Controlling saturation to improve per- and polyfluoroalkyl substance (PFAS) removal in biochar-amended stormwater bioretention systems†
Received
17th October 2023
, Accepted 28th March 2024
First published on 28th March 2024
Abstract
Black carbon-amended bioretention systems are an increasingly popular strategy for the removal of organic contaminants, including poly- and perfluoroalkyl substances (PFASs) and other trace organic contaminants (TOrCs), from urban stormwater. Many PFASs preferentially accumulate at the air–water interface, but detention time requirements for stormwater bioretention systems typically result in full saturation of the bioretention systems, effectively removing their air–water interfaces. This study assessed the effect of bioretention system saturation on removal of PFASs, metals, and hydrophilic TOrCs. A field-aged mixture of 40% v/v sand, 30% v/v zeolite, and 30% v/v biochar was packed into columns which were operated with hydraulic controls to remain unsaturated or fully saturated throughout the duration of the experiment. Twenty-four storm events sized to a 95th percentile storm at a California, United States military site were simulated using synthetic stormwater fortified with aqueous film forming foam-derived PFASs, TOrCs, and metals to mimic real-world conditions. Unsaturated conditions outperformed saturated conditions for removal of all PFASs analyzed. A simulated inadvertent system perturbation (i.e. flooding event) in the unsaturated columns did not result in significantly greater mobilization of PFAS mass, suggesting that more sorption to the biochar occurred as a result of the transient retention of PFASs at the air–water interface reducing kinetic sorption limitations. Overall, maintaining unsaturated conditions in a biofilter may extend the sorptive filter lifetime for PFASs by up to 83%. The results have implications for bioretention system application and design for PFAS removal in contaminated catchment areas.
Water impact
When stormwater biofilters are not saturated, per- and polyfluoroalkyl substances (PFASs) sorb to the air–water interface, likely reducing kinetic limitations to sorption. The design of black carbon-amended biofilters for PFAS removal can be improved by preventing saturation. Filter lifetimes may roughly double by installing inlet flow controls to maintain unsaturated conditions.
|
Introduction
Urban stormwater runoff is an important vector for releases of poly- and perfluoroalkyl substances (PFASs),1,2 metals,3 and trace organic contaminants (TOrCs)4 into surrounding ecosystems, potentially affecting drinking water supply and beneficial use of runoff. Sources of PFASs to stormwater include road dust,5 consumer products,6 runoff from firefighting training grounds,7 and wastewater discharges.8 Atmospheric sources of PFASs can also contaminate rainwater and contribute to and mobilize existing surface-bound PFASs.9 Particularly at sites where aqueous film-forming foam (AFFF) has been released, there is increasing evidence that PFASs can be absorbed10 and slowly released7 from concrete, resulting in the potential for traditional grey stormwater infrastructure and other cementitious surfaces to serve as long-term PFAS sources. Even when not impacted by PFASs, larger impervious surfaces in urban environments and on military facilities increase the intensity and volume of runoff and the pollutant load into receiving waterways, which may be mitigated by low-impact development.11
In March 2023, US EPA proposed a maximum contaminant level (MCL) of 4 ng L−1 for PFOS and PFOA in drinking water, and the US EPA 2022 draft freshwater aquatic life chronic water quality criteria for PFOS and PFOA are 8.4 μg L−1 and 94 μg L−1 respectively.12 As urban stormwater is increasingly being viewed as a potential drinking water supply source,13 there is a growing need to mitigate PFAS releases in stormwater runoff, both for water supply concerns as well as total releases to surface waters. Drinking water PFAS treatment technologies are well-developed14,15 but generally inappropriate for stormwater settings: stormwater treatment systems are often distributed (rather than centralized)16 and noncontinuous flow17 and background dissolved organic carbon levels18 may further limit the efficacy of PFAS drinking water technologies such as nanofiltration and ion exchange. Destructive stormwater treatment technologies have been evaluated for AFFF-impacted sites,19 but because these require high energy input and are expensive, they may be infeasible for implementation across large watersheds. Smaller scale, decentralized stormwater infrastructure on a catchment scale generally shows passive treatment benefits over active treatment.20,21 However, very few decentralized stormwater treatment technologies have been intentionally designed for organic contaminant treatment, much less PFASs.22
