily inheriting from organic precursors during synthesis. For instance, by choosing p-phenyldiacetonitrile (with –CN groups) and terephthalaldehyde (with –CHO groups) as precursors, the gram-scale synthesis of bright red/green/blue solid-state bandgap fluorescent carbon quantum rings (R/G/B-SBF-CQRs) with QYs up to 30–46% was reported (Fig. 2(a)–(c)).50 By employing surface salicylaldehyde-type ligand modulation, SSCDs were successfully engineered to exhibit a wide emission-color range of nearly 300 nm, spanning from blue to deep red (Fig. 2(d) and (e)).51,52 However, at present, most SSCDs show poor solubility in organic solvents, which greatly limits their application in electroluminescent LEDs. Recently, orange and yellow solid-state emissive CDs were prepared through the solvothermal treatment of 4,4-diformyltriphenylamine, terephthalonitrile and acetonitrile in an alkaline solution of ethanol, which shows high PLQYs of 25% and 20%, respectively (Fig. 2(f) and (g)).48
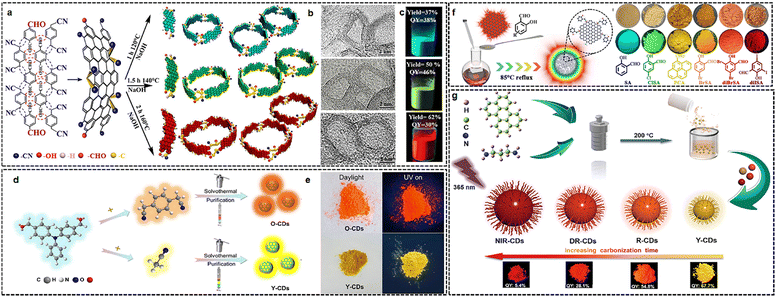 |
| Fig. 2 (a)–(c) Schematic illustration of the synthesis of R/G/B-SBF-CQRs. Reprinted with permission from ref. 50. Copyright 2021 John Wiley and Sons; (d) and (e) synthesis of polychromatic SSF CDs. Reprinted with permission from ref. 48. Copyright 2023 John Wiley and Sons; (f) schematic illustration of the fabrication process of CDs with tunable color. Reprinted with permission from ref. 51. Copyright 2023 John Wiley and Sons; (g) synthesis and characterization of O-CDs and Y-CDs. Reprinted with permission from ref. 52. Copyright 2023 John Wiley and Sons. | |
3. Classification of solid-state photoluminescent CDs
Solid-state photoluminescent CDs exhibit not only traditional fluorescence but also room-temperature phosphorescence (RTP) and thermally activated delayed fluorescence (TADF). These different luminescence properties rely on the various spin states involved in the radiative relaxation process.6,14,53 Fluorescence is the phenomenon of light emission that occurs within nanoseconds when an electron transitions from a singlet excited state (S1) to a ground state (S0) of the same spin multiplicity. On the other hand, phosphorescence involves the emission of light between states of different spin multiplicity, specifically from a triplet excited state (T1) to the ground state (S0), and it typically lasts for microseconds to seconds. In addition, when T1 and S1 are close in energy, the singlet–triplet energy splitting (ΔEST) is small, and the endothermic RISC process can be overcome by the thermal motions of the molecule atoms. As a result, nonradiative triplet excitons, due to spin-forbidden T1 → S0 transition, are transformed into singlet excitons via RISC, leading to TADF emission (S1 → S0) with lifetimes in the range of hundreds of nanoseconds to dozens of milliseconds (Fig. 3). In general, the external quantum efficiency (EQE) is one of the most essential parameters for evaluating the performance of electroluminescent LEDs, which is defined as the ratio of the number of emitted photons outside the device to the number of charges injected into the device. However, only the radiative transition process of singlet excitons from S1 to S0 can be utilized for emitting light, i.e., fluorescence, which is quantum mechanically allowed. Therefore, in organic fluorescent materials, the electrically generated 75% triplet excitons are emitted as heat rather than light. Even if the PLQY of conventional fluorescent molecules can reach nearly 100%, in theory, the maximum EQE (EQEmax) value may only reach roughly 5% due to the light out-coupling efficiency of ∼20% in the electroluminescent device. In contrast, phosphorescent and TADF emitters are capable of harvesting light from both triplet and singlet excitons, allowing a great breakthrough of the exciton statistical limit in fluorescent counterparts from 25% to 100%, which is critical for enhanced efficiency.
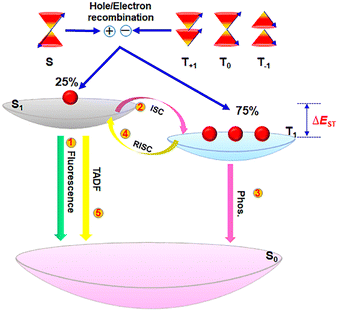 |
| Fig. 3 Electroluminescence processes in phosphorescent and TADF materials. The transition process of electrically generated excitons for fluorescence (①), phosphorescence (②–③) and TADF (④–⑤). ①: fluorescence, ②: intersystem crossing (ISC), ③: phosphorescence, and ④: reversible intersystem crossing (RISC). | |
3.1 Fluorescent solid-state CDs
So far, incorporation of CDs into solid-state matrices such as boron oxynitride (BNO),54 agar,55 or poly(vinyl alcohol) (PVA)27 has been considered as a main solution to minimize aggregation-caused PL quenching by forming intermolecular interactions such as hydrogen bonding. Considering that problems like phase separation caused by the matrix can be detrimental to LEDs, we would like to emphasize the importance of using CDs that possess inherent solid-state fluorescence properties, eliminating the need for additional composite matrices.
