DOI:
10.1039/D4IM00066H
(Review Article)
Ind. Chem. Mater., 2024, Advance Article
Recent advances on cellulose-based solid polymer electrolytes
Received
5th June 2024
, Accepted 22nd July 2024
First published on 29th July 2024
Abstract
Solid-state Li-metal batteries with solid-state electrolytes have attracted increasing attention due to their high energy density and intrinsically high safety. Among diverse available solid-state electrolytes, cellulose-based solid polymer electrolytes (CSPEs) are particularly attractive and have showcased great promise because of their multiple merits including abundant reserves, abundant polar groups, chemical stability and high flexibility. This review surveys currently-developed solid electrolytes based on modified cellulose and its composites with diverse organic and inorganic fillers. Common preparation methods for solid electrolyte membranes are discussed in detail, followed by a sequential overview of various modification and compositing strategies for improving Li-ion transport in CSPEs, and a summary of the current existing challenges and future prospects of CSPEs to achieve high-performance solid batteries.
Keywords: Cellulose-based solid polymer electrolytes (CSPEs); Li-metal batteries; Ionic conductivity; Interface stability.
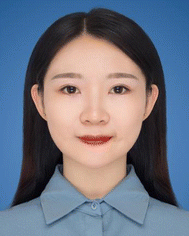 Xiaoqi Gong | Xiaoqi Gong is currently a Ph.D. student in Sun Yat-Sen University under the supervision of Prof. Dingshan Yu. She received her B.E. from Qingdao Agricultural University in 2018 and M.E. from Fujian Agriculture and Forestry University of Light Industry Technology and Engineering in 2021. Her research interests lie in cellulose-based solid polymer electrolytes in Li-metal batteries. |
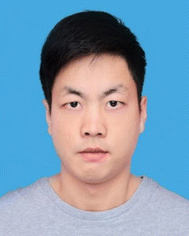 Cong Liu | Cong Liu is currently a postdoctoral fellow in Sun Yat-Sen University. He received his M.S. and Ph.D. degrees from South China Normal University in 2020 and Sun Yat-Sen University in 2024. His research interests focus on Li–S batteries, solid-state batteries and Li-metal batteries. |
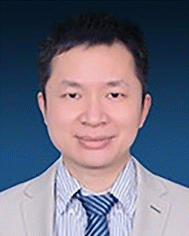 Dingshan Yu | Dingshan Yu received his Ph.D. in optical engineering in 2008 from Sun Yat-sen University (China). After postdoctoral training at the University of Dayton (USA), Case Western Reserve University (USA), and Nanyang Technological University (Singapore), he joined Sun Yat-sen University as a professor in 2015. His research interests include design and synthesis of polymeric functional composites and carbon materials as well as their application in optoelectronic devices and energy storage and conversion systems. |
1 Introduction
Since the commercialization of Li-ion batteries (LIBs) in the 1990s, they have achieved rapid growth in the rechargeable battery market, which stimulated the development of portable electronics in form and function.1–3 The success of LIBs is largely attributed to the use of organic electrolytes, which endow the highest operation voltages (currently 3.7 V).4,5 Nevertheless, these highly volatile and flammable organic electrolytes can trigger serious safety issues under some abuse conditions (i.e., short-circuited or overcharged).6–8 In addition, the limited energy density provided by commercial LIBs cannot fulfil the ever-increasing demands of hybrid electrical vehicles and large-scale power-grid systems. Luckily, Li-metal batteries provide a super-high theoretical specific capacity (3860 mA h g−1) and the most negative electrochemical potential (−3.04 V vs. SHE), which can further pair with a desirable cathode to potentially achieve a larger capacity and a higher operating voltage, eventually realizing a high energy density.9–12 Yet, the growth of dendrites on Li anode surfaces during charging can puncture the separator and lead to a series of safety hazards.13,14 Thus, significant efforts have focused on the development of high-performance battery systems with high energy density, good safety and affordable cost.15 Solid-state Li batteries using a solid electrolyte and “holy-grail” Li-metal anode have emerged as a promising solution.16,17 Note that solid-state electrolytes can effectively avoid the short-circuiting and electronic conduction during the crossover of Li+ between the anode and cathode.18,19 In general, solid-state electrolytes are classified into inorganics, polymers and composites.20 Among them, inorganic solid electrolytes mainly include oxides (e.g. garnet-type, perovskite-type, NASICON-type), sulfides, etc.21 Oxide electrolytes have the advantages of good thermal stability, wide electrochemical window, and high mechanical strength, making them the fastest developing solid-state electrolytes in recent years, while their disadvantages lie in modest room-temperature (RT) ionic conductivity, strenuous fabrication process, and huge interfacial impedance with electrodes.22–26 As for sulfides, they always exhibit a high RT ionic conductivity (∼10−2 S cm−1). However, sulfide electrolytes tend to suffer from sensitivity to H2O/O2, rigorous preparation conditions, and poor solid–solid contact with electrodes, which seriously hinder their practical uses in solid-state Li-metal batteries. By comparison, solid polymer electrolytes (SPEs) show multiple advantages in terms of high flexibility, excellent processability and good interface compatibility with the electrodes.27–31 However, the crystallization phase in SPEs (e.g. poly(ethylene oxide,32 poly(vinylidene fluoride),33 polyacrylonitrile,34 polycarbonate,35 and so on) do not favour Li-ion migration and therefore compromise the overall ionic conductivity of the electrolyte at RT.32–39 On the other hand, the narrow electrochemical stable window of SPEs cannot maintain electrochemical and chemical stability with the paired cathode.40,41 Thus, it is highly desirable but challenging to overcome the drawbacks of conventional SPEs as described above.42,43
Among various SPEs, cellulose-based solid polymer electrolytes (CSPEs) have gained increasing attention due to their merits in material abundance, biodegradable property, cohesive energy, chemical stability, and high flexibility. In particular, the abundant polar chemical groups (such as –OH, –O–) in cellulose can facilitate the dissolution of lithium salts and thereby afford the ion conduction ability.44–49 However, the poor solubility of cellulose materials has plagued the deployment of CSPEs. Given this, modifying cellulose through etherification, esterification, grafting copolymerization or crosslinking can be regarded as a feasible approach to overcome the insolubility issues. Accordingly, amorphous cellulose derivatives such as cellulose acetate and oxidized carboxymethyl cellulose with diverse functional groups such as high electron-donor moieties are often explored for uses in SSLBs.50–53 Moreover, CSPEs are also constrained by lower ionic conductivity due to their packed molecular chains that can prevent Li-ion movement.54 Substantial studies have demonstrated that desirable fillers55–58 such as inorganic fillers, ionic liquids, or plastic crystalline molecules can be introduced to disrupt the regularity of the cellulose chains and tune the Li+ chemical environment for improving Li-ion transport.59,60 To date, a series of cellulose and its derivatives have been developed as solid electrolytes. However, to our knowledge, there are only a few comprehensive reviews regarding cellulose-based solid electrolytes.61,62 These available reviews mainly focused on the cellulose-derived solid electrolytes for Li ion batteries rather than Li metal batteries.
In this review, we present a comprehensive overview on recent achievements on CSPEs mainly within the past five years, which focuses on cellulose and its derivatives as well as their composites with diverse inorganic and organic fillers. Besides, Li-ion transport behavior in CSPEs is discussed in detail. The purpose of our review is to establish a contemporary concept of the current field of cellulose-based solid electrolytes and to provide inspiration for its future development in solid Li-metal batteries. As shown in Fig. 1, we discuss in detail several common preparation methods, followed by diverse modifications (i.e., coordination, chemical grafting and cross-linking) and compositing strategies with different inorganic and organic fillers for improving the ionic conductivity and interface stability of CSPEs. Finally, current challenges and future perspectives of CSPEs are concluded to promote their development.
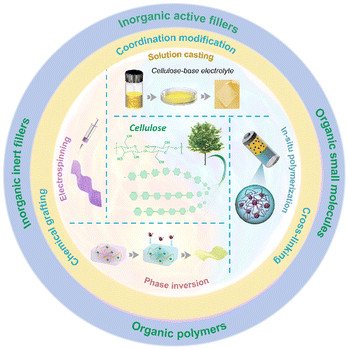 |
| Fig. 1 Schematic diagram of preparation methods, modification methods, and classification of composite materials for cellulose-based solid electrolytes. | |
2 Preparation of cellulose-based solid electrolytes
Generally, the preparation method of solid polymer electrolytes affects their physicochemical properties especially the ionic conductivity. Various methods have been explored to construct CSPEs including solution casting, hot pressing, electrostatic spinning, and in situ polymerization.63,64
2.1 Solution casting
Solution casting is the most common preparation method of SPEs, which can facilely regulate the film thickness and allow for large-area production. The solidification process is achieved by evaporating the solvent in the SPE slurry on an inert mold or by doctor-blade casting as thin films.65 It should be pointed out that the solvents used in the casting process should be volatile and chemically inert to the dispersed material. Considering that original cellulose is poorly soluble in most solvents, dissoluble cellulose derivatives were often adopted for the design of high-performance SPEs in recent studies.66 To overcome the insoluble limitation of cellulose, the esterifying strategy was used to modify original cellulose and improve its solution processability.67 The resulting derivative could be readily dissolved in 2-butanone without any precipitation even after being settled for 1 month (Fig. 2a), which was then grated with ion-conducting polymer segments to attain the brush-like polymer. As such, by casting a homogeneous solution of brush-like cellulose and LiTFSI, a free-standing and stretchable solid electrolyte could be easily acquired, which had a thickness of ∼100 μm and showed an ordered nanolayered structure (Fig. 2b and c). This cellulose-based electrolyte with brush-like structures could provide an environment capable for low crystallinity (high segmental mobility) and increased interactions supporting rapid ion transport, thus improving the interfacial stability. Accordingly, the voltage profiles of the assembled Li//Li symmetric cells remained stable even after 700 h of cycling, implying good stability of this solid electrolyte against Li anodes. Beyond this, due to the water-solubility character, methyl cellulose is usually utilized as the polymer matrix for producing an SPE (Fig. 2d). As shown in Fig. 2e and f, the resulting SPE has a thickness of only 20 μm and a smooth surface, which demonstrated a good conductivity of 2 × 10−4 S cm−1 and a broad electrochemical window of 4.8 V, showing excellent solid–solid contacts with electrodes. This endows the assembled solid LiFePO4//Li cell with an excellent reversible capacity of 130 mA h g−1 at 0.2C.68
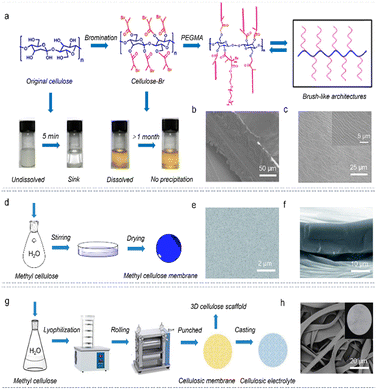 |
| Fig. 2 (a) Synthetic route for the cellulose-based polymer with brush-like structure (copyright 2020 American Chemical Society);67 (b) cross-sectional and (c) surface SEM images of the CSPE (copyright 2020 American Chemical Society);67 (d) preparation process of the methyl cellulose-based membrane (copyright 2013 The Royal Society of Chemistry);68 (e) surface and (f) cross-section SEM images of the cellulosic membrane (copyright 2013 The Royal Society of Chemistry);68 (g) schematic diagram of the preparation process and (h) SEM image of the cellulosic electrolyte; the inset (h) shows the digit photograph (copyright 2022 Elsevier B.V.).69 | |
In addition, cellulose can function as a mold for casting other electrolyte materials. For example, a three-dimensional (3D) cellulose scaffold was fabricated by lyophilization (Fig. 2g),69 which can be coupled with 1 M LiPF6 to garner CSPEs (Fig. 2h). At 25 °C, this solid electrolyte exhibited good ion-conduction ability (0.70 mS cm−1). The designed 3D network structure can guide the homogeneous Li+ flux between the electrolyte and Li anode, resulting in a stable and smooth solid electrolyte interface (SEI) formation. Thereby, the reversible Li+ plating/stripping behavior at 1 mA cm−2/1 mA h cm−2 can proceed over 400 h with a low polarization voltage of 40 mV, indicating the excellent dendrite-inhibiting ability. Furthermore, the constructed solid LiFePO4//Li cells displayed good rate capability and long-term durability.