Some green stormwater control measures such as biofilters have been found to treat organic contaminants in runoff when including black-carbon products such as granulated activated carbon,23 which is well-studied for PFAS treatment in drinking water,24,25 and lower-cost and more sustainable yet similarly effective products such as biochar.26–29 While traditional stormwater control measures focus on treating water quality, enhancing them with black carbon amendments improves stormwater systems by enabling contaminant removal via sorption and transformation;30 biologically active biochar-amended filters have been shown to enhance removal of organic contaminants as well.31 As such, biochar-amended stormwater biofilters have become an attractive option for contaminant removal, and work well for a wide suite of contaminants,22,29,32 particularly when other amendments are also considered. For example, while some have found the relative contribution of certain zeolites to the removal of PFASs from stormwater to be negligible,33 enhanced removal of metals by zeolites has been observed.34 The limited data for PFAS removal in black carbon-amended stormwater biofilters33 suggest these compounds may be a limiting pollutant: breakthrough of PFASs such as PFOS may occur well before other contaminants of concern. This is likely driven by lower sorption partitioning coefficient values, especially for shorter-chain PFASs.35
Many PFASs preferentially accumulate at the air–water interface (AWI),36,37 which has likely led to significant retention of PFASs in vadose zone soils.38,39 The release of PFASs from impacted soils is the subject of much study,40,41 as limited evidence points to a role for kinetic limitations on the desorption from solids42 as well as a potential role for collapsing AWIs in PFAS releases.43 If not hydraulically controlled to maintain an unsaturated state, bioretention systems, much like soils, undergo multiple wet–dry cycles as dictated by local climactic conditions. These re-saturation events (i.e., precipitation events) thus effectively remove the majority of AWIs as the wetting front moves through the filter. Hydraulic controls have also been shown to improve flows in stormwater retention systems: dynamic controls with outlet valves provide higher removal of suspended solids in stormwater basins.44 While previous studies indicate that biochar-amended biofilters have promise for treatment of PFASs in stormwater,33 those filters were tested under saturated conditions. What remains unclear, however, is whether hydraulic controls that maintain unsaturated conditions within stormwater biofilters can enhance PFAS removal.
This study aimed to assess the effect of bioretention system saturation on removal of PFASs, metals, and TOrCs in bench-scale simulated stormwater biofilters. To achieve this, a field-aged mixture of sand, zeolite, and biochar was packed into columns which were operated with hydraulic controls to remain unsaturated or saturated throughout the duration of the experiment. Storm events, sized to 95th percentile storms for a coastal U.S. military facility in southern California, USA,45 were simulated using synthetic stormwater fortified with AFFF-derived PFASs, dissolved organic carbon, TOrCs, and metals to mimic real-world conditions.33 The longer-term potential for leaching of PFASs from the stormwater-dosed columns, such as from collapsing AWIs within the filter media, was also assessed by simulating a disruption of the hydraulic controls (i.e., a flooding event creating full saturation in both systems) with clean synthetic stormwater. The resultant data were then evaluated with respect to PFAS breakthrough, retention, and leaching.
Experimental
Materials and methods
Experimental setup.
Column experimental design was based partially on previous experiments.33 A mixture of 30 v% zeolite (BioGreen Technologies Inc., Boulder, CO, USA), 30 v% high-temperature gasification biochar (Biochar Supreme Environmental Ultra, Everson, WA, USA), and 40 v% sand (silica concrete sand) was mixed by a local media supplier and delivered to a site at Colorado School of Mines for use in a full-scale bioretention system, where it was field-aged for six months before a representative sample was taken for use in column experiments. The media mixture was sieved to 20 μm to 1.68 mm (12–40 mesh). Clear polyvinyl chloride (PVC) columns (90 cm length, 7.2 cm diameter) of a sufficient column-diameter to maximum grain-diameter (12 mesh; 1.68 mm maximum) ratio to ensure proper fluid hydraulics46 were roughed inside with a wire brush to reduce preferential flow paths and capped at the bottom with PVC endcaps and PVC reducing adapters connected to polytetrafluoroethylene tubing. Each column (six in total, three replicates each for both experimental conditions) was packed with 5 cm coarse silica barrier sand, 15 cm of engineered media mixture, and 5 cm of coarse silica sand to act as a protective layer. Columns were operated in gravity-driven downflow mode.