The initial example of CDs, synthesized through one-pot hydrothermal treatment of poly(vinyl alcohol) (PVA) and ethylenediamine, showcases robust yellow-green solid-state fluorescence when in an aggregated state.36 Afterwards, continuous efforts have been channelled towards designing efficient blue, green, and yellow fluorescent SSCDs.35,56–60 In general, SSCDs can be designed systematically through major strategies based on hydrogen bonds, electrostatic interaction, and specific functional groups, aiming at increasing the distance between adjacent CDs to depress the unfavourable ACQ. For instance, through surface ligand modulation, fluorescent SSCDs from blue to deep red were obtained by deliberately altering the conjugation ability between substituent ligands and CD cores. The structure–property studies reveal that intra- and inter-molecular hydrogen bonding inside CDs provokes the emission properties in the aggregated state (Fig. 4(a)–(c)).51 In addition, multicolor photoluminescence can be achieved by the self-assembly of solution-state fluorescent CDs modified with chlorosalicylaldehyde ligands (Fig. 4(d) and (e)).61
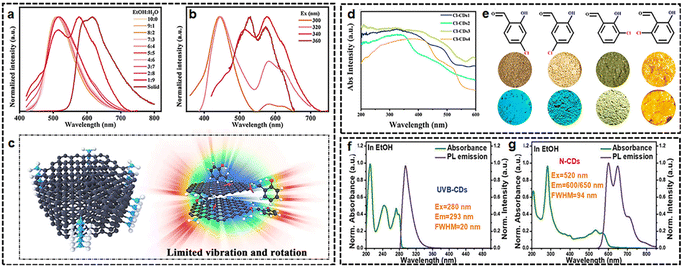 |
| Fig. 4 (a)–(c) Optical properties of diBrSA-CDs. Reprinted with permission from ref. 51. Copyright 2023 John Wiley and Sons; (d), (e) solid-state photoluminescence properties of Cl-CDs1–4. Reprinted with permission from ref. 61. Copyright 2023 American Chemical Society; (f), (g) UV-vis absorption and PL spectra of UVB-CDs, N-CDs in EtOH solution and UV-vis absorption and PL spectra of UVB-CD powder. Reprinted with permission from ref. 64. Copyright 2023 John Wiley and Sons. | |
However, it remains a great challenge to obtain CDs with ultraviolet (UV, 200–400 nm) emission in the solid state.62,63 By employing acetic acid to promote fractions of sp3 bonding to decrease the size of sp2 conjugating units in the CDs, solid-state UVB emissive CDs (λmax = 308 nm/λex = 265 nm) with a high PLQY of 20.20% and narrow full width at half maximum (FWHM) of 24 nm has been developed (Fig. 4(f) and (g)).64
3.2 Phosphorescent solid-state CDs
Benefiting from the abundant energy levels, the emerging quantum-confined CDs have demonstrated tremendous potential in the realms of RTP and TADF for next-generation displays.65 In the last few years, steady efforts have been made in developing phosphorescent and delay fluorescent CDs through host–guest complexation strategies to prevent unnecessary triplet energy dissipation, in which isolated guest CDs are homogeneously dispersed in hosts such as the crystalline frameworks,39 SiO2, boric acid66 and inorganic crystalline nanocomposites.67 However, the strategy using CDs composite to achieve triplet-excited-state-involved is more vulnerable to phase segregation, poor thermal stability and conductivity, which severely impedes their practical applications, and thus, undoubtedly, single-component CDs with intrinsic RTP and TADF properties are highly desired.68 For example, blue-green self-protective RTP emission based on fluorine and nitrogen co-doped CDs, have been demonstrated, indicating the intrinsic ability of ISC in CDs.69 Before long, we report the first successful demonstration of single-component white emission derived from blue-yellow fluorescence–phosphorescence dual emission with an overall PLQY of 25% and a relatively high yellow phosphorescence with a ΦP of 6% under ambient conditions based on the white emissive carbon nitride QDs (W-CNQDs) (Fig. 5(a)–(e)).70 Experimental and theoretical investigations reveal that the key role of the carbonyl groups at the rim of W-CNQDs has been uncovered in assisting the ISC arising from the carbonyl (nπ*) mediated intermolecular (ππ*) electronic coupling. Moreover, by adding long chains to the periphery, red phosphorescent CDs with emission peaks at 620 nm with a QY of up to 42.3% were synthesized (Fig. 5(f)–(i)).71 In addition, some efforts have been made to explore CD-based multicolor phosphorescence. For instance, a universal approach has been devised, utilizing efficient radiative energy transfer to enable multicolor phosphorescent CDs that can be excited in the NIR region.72
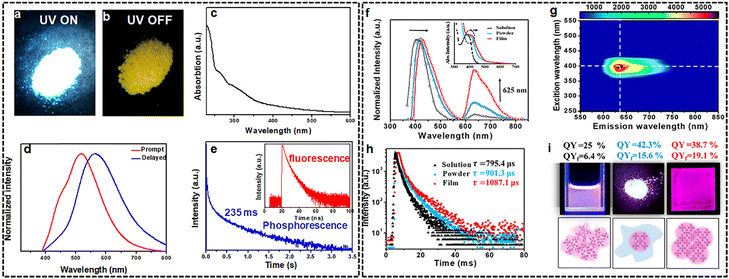 |
| Fig. 5 (a)–(e) optical properties of W-CNQDs. Reprinted with permission from ref. 70. Copyright 2019 the Royal Society of Chemistry; (f)–(h) photophysical properties of the CDOFs. Reprinted with permission from ref. 71. Copyright 2021 American Chemical Society. | |
3.3 Delay fluorescent solid-state CDs
Compared to RTP emitters, researches about the design of TADF counterpart are relatively rare. The main strategy is based on a rigid matrix host–guest system by embedding CDs into various matrices, including zeolites,29 SiO2/NaOH,73,74 and potassium aluminum sulfate.39 TADF CDs have been designed by incorporating them into urea, (3-aminopropyl) triethoxysilane, and sodium hydroxide (NaOH) through the introduction of ionic bonds. The as-prepared CDs displayed broadband delayed emission spanning from blue to deep red with two emission peaks at ≈600 and 640 nm (Fig. 6(a)–(d)).73 Indeed, CDs with intrinsic TADF properties without using matrices are of great significance to developing CD-based optoelectronics. In general, a small ΔEST plays a crucial role as the primary driving force for RISC, which can be achieved through electron-donating and electron–accepting interactions, either intramolecularly or intermolecularly with facilitated charge transfer. These interactions effectively minimize the overlap between the HOMO and the LUMO of the molecules involved. For instance, a new design approach is reported to control ΔEST in graphene QDs/graphene oxide QDs by varying the ratio of oxygenated carbon to sp2 carbon (γOC) (Fig. 6(e) and (f)).75 It is demonstrated that ΔEST decreases from 0.365 to 0.123 eV as γOC increases from 4.63% to 59.6%, which in turn induces a dramatic transition from RTP to TADF. Experimental results elucidate that such behavior with the changing afterglow characteristics is attributed in part to the distorted molecular geometry and to the change in the spin–orbit coupling matrix element value by increasing oxygen functional groups, opening a new approach to engineering the ΔEST in GQD for the controlled realization of smart multimodal afterglow materials.