Despite some advantages of the solution-casting method, it still confronts some inevitable problems: (1) toxic solvent evaporation leads to environmental pollution; (2) low film-forming efficiency; (3) possible recrystallisation of the polymer during prolonged drying; and (4) inhomogeneity of the electrolyte film. Thus, it is urgent to find a solution to overcome the above drawbacks.
2.2 Phase inversion
Phase inversion pertains to the process in which a polymer converts from a solution to a 3D porous solid-state, accompanied by solvent and non-solvent changes.70 Phase inversion can take place in various ways including non-solvent induced phase separation, thermally induced phase separation, solvent-evaporation phase inversion and vapor-deposition phase inversion.71 The formed two methods are quite simple and do not require the use of pore-forming agents to prepare films with porous character.72
During non-solvent induced phase separation, the polymer is dissolved in a diluent to produce a relatively homogeneous solution, and subsequently an extractant is slowly added into the above solution to extract the solvent, resulting in a unique two-phase structure where the polymer is a continuous phase and the solvent is a dispersed phase. Eventually, a polymer membrane with certain porosity was generated after removing the solvent.76,77 Accordingly, a porous carboxymethyl cellulose membrane was produced using a non-solvent evaporation technique, in which the pore structure could be regulated by varying the volume ratio of DMF solvent (Fig. 3a).73 The ionic conductivity and Li+ transference number of the as-obtained porous membrane saturated with 1 M LiPF6 electrolyte at 25 °C can reach 4.8 × 10−4 S cm−1 and 0.46, respectively. When being coupled with the LiFePO4 cathode, the assembled full cell yielded a highly reversible capacity of ∼140 mA h g−1 at 0.2C, along with a stable cycling behavior. In addition, a self-supporting ethyl cellulose/poly(vinylidene fluoride) solid electrolyte was acquired through a non-solvent induced phase separation.78 Distinct from the above report, the structure of the porous membrane was modulated by the addition of ethyl cellulose rather than the solvent. Of note, the polar hydroxyl groups in ethyl cellulose chains and the appropriate pores favor Li-ion migration. Therefore, the as-prepared solid electrolytes presented a high ionic conductivity of 1.33 × 10−3 S cm−1 and excellent interfacial contacts with Li anodes, which endows a 5 V high-voltage Li-metal battery with excellent cycling ability. To suppress the formation of macro voids and improve the pore interconnectivity, an additional pore-forming agent (polyvinyl pyrrolidone) was introduced during phase the inversion process for synthesizing the acetate cellulose-based membrane (Fig. 3b).74 After absorbing a certain amount of liquid electrolyte, the obtained solid electrolyte membrane with a thickness of 40 μm and an average pore size of 2 μm affords a superior ionic conductivity of 4.7 × 10−3 S cm−1 (Fig. 3c and d). This solid electrolyte with a dense structure affords a more stable contact interface between the solid electrolyte and Li anode, enabling reversible Li stripping/plating over 900 h at 0.5 mA h cm−2.
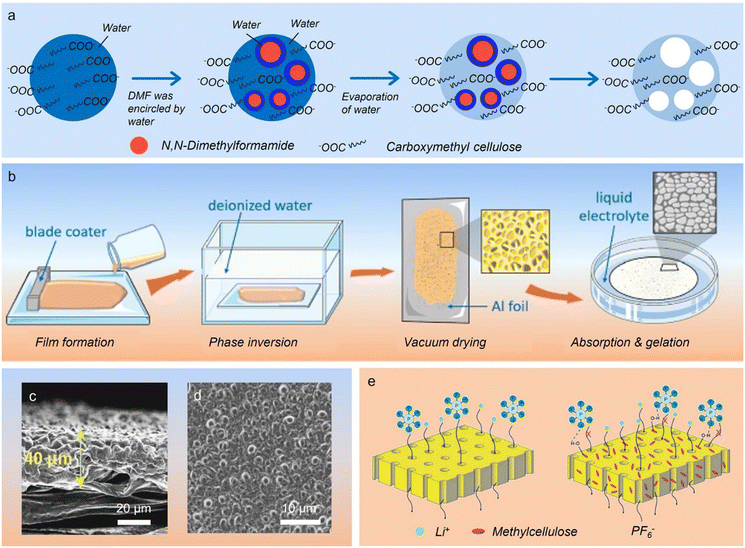 |
| Fig. 3 (a) Schematic process for preparing porous cellulosic membranes via phase separation (copyright 2015 Elsevier B.V.);73 (b) preparation scheme of porous cellulose-based membranes through a phase inversion method (copyright 2022 Wiley-VCH);74 (c) cross-section and (d) top surface SEM images of the porous cellulosic membrane (copyright 2022 Wiley-VCH);74 (e) schematic illustration of ion transport in microporous membranes of hydrophilic high-density polyethylene/methyl cellulose blend (copyright 2016 Elsevier B.V.).75 | |
Thermally induced phase separation is another emerging method for preparing microporous polymer membranes, proposed by Castro in 1981. Unlike non-solvent induced phase separation, the pore formation in such a strategy mainly depends on the removal of heat energy from the solution. Therefore, the solvent will play a decisive role in the preparation of solid membranes with porous structures.79 In this context, a high-boiling-point and low-volatility dioctyl phthalate was selected as the solvent to induce phase separation, which led to the formation of a hydrophilic high-density cellulose-based microporous membrane (Fig. 3e).75 The acquired solid membrane saturated with 1 M LiPF6 showed a high conductivity of 1.01 × 10−3 S cm−1 and excellent interfacial contact with Li metal, thereby generating a stable SEI layer conducive to Li-ion transport.
2.3 Electrostatic spinning
Electrospinning is a universal yet powerful technique for synthesizing 1D nanofibers with the required diameters, which has sparked surging interest since its invention in 1934 owing to its capability to produce a variety of nanofibers, such as polymers, inorganics, and polymer/inorganic composites, showing practical uses in various fields including drug delivery, catalytic materials, energy storage and so on.80 Per these merits, electrospinning has been widely utilized to design and fabricate solid polymer electrolytes.
Among various celluloses, cellulose acetate is a kind of spinnable material. Kang et al.81 produced a novel nanofibrous membrane through electrospinning a blend polymer of polyvinylidene fluoride and cellulose acetate (Fig. 4a, b and d). The solid electrolyte based on the spun membrane has a satisfying electrolyte uptake (768.2%) (Fig. 4c), large breaking strength (11.1 MPa), good thermal stability (no shrinkage at 80 °C) and high ionic conductivity (2.61 × 10−3 S cm−1 at 25 °C). Sabrina et al.82 constructed another solid polymer electrolyte based on the electrospun cellulose acetate membrane. In this electrolyte, polar ester groups electrostatically interact with TFSI−, thereby promoting Li-salt dissociation and favoring Li-ion transfer (Fig. 4e and f). Note that the residual solvent can reduce the crystallinity of polymers, promoting molecular chain movement conducive to Li-ion transport. This is supported by a prominent ionic RT conductivity of 2.68 × 10−3 S cm−1. Further, the interfacial compatibility with Li anodes was assessed by long-term cycling performance of Li//Li cells with this acquired solid electrolyte, which could cycle stably for over 1200 h and maintain a steady polarization voltage. Furthermore, a composite containing cellulose acetate and poly-L-lactic acid was electrospun into a nanofiber membrane for solid polymer electrolytes.84 The rigid cellulose molecular segments and the soft poly-L-lactic acid chains respectively rendered excellent thermal properties and fast ion conduction. This, coupled with a functional clay, resulted in a superb ionic conductivity of 1.52 × 10−3 S cm−1 at 25 °C. The as-garnered solid electrolyte also showed good adaptability with Li metal anodes, bringing about a stable SEI layer that can conduct Li ions and prevent dendrite generation for attaining a good cycling performance. Taken together, the electrospinning approach has been proved to be a facile method to prepare desirable polymer membranes, and the resulting solid electrolyte is mainly acquired via swelling the cellulosic membranes in organic liquid electrolytes.
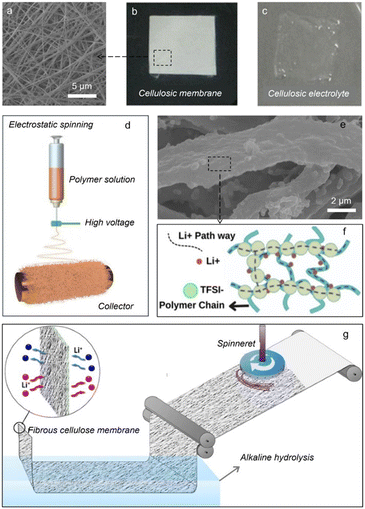 |
| Fig. 4 (a) SEM image and photographs of cellulosic membranes (b) before and (c) after immersion in the electrolyte (copyright 2016 Springer);81 (d) schematic diagram of electrospinning; (e) SEM image and (f) schematic illustration of Li-ion transport of cellulosic membranes (copyright 2024 Springer);82 (g) schematic representation of mass production of a fibrous cellulose membrane using the force-spinning technique (copyright 2015 Springer).83 | |
Considering that some celluloses cannot be spun directly, they can serve as a functional nanofiller for spinnable composites. For example, with a nanocrystalline cellulose as the additive, the solid composite electrolyte showed an improved tensile strength of 17 MPa and a good ionic conductivity of 4 × 10−4 S cm−1 at 30 °C.85 On the other hand, we can also note that electrospinning is carried out under a strong electric field, which draws fibers through the electrostatic force, suffering from a low spinning rate and high production cost. It is thus required to develop other spinning methods without an electric field, such as wet spinning, melt spinning and force spinning. Recently, a porous acetate cellulosic membrane with a three-dimensional network was force-spun (Fig. 4g).83 This porous membrane can achieve a high porosity of 76% and the resulting electrolyte yielded a higher ionic conductivity of 2.12 × 10−3 S cm−1 at 25 °C.
2.4 In situ polymerization
In situ polymerization is another strategy for the preparation of solid polymer electrolytes, in which small monomers can be polymerized under various external stimuli.86 During in situ polymerization, long and short chains are cross-linked to form an amorphous network, while unreacted small molecules are strapped in the cross-linked network via bonding, affording the solid polymer electrolyte with excellent ionic conductivity and mechanical properties.87 By in situ UV-induced cross-linking of allyl-modified cellulose and cellulose acetate, a solid electrolyte with a semi-interpenetrating network was obtained (Fig. 5a).88 After doping black phosphorus nanosheets, the resulting electrolytes exhibited a high ionic conductivity (5.21 × 10−3 S cm−1 at 25 °C) and a notable Li+ transference number (0.72). Similarly, Yu et al.89 adopted in situ UV curing to develop a self-standing cross-linked cellulose-based solid electrolyte, in which the cross-linked structure contributes to the integrity of the SPE during its use, while polar functional groups (–OH, –OCH3 and C–O–C) facilitate Li-salt dissociation and Li+ migration. As such, this solid electrolyte affords an increased Li+ transference number up to 0.9. When paired with the LiFePO4 cathode, the constructed full cell exhibited an initial discharge capacity of 150.6 mA h g−1. Moreover, owing to the stable passivation layer and good electrode/electrolyte interface compatibility, a high-capacity retention of 91.2% after cycles was achieved. In addition to UV irradiation, in situ thermal polymerization can also be applied to construct a h-BN-reinforced cellulosic solid electrolyte (Fig. 5c),90 where the h-BN filler can interact with not only the cellulose molecular chain but also TFSI− anions. This led to the improved ionic transport (8.9 × 10−3 S cm−1 at 30 °C) and a wide electrochemical stability window (up to 5.5 V). Due to the compatible interface between the electrodes and electrolyte, the solid LiCoO2//Li battery can stably cycle over 200 times, along with 99.8% coulombic efficiency during the whole cycling.