The effects of constantly saturated or constantly unsaturated treatment conditions were evaluated using hydraulic controls on three replicate columns for each condition. Constantly saturated conditions were maintained with outlet controls by fixing the outflow tubing outlet at the top of the barrier sand, creating a saturated zone (Fig. 1) that remained throughout the total length of the experiment. The three constantly unsaturated columns were allowed to drain freely by gravity, and inlet controls were employed to maintain unsaturated conditions by reducing the influent hydraulic loading: the field-scale equivalent of this design would be a stormwater detention basin that drains into a “polishing” biofilter, where flow from the detention basin into the biofilter is modulated with outlet controls regulating the inlet flow into the biofilter. The variable outlet flow prevented the calculation of an empty bed contact time, but is representative of biofilter conditions that would be expected in the field. The empty bed volume (EBV) in each column (calculated as total volume of engineered media, not including barrier sand) was 0.68 L.
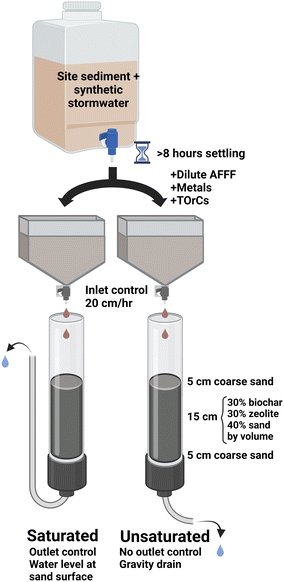 |
| Fig. 1 Diagram of experimental setup showing synthetic stormwater generation, inflow, engineered media description, and saturated and unsaturated conditions. | |
During the challenge phase, the top layer barrier sand was removed and replaced with clean sand as needed to maintain sufficient flow. Of note, columns that maintained saturation required more regular maintenance than the unsaturated columns: three different sand replacement events were performed on all three saturated columns during the eight-week study to remove biofilm clogs and maintain sufficient hydraulic conductivity to not overflow the column, compared to one cleaning event for one column for the unsaturated conditions.
Synthetic stormwater.
Synthetic stormwater was generated in 20 L high-density polyethylene (HDPE) carboys by mixing salts and catch-basin site sediment from Naval Weapons Station Seal Beach (NWSSB), CA, USA at 3 g L−1 with straw-derived dissolved organic carbon (DOC) concentrate generated as described in previous experiments,33 shaken vigorously, then allowed to settle at least 8 hours. The settled stormwater was then decanted into two additional 20 L HDPE carboys for fortification with a suite of contaminants. These included PFASs from diluted AFFF (previously characterized as primarily electrochemical fluorination (ECF)-derived47) at 4 μg as PFOS L−1; hydrophilic TOrCs (Hi-TOrCs) mecoprop, fipronil, imidacloprid, atrazine, diuron, and benzotriazole at 40 μg L−1 and metals Ni, Zn, Cu, Cd, and Pb at 200 μg L−1. AFFF was used to deliver an environmentally relevant mixture of PFASs. A suite of hydrophobic TOrCs (list in Table S6†) was included at 0.1 μg L−1 to provide a representative contaminant mixture to that found in urban runoff.
Spike solutions were prepared as previously described.33 Once dosed with contaminants, the synthetic stormwater was then introduced into individual HDPE cone bottom tanks (9.5 L/2.5 gal) fit with a 0.635 cm inner diameter on/off ball valve and a 0.635 cm inner diameter precision flow-adjustment valve, connected to PTFE tubing. Though PTFE is typically avoided for experimental PFAS work, target and suspect analysis for PFASs (see below) during the initial sampling events indicated that the PTFE tubing did not leach measurable PFASs, which is consistent with prior studies.48 Inlet flow was maintained at approximately 20 cm h−1, a typical specification for stormwater growing media infiltration rate.
Conditioning and challenge experiments.