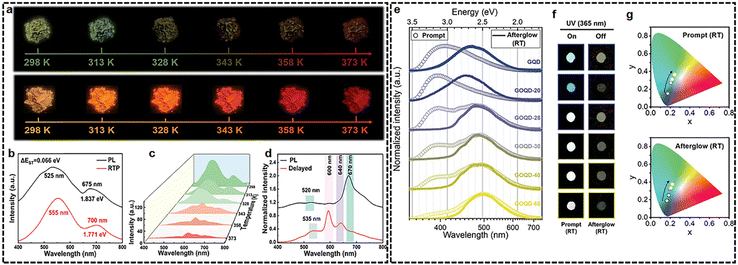 |
| Fig. 6 (a)–(d) Photophysical properties of CDs@SiO2 and CDs@SiO2/NaOH. Reprinted with permission from ref. 73. Copyright 2023 John Wiley and Sons; (e) and (f) emergence on tunable afterglow for the series of GQDs depending on the oxidation degree. Reprinted with permission from ref. 75. Copyright 2020 John Wiley and Sons. | |
Although CDs with RTP or TADF properties are capable of harvesting triplet excitons for the realization of high-performance electroluminescence. However, CD-based triplet-state emissions, especially the TADF CDs, are rare and generally require the matrix assistance due to a large ΔEST value, which severely impedes their practical applications. Accordingly, from a fundamental perspective, incorporating heteroatoms (N, O, S, P, B and so on) into the π skeletons can facilitate the effective RISC. Furthermore, the CD-based TADF with long wavelength emission (orange or red) as well as narrow-band (FWHM < 50 nm) emission remains underexplored. Additionally, more efforts should be devoted to advanced and high-resolution characterization techniques as well as to computational simulations, which may increase our understanding of structure–property relationships, and the fundamental TADF mechanisms.
4. Application of solid-state CDs in LEDs
LEDs are the enabling elements of the rapidly growing solid-state lighting and full-color display industry.58,76 The emerging SSCDs appear to be promising alternatives to organic molecules and toxic metal-based semiconductor QDs due to their tunable stable fluorescence emission, solution processability, low cost and environment-friendliness.14,77,78 In particular, SSCDs can overcome phase segregation, color aging, poor stability, and the complicated device fabrication procedure, making them competitive for the fabrication of large-area displays and lighting devices against their ACQ counterparts.20 Generally, the applications of SSCDs in LEDs can be divided into two categories, i.e., phosphor-converted LEDs and electroluminescent LEDs.
4.1 Phosphor-converted LEDs
As a direct benefit of such scarce solid luminescence from carbon nanomaterials, the first example of W-LEDs with color coordinates of (0.28, 0.27) was achieved by using the low-temperature-synthesized and toxic ion-free inter-crossed carbon nanorings as solid phosphors on blue LED chips.33 Subsequently, great efforts have been made to prepare efficient blue, green, and yellow emissive solid-state CD-based phosphors for application in WLEDs.79 However, efficient long-wavelength emissive CDs, especially the deep-red and NIR regions (>640 nm), are very scarce due to the lack of rational solutions. Moreover, the PLQYs of SSCDs are usually less than 30%, except for highly efficient blue/green CDs, which dramatically hinder their practical applications. By employing highly efficient solid-state fluorescent CDs (from the Vis to NIR region) as phosphors, a plant growth LED lamp combined with deep-red and NIR CDs, and a blue-LED chip is fabricated (Fig. 7(a)–(d)).52 The PL spectrum and the absorption bands of plant photosynthetic pigments matched well in blue and red regions. Compared with sunlight and white LEDs, the plants irradiated by a growth LED lamp show better growth in terms of leaves and rhizome length under the same experimental conditions.
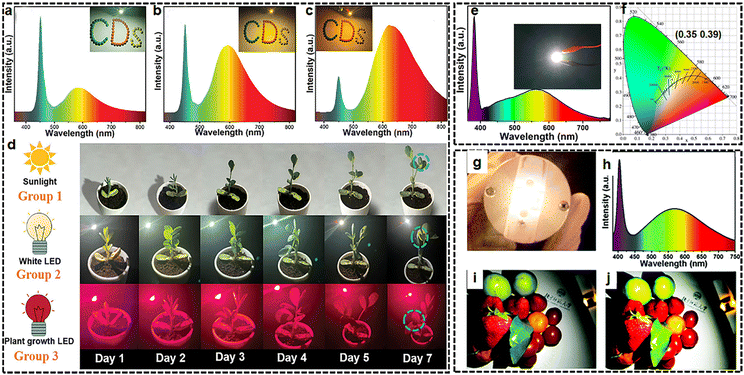 |
| Fig. 7 (a)–(d) The plant growth LED device based on a blue-LED chip and deep-red/NIR-emitted CDs. Reprinted with permission from ref. 52. Copyright 2022 John Wiley and Sons; (e), (f) fluorescence–phosphorescence dual emissive carbon nitride quantum dots efficient single-component WLEDs. Reprinted with permission from ref. 70. Copyright 2019 the Royal Society of Chemistry; (g)–(j) the application of solid ultra-BWCQDs for warm WLEDs. Reprinted with permission from ref. 82. Copyright 2019 the Royal Society of Chemistry. | |
Nowadays, combining yellow SSCD phosphors with a blue diode chip is still the most established method for fabricating single-component WLEDs.80 However, due to the lack of a sufficient red component, these cold WLEDs (correlated color temperature, CCT > 5000 K) exhibit an inferior color rendering index (CRI) and the excessive blue light from the chips would do harm to the human retina. This, therefore, severely impedes their practical application in indoor lighting. By general consensus, realizing high quality warm WLEDs (CRI > 75 and CCT < 4500 K) urgently requires the development of ultrabroad-band emitting single-component phosphors that cover the entire visible spectral window from 400 to 700 nm with a sufficient red component.81 By using blue-yellow fluorescence–phosphorescence dual emissive W-CNQD, a WLED is fabricated by integrating W-CNQD phosphors into a UV-LED chip, which shows favorable white light characteristics with color coordinates (CIEs) and a CRI of (0.35, 0.39) and 85, respectively (Fig. 7(e) and (f)).70 In addition, our groups coated solid ultra-BWCDs on the surface of a UV-LED chip (400 nm) to produce warm WLEDs. The WLEDs exhibited a CRI value of 91.30. Compared with the commercial WLED lamp (CRI ≈ 85), the warm WLED lamp is more capable of showing the true colors of the fruits (Fig. 7(g)–(j)).82
4.2 Electroluminescent LEDs
Electroluminescent LEDs, converting electricity directly into light, possess potentially higher efficiency. Thus, it is of great significance to develop potential display and lighting applications. Due to the absence of phase separation and the ability to maintain a uniform surface film morphology throughout device operation, SSCDs have demonstrated significant potential in non-doped electroluminescent LEDs that comprise a solitary molecule emitter within the emissive layer. Considering the complexity of electroluminescent devices, multiple functional layers are often required to function simultaneously. Limited research has been conducted and reported on the SSCD-based electroluminescent LEDs.28,83,84 Taking advantage of the nonplanar structure and good carrier mobility of triphenylamine unit, by employing the as-prepared CDs as a single emissive layer, high-performance orange-color and green-color electroluminescent LEDs have been successfully fabricated, with a maximum brightness of 9450/4236 cd m−2, a high current efficiency of 1.57/2.34 cd A−1 and a low turn-on voltage of 3.1/3.6 eV (Fig. 8(a)–(d)).48 In order to utilize triplet states for breaking through the 5% EQE limitation of the traditional fluorescence device, the LEDs based on the red phosphorescent (625 nm) CDOFs exhibited a red emission with a maximum luminance of 1818 cd m−2 and an EQE of 5.6% (Fig. 8(e)–(j)).71 This work explores the possibility of a new perspective for developing high-performance solid-state CD-based electroluminescent LEDs. In addition, benefiting from the good solution processability and film forming ability, and a high electron/hole mobility of 3.59 × 10−4/7.12 × 10−4 cm2 V−1 s−1, high-performance red solid-state fluorescent CD-based electroluminescent LEDs (2967 cd m−2 and 1.38 cd A−1) were fabricated via systematic optimization of device engineering.85