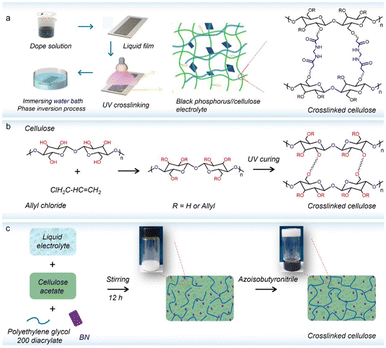 |
| Fig. 5 (a) In situ polymerization for the preparation of the cross-linked cellulose membrane (copyright 2022 Elsevier B.V.);88 (b) the cross-linking process of the cellulose-based electrolyte (copyright 2020 Elsevier B.V.);89 (c) the preparation process of the cellulose-based composite electrolyte (copyright 2021 Elsevier B.V.).90 | |
In situ chemical polymerization is regarded as a robust fabrication tool in the lab-scale for solid electrolyte fabrication, which endows the solid electrolyte with an uninterrupted Li+ transport channel and benign interface contact with the filler or electrode. Yet, their further advancement is plagued by the monomer selection, and the unreacted small molecules could be detrimental to mechanical strength.91
Taken together, we have summarized the ionic conductivity and electrochemical stability window of cellulose-based solid electrolytes prepared by various methods. As displayed in Table 1, the obtained cellulose-based solid electrolytes can deliver a desirable ionic conductivity and a wide electrochemical stability window.
Table 1 The ionic conductivity and electrochemical stability window of cellulose-based solid electrolytes prepared by various methods
Preparation methods |
Solid-state electrolytes |
Ionic conductivity (S cm−1) |
Temp. (°C) |
Stable window (V) |
Ref. |
Solution casting |
Brush-like cellulose-based electrolyte |
8.0 × 10−5 |
30 |
4.9 |
67 |
Methyl cellulose-based electrolyte |
2.0 × 10−4 |
25 |
4.8 |
68 |
Methyl cellulose-based electrolyte |
7.0 × 10−4 |
25 |
4.8 |
69 |
Phase inversion |
Porous cellulose-based electrolyte |
4.8 × 10−4 |
25 |
4.8 |
73 |
Porous ethyl cellulose/PVDF-based electrolyte |
6.9 × 10−4 |
25 |
5.3 |
78 |
Porous cellulose acetate-based electrolyte |
4.7 × 10−4 |
25 |
5.0 |
74 |
Electrostatic spinning |
Cellulose acetate/PVDF-based electrolyte |
2.6 × 10−3 |
25 |
4.5 |
81 |
Cellulose acetate-based electrolyte |
4.7 × 10−4 |
25 |
5.0 |
82 |
Cellulose acetate/poly-L-lactic/halloysite-based electrolyte |
1.5 × 10−3 |
25 |
5.2 |
84 |
In situ polymerization |
Porous cellulose/black phosphorous-based electrolyte |
5.2 × 10−3 |
25 |
5.2 |
88 |
Allyl-modified cellulose-based electrolyte |
4.4 × 10−3 |
25 |
4.8 |
89 |
Cellulose acetate/PEG/BN-based electrolyte |
8.9 × 10−3 |
30 |
5.5 |
90 |
3 Solid electrolytes based on modified celluloses
Cellulose is the most abundant natural polymer, and its molecular chains mainly consist of thousands of linked anhydrous glucose units that are rich in oxygen-containing polar chemical groups (such as –OH, –O–), which can solvate Li+ and favour Li+ movement, showing its potential as a solid electrolyte.92 However, the dense polymeric chains inevitably hinder Li+ migration. Modifying cellulose has become a feasible approach to mitigate these problems.
3.1 Coordination modification
Recently, Hu et al.93 discovered that Cu2+ coordination could open the molecular channels within cellulose (Fig. 6a and b), thus realizing rapid Li+ transport via a typical ion-hopping mechanism. Based on this, a Cu2+-coordinated cellulose ion conductor was constructed (Fig. 6c), which not only delivered a high ionic conductivity (1.5 × 10−3 S cm−1 at 25 °C), but also yielded a large transference number (0.78) and a good interfacial stability (4.5 V). Alternatively, by using Cu2+ as the metal node, an ion-conducting cellulose-derived supramolecule framework was also developed (Fig. 6d), in which the cellulose polymeric chains coordinate with Cu2+ with the assistance of Na+ and OH− to form a unique crystalline structure.94 Such a cellulose-based scaffold exhibited a high RT ionic conductivity of 0.23 S cm−1. It is suggested that improved Li+ conduction comes from the angstrom-scale open channels (∼10 Å in diameter). This molecular-channel engineering approach assisted by cation-coordination chemistry can be expanded to other polymers and cations for more high-performance electrolytes. On the other hand, the ionic conductivity of solid polymer electrolytes at low temperature is also crucial. In this regard, Wang et al.95 proposed a strong Al–O–C coordination to design cellulose-based electrolytes by incorporating natural bentonite (Fig. 6e). Due to the coordination interaction, two bentonite nanoplatelets were separated by cellulose to form interstitial spaces, which could serve as a straight pathway for ion migration (Fig. 6f). Thus, the acquired solid electrolyte can deliver an outstanding ionic conductivity (89.9 mS cm−1 at 25 °C) and good compatibility with Li anodes. More impressively, the ionic conductivity at −20 °C was still as high as 25.8 mS cm−1.
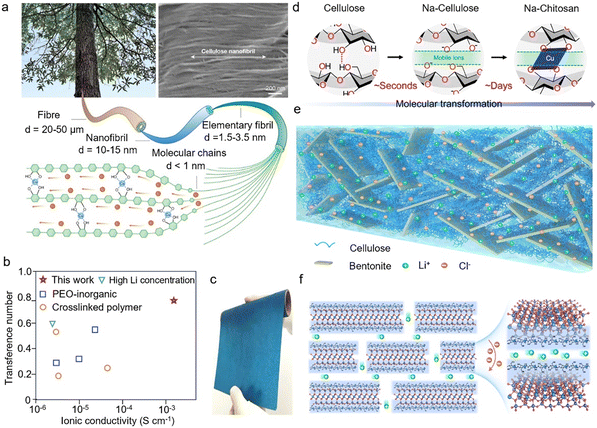 |
| Fig. 6 (a) Schematic diagram of cellulose sources, mechanism of Cu2+ ion interaction with cellulose hydroxyl groups and SEM image of nanocellulose (copyright 2021 Springer Nature);93 (b) comparison of Li-ion transference number and ionic conductivity of Cu2+-coordinated cellulose with other materials (copyright 2021 Springer Nature);93 (c) optical image of the Cu2+-coordinated cellulose membrane (copyright 2021 Springer Nature);93 (d) schematic showing the Cu2+-coordination at the molecular level (copyright 2022 American Association for the Advancement of Science);94 (e) schematic structure of the cellulose/bentonite composite (copyright 2022 Nature Publishing Group);95 (f) ions moving on the layered bentonite nanoplatelets with cellulose layers (copyright 2022 Nature Publishing Group).95 | |
3.2 Chemical grafting
Recent studies have proved that grafting task-specific functional segments on cellulose can endow cellulose-derived solid electrolytes with high ionic conductivity.96,97 To this end, CH3COO− groups were grafted on cellulose chains to break the strong hydrogen bond interactions and provide high-speed Li+ transport pathways (Fig. 7a).96 The prepared cellulose-based electrolyte supplied a high ionic conductivity of 1.1 × 10−3 S cm−1 and a large Li+ transference number of 0.85 at 30 °C (Fig. 7b), which enables highly stable Li stripping/plating cycling for over 1800 h at 0.1 mA cm−2, indicating a more stable and compatible interface between the designed CSPEs and Li metal anodes. Alternatively, ion-conducting segments were covalently grafted onto cellulose through atom transfer radical polymerization, leading to a brush-like cellulose polymer electrolyte with good ionic conductivity.67 Such brush-like structure not only helps improve mechanical strength, but also facilitates the formation of more ion channels. On the other hand, the relatively rigid nature of cellulose and possible side reactions between aliphatic hydroxyl groups and Li anodes could result in terrible interfacial compatibility. Given that polyethylene glycol (PEG) has typically interfacial stability with Li metal, it was found that grafting cellulose with low-molecular-weight PEG can realize a wide electrochemical stable window of 4.8 V (Fig. 7c and d).97 The above facts indicate that cellulose can provide a promising platform to graft other functional groups in one cellulose material for high-performance solid polymer electrolytes and beyond.
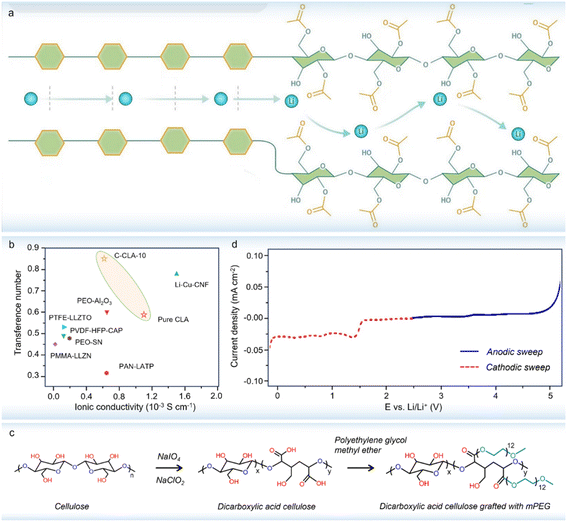 |
| Fig. 7 (a) The CH3COO− structures inserted into cellulose molecule chains open Li+ transport channels to improve the ionic conductivity (copyright 2023 Wiley-VCH);96 (b) comparison of ionic conductivity and Li+ transference number of the CH3COO−-grafted cellulose-based electrolyte with other materials (copyright 2023 Wiley-VCH);96 (c) cellulose synthesis reaction grafted with PEG (copyright 2020 Elsevier B.V.);97 (d) linear sweep voltammetry curve of the PEG-grafted cellulose-based electrolyte (copyright 2020 Elsevier B.V.).97 | |
3.3 Cross-linking
Besides the above modified strategies, cross-linking can also design a cellulose-based solid electrolyte with high performance, achieved by physical blending, chemical covalent bonding or strong non-covalent interactions.98 For instance, by physically cross-linking methyl 2-hydroxyethyl cellulose and poly(ethylene oxide) (Fig. 8a),99 the interaction network can provide multidimensional Li+ transport pathways and substantial polar groups can effectively attract more TFSI−, affording a high ionic conductivity (1.3 × 10−4 S cm−1 at 30 °C) and a broad electrochemical stable window (5.56 V). Importantly, this solid electrolyte also showed good interface compatibility with Li anodes, enabling a reversible Li plating/stripping cycling for over 1000 cycles. In addition to physical interactions, another solid electrolyte was designed through covalently crosslinking allyl cellulose and PEG (polyethylene glycol) (Fig. 8b). With an appropriate degree of PEG functionality, the cellulose-based electrolyte can provide an ionic conductivity of 1.82 × 10−3 S cm−1 at 25 °C and a Li+ transference number of 0.81.100 Due to the superior interfacial stability, as-assembled solid Li//Li cells can suppress the growth of lithium dendrites even after 1000 h of long-term cycling.