Columns were conditioned with clean synthetic stormwater (stormwater prepared with salts, straw-derived DOC, and site sediment but without added contaminants) for 100 EBV (17 instances of 4 L per column “storm events” over six weeks), with one to two antecedent dry days between events. The challenge phase consisted of 24 individual 4 L “storm events” per column sized to a 95th percentile design storm at NWSSB,45 or 1.778 cm (0.7′′) precipitation over a hypothetical catchment area of which the filter area was assumed to be 2%. The total amount of stormwater volume was equivalent to 1.5 years of precipitation in Long Beach, CA, an adjacent city to NWSSB.49 Each storm event was dosed into the columns three times a week for eight weeks, with one to two antecedent dry days between each simulated storm, and lasted 4–6 hours. Saturated-condition columns were kept saturated during conditioning and challenge phase storm events, while unsaturated columns drained freely during the conditioning and challenge phases. Influent samples were taken as 1 mL composites from all six individual spiking tanks for a total of 6 mL. One composite effluent sample was taken per column per each storm event at 1 hour intervals, consistent with a time compositing sample collection method;50 consistent volumes of sample were collected every hour for three hours after influent flow began, for a total of 6 mL sample taken for PFASs in a pre-weighed 15 mL polypropylene (PP) tube, 10 mL sample for metals in a 15 mL PP tube, and a 5–15 mL sample for hydrophilic TOrCs in a 20 mL glass scintillation vial.
After the challenge phase was complete, a 40 ppm bromide tracer test was conducted with 2 L clean synthetic stormwater per column (ESI;† Fig. S9). Each column was then cored with a 3.175 cm (1.25′′) PVC tube to preserve media contaminant content while a flush event was conducted to determine the effect of inadvertent column saturation in field applications (i.e., a simulated failure of the inlet controls). During the flush event, the water level in the unsaturated columns was raised to ensure complete saturation using an equivalent elevation-based outflow control. Columns were dosed with a double storm event volume (8 L) and then allowed to drain completely. Three composite effluent samples were taken throughout the flushing event. After completion of the challenge and flushing events, the filter media retained from the core was divided into three equivalent 5 cm sections and each section was individually dried and homogenized.
Analytical methods.
PFAS water and biofilter media samples were stored at −20 °C, thawed immediately prior to analysis, then prepared as previously described42,51 and analyzed via LC-QToF-MS (X500R; SCIEX, Framingham, MA) operated in negative electrospray mode. For both aqueous and solid-phase samples, HRMS data were processed in SCIEX OS v1.6.2 for target compounds listed in the ESI† and suspect data analyzed using a custom XIC list with >1400 PFASs and a custom spectral library with >300 PFASs; semiquantitative analysis was performed by estimation using a calibration and internal standard for a target compound based on chain length and ionizable moiety. More details on the semiquantitative method used in this study are available in Nickerson et al.52
PFAS solid media extraction was conducted using a method previously described in Nickerson et al.;52 a 0.50 ± 0.01 g aliquot of this filter media was weighed into a 50 mL polypropylene centrifuge tube, spiked with 4 ng internal standard, and extracted using two rounds of basic methanol and two rounds of acidic methanol, then reconstituted using 1% acetic acid in methanol. For the 18 samples analyzed, a method blank with internal standard was also prepared, and two samples (11%) were extracted in triplicate.
For PFAS water analysis, one method blank in a 15 mL PP sample vial using water, solvents, and internal standard was prepared for every batch of 20 samples. For water and soil analysis, one laboratory blank containing water, solvents, and internal standard was analyzed per 10 samples analyzed, along with one double blank and one quality control sample calibrated to a mid-range point on the calibration curve. The batch of ten samples preceding and succeeding a quality control sample was reacquired if the quality control concentration was not within 70–130% of its expected value. Limits of quantitation (LOQs) were determined using either the lowest adjacent point of a minimum of five on the calibration curve, or twice the concentration of the lowest method or laboratory blank, whichever was higher. A table of LOQs is provided in the ESI† (Tables S7–S9).
Metals samples were filtered using 0.45 μm PES syringe filters (SF14501, Tisch Scientific, OH, USA) and acidified using 200 μL nitric acid to pH <2 and analyzed via ICP-AES using US EPA method 200.7.53 Hi-TOrC samples were filtered using 0.45 μm glass fiber syringe filters (SF15159, Tisch Scientific, OH, USA), transferred to 2 mL glass vials, spiked with internal standards, and analyzed at Stanford University using LC–MS/MS (API 3000, Applied Biosystems, MA, USA).33 Bromide samples from the tracer test were filtered using 0.45 μm PES syringe filters and refrigerated, then analyzed via IC (Dionex ICS-900; ThermoFisher Scientific). DO, pH, and conductivity were analyzed every two weeks (every 6 storm events) using a portable Hach multimeter (HQ40D) and DO/pH probes. Nitrate, phosphate, and ammonia concentrations were analyzed twice during the experiment spectrophotometrically using HACH TNTplus vial tests 835, 843, and 830, respectively.