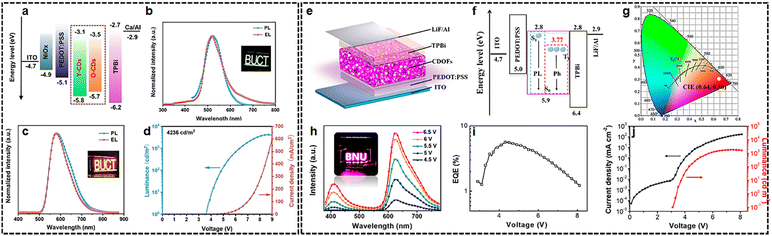 |
| Fig. 8 (a)–(d) Preparation and device performance of O-LEDs and G-LEDs. Reprinted with permission from ref. 48. Copyright 2023 John Wiley and Sons; (e)–(j) LED structure, energy diagram, and performance characterization of red phosphorescent CDs. Reprinted with permission from ref. 71. Copyright 2021 American Chemical Society. | |
5. Prospects and challenges of solid-state CDs for lighting and displays
In recent years, significant efforts have been made to develop next-generation solid-state lighting and display technologies based on SSCDs. For phosphor-based WLEDs, the current efficiencies of CD-based warm WLEDs are still relatively low compared with well-developed QD-WLEDs with a high luminous efficiency and CRI. Consequently, it is imperative to synthesize SSCDs on a large scale with high QYs that are comparable to traditional semiconductor QDs, particularly for longer wavelengths beyond the blue light region. Additionally, single component warm WLEDs based on intrinsically broadband white emissive SSCDs covering the entire visible spectral window from 400 to 700 nm are highly desired for solid-state lighting. In comparison to phosphor-converted LEDs, electroluminescence based on SSCDs holds great significance due to its potential for achieving higher electro-optical conversion efficiency. However, the research on SSCD-based electroluminescent LEDs, especially for high color purity display technology, is still at an early stage with a low EQE and broad FWHM (>50 nm) compared with well-developed QD emitters. Furthermore, efficient color-tunable RTP or TADF CDs as well as optimization of the charge injection with better controlled device fabrication are of great necessity and significance for the realization of full-color displays. Hence, there are still several challenges that need to be addressed for the practical LED applications of SSCDs.
5.1 Efficient full-color triplet-excited-state-involved solid-state CDs
For next-generation electroluminescent displays, high-performance RTP and TADF CDs as well as short lifetimes are highly desired for breaking through the 5% EQE limitation of traditional fluorescent devices. However, it should be noted that most of the reported triplet-excited-state-involved emissions from CDs in host–guest systems have shown relatively dim luminescence due to inefficient SOC and longer lifetimes in the second regime. Strictly speaking, this emission can be classified as persistent afterglow, which poses a significant challenge for their practical applications. Accordingly, the most critical way to develop efficient CD-based RTP or TADF emitters is to reduce ΔEST by manipulating the separation of the HOMO and the LUMO for boosting the ISC or RISC. In fact, CDs possessing dominant (π, π*) characteristics in singlet and triplet states normally have a large ΔEST due to its plane sp2 π-conjugation domains and, consequently, a slow kISC value. By following the design principles of pure organic RTP or TADF emitters, CDs can be utilized as either donors or acceptors. Through the incorporation of acceptor or donor subunits, intramolecular charge-transfer states can be generated, acting as intermediate states to minimize ΔEST. For instance, electron-donating aromatics (donor) such as carbazole, diphenylamine, phenoxazine, etc., can be grafted into the CDs containing electron-donating groups (acceptor) through the one-pot reactions or post-synthetic modification for efficient kISC. In addition, noteworthily, multiple resonance (MR) TADF emitters with planar boron- and oxygen (or nitrogen)-containing arene compounds can be taken as an example for designing efficient CD-based TADF emitters.86 Specifically, it is likely to select polycyclic aromatic hydrocarbons (PAHs) containing boron, nitrogen, phosphorus, and sulfur to prepare nanographene, which is potentially advantageous for the production of complementary resonance effects, and hence the electron density distributions on the HOMO and LUMO orbitals are offset by one atom, leading to a small ΔEST value.87
5.2 Tailored design of solid-state CDs with narrow bandwidth emission
Narrowband emission is critically vital for the development of energy-saving and high-quality displays with a wide color gamut.49 Despite some progress in the preparation of narrow bandwidth CDs ranging from blue to red with high PLQYs, it is important to note that all the reported CDs to date have only demonstrated efficient light emission in solutions.88,89 SSCDs with narrow bandwidths have not been realized yet. The most significant challenges lie in the rational synthesis of SSCDs with narrow bandwidth and understanding their intrinsic mechanisms at the molecular level. Recently, a variety of B, N-PAHs have been developed as narrow bandwidth TADF solid-state emitters possessing planar and conjugate structures for improved color purity, in which electron donating atom (donor) and electron deficient atom (acceptor) disposed para to each other. Hence, there is a strong need for a simple and versatile approach that allows for the incorporation of multiple boron centres and the modulation of their arrangement and electron-accepting characteristics. Such an approach would enable systematic investigations into the structure–property relationship of nanographene as practical functional π-conjugated materials. Choosing precursors with B–N-containing skeletons to enlarge planar π-systems, may become a feasible strategy for the development of novel CD-based TADF emitters. For instance, PAH with carbazole groups is considered as donor units and boron tribromide as acceptor units in the synthesis of SSCDs. The nitrogen atom exhibits an opposite resonance effect compared to boron atoms. para-Substitution relative to the nitrogen atom can enhance the resonance effect and effectively increase the separation between the HOMO and the LUMO without the need for introducing additional donor or acceptor groups, leading to a smaller ΔEST. Additionally, narrowband emissive CDs can be developed through various modification agents, including small molecules and polymers.