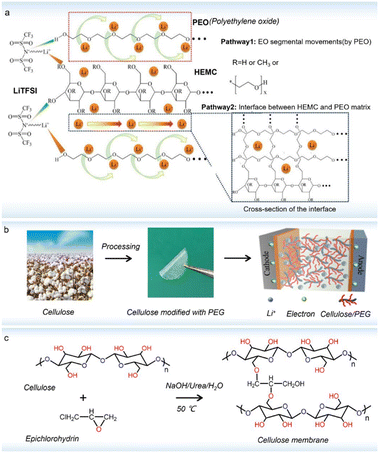 |
| Fig. 8 (a) Schematic illustration of possible Li+ transport pathways and mechanism in the cellulose//polyethylene oxide (PEO) electrolyte (copyright 2019 The Royal Society of Chemistry);99 (b) processing from cotton to cellulose and then modified with PEG (copyright 2022 Elsevier B.V.);100 (c) the cross-linking process of cellulose with epichlorohydrin (copyright 2019 Elsevier B.V.).101 | |
Besides this, the introduction of small molecules as cross-linkers was also used to bridge the cellulose polymeric chains (Fig. 8c), resulting in a high-performance solid membrane with a good tensile fracture strength of 14.6 MPa. Such a bridge effect can endow the solid electrolyte with a 3D porous network structure that provides many channels for Li+ transport, while the –OH groups on cellulose chains can interact with the anion part of Li-salts to promote Li+ movement. Thus, the prepared solid electrolyte yielded a superior RT ionic conductivity (6.34 × 10−3 S cm−1), a high Li+ transference number (0.82), and an excellent interfacial compatibility (4.6 V).101
In summary, a certain cross-linking can endow the solid polymer electrolyte with a 3D interpenetrated network, which, upon further coupling with high-density polar groups, can favor rapid Li+ conduction for efficient solid electrolytes.102
4 Solid electrolytes based on cellulose-derived composites
Generally, it is believed that incorporating an inorganic material into the polymer host can improve the ionic conductivity, enhance the mechanical properties, broaden the electrochemical window, and lower the interfacial resistance of SPEs.103 Particularly, inorganics can induce surface interactions with polymer segments and then decrease the crystallization tendency of the polymer.104 Moreover, their surface functional groups facilitate Li-salt dissociation due to Lewis acid–basic interaction, thus increasing the ionic conductivity. Given the contribution to Li+ migration, currently-reported inorganics in SPEs can be divided into inert fillers and active fillers. It should be noted that active inorganics are ion conductors and can partake in ion movement in solid electrolytes, while inert fillers indirectly influence the ionic conductivity of SPEs via the interaction effects as described below.105
On the other hand, another possible avenue in achieving a high ionic conductivity is the addition of organic small molecules (such as ionic liquids and plasticizers) and other polymers.106 For ionic liquids, their addition can directly improve the ionic conductivity of solid polymer electrolytes. The incorporation of plasticizers into polymer electrolytes can increase the Li-salt solvating power, free volume and the level of flexibility, thus enabling a high ionic conduction and good electrochemical stability. As for organic polymers, the addition always shows good phase compatibility while largely increasing the amorphous region, which coupled with rich polar groups favors the dissociation of ion pairs and Li-ion movement.
4.1 Composited with inorganic active fillers
There are three kinds of Li-ion transfer pathways in inorganic active filler-doped solid polymer electrolytes: (1) Li ions pass through the active inorganics; (2) Li ions transport through the polymer phase; (3) Li ions transport along the polymer–ceramic interface.107 Among various active fillers, metal oxides (including garnet-type, perovskite-type, and NASICON-type) and sulfides are widely adopted.108
Garnet-type Li-ion conductors have a general chemical formula of A3B2(MO4)3, where A, B, and M denote eight-, six-, and four-coordinated cation sites, respectively. Importantly, garnet-type solid electrolytes typically show high ionic conductivity, a large Li+ transference number, a wide electrochemical stability window and good stability against Li anodes.109 The addition of garnet-type inorganic fillers can improve the physicochemical properties of solid polymer electrolytes.110 Recently, a robust nacre-like cellulose-based electrolyte has been designed via compositing bacterial cellulose with Li6.4La3Zr1.4Ta0.6O12 (LLZTO) particles (Fig. 9a).111 Benefiting from the decoupled cellulosic segment and additional Li+ transport pathways provided by LLZTO, the as-prepared solid electrolyte at 25 °C showed a high ionic conductivity (1.68 × 10−3 S cm−1), a remarkable Li+ transfer number (0.92) and a wide electrochemically stable window (5.3 V). By using this solid electrolyte, the Li//Li cells demonstrated a stable plating/stripping process for 870 h, indicating a stable interface that inhibits dendrite growth. Further, the assembled solid Li metal battery with an N/P ratio of 0.74 delivered a high areal capacity of 4.2 mA h cm−2.
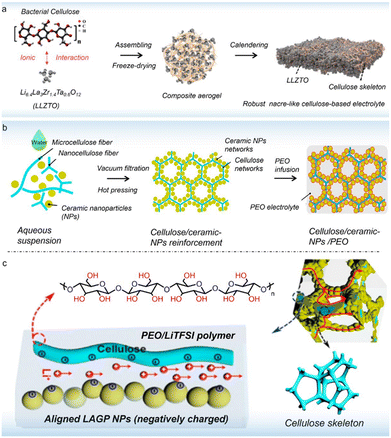 |
| Fig. 9 (a) Schematic illustration of the fabrication of the multifunctional cellulose/LLZTO solid composite electrolyte (copyright 2022 Elsevier B.V.);111 (b) schematic illustration of fabrication processes for the cellulose/ceramic/PEO solid electrolyte (copyright 2020 American Chemical Society);112 (c) schematic illustration of the proposed Li+ conducting mechanism in the cellulose/ceramic/PEO solid electrolyte (copyright 2020 American Chemical Society).112 | |
NASICON-type ceramics with a composition of LiM2(PO4)3 (M = Ge, Ti, Zr) were firstly reported in 1989, in which the MO6 octahedron and the XO4 tetrahedron are connected at a common angle to form Li+ transfer channels. It has been shown that NASICON-type filler introduction can improve the ionic conduction of polymer-based electrolytes. For example, an ionically conductive and mechanically robust composite polymer electrolyte with 3D cellulose/Li1.5Al0.5Ge1.5(PO4)3 (LAGP)/PEO networks has been explored (Fig. 9b).112 As depicted in Fig. 9c, the designed electrolyte with LAGP ceramics can offer 3D continuous Li+ pathways along the polymer–ceramic interface. Moreover, the abundant –OH groups on cellulose afford a negatively charged surface, which facilitates Li+ migration by surface-charge-governed ion transport. As a result, the cellulose-based solid electrolyte displayed an ionic conductivity of 1.1 × 10−4 S cm−1 at 60 °C, and exhibited a low interfacial impedance in both solid-state Li//Li and LiFePO4//Li cells.
Perovskite-type Li-ion conductors (such as Li3xLa2/3−xTiO3, LLTO, 0 < x < 0.16) have a cubic structure with space groups of P4/mmm and
mmm, and are well-known for their high RT conductivity in the magnitude of 10−3 S cm−1 and excellent stability at high voltages. Recently, Lu et al.113 composited perovskite-type LLTO and 2 wt% bacterial cellulose into a solid-state electrolyte. The presence of cellulose can reduce the grain-boundary thickness and crystalline phase of LLTO, which in turn improves the overall ionic conductivity by facilitating Li ion migration across the grain boundaries. Beyond those, sulfide-type electrolytes always have an extremely-high ion-conductivity of 10−2 S cm−1 at 25 °C, which can serve as functional fillers to regulate Li+ conduction in cellulose-based electrolytes, but have not been explored.
4.2 Composited with inorganic inert fillers
In addition to inorganic active fillers, inert fillers such as Al2O3, SiO2, TiO2 and MgO were also found to be effective in enhancing mechanical strength, decreasing polymeric crystallinity, and improving Li+ conductivity of solid polymer electrolytes.114 Among them, Al2O3 is widely used in the preparation of solid composite electrolytes.115 For example, an Al2O3-reinforced cellulose-based solid electrolyte has been designed via a facile UV curing process.116 In this case, the acidic Al2O3 particles with a large number of surface –OH groups can interact with cellulose polymeric chains and thereby favor the formation of Li+ transfer pathways at the Al2O3–polymer interface. Moreover, the Lewis-acid surface on Al2O3 can promote Li-salt dissociation through a series of “ion-ceramic pair” formation. These effects endow the solid electrolyte with a high ionic conductivity (2 × 10−4 S cm−1 at 25 °C and 10−3 S cm−1 at 80 °C). Likewise, an integrated cellulose-based electrolyte cross-linked with SiO2 nanofillers has also been explored,117 which showed a good RT ionic conductance (4.1 × 10−4 S cm−1), a desirable Li+ transfer number (0.54), and a superior interfacial stability (5.1 V). The LiFePO4//Li cell involving this solid electrolyte can stably run for 1000 cycles at 2C, accompanied with a stable coulombic efficiency exceeding 99.3%.
Apart from these oxides, other inert fillers (including MOFs, COFs, h-BN, C3N4, MoS2 and so on) can also be introduced into cellulose-based electrolytes for promoted electrochemical performance.119 For instance, Huang et al.118 have in situ grown nanosized ion-conductive MOF particles on a bacterial cellulose skeleton for constructing solid composite electrolytes (Fig. 10a). The designed MOFs with a high-density of electronegative groups (–SO3H) can serve as a continuous path for ion transport, while the bacterial cellulose network provided an additional linear Li+ transfer highway (Fig. 10b). As such, the as-garnered electrolyte exhibited a high ionic conductivity of 7.88 × 10−4 S cm−1 with a Li+ transference number up to 0.88 at 25 °C, a wide electrochemical window (up to 5.10 V), and excellent interface contact with electrodes. More impressively, the fabricated solid cell maintained a long-lived cycling performance for 600 loops at 3C, along with a decay rate of 0.02% per cycle. On the other hand, in situ thermal polymerization has been carried out to prepare a cellulose-based solid electrolyte with layered h-BN fillers.120 A tremendous amount of polar groups in cellulose chains can coordinate with Li+ and facilitate the transport of Li+, while the Lewis acid–base properties of h-BN fillers can weaken ion pairing and induce interaction with polymer segments, advantageous to dissociating Li-salts and delaying the high-voltage decomposition of the polymer electrolyte, respectively. Therefore, the obtained solid electrolyte presented a high ionic conductivity of 8.9 × 10−3 S cm−1 at 60 °C and a wide oxidative stability window of up to 5.5 V.
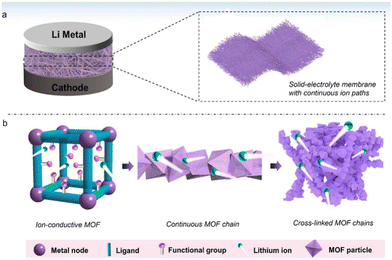 |
| Fig. 10 (a) Schematic illustration of the solid electrolyte of the cross-linked MOF grown on bacterial cellulose;118 (b) bottom-up synthesis of cross-linked MOF chains on a bacterial cellulose skeleton. (copyright 2021 American Chemical Society).118 | |
4.3 Composited with organic small molecules
Relative to inorganic fillers, organic small molecules including ionic liquids and plasticizers can induce the plasticizing effect in solid polymer electrolytes, which has been proven to augment the mobility of Li+. In general, ionic liquids have a strong ability to dissolve Li salts, high Li+ conduction and high electrochemical stability, which thus play a promoting role in high-performance solid electrolytes.121 In this regard, Gosavi et al.122 showed an ionically conductive, thermally stable and high-voltage cellulose-based solid electrolyte involving an ionic liquid (Fig. 11a and b). Here, a unique ionic interaction induced by cellulose molecular chains and the ionic liquid can assist Li ion mobility from one site to another, resulting in a high ionic conductivity (5.24 × 10−3 S cm−1 at 25 °C). In addition, a small molecule (CH3–O–(CH2–CH2–O)4–CH3, named as G4) reported by Chinnam et al.123 can interact with Li-salts to form [G4Li]+[TFSI]−, which acts as a novel ionic liquid to assist in realizing a self-standing, highly ionic conductive solid cellulose-based electrolyte (Fig. 11c). The resultant solid electrolyte can deliver a large RT conductivity (>10−4 S cm−1), good thermal stability (200 °C), and stable interfacial contacts (5.0 V). With this solid electrolyte, the plating/stripping process at low current densities for Li//Li cells is quite stable, and the solid LiFePO4//Li cells can stably cycle over 100 cycles with 99% coulombic efficiency (Fig. 11d).