Statistical methods.
To compare the mass released between two groups (i.e., for the flush event), and effluent concentration differences, statistical significance was determined using a two-tailed Student's paired t-test. Results were deemed significant at p = 0.05. The relative standard deviation was calculated to illustrate the homogeneity of the column soil concentrations.
Results & discussion
Removal of PFASs under constantly unsaturated conditions
Both saturated and unsaturated column configurations provided substantial water quality improvement with respect to PFASs, Hi-TOrCs, and metals for the duration of the experiment (Fig. 2 and S1–S8†). Across all PFASs analyzed, unsaturated conditions generally outperformed saturated conditions, likely due to preferential PFAS retention at the AWI that was maintained by the constant unsaturated conditions. Influent concentration of target PFASs varied in magnitude from 3920 ± 1280 ng L−1 PFOS to 6 ± 3 ng L−1 FBSA throughout the experiment (full description of influent in Tables S2–S5†). Although these influent PFAS concentrations are higher than typically found in stormwater,54 these elevated concentrations were used to facilitate observation of breakthrough for multiple PFASs, and may also be representative of runoff from some PFAS source areas.10
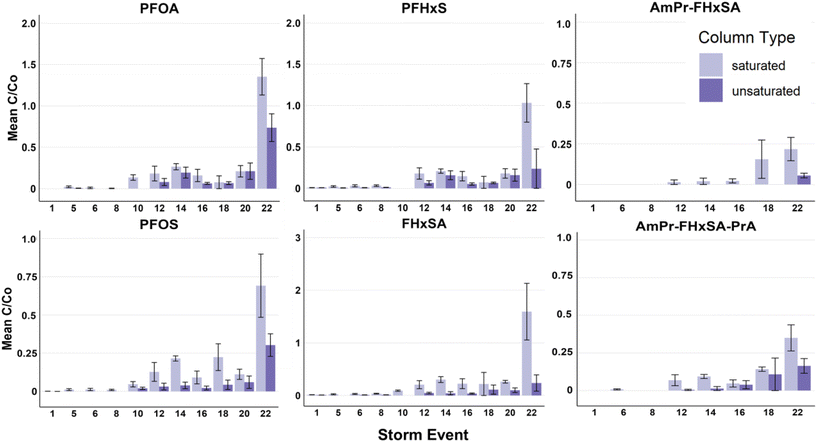 |
| Fig. 2 Saturated vs. unsaturated removal efficiency (influent/effluent) for select PFASs for storm events 1–22. The twenty-fourth storm event was omitted due to an error in the spiking solution; influent and effluent values and figures including storm 24 can be found in the ESI.† | |
An evaluation of the number of storms to initial breakthrough (defined as 10% breakthrough, C/Co = 0.1) indicates that the retention of PFASs in the unsaturated columns was generally chain-length dependent (shorter-chain PFASs were less retained than longer-chain PFASs; Fig. 3). Except for PFHpS and PFOS (for which 10% breakthrough was observed for both at the 22nd simulated storm event), chain-length dependent breakthrough was consistently observed. However, in contrast to what has been observed in previous saturated experiments by Pritchard et al.33 where PFHxS broke through 16% faster than FHxSA in zeolite + biochar columns and 91% faster than FHxSA in zeolite + regenerated activated carbon columns, here PFHxS and FHxSA broke through at approximately the same time in unsaturated conditions, though FHxSA exhibited full breakthrough (i.e., C/Co = 1) by the 22nd storm in the saturated conditions in this study (Fig. 2 and 3).