5.3 Enhancing the organic solubility of solid-state CDs
Solution processes, such as spin coating and inkjet printing, have demonstrated great potential in enabling low-cost and large-area manufacturing of QLEDs. Therefore, it is crucial to realize CDs with good solubility that can readily form uniform thin films with low surface roughness.48,90–92 Undoubtedly, the requirement for good solution processability poses an additional challenge in the design of SSCD emitters. On one hand, these emitters must have good enough solubility in common organic solvents to make a solution process feasible. On the other hand, these molecules should form high quality and pinhole-free uniform films without aggregation and phase separation in the solution process. Furthermore, these films are expected to exhibit excellent morphological and thermal stabilities during device operation at high current density. Generally, SSCDs prepared from ‘‘bottom-up’’ synthetic approaches are functionalized with abundant groups, such as carboxyl, amidogen, and hydroxyl groups, indigenously endowing their good solubility. The precursors chosen often determine the solubility of the resulting SSCDs, triphenylamine structures are ideal for the synthesis of CDs. For example, triphenylamine-derived solid-state emissive O-CDs and Y-CDs were synthesized, showing good solubility in various non-polar organic solvents and having good electrical conductivity.48 Furthermore, the post-synthesis modification of CDs is another potential strategy to regulate their solubility. White-light-emitting GQDs through oxidative-cutting and post-synthesis functionalization with bulky Fréchet's dendritic wedges were synthesized,93 which is soluble in the common organic solvents, such as dichloromethane, tetrahydrofuran, and acetonitrile.
5.5 Balanced charge injection and transportation
Currently, the primary focus in CD-based LED research is to enhance the quality of the emissive layer. However, the optimization of the device structure has not received sufficient attention. In addition to the fabrication of a high-performance CD-based emissive layer, interface optimization of the device is also important for achieving high-quality LEDs, as already demonstrated in OLED, QLED, and perovskite LED areas. The carrier mobility and energy level structure of the interface transport layer directly determine the efficiency and balance of the carrier injection and the blocking effect of the reverse carrier.94 The selection of appropriate interface transport layer materials can ensure the formation of a better energy level alignment, thus improving the performance of the device. For instance, the introduction of a TFB layer could remarkably improve the charge injection and balance in the active emission layer and thereby improve the device performance.15
5.5 Chemical functionalization
Chemical functionalization of CDs is a potential strategy to regulate their solid-state photoluminescence, FWHM, and solubility properties. Generally, CDs prepared from ‘‘bottom-up’’ synthetic approaches are indigenously functionalized with abundant surface groups, especially oxygen related functional groups, such as carboxyl and hydroxyl, which impart suitable chemically reactive groups for surface passivation and functionalization. For instance, modifying dendrimers or polymers at the edges of CDs may be a feasible strategy for preventing self-aggregation quenching and resulting in efficient solid-state luminescence.93 SSCD-based narrow bandwidths possibly will be achieved by introducing heteroatoms such as O, N, B and Se to inhibit strong vibronic coupling and meanwhile suppress the structural changes between the S0 and excited S1 states for achieving high color purity light emission.95 Moreover, to increase the organic solubility of the desired SSCDs, one can use precursors that contain a significant number of tert-butyl functional groups covalently attached to the edges of the CD moieties.96
Author contributions
Conceptualization: F. Y.; writing – original draft: T. Y. and Q. T. (contributed equally to this work); writing review & editing: C. L., S. L., W. S., Z. S., Y. S., H. X., Y. H., S. W., Y. Z., X. L., Y. L., L. F. and F. Y.; supervision: L. F. and F. Y.
Conflicts of interest
There are no conflicts to declare.
Acknowledgements
Ting Yuan and Qian Teng contributed equally to this work. This work was supported by the NSFC of China (22172008, 21872010, 22302012, and 22305052), the National Key Research and Development Program of China (2019YFE0112200), the China Postdoctoral Science Foundation (2021M690803), and the National Science Fund for Excellent Young Scholars (Overseas).
Notes and references
- F. Yuan, S. Li, Z. Fan, X. Meng, L. Fan and S. Yang, Nano Today, 2016, 11, 565–586 CrossRef CAS.
- C. Hu, M. Li, J. Qiu and Y. P. Sun, Chem. Soc. Rev., 2019, 48, 2315–2337 RSC.
- T. C. Wareing, P. Gentile and A. N. Phan, ACS Nano, 2021, 15, 15471–15501 CrossRef CAS PubMed.
- X. Xu, Y. Gu, H. J. Ploehn, L. Gearheart, K. Raker and W. A. Scrivens, J. Am. Chem. Soc., 2004, 126, 12736–12737 CrossRef CAS.
- Y. P. Sun, Y. Lin, W. Wang, K. A. S. Fernando, P. Pathak, M. J. Meziani, B. A. Harruff, X. Wang, H. F. Wang, P. G. Luo, H. Yang, M. E. Kose, B. L. Chen, L. M. Veca and S. Y. Xie, J. Am. Chem. Soc., 2006, 128, 7756 CrossRef CAS PubMed.