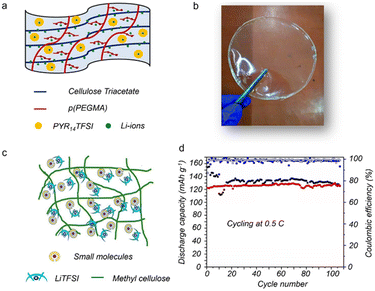 |
| Fig. 11 (a) Available sites for Li coordination and (b) photograph of the cellulose-based solid electrolyte with an ionic liquid (PYR14TFSI) (copyright 2021 American Chemical Society);122 (c) the methyl cellulose-based electrolyte with small molecules (copyright 2020 American Chemical Society);123 (d) cycling performance at 0.5C of the solid LiFePO4//Li cell (copyright 2020 American Chemical Society).123 | |
Regarding plastic crystals with excellent thermal stability, high dielectric constant, and solvation capabilities, succinonitrile molecules were adopted to reinforce the cellulose-based electrolyte,124 which showed a good ionic conductivity of 2.1 × 10−4 S cm−1 at 25 °C and a tensile strength of over 0.5 MPa. In addition, the practical use of the as-acquired electrolyte can hinder dendrite growth and suppress adverse reactions by stabilizing the Li metal interface, endowing the solid cells with a high initial capacity and long-term cyclability. To date, there are still few studies related to the construction of cellulose-based electrolytes using plastic crystals as plasticizers, which will be a future research direction.
4.4 Composited with organic polymers
Apart from organic small molecules, traditional polar polymers such as poly(ether ethylene oxide) (PEO), poly(vinylidene fluoride) (PVDF), poly(vinylidene fluoride–hexafluoropropylene) (PVDF–HFP), and poly(ethylene glycol) methyl ether methacrylate (PEGMA) can be composited with cellulose to design state-of-the-art solid electrolytes.125 Here, we briefly summarize the recent developments in these composite solid electrolytes.
PEO-based solid electrolytes have attracted extensive interest in the past decades due to their multiple merits such as easy processing, great flexibility and favorable interfacial compatibility.126 However, PEO-based solid electrolytes generally suffer from poor mechanical strength, inferior ionic conductivity, narrow electrochemical window and small Li+ transfer number, greatly limiting their practical utilization in solid state batteries. It has been proven that a trade-off between ionic conductivity and mechanical strength can be realized by directly compositing PEO with cellulose (Fig. 12a).127 In this composite electrolyte, PEO interacts with bacterial cellulose through H-bonding, which would suppress the bending of PEO chains around Li atoms and weaken the Li-binding strength, greatly reducing the transfer resistance for Li leaving the PEO binding sites, and thus improving the Li+ conductivity. The composite electrolyte exhibited higher tensile strength (4.43 MPa) than the pure PEO-based electrolyte (1.34 MPa). Thus, good interface compatibility and effective dendrite inhibition are achieved, which leads to a long cycling life of 1160 h in the Li//Li symmetrical cell. Furthermore, the assembled solid-state Li metal battery can reflect a reversible specific capacity of 149.8 mA h g−1.
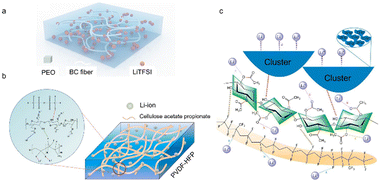 |
| Fig. 12 (a) Schematic diagram of the bacterial cellulose–PEO composite solid polymer electrolyte (copyright 2020 Wiley-VCH);127 schematic illustration of (b) the PVDF–HFP/cellulose acetate propionate solid electrolyte (copyright 2022 Springer)128 and (c) the possible Li-ion transport mechanism (copyright 2023 springer).129 | |
PVDF or its derivative-based polymer electrolytes have a satisfactory dielectric constant, good electrochemical stability and sufficient mechanical strength. Yet, these solid electrolytes in general suffer from a low ionic conductivity derived from their high glass transition temperature. Compositing PVDF-based materials with other polymers can significantly reduce their crystalline region without obvious phase separation, thus increasing the ionic conductivity.130 Kong et al.128 fabricated a novel polymer electrolyte consisting of cellulose acetate propionate, PVDF–HFP and Li-salts by a facile blending process (Fig. 12b). Note that the cellulose species in the obtained electrolyte played crucial roles in decreasing the PVDF–HFP crystalline phase, promoting LiTFSI dissociation, and providing migration pathways for Li+, giving rise to an ionic conductivity of 1.25 × 10−4 S cm−1 at 30 °C. Moreover, this composite electrolyte demonstrated high mechanical strength and interface stability with Li metal anodes, and accordingly the assembled LiFePO4//Li cell displayed excellent cycling stability and good rate capability. Similarly, Liu et al.129 designed a composite solid polymer electrolyte using cellulose acetate, PVDF–HFP and LiTFSI, from which the O atoms in cellulose acetate can not only promote the Li-salt dissociation but also interact with F atoms in TFSI anions, producing a more orderly arrangement of ionic clusters conducive to Li-ion transport, as observed in Fig. 12c. Therefore, a RT ionic conductivity of 4.33 × 10−4 S cm−1, a Li-ion transfer number of 0.60 and a wide electrochemical window of 4.8 V were achieved. Moreover, the stable interface between the composite electrolyte and electrodes endowed the solid battery with good cyclability.
PEGMA has a structure similar to poly(ethylene glycol), but with ether groups and polymerizable acrylic ester end groups, which can dissolve and capture solvent molecules in polymer electrolytes. The combination of cellulose and PEGMA allows for greater availability of ether and ester functional groups, where the oxygen atoms form temporary ionic interactions with Li+ and help transport them via a hopping mechanism, which ultimately improves ionic conductivity.131 Accordingly, a self-standing solid polymer electrolyte based on cellulose triacetate and PEGMA has been developed by Kale et al.132 Due to the existence of polar functional groups containing C
O, Li ions can move along the cellulose polymer chain through coordination effects. In addition, PEGMA together with cellulose triacetate can produce a polymer network that supplies coordination sites and free space for Li+ transportation. Thus, this composite electrolyte affords a high ionic conduction of 1.8 × 10−3 S cm−1 at 25 °C and a large transfer number of 0.7. Meanwhile, the interfacial compatibility between this solid electrolyte and Li anode could markedly improve the cycling performance of both Li//Li cells and LiFePO4//Li cells.
5 Conclusion and perspective
Cellulose and its derivatives have attracted significant interest owing to their multiple merits of raw material reserve, high strength, abundant polar groups and electrochemical stability. In the past decade, great efforts have devoted to developing high-performance CSPEs capable of suppressing the uneven growth of Li. Herein, we have comprehensively reviewed the recent advances in CSPEs from the perspectives of common preparation methods and diverse modification and compositing strategies for improving their ionic conductivity. Despite some progress, it is still a huge challenge to achieve CSPEs with sufficient advantages for practical uses. In this regard, the discussion of current challenges and future research directions of cellulose-based solid electrolytes is provided as below.
(1) Cellulose is abundantly available from various natural sources, but very hard to further process owing to its typical amphiphilicity, which engenders a poor solubility in most solvents, largely limiting its broad application. Current cellulose solvents mainly include NaOH/urea solutions or ionic liquids, which suffer from unwanted residues and high costs, respectively. Thus, further efforts are needed to develop new types of green solvents that can dissolve cellulose. Additionally, modifying cellulose through etherification, esterification, or grafting target segments is another feasible approach to solve the insolubility problem. In general, the excellent properties of cellulose-based materials mainly depend on their functional groups. Moreover, the functionalization efficiency of these cellulose-derived materials is largely influenced by modification conditions (e.g. reaction temperature and time). Thus, it is highly desirable to conduct research on functional efficiency, which will bring about in-depth cognition of cellulose-based polymers.
(2) Although the abundant –OH groups in cellulose facilitate Li-salt dissociation, their packed polymer chains of individual cellulose could result in rather low RT ionic conduction. By desirable modification, the intermolecular H-bonds are broken. This endows the composite electrolytes with Li+ conductivity up to 10−3 or 10−4 S cm−1, but it still cannot be comparable to organic liquid electrolytes (10−2 S cm−1). Particularly after adding nanofillers into cellulose, the inevitable phase separation can result in poor conduction of Li-ions. Additionally, the size, addition amount, degree of dispersion, and structure of nanofillers can also influence the ionic conductivity and mechanical properties of cellulose-based composite electrolytes. Yet, in-depth investigation on the underlying mechanism is barely reported so far.
(3) Another main challenge regarding the use of cellulose-based electrolytes lies in how to control materials' porosity (especially pore size and distribution) and thickness. Thinner solid cellulose-based electrolytes are conducive to the increase in battery energy density, while mechanically strong and flexible properties are also crucial to the suppression of Li dendrites. Thus, it is highly required to break the above trade-off for achieving thin and mechanically strong solid electrolytes.
Data availability
The data that support the findings of this study are available from the corresponding author upon reasonable request.
Conflicts of interest
There are no conflicts to declare.
Acknowledgements
We gratefully acknowledge financial support from industrial: Guangzhou Lushan New Materials Co. Ltd. (No. HT-99982022-0391). The authors also appreciate financial support from Guangdong Basic and Applied Basic Research Foundation (No. 2020B1515420001 and 2023B1515040027) and Fundamental Research Funds for the Central Universities, Sun Yat-sen University (No. 23yxqntd002).
References
- A. Majed, M. Torkamanzadeh, C. Nwaokorie, K. Eisawi, C. Dun, A. Buck, J. Urban, M. Montemore, V. Presser and M. Naguib, Toward MBenes battery electrode materials: Layered molybdenum borides for Li-ion batteries, Small Methods, 2023, 7, 2300193 CrossRef CAS PubMed.
- Y. Zhang, S. Li, J. Shi, J. Lai, Z. Zhuang, J. Liu, W. Yang, L. Ma, Y. Cai, J. Xu and Q. Zheng, Revealing the key role of non-solvating diluents for fast-charging and low temperature Li-ion batteries, J. Energy Chem., 2024, 94, 171–180 CrossRef CAS.
- R. Pandya, A. Mathieson, B. D. Boruah, H. B. de Aguiar and M. de Volder, Interrogating the light-induced charging mechanism in Li-ion batteries using operando optical microscopy, Nano Lett., 2023, 23, 7288–7296 CrossRef CAS PubMed.
- L. Wang, G. Rahamim, K. Vudutta, N. Leifer, R. Elazari, I. Behar, M. Noked and D. Zitoun, Influence of the halogen in argyrodite electrolytes on the electrochemical performance of all-solid-state lithium batteries, Energy Technol., 2023, 11, 2201116 CrossRef CAS.
- J. Xu, T. P. Pollard, C. Yang, N. Dandu, S. Tan, J. Zhou, J. Wang, X. He, X. Zhang, A. Li, E. Hu, X. Yang, A. Ngo, O. Borodin and C. Wang, Lithium halide cathodes for Li metal batteries, Joule, 2023, 7, 83–94 CrossRef CAS.
- S. Yuan, K. Ding, X. Zeng, D. Bin, Y. Zhang, P. Dong and Y. Wang, Advanced nonflammable organic electrolyte promises safer Li-metal batteries: From solvation structure perspectives, Adv. Mater., 2023, 35, 2206228 CrossRef CAS PubMed.
- A. L. Yang, C. Yang, K. Xie, S. Xin, Z. Xiong, K. Y. Li, Y. G. Guo and Y. You, Benchmarking the safety performance of organic electrolytes for rechargeable lithium batteries: A thermochemical perspective, ACS Energy Lett., 2023, 8, 836–843 CrossRef CAS.