- T. Yuan, T. Meng, P. He, Y. Shi, Y. Li, X. Li, L. Fan and S. Yang, J. Mater. Chem. C, 2019, 7, 6820–6835 RSC.
- S. Miao, K. Liang, J. Zhu, B. Yang, D. Zhao and B. Kong, Nano Today, 2020, 33, 100879 CrossRef CAS.
- G. Ragazzon, A. Cadranel, E. V. Ushakova, Y. Wang, D. M. Guldi, A. L. Rogach, N. A. Kotov and M. Prato, Chem, 2021, 7, 606–628 CAS.
- L. Ethordevic, F. Arcudi, M. Cacioppo and M. Prato, Nat. Nanotechnol., 2022, 17, 112–130 CrossRef PubMed.
- Z. Ding, F. Li, J. Wen, X. Wang and R. Sun, Green Chem., 2018, 20, 1383–1390 RSC.
- X. Li, M. Rui, J. Song, Z. Shen and H. Zeng, Adv. Funct. Mater., 2015, 25, 4929–4947 CrossRef CAS.
- Y. Yan, J. Gong, J. Chen, Z. Zeng, W. Huang, K. Pu, J. Liu and P. Chen, Adv. Mater., 2019, 31, 1808283 CrossRef PubMed.
- B. Zhao and Z. Tan, Adv. Sci., 2021, 8, 2001977 CrossRef CAS PubMed.
- T. Yuan, T. Meng, Y. Shi, X. Song, W. Xie, Y. Li, X. Li, Y. Zhang and L. Fan, J. Mater. Chem. C, 2022, 10, 2333–2348 RSC.
- F. Yuan, Y.-K. Wang, G. Sharma, Y. Dong, X. Zheng, P. Li, A. Johnston, G. Bappi, J. Z. Fan, H. Kung, B. Chen, M. I. Saidaminov, K. Singh, O. Voznyy, O. M. Bakr, Z.-H. Lu and E. H. Sargent, Nat. Photon., 2019, 14, 171–176 CrossRef.
- Y. Shi, W. Su, F. Yuan, T. Yuan, X. Song, Y. Han, S. Wei, Y. Zhang, Y. Li, X. Li and L. Fan, Adv. Mater., 2023, 2210699 CrossRef CAS.
- J. Wang, Y. Yang and X. Liu, Mater. Adv., 2020, 1, 3122–3142 RSC.
- F. Yan, C. Yi, J. Sun, Y. Zang, Y. Wang, M. Xu and J. Xu, Mikrochim. Acta, 2021, 188, 412 CrossRef CAS PubMed.
- H. J. Yoo, B. E. Kwak and D. H. Kim, J. Phys. Chem. C, 2019, 123, 27124–27131 CrossRef CAS.
- J. Ren, L. Stagi and P. Innocenzi, Prog. Solid State Chem., 2021, 62, 100295 CrossRef CAS.
- T. Feng, S. Zhu, Q. Zeng, S. Lu, S. Tao, J. Liu and B. Yang, ACS Appl. Mater. Interfaces, 2018, 10, 12262–12277 CrossRef CAS PubMed.
- A. Xu, G. Wang, Y. Li, H. Dong, S. Yang, P. He and G. Ding, Small, 2020, 16, e2004621 CrossRef PubMed.
- M. Godumala, S. Choi, M. J. Cho and D. H. Choi, J. Mater. Chem. C, 2019, 7, 2172–2198 RSC.
- J. Zhu, X. Bai, Y. Zhai, X. Chen, Y. Zhu, G. Pan, H. Zhang, B. Dong and H. Song, J. Mater. Chem. C, 2017, 5, 11416–11420 RSC.
- T. Loythaworn, S. Petdee, P. Chasing, N. Chantanop, P. Therdkatanyuphong, W. Waengdongbung, T. Sudyoadsuk and V. Promarak, Mater. Chem. Front., 2022, 6, 3225–3236 RSC.
- D. Zhou, D. Li, P. Jing, Y. Zhai, D. Shen, S. Qu and A. L. Rogach, Chem. Mater., 2017, 29, 1779–1787 CrossRef CAS.
- M. Park, Y. Jeong, H. S. Kim, W. Lee, S. H. Nam, S. Lee, H. Yoon, J. Kim, S. Yoo and S. Jeon, Adv. Funct. Mater., 2021, 31, 2102741 CrossRef CAS.
- X. He, Y. Liu, C. J. Butch, B. R. Lee, F. Guo, J. Wu, Z. Wang, Q. Lu, J. H. Jeong, Y. Wang and S. H. Park, Small, 2019, 15, e1902735 CrossRef.
- J. C. Liu, Y. Yu, Y. Yan, H. Y. Zhang, J. Y. Li and J. H. Yu, Sci. Adv., 2017, 3, e1603171 CrossRef PubMed.
- D. Zhou, S. Qu, D. Li, P. Jing, W. Ji, D. Shen and A. L. Rogach, Small, 2017, 13, 1602055 CrossRef PubMed.
- J. Guo, Y. Lu, A. Q. Xie, G. Li, Z. B. Liang, C. F. Wang, X. Yang and S. Chen, Adv. Funct. Mater., 2022, 32, 2110393 CrossRef CAS.
- B. E. Kwak, H. J. Yoo and D. H. Kim, Adv. Opt. Mater., 2019, 7, 1900932 CrossRef CAS.
- X. Li, Y. Liu, X. Song, H. Wang, H. Gu and H. Zeng, Angew. Chem., Int. Ed., 2015, 54, 1759–1764 CrossRef CAS PubMed.
- Z. Xie and C. Liu, Adv. Mater., 2012, 24, 1716 CrossRef CAS PubMed.
- X. Xu, L. Mo, Y. Li, X. Pan, G. Hu, B. Lei, X. Zhang, M. Zheng, J. Zhuang, Y. Liu and C. Hu, Adv. Mater., 2021, 33, e2104872 CrossRef PubMed.
- Y. Chen, M. Zheng, Y. Xiao, H. Dong, H. Zhang, J. Zhuang, H. Hu, B. Lei and Y. Liu, Adv. Mater., 2016, 28, 312–318 CrossRef CAS PubMed.
- J. Shao, S. Zhu, H. Liu, Y. Song, S. Tao and B. Yang, Adv. Sci., 2017, 4, 1700395 CrossRef PubMed.
- H. He, R. Zhang, P. Zhang, P. Wang, N. Chen, B. Qian, L. Zhang, J. Yu and B. Dai, Adv. Sci., 2023, 10, e2205557 CrossRef PubMed.
- H. Zhang, B. Wang, X. Yu, J. Li, J. Shang and J. Yu, Angew. Chem., Int. Ed., 2020, 59, 19390–19402 CrossRef CAS PubMed.
- C. Xia, S. Zhu, T. Feng, M. Yang and B. Yang, Adv. Sci., 2019, 6, 1901316 CrossRef CAS PubMed.
- H. Wang, L. Ai, H. Song, Z. Song, X. Yong, S. Qu and S. Lu, Adv. Funct. Mater., 2023, 2303756 CrossRef CAS.
- S. H. Li, Y. C. Li, X. H. Li, J. Zhu, L. Z. Fan and S. H. Yang, ACS Appl. Mater. Interfaces, 2017, 9, 22332 CrossRef CAS PubMed.
- C. He, P. Xu, X. Zhang and W. Long, Carbon, 2022, 186, 91–127 CrossRef CAS.
- S. Yang, Y. Li, L. Chen, H. Wang, L. Shang, P. He, H. Dong, G. Wang and G. Ding, Small, 2023, 2205957, DOI:10.1002/smll.202205957.
- Q. Lou, Q. Ni, C. Niu, J. Wei, Z. Zhang, W. Shen, C. Shen, C. Qin, G. Zheng, K. Liu, J. Zang, L. Dong and C. X. Shan, Adv. Sci., 2022, 9, e2203622 CrossRef PubMed.
- T. Yuan, F. Yuan, L. Sui, Y. Zhang, Y. Li, X. Li, Z. A. Tan and L. Fan, Angew. Chem., Int. Ed., 2023, 62, e202218568 CrossRef CAS PubMed.
- H. Jia, Z. Wang, T. Yuan, F. Yuan, X. Li, Y. Li, Z. Tan, L. Fan and S. Yang, Adv. Sci., 2019, 6, 1900397 CrossRef PubMed.
- B. Zhao, H. Ma, H. Jia, M. Zheng, K. Xu, R. Yu, S. Qu and Z. Tan, Angew. Chem., Int. Ed., 2023, 62, e202301651 CrossRef CAS PubMed.
- W. Kwon, Y. H. Kim, J. H. Kim, T. Lee, S. Do, Y. Park, M. S. Jeong, T. W. Lee and S. W. Rhee, Sci. Rep., 2016, 6, 24205 CrossRef CAS PubMed.
- T. Meng, Z. Wang, T. Yuan, X. Li, Y. Li, Y. Zhang and L. Fan, Angew. Chem., Int. Ed., 2021, 60, 16343–16348 CrossRef CAS PubMed.
- L. Ai, Z. Song, M. Nie, J. Yu, F. Liu, H. Song, B. Zhang, G. I. N. Waterhouse and S. Lu, Angew. Chem., Int. Ed., 2023, 62, e202217822 CrossRef CAS PubMed.
- B. Xu, J. Li, J. Zhang, H. Ning, X. Fang, J. Shen, H. Zhou, T. Jiang, Z. Gao, X. Meng and Z. Wang, Adv. Sci., 2023, 10, e2205788 CrossRef PubMed.
- Z. Yang, Z. Mao, Z. Xie, Y. Zhang, S. Liu, J. Zhao, J. Xu, Z. Chi and M. P. Aldred, Chem. Soc. Rev., 2017, 46, 915–1016 RSC.
- M. Park, J. Lee, J. Kim, J. Lee, S. E. Lee, S. Yoo and S. Jeon, Adv. Mater., 2018, 30, 1802951 CrossRef.
- C. Luk, W. Zhang, S. Yu, K. Teng and S. Lau, J. Mater. Chem. C, 2012, 22, 22378 RSC.
- J. Wang, J. Zheng, Y. Yang, X. Liu, J. Qiu and Y. Tian, Carbon, 2022, 190, 22–31 CrossRef CAS.
- G. Hu, Y. Wang, S. Zhang, H. Ding, Z. Zhou, J. Wei, X. Li and H. Xiong, Carbon, 2023, 203, 1–10 CrossRef CAS.
- T. Feng, Q. Zeng, S. Lu, X. Yan, J. Liu, S. Tao, M. Yang and B. Yang, ACS Photonics, 2017, 5, 502–510 CrossRef.
- J. He, Y. He, Y. Chen, B. Lei, J. Zhuang, Y. Xiao, Y. Liang, M. Zheng, H. Zhang and Y. Liu, Small, 2017, 13, 1700075 CrossRef.
- H. Yang, Y. Liu, Z. Guo, B. Lei, J. Zhuang, X. Zhang, Z. Liu and C. Hu, Nat. Commun., 2019, 10, 1789 CrossRef PubMed.
- T. Liu, G. Yin, Z. Song, J. Yu, X. Yong, B. Zhang, L. Ai and S. Lu, ACS Mater. Lett., 2023, 5, 846–853 CrossRef CAS.
- L. Tang, X. Cao, J. Lin, H. Jiang, X. Li, K. S. Teng, S. Z. C. M. Luk, J. Hao and S. P. Lau, ACS Nano, 2012, 6, 5102 CrossRef CAS.
- S. Song, J. Wei, Q. Lou, Y. Shang and C. Shan, Nano Lett., 2019, 19, 5553 CrossRef CAS.
- J. Xu, Q. Liang, Z. Li, V. Y. Osipov, Y. Lin, B. Ge, Q. Xu, J. Zhu and H. Bi, Adv. Mater., 2022, 34, 2200011 CrossRef CAS.
- Q. Cao, K. K. Liu, Y. C. Liang, S. Y. Song, Y. Deng, X. Mao, Y. Wang, W. B. Zhao, Q. Lou and C. X. Shan, Nano Lett., 2022, 22, 4097–4105 CrossRef CAS PubMed.
- W. Li, Z. Zhou, H. Zhang, X. Zhang, J. Zhuang, Y. Liu, B. Lei and C. Hu, Angew. Chem., Int. Ed., 2019, 58, 7278 CrossRef CAS PubMed.