- S. Li, Q. Tian, J. Chen, Y. Chen, P. Guo, C. Wei, P. Cui, J. Jiang, X. Li and Q. Xu, An intrinsically non-flammable organic electrolyte for wide temperature range supercapacitors, Chem. Eng. J., 2023, 457, 141265 CrossRef CAS.
- W. Jiang, S. Wang, Y. Wang, J. Hu, D. Yang and W. Zhang, Silica-modified 3D porous copper current collectors toward stable lithium metal anodes, Energy Technol., 2023, 11, 2300053 CrossRef CAS.
- B. Acebedo, M. C. Morant-Miñana, E. Gonzalo, I. Ruiz de Larramendi, A. Villaverde, J. Rikarte and L. Fallarino, Current status and future perspective on lithium metal anode production methods, Adv. Energy Mater., 2023, 13, 2203744 CrossRef CAS.
- W. Zhao, K. Zhang, F. Wu, X. Wang, R. Guo, K. Zhang, Y. Yuan, Y. Bai and C. Wu, Moisture-assistant chlorinated separator with dual-protective interface for ultralong-life and high-rate lithium metal batteries, Chem. Eng. J., 2023, 453, 139348 CrossRef CAS.
- M. Zhu, X. Zhao, R. Yan and J. Zhang, Recent research progress of alloy-containing lithium anodes in lithium-metal batteries, Curr. Opin. Solid State Mater. Sci., 2023, 27, 101079 CrossRef CAS.
- W. Ren, K. Zhu, W. Zhang, H. Liang, L. Xu, L. Wang, C. Yang, Y. Yang, P. Zhang, F. Wang, Y. Wang and W. Li, Dendrite-free lithium metal battery enabled by dendritic mesoporous silica coated separator, Adv. Funct. Mater., 2023, 33, 2301586 CrossRef CAS.
- H. Ma, J. Yu, M. Chen, X. Han, J. Chen, B. Liu and S. Shi, Amino-enabled desolvation sieving effect realizes dendrite-inhibiting thin separator for durable aqueous zinc-ion batteries, Adv. Funct. Mater., 2023, 33, 2307384 CrossRef CAS.
- Z. Li, N. Hu and L. Fan, Nanocomposite phase change materials for high-performance thermal energy storage: A critical review, Energy Storage Mater., 2023, 55, 727–753 CrossRef.
- W. Jiang, G. Qiu, D. Wu, J. Zhang, L. Huang, Z. Yue, Y. Dai and F. Sun, Superlithiophilic Ag particles embedded in flexible polymer solid electrolyte interface films for dendrite-free lithium metal anodes, ACS Sustainable Chem. Eng., 2023, 11, 13508–13518 CrossRef CAS.
- A. J. Sanchez and N. P. Dasgupta, Lithium metal anodes: Advancing our mechanistic understanding of cycling phenomena in liquid and solid electrolytes, J. Am. Chem. Soc., 2024, 146, 4282–4300 CrossRef CAS PubMed.
- M. Yang, S. Wang, J. Fu, Y. Zhu, J. Liang, S. Cheng, S. Hu, J. Hu, J. He and Q. Li, Quantum size effect to induce colossal high-temperature energy storage density and efficiency in polymer/inorganic cluster composites, Adv. Mater., 2023, 35, 2301936 CrossRef CAS PubMed.
- M. Yang, F. Yuan, W. Shi, W. Ren, M. Guo, C. Ouyang, L. Zhou, N. Sun, Y. Xiao, E. Xu, X. Zhang, Y. Wei, X. Deng, C. Nan, X. Wang and Y. Shen, Sub-nanowires boost superior capacitive energy storage performance of polymer composites at high temperatures, Adv. Funct. Mater., 2023, 33, 2214100 CrossRef CAS.
- M. A. K. Y. Shah, Y. Lu, N. Mushtaq, M. Alomar, M. Yousaf, N. Akbar, N. Arshad, M. S. Irshad and B. Zhu, Showcasing the potential of iron-doped electrolytes to enhance the ionic conduction for a low-temperature ceramics fuel cell, ACS Appl. Energy Mater., 2023, 6, 10829–10841 CrossRef CAS.
- N. Sarfraz, N. Kanwal, M. Ali, K. Ali, A. Hasnain, M. Ashraf, M. Ayaz, J. Ifthikar, S. Ali, A. Hendi, N. Baig, M. Ehsan, S. Shah, R. Khan and I. Khan, Materials advancements in solid-state inorganic electrolytes for highly anticipated all solid Li-ion batteries, Energy Storage Mater., 2024, 103619 CrossRef.
- Y. Nikodimos, W. Su and B. Hwang, Halide solid-state electrolytes: Stability and application for high voltage all-solid-state Li batteries, Adv. Energy Mater., 2022, 13, 2202854 CrossRef.
- H. Liu, Y. Liang, C. Wang, D. Li, X. Yan, C. W. Nan and L. Z. Fan, Priority and prospect of sulfide-based solid-electrolyte membrane, Adv. Mater., 2023, 35, 2206013 CrossRef CAS PubMed.
- G. Liu, J. Yang, J. Wu, Z. Peng and X. Yao, Inorganic sodium solid electrolytes: Structure design, interface engineering and application, Adv. Mater., 2024, 2311475 CrossRef PubMed.
- Y. Li, Z. Fu, S. Lu, X. Sun, X. Zhang and L. Weng, Polymer nanofibers framework composite solid electrolyte with lithium dendrite suppression for long life all-solid-state lithium metal battery, Chem. Eng. J., 2022, 440, 2310476 Search PubMed.
- P. Jiang, G. Du, J. Cao, X. Zhang, C. Zou, Y. Liu and X. Lu, Solid-state Li ion batteries with oxide solid electrolytes: Progress and perspective, Energy Technol., 2022, 11, 2201288 CrossRef.
- P. Wang, J. Liu, W. Cui, X. Li, Z. Li, Y. Wan, J. Zhang and Y.-Z. Long, Electrospinning techniques for inorganic-organic composite electrolytes of all-solid-state lithium metal batteries: A brief review, J. Mater. Chem. A, 2023, 11, 16539–16558 RSC.
- Y. Liao, X. Xu, X. Luo, S. Ji, J. Zhao, J. Liu and Y. Huo, Recent progress in flame-retardant polymer electrolytes for solid-state lithium metal batteries, Batteries, 2023, 9, 439 CrossRef CAS.
- S. Cao, Q. Shen and L. Zhang, Dual-coordination induced poly(vinylidene fluoride)/Li6.4Ga0.2La3Zr2O12/succinonitrile composite solid electrolytes toward enhanced rate performance in all-solid-state lithium batteries, ACS Appl. Mater. Interfaces, 2023, 15, 37422–37432 CrossRef CAS PubMed.
- X. Fu, Y. Zhou, J. Huang, L. Feng, P. Yu, Q. Zhang, W. Yang and Y. Wang, Rethinking the electrode multiscale microstructures: A review on structuring strategies toward battery manufacturing genome, Adv. Energy Mater., 2023, 13, 2301385 CrossRef CAS.
- Y. Chao, Y. Han, Z. Chen, D. Chu, Q. Xu, G. Wallace and C. Wang, Multiscale structural design of 2D nanomaterials-based flexible electrodes for wearable energy storage applications, Adv. Sci., 2023, 11, 2305558 CrossRef PubMed.
- J. Chattopadhyay, T. Pathak and D. Santos, Applications of polymer electrolytes in lithium-ion batteries: A review, Polymer, 2023, 15, 3907 CAS.
- A. Mong, J. Shin, M. Lee and D. Kim, Accelerated single Li-ion transport in solid electrolytes for lithium-sulfur batteries: Poly(arylene ether sulfone) grafted with pyrrolidinium-terminated poly(ethylene glycol), Small, 2023, 2309162 Search PubMed.
- S. Huo, L. Sheng, W. Xue, L. Wang, H. Xu, H. Zhang and X. He, Challenges of polymer electrolyte with wide electrochemical window for high energy solid-state lithium batteries, InfoMat, 2023, 5, e12394 CrossRef CAS.
- Z.-Y. Li, Z. Li, J.-L. Fu and X. Guo, Sodium-ion conducting polymer electrolytes, Rare Met., 2022, 42, 1–16 Search PubMed.
- X. Liu, H. Jia and H. Li, Flame-retarding quasi-solid polymer electrolytes for high-safety lithium metal batteries, Energy Storage Mater., 2024, 67, 103263 CrossRef.
- X. Chen, X. Li, L. Luo, S. He, J. Chen, Y. Liu, H. Pan, Y. Song and R. Hu, Practical application of all-solid-state lithium batteries based on high-voltage cathodes: Challenges and progress, Adv. Energy Mater., 2023, 13, 2301230 CrossRef CAS.
- J. Kang, D. Y. Han, S. Kim, J. Ryu and S. Park, Multiscale polymeric materials for advanced lithium battery applications, Adv. Mater., 2022, 35, 2203194 CrossRef PubMed.
- M. Wang, H. Zhang, Y. Li, R. Liu and H. Yang, Accelerated ion transportation in liquid crystalline polymer networks for superior solid-state lithium metal batteries, Chem. Eng. J., 2023, 476, 146658 CrossRef CAS.
- D. Liu, Z. Lu, Z. Lin, C. Zhang, K. Dai and W. Wei, Organoboron and cyano-grafted solid polymer electrolytes boost the cyclability and safety of high-voltage lithium metal batteries, ACS Appl. Mater. Interfaces, 2023, 15, 21112–21122 CrossRef CAS PubMed.
- Z. Qiao, Z. Yang, M. Li, Y. Feng, X. Qu, H. Qu and X. Zhang, One-pot synthesis of quaternary pyridinium-type cationic-based porous organic frameworks: Enhanced poly(ethylene oxide) composite polymer electrolytes for all-solid-state Li-S batteries, ACS Appl. Energy Mater., 2023, 6, 12128–12137 CrossRef CAS.
- X. Wang, R. Kerr, F. Chen, N. Goujon, J. M. Pringle, D. Mecerreyes, M. Forsyth and P. C. Howlett, Toward high-energy-density lithium metal batteries: Opportunities and challenges for solid organic electrolytes, Adv. Mater., 2020, 32, 1905219 CrossRef CAS PubMed.
- N. L. Nxumalo and P. N. Mahlambi, Molecularly imprinted polymer-based adsorbents for the selective removal of pharmaceuticals from wastewater: Adsorption kinetics, isotherms, and thermodynamics studies, Ind. Eng. Chem. Res., 2023, 62, 16525–16544 CrossRef CAS.
- W. Li, Y. Wang, R. Liu, W. Chen, H. Zhang and Z. Zhang, Gel polymer-based composite solid-state electrolyte for long-cycle-life rechargeable Zinc-air batteries, ACS Sustainable Chem. Eng., 2023, 11, 3732–3739 CrossRef CAS.
- H. Zhang, X. Gan, Z. Song and J. Zhou, Amphoteric cellulose-based double-network hydrogel electrolyte toward ultra-stable Zn anode, Angew. Chem., 2023, 62, e202217833 CrossRef CAS PubMed.
- L. Yang, X. Zhao, W. Zhang, K. Ren, X. Luo, J. Cao, S. Zheng, W. Li and X. Wu, “Pore-Hopping” ion transport in cellulose-based separator towards high-performance sodium-ion batteries, Angew. Chem., 2023, 135, e202300258 CrossRef.
- Z. Zheng, S. Yan, Y. Zhang, X. Zhang, J. Zhou, J. Ye and Y. Zhu, 56 hydrogel membrane for the high-performance quasi-solid-state Zinc-ion batteries, Chem. Eng. J., 2023, 475, 146314 CrossRef CAS.
- H. Chen, Z. Wang, Y. Feng, S. Cai, H. Gao, Z. Wei and Y. Zhao, Cellulose-based separators for lithium batteries: Source, preparation and performance, Chem. Eng. J., 2023, 471, 144593 CrossRef CAS.
- X. Li, Y. Zhang, J. Chen, Y. Wang, Z. Cheng, X. Chen and M. Guo, A cellulose-based interpenetrating network hydrogel electrolyte for flexible solid-state supercapacitors, Cellulose, 2023, 30, 2399–2412 CrossRef CAS.
- S. Billakanti, A. K. Othayoth, H. Vignesh Babu, A. M. Shanmugharaj, P. Bantumelli and K. Muralidharan, 2D-channel-forming catechol-based polyphosphates as solid polymer electrolytes and their microstructure-assisted Li-ion conductivity, ACS Appl. Energy Mater., 2023, 6, 5290–5299 CrossRef CAS.
- S. Wu, W. Yang, Z. Liu, Y. Li, H. Fan, Y. Zhang and L. Zeng, Organic polymer coating induced multiple heteroatom-doped carbon framework confined Co1-xS@NPSC core-shell hexapod for advanced sodium/potassium ion batteries, J. Colloid Interface Sci., 2024, 660, 97–105 CrossRef CAS PubMed.
- S. Wu, Y. Li, L. Chen, Y. Zhang, L. Zeng and H. Fan, Hexapod cobalt phosphosulfide nanorods encapsulating into multiple hetero-atom doped carbon frameworks for advanced sodium/potassium ion battery anodes, Chin. Chem. Lett., 2024, 24, 109796 CrossRef.
- Y. Yuan, R. Li and S. Peng, Research progress on chemical modification of waste biomass cellulose to prepare heavy metal adsorbents, Polym. Bull., 2022, 80, 11671–11700 CrossRef.
- Y. Wu, H. Si, X. Yu, F. Fu, Z. Wang, J. Yao and X. Liu, Enhancing the solubility and antimicrobial activity of cellulose through esterification modification using amino acid hydrochlorides, Int. J. Biol. Macromol., 2023, 226, 793–802 CrossRef CAS PubMed.
- T. Zhang, X. Zhao, C. Zhang, Y. Zhang, Y. Zhang, Y. Feng, Q. Chi and Q. Chen, Polymer nanocomposites with excellent energy storage performances by utilizing the dielectric properties of inorganic fillers, Chem. Eng. J., 2021, 408, 127314 CrossRef CAS.
- Y. Gu, X. Zhao, K. Li, J. Cao, X. Wang, J. Guo, H. Liu, S. Zheng, D. Liu, H. Wu and X. Wu, Homeostatic solid solution reaction in phosphate cathode: breaking high-voltage barrier to achieve high energy density and long life of sodium-ion batteries, Adv. Mater., 2024, 2404532 CrossRef PubMed.
- X. Huang, L. Zhang, X. Zhao, Y. Heng, T. Wang, H. Geng and X. Wu, Hollow Na0.62K0.05Mn0.7Ni0.2Co0.1O2 polyhedra with exposed stable {001} facets and K riveting for sodium-ion batteries, Sci. China Mater., 2023, 66, 79–87 CrossRef.
- A. Curreri, S. Mitragotri and E. Tanner, Recent advances in ionic liquids in biomedicine, Adv. Sci., 2021, 8, 2004819 CrossRef CAS PubMed.
- Y. Kim, Y. Kim, X. Wang, B. Min and S. Park, TEMPO-oxidized cellulose nanofibril films incorporating graphene oxide nanofillers, Polymer, 2023, 15, 2646 CAS.
- N. Li, R. Zhang, X. Yang and D. Lin, Bacterial cellulose nanofibers used as nanofillers improved the fresh-keeping performance of gelatin-based edible coating for fresh-cut apples, J. Food Sci., 2023, 88, 4131–4145 CrossRef CAS PubMed.
- L. Jabbour, R. Bongiovanni, D. Chaussy, C. Gerbaldi and D. Beneventi, Cellulose-based Li-ion batteries: A review, Cellulose, 2013, 13, 1523–1545 CrossRef.
- L. Zhang, H. Ga, G. Jin, S. Liu, J. Wu, H. Wu, Y. Yang, Q. Wang and S. Wang, Cellulose-based electrolytes for advanced lithium-ion batteries: Recent advances and future perspectives, ChemNanoMat, 2022, 8, e202200142 CrossRef CAS.
- Z. Alipour, V. Babu Borugadda, H. Wang and A. K. Dalai, Syngas production through dry reforming: A review on catalysts and their materials, preparation methods and reactor type, Chem. Eng. J., 2023, 452, 146314 CrossRef.
- T. Du, X. Han, X. Yan, J. Shang, Y. Li and J. Song, MXene-based flexible sensors: Materials, preparation, and applications, Adv. Mater. Technol., 2023, 8, 220029 Search PubMed.
- L. Zhang, K. Wang, S. Weng and X. Jiang, Super strong and tough anisotropic hydrogels through synergy of directional freeze-casting, metal complexation and salting out, Chem. Eng. J., 2023, 463, 142414 CrossRef CAS.
- Y. Tong, S. Huang, X. Meng and Y. Wang, Aqueous-cellulose-solvent-derived changes in cellulose nanocrystal structure and reinforcing effects, Polymer, 2023, 15, 3030 CAS.
- S. Wang, L. Zhang, Q. Zeng, X. Liu, W. Lai and L. Zhang, Cellulose microcrystals with brush-like architectures as flexible all-solid-state polymer electrolyte for lithium-ion battery, ACS Sustainable Chem. Eng., 2020, 8, 3200–3207 CrossRef CAS.
- S. Xiao, F. Wang, Y. Yang, Z. Chang and Y. Wu, An environmentally friendly and economic membrane based on cellulose as a gel polymer electrolyte for lithium ion batteries, RSC Adv., 2014, 4, 76–81 RSC.
- G. Zhong, P. Wang, K. Lu, H. Cao, W. Shi, W. Yan and Y. Zhu, Cellulose-based gel-type electrolyte fabricated by lyophilization to enable uniform Li+ ion flux distribution for stable Li metal anodes with high-rate capability, Appl. Mater. Today, 2023, 30, 101705 CrossRef.
- M. Yadav, S. Upadhyaya and K. Singh, Enhancing the hydrophobicity and surface roughness of synthesized PVDF membrane using evaporation and non-solvent-induced phase separation, Arabian J. Sci. Eng., 2024, 1–12 CAS.
- G. F. Godshall, D. A. Rau, C. B. Williams and R. B. Moore, Additive manufacturing of poly(phenylene sulfide) aerogels via simultaneous material extrusion and thermally induced phase separation, Adv. Mater., 2023, 2307881 CrossRef PubMed.
- E. Caliskan, S. Shishatskiy, V. Abetz and V. Filiz, Pioneering the preparation of porous PIM-1 membranes for enhanced water vapor flow, RSC Adv., 2024, 14, 9631–9645 RSC.
- Y. S. Zhu, S. Y. Xiao, M. X. Li, Z. Chang, F. X. Wang, J. Gao and Y. P. Wu, Natural macromolecule based carboxymethyl cellulose as a gel polymer electrolyte with adjustable porosity for lithium-ion batteries, J. Power Sources, 2015, 288, 368–375 CrossRef CAS.
- Z. Xu, D. Guo, Z. Liu, Z. Wang, Z. Gu, D. Wang and X. Yao, Cellulose acetate-based high-electrolyte-uptake gel polymer electrolyte for semi-solid-state lithium-oxygen batteries with long-cycling stability, Chem. – Asian J., 2022, 17, e202200712 CrossRef CAS PubMed.
- H. Liao, H. Hong, H. Zhang and Z. Li, Preparation of hydrophilic polyethylene/methylcellulose blend microporous membranes for separator of lithium-ion batteries, J. Membr. Sci., 2016, 498, 147–157 CrossRef CAS.
- X. Wang, D. Chen, T. He, Y. Zhou, L. Tian, Z. Wang and Z. Cui, Preparation of lateral flow PVDF membrane via combined vapor and non-solvent-induced phase separation (V-NIPS), Membranes, 2023, 13, 91 CrossRef CAS PubMed.
- J. Y. Seo, Y. J. Choi, Y. Kang and K. Baek, Protection group assisted non-solvent induced phase separation for PO3H2 functionalized PVDF ultrafiltration membranes with a heavy metal removal capability, J. Membr. Sci., 2024, 695, 122468 CrossRef CAS.
- X. Zuo, X. Ma, J. Wu, X. Deng, X. Xiao, J. Liu and J. Nan, Self-supporting ethyl cellulose/poly(vinylidene fluoride) blended gel polymer electrolyte for 5 V high-voltage lithium-ion batteries, Electrochim. Acta, 2018, 271, 582–590 CrossRef CAS.
- J. Chen, Y. Wang, Z. Gu, J. Huang, W. He and P. Liu, Rational design of hierarchical yolk-double shell Fe@NCNs/MnO2 via thermal-induced phase separation toward wideband microwave absorption, Carbon, 2023, 204, 305–314 CrossRef CAS.
- M. Zulkifli, D. Nordi, N. Shaari and S. K. Kamarudin, Overview of electrospinning for tissue engineering applications, Polymers, 2023, 11, 2418 CrossRef PubMed.
- W. M. Kang, X. M. Ma, H. H. Zhao, J. G. Ju, Y. X. Zhao, J. Yan and B. W. Cheng, Electrospun cellulose acetate/poly(vinylidene fluoride) nanofibrous membrane for polymer lithium-ion batteries, J. Solid State Electrochem., 2016, 20, 2791–2803 CrossRef CAS.
- Q. Sabrina, Sudaryanto, N. Majid, A. Sugawara, Y. Hsu, R. Yudianti and H. Uyama, Electrospinning of bacterial cellulose modified with acetyl groups for polymer electrolyte li-ion Batteries, J. Electron. Mater., 2024, 53, 2062–2075 CrossRef CAS.
- B. C. Weng, F. H. Xu, M. Alcoutlabi, Y. B. Mao and K. Lozano, Fibrous cellulose membrane mass produced via forcespinning for lithium-ion battery separators, Cellulose, 2015, 22, 1311–1320 CrossRef CAS.
- M. Zhu, J. Lan, C. Tan, G. Sui and X. Yang, Degradable cellulose acetate/poly-L-Iactic acid/halloysite nanotube composite nanofiber membranes with outstanding performance for gel polymer electrolytes, J. Mater. Chem. A, 2016, 4, 12136–12143 RSC.
- B. S. Lalia, Y. A. Samad and R. Hashaikeh, Nanocrystalline cellulose-reinforced composite mats for lithium-ion batteries: electrochemical and thermomechanical performance, J. Solid State Electrochem., 2012, 17, 575–581 CrossRef.
- J. Pan, N. Wang and H. Fan, Gel polymer electrolytes design for Na-ion batteries, Small Methods, 2022, 6, 2201032 CrossRef CAS PubMed.
- W. Chae, B. Kim, W. S. Ryoo and T. Earmme, A brief review of gel polymer electrolytes using in situ polymerization for lithium-ion polymer batteries, Polymer, 2023, 15, 803 CAS.
- Y. Huang, Y. Wang and Y. Fu, All-cellulose gel electrolyte with black phosphorus-based lithium-ion conductors toward advanced lithium-sulfurized polyacrylonitrile batteries, Carbohydr. Polym., 2022, 296, 119950 CrossRef CAS PubMed.
- F. Yu, H. Zhang, L. Zhao, Z. Sun, Y. Li, Y. Mo and Y. Chen, A flexible cellulose/methyl cellulose gel polymer electrolyte endowing superior Li+ conducting property for lithium-ion battery, Carbohydr. Polym., 2020, 246, 116622 CrossRef CAS PubMed.
- M. Liu, S. Zhang, G. Li, C. Wang, B. Li, M. Li, Y. Wang, H. Ming, Y. Wen, J. Qiu, J. Chen and P. Zhao, A cross-linked gel polymer electrolyte employing cellulose acetate matrix and layered boron nitride filler prepared via in situ thermal polymerization, J. Power Sources, 2021, 484, 229235 CrossRef CAS.
- Q. Yang, N. Deng, J. Chen, B. Cheng and W. Kang, The recent research progress and prospect of gel polymer electrolytes in lithium-sulfur batteries, Chem. Eng. J., 2021, 413, 127427 CrossRef CAS.