- D. C. Green, M. A. Levenstein, S. Zhang, B. R. G. Johnson, J. Pablo, A. Ward, S. W. Botchway and F. C. Meldrum, Nat. Commun., 2019, 10, 206 CrossRef.
- S. Tao, Y. Geng, S. Zhu, S. A. T. Redfern, Y. Song, T. Feng, W. Xu and B. Yang, Angew. Chem., Int. Ed., 2018, 57, 2417 CrossRef.
- K. Jiang, X. Gao, C. Cai and H. Lin, Angew. Chem., Int. Ed., 2018, 57, 6216 CrossRef CAS.
- T. Yuan, F. Yuan, X. Li, Y. Li, L. Fan and S. Yang, Chem. Sci., 2019, 10, 9801–9806 RSC.
- Y. Shi, Z. Wang, T. Meng, T. Yuan, R. Ni, Y. Li, X. Li, Y. Zhang, Z. Tan, S. Lei and L. Fan, J. Am. Chem. Soc., 2021, 143, 18941–18951 CrossRef CAS.
- Y. Zheng, P. Liang, X. Xu, X. Zhang, H. Li, C. Zhang, C. Hu, X. Zhang, B. Lei, W. Y. Wong, Y. Liu and J. Zhuang, Angew. Chem., Int. Ed., 2021, 60, 22253 CrossRef CAS PubMed.
- Q. Lou, N. Chen, J. Zhu, K. Liu, C. Li, Y. Zhu, W. Xu, X. Chen, Z. Song, C. Liang, C. X. Shan and J. Hu, Adv. Mater., 2023, 35, e2211858 CrossRef.
- J. He, Y. Chen, Y. He, X. Xu, B. Lei, H. Zhang, J. Zhuang, C. Hu and Y. Liu, Small, 2020, 16, e2005228 CrossRef PubMed.
- M. Park, H. S. Kim, H. Yoon, J. Kim, S. Lee, S. Yoo and S. Jeon, Adv. Mater., 2020, 32, 2000936 CrossRef CAS.
- D. Chen, H. Gao, X. Chen, G. Fang, S. Yuan and Y. Yuan, ACS Photonics, 2017, 4, 2352–2358 CrossRef CAS.
- F. Yuan, Z. Wang, X. Li, Y. Li, Z. Tan, L. Fan and S. Yang, Adv. Mater., 2017, 29, 1604436 CrossRef.
- F. Yuan, T. Yuan, L. Sui, Z. Wang, Z. Xi, Y. Li, X. Li, L. Fan, Z. Tan, A. Chen, M. Jin and S. Yang, Nat. Commun., 2018, 9, 2249 CrossRef PubMed.
- Y. Zhan, B. Shang, M. Chen and L. Wu, Small, 2019, 15, e1901161 CrossRef PubMed.
- Z. Wang, F. Yuan, X. Li, Y. Li, H. Zhong, L. Fan and S. Yang, Adv. Mater., 2017, 29, 1702910 CrossRef PubMed.
- Q. Li, Y. Li, S. Meng, J. Yang, Y. Qin, J. Tan and S. Qu, J. Mater. Chem. C, 2021, 9, 6796–6801 RSC.
- T. Meng, T. Yuan, X. Li, Y. Li, L. Fan and S. Yang, Chem. Commun., 2019, 55, 6531–6534 RSC.
- Y. Ding, P. He, S. Li, B. Chang, S. Zhang, Z. Wang, J. Chen, J. Yu, S. Wu, H. Zeng and L. Tao, ACS Nano, 2021, 15, 14610–14617 CrossRef CAS PubMed.
- Z. Wang, N. Jiang, M. Liu, R. Zhang, F. Huang and D. Chen, Small, 2021, 17, e2104551 CrossRef PubMed.
- M. Zheng, H. Jia, B. Zhao, C. Zhang, Q. Dang, H. Ma, K. Xu and Z. Tan, Small, 2023, 2206715, DOI:10.1002/smll.202206715.
- J. M. Ha, A. Pathak, J.-E. Jeong and H. Y. Woo, NPG Asia Mater., 2021, 13, 53 CrossRef CAS.
- S. Madayanad Suresh, D. Beljonne, Y. Olivier and E. Zysman-Colman, Adv. Funct. Mater., 2020, 30, 1908677 CrossRef CAS.
- F. Yuan, Z. Xi, X. Li, Y. Li, H. Zhong, L. Fan and S. Yang, Nano Res., 2019, 12, 1669 CrossRef CAS.
- J. Liu, D. Li, H. Yao, Z. Huo, Y. Li, K. Zhang, S. Zhu, H. Wei, W. Xu, J. Jiang and B. Yang, Adv. Mater., 2020, 32, 1906641 CrossRef CAS PubMed.
- G. Zhao, P. Wang, X. Huang, H. Chen, Y. Zhang, D. Zhang, W. Jiang, Y. Sun and L. Duan, Angew. Chem., Int. Ed., 2022, 61, e202212861 CrossRef CAS.
- J. Zhang, Z. Ma, F. Yuan, X. Zhou, H. Wang, Z. Liu, J. Qing, H. Chen, X. Li, S. Su, J. Xie, Z. Shi, L. Hou and C. Shan, Adv. Mater., 2023, 35, 2209002 CrossRef CAS PubMed.
- F. Yuan, A. Johnston, Y. Wang, C. Zhou, Y. Dong, B. Chen, H. Chen, J. Z. Fan, G. Sharma, P. Li, Y. Gao, O. Voznyy, H. Kung, Z. H. Lu, O. M. Bakr and E. H. Sargent, Sci. Adv., 2020, 6, eabb0253 CrossRef CAS PubMed.
- R. Sekiya, H. Murakami and T. Haino, Angew. Chem., Int. Ed., 2014, 53, 5619 CrossRef CAS PubMed.
- H. Jia, H. Ma, X. Liu, D. Xu, T. Yuan, C. Zou and Z. A. Tan, Appl. Phys. Rev., 2022, 9, 021406 CAS.
- F. Arcudi, S. Rebeccani, M. Cacioppo, A. Zanut, G. Valenti, F. Paolucci and M. Prato, Adv. Sci., 2021, 8, 2100125 CrossRef CAS PubMed.
- X. Yan, X. Cui and L.-S. Li, J. Am. Chem. Soc., 2010, 132, 5944 CrossRef CAS PubMed.
|
This journal is © The Royal Society of Chemistry 2024 |
Click here to see how this site uses Cookies. View our privacy policy here.