- J. Courtenay, R. Sharma and J. Scott, Recent advances in modified cellulose for tissue culture applications, Molecules, 2018, 23, 654 CrossRef PubMed.
- C. Yang, Q. Wu, W. Xie, X. Zhang, A. Brozena, J. Zheng, M. N. Garaga, B. H. Ko, Y. Mao, S. He, Y. Gao, P. Wang, M. Tyagi, F. Jiao, R. Briber, P. Albertus, C. Wang, S. Greenbaum, Y. Hu, A. Isogai, M. Winter, K. Xu, Y. Qi and L. Hu, Copper-coordinated cellulose ion conductors for solid-state batteries, Nature, 2021, 598, 590–596 CrossRef PubMed.
- Q. Dong, X. Zhang, J. Qian, S. He, Y. Mao, A. H. Brozena, Y. Zhang, L. Xu, Y. Liang, Y. Yao, T. Li and L. Hu, A cellulose-derived supramolecule for fast ion transport, Sci. Adv., 2022, 8, eadd2031 CrossRef CAS PubMed.
- S. Wang, L. Yu, S. Wang, L. Zhang, L. Chen, X. Xu, Z. Song, H. Liu and C. Chen, Strong, tough, ionic conductive, and freezing-tolerant all-natural hydrogel enabled by cellulose-bentonite coordination interactions, Nat. Commun., 2022, 13, 3408 CrossRef CAS PubMed.
- D. Wang, H. Xie, Q. Liu, K. Mu, Z. Song, W. Xu, L. Tian, C. Zhu and J. Xu, Low-Cost, High-strength cellulose-based quasi-solid polymer electrolyte for solid-state lithium-metal batteries, Angew. Chem., 2023, 62, e202302767 CrossRef CAS PubMed.
- S. Nematdoust, R. Najjar, D. Bresser and S. Passerini, Partially oxidized cellulose grafted with polyethylene glycol mono-methyl ether (m-PEG) as electrolyte material for lithium polymer battery, Carbohydr. Polym., 2020, 240, 116339 CrossRef CAS PubMed.
- Z. Liu, R. Wang, Q. Ma, H. Kang, L. Zhang, T. Zhou and C. Zhang, Application of cellulose-based hydrogel electrolytes in flexible batteries, Carbon Neutralization, 2022, 2, 126–139 CrossRef.
- H. Wu, J. Wang, Y. Zhao, X. Zhang, L. Xu, H. Liu, Y. Cui, Y. Cui and C. Li, A branched cellulose-reinforced composite polymer electrolyte with upgraded ionic conductivity for anode stabilized solid-state Li metal batteries, Sustainable Energy Fuels, 2019, 10, 2642–2656 RSC.
- H. Zhang, S. Wang, A. Wang, Y. Li, F. Yu and Y. Chen, Polyethylene glycol-grafted cellulose-based gel polymer electrolyte for long-life Li-ion batteries, Appl. Surf. Sci., 2022, 593, 153411 CrossRef CAS.
- Z. Du, Y. Su, Y. Qu, L. Zhao, X. Jia, Y. Mo, F. Yu, J. Du and Y. Chen, A mechanically robust, biodegradable and high-performance cellulose gel membrane as gel polymer electrolyte of lithium-ion battery, Electrochim. Acta, 2019, 299, 19–26 CrossRef CAS.
- T. Rasheed, A. Naveed, J. Chen, B. Raza and J. Wang, Revisiting the role of polymers as renewable and flexible materials for advanced batteries, Energy Storage Mater., 2022, 45, 1012–1039 CrossRef.
- Y. An, X. Han, Y. Liu, A. Azhar, J. Na, A. K. Nanjundan, S. Wang, J. Yu and Y. Yamauchi, Progress in solid polymer electrolytes for lithium-ion batteries and beyond, Small, 2021, 18, 2103617 CrossRef PubMed.
- S. Gupta and A. Henson, Engineered nanocomposites through embedding of smaller “organic inorganic” nanoparticles in thermoplastic poly(2-vinylpyridine) polymer matrix, Composites, Part B, 2024, 272, 111207 CrossRef CAS.
- S. Li, L. Li, H. Yang, Y. Zhao and Y. Shan, A review of composite polymer electrolytes for solid-state lithium-sulfur batteries: Synthesis methods, optimal design, and critical challenges, Chem. Eng. J., 2024, 484, 149433 CrossRef CAS.
- X. Wu, Y. Zhang and S. Peng, An ambient-temperature superionic conductive, electrochemically stable, plastic cross-linked polymer electrolyte for lithium metal battery, J. Appl. Polym. Sci., 2024, 141, e522234 Search PubMed.
- C. Yan, Realizing high performance of solid-state lithium metal batteries by flexible ceramic/polymer hybrid solid electrolyte, Rare Met., 2020, 39, 458–459 CrossRef CAS.
- J. Liang, J. Luo, Q. Sun, X. Yang, R. Li and X. Sun, Recent progress on solid-state hybrid electrolytes for solid-state lithium batteries, Energy Storage Mater., 2019, 21, 308–334 CrossRef.
- S. Kundu and Y. Ein-Eli, A review on design considerations in polymer and polymer composite solid-state electrolytes for solid Li batteries, J. Power Sources, 2023, 553, 232267 CrossRef CAS.
- C. Yan, P. Zhu, H. Jia, Z. Du, J. Zhu, R. Orenstein, H. Cheng, N. Wu, M. Dirican and X. Zhang, Garnet-rich composite solid electrolytes for dendrite-free, high-rate, solid-state lithium-metal batteries, Energy Storage Mater., 2020, 26, 448–456 CrossRef.
- C. Ding, Y. Liu, L. K. Ono, G. Tong, C. Zhang, J. Zhang, J. Lan, Y. Yu, B. Chen and Y. B. Qi, Ion-regulating hybrid electrolyte interface for long-life and low N/P ratio lithium metal batteries, Energy Storage Mater., 2022, 50, 417–425 CrossRef.
- C. Wang, D. Huang, S. Li, J. Yu, M. Zhu, N. Liu and Z. Lu, Three-dimensional-percolated ceramic nanoparticles along natural-cellulose-derived hierarchical networks for high Li+ conductivity and mechanical strength, Nano Lett., 2020, 20, 7397–7404 CrossRef CAS PubMed.
- X. Lu, M. Duan and J. Li, Increase of the ionic conductivity of perovskite-type lithium-ion conductor by bacterial cellulose templating, Ceram. Int., 2023, 49, 24981–24988 CrossRef CAS.
- S. Johari, N. Tajuddin, H. Hanibah and S. K. Deraman, A review: Ionic conductivity of solid polymer electrolyte based polyethylene oxide, Int. J. Electrochem. Sci., 2021, 16, 211049 CrossRef CAS.
- Y. Zhang, J. Wang and Z. Xue, Electrode protection and electrolyte optimization via surface modification strategy for high performance lithium batteries, Adv. Funct. Mater., 2023, 34, 2311925 CrossRef.
- A. Chiappone, J. R. Nair, C. Gerbaldi, R. Bongiovanni and E. Zeno, UV-cured Al2O3-laden cellulose reinforced polymer electrolyte membranes for Li-based batteries, Electrochim. Acta, 2015, 153, 97–105 CrossRef CAS.
- H. Li, J. Yang, Z. Xu, H. Lu, T. Zhang, S. Chen, J. Wang, Y. N. Li and S. Hirano, Integrated composite polymer electrolyte cross-linked with SiO2-reinforced layer for enhanced Li-ion conductivity and lithium dendrite inhibition, ACS Appl. Energy Mater., 2020, 3, 8552–8561 CrossRef CAS.
- Q. Zeng, J. Wang, X. Li, Y. Ouyang, W. He, D. Li, S. Guo, Y. Xiao, H. Deng, W. Gong, Q. Zhang and S. Huang, Cross-linked chains of metal-organic framework afford continuous ion transport in solid batteries, ACS Energy Lett., 2021, 6, 2434–2441 CrossRef CAS.
- Y. Zhao, R. Wei, X. Feng, L. Sun, P. Liu, Y. Su and L. Shi, Dual-mode luminescent nanopaper based on ultrathin g-C3N4 nanosheets grafted with rare-earth up conversion nanoparticles, ACS Appl. Mater. Interfaces, 2016, 8, 21555–21562 CrossRef CAS PubMed.
- L. P. Teo, M. Buraidah and A. Arof, Development on solid polymer electrolytes for electrochemical devices, Molecules, 2021, 26, 6499 CrossRef CAS PubMed.
- S. Chen, K. Wen, J. Fan, Y. Bando and D. Golberg, Progress and future prospects of high-voltage and high-safety electrolytes in advanced lithium batteries: From liquid to solid electrolytes, J. Mater. Chem. A, 2018, 6, 11631–11663 RSC.
- S. Kale, T. Nirmale, N. Khupse, B. Kale, M. Kulkarni, S. Pavitran and S. Gosavi, Cellulose-derived flame-retardant solid polymer electrolyte for lithium-ion batteries, ACS Sustainable Chem. Eng., 2021, 9, 1559–1567 CrossRef CAS.
- S. Chereddy, J. Aguirre, D. Dikin, S. Wunder and P. Chinnam, Gel electrolyte comprising solvate ionic liquid and methyl cellulose, ACS Appl. Energy Mater., 2019, 3, 279–289 CrossRef.
- B. Zhao, M. Yang, J. Li, S. Li, G. Zhang, S. Liu, Y. Cui and H. Liu, Cellulose-based plastic crystal electrolyte membranes with enhanced interface for solid-state lithium batteries, Energy Technol., 2021, 9, 2100114 CrossRef CAS.
- J. Zheng, W. Li, X. Liu, J. Zhang, X. Feng and W. Chen, Progress in gel polymer electrolytes for sodium-ion batteries, Energy Environ. Mater., 2022, 6, e12422 CrossRef.
- S. Zhao, Q. Wu, W. Ma and L. Yang, Polyethylene oxide-based composites as solid-state polymer electrolytes for lithium metal batteries: A mini review, Front. Chem., 2020, 8, 640 CrossRef CAS PubMed.
- Y. Li, Z. Sun, D. Liu, S. Lu, F. Li, G. Gao, M. Zhu, M. Li, Y. Zhang, H. Bu, Z. Jia and S. Ding, Bacterial cellulose composite solid polymer electrolyte with high tensile strength and lithium dendrite inhibition for long life battery, Energy Environ. Mater., 2020, 4, 434–443 CrossRef.
- C. Gao, X. Li, G. Wei, S. Wang, X. Zhao and F. Kong, Cellulose acetate propionate incorporated PVDF-HFP based polymer electrolyte membrane for lithium batteries, Compos. Commun., 2022, 33, 101226 CrossRef.
- Q. Ma, D. Liu, B. Wang, W. Liu, G. Xiong and J. Liu, Cellulose acetate-promoted polymer-in-salt electrolytes for solid-state lithium batteries, J. Solid State Electrochem., 2023, 6, 1411–1421 Search PubMed.
- Y. Wu, Y. Li, Y. Wang, Q. Liu, Q. Chen and M. Chen, Advances and prospects of PVDF based polymer electrolytes, J. Energy Chem., 2022, 64, 62–84 CrossRef CAS.
- A. Konarov, S. Myung and Y. Sun, Cathode materials for future electric vehicles and energy storage systems, ACS Energy Lett., 2023, 8, 1042–1049 CrossRef.
- T. Nirmale, I. Karbhal, R. Kalubarme, M. Shelke, A. Varma and B. Kale, Facile synthesis of unique cellulose triacetate based flexible and high-performance gel polymer electrolyte for lithium-ion batteries, ACS Appl. Mater. Interfaces, 2017, 9, 34773–34782 CrossRef CAS PubMed.
|
This journal is © Institute of Process Engineering of CAS 2024 |
Click here to see how this site uses Cookies. View our privacy policy here.