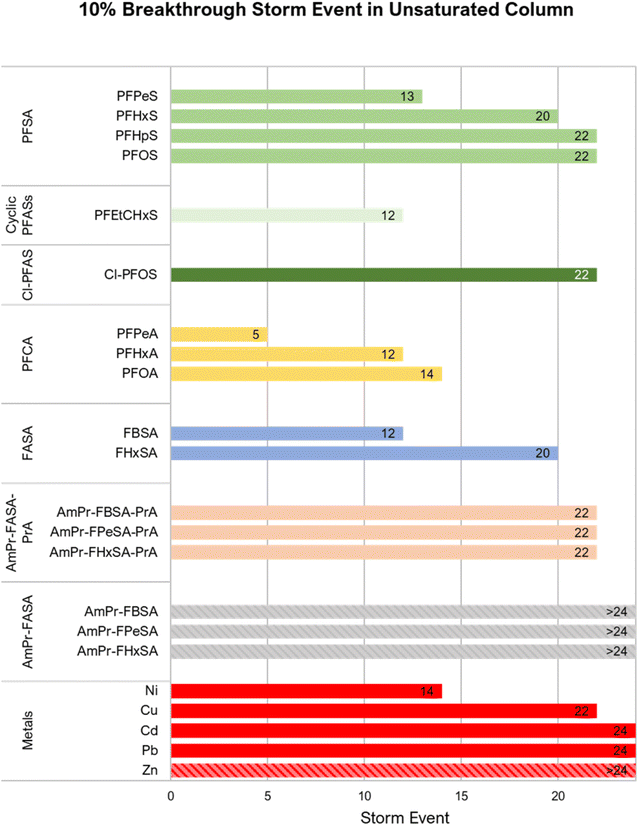 |
| Fig. 3 Mean 10% breakthrough (C/Co = or >0.1) storm event (storm event = 4 L; 95th percentile storm event at a relevant military site in Seal Beach, CA, USA) in unsaturated columns only, in order of chain length for select target and suspect PFASs and metals, grouped by contaminant class. | |
Removal of Hi-TOrCs and metals
Hi-TOrCs and metals were effectively removed in both the saturated and unsaturated columns. Only the chlorophenoxy herbicide mecoprop reached 5% breakthrough by the end of the challenge phase in saturated columns, with no breakthrough in unsaturated columns: all other Hi-TOrCs were not detected or did not increase appreciably during the experiment (Fig. S8†). In short, despite shorter contact time (observed by less ponding occurring in and superior PFAS removal in unsaturated columns), removal of the other TOrCs studied did not suffer. In contrast to PFAS removal, the removals for the metals Cd, Zn, and Pb were not significantly different between the two conditions. However, Cu removal performed better under unsaturated conditions (p = 0.03) and Ni removal was more efficient under saturated conditions (p = 0.005). Pb exhibited lower concentration than expected in the influent; it likely partitioned to suspended sediment, complexed with DOC, or precipitated as lead (II) phosphate. The Zn-containing catch-basin material potentially preloaded the columns during the conditioning phase. Nevertheless, Zn removal was constant throughout the experiment, indicating that Zn removal capacity had not (yet) been exceeded.
Depth profile and mass balance of PFASs
To evaluate the distribution of PFASs in the filter bed at the completion of the experiment (prior to the flushing event), a depth profile of the PFASs extracted from the cored section of the biofilters is provided in Fig. 4. Consistent with distribution results in ion-exchange resin used to treat AFFF-impacted groundwater,55 the more mobile shorter-chain PFASs such as PFPeS and PFPeA are relatively more concentrated in the lower part of the columns, likely because of displacement by longer-chain PFASs with higher sorption affinities for the geomedia. Concentrations of the longer chain PFASs such as PFOA are relatively elevated at the top of the columns: within each PFAS class, there is a clear trend of increasing perfluoroalkyl chain length resulting in relatively elevated concentrations in the upper portion of the column as compared to the lower part of the column. The relative concentration of FHxSA, a PFAS that was present in the AFFF but also may have formed from the transformation of other polyfluoroalkyl species,56 is elevated in the upper and middle sections of the unsaturated column despite later breakthrough than the longer-chain PFASs (e.g., PFHpA, PFHpS and Cl-PFOS), and this relative enrichment is much higher than the other C6 PFASs (i.e., PFHxS and PFHxA). This profile could indicate transformation occurring in the most oxic (upper) sections of the column, with the resultant FHxSA immediately sorbing to the column as the treated water percolates. In general terms, the saturated columns show more homogeneous concentrations across the column (average variance across column 0.04); unsaturated columns show greater concentrations in the center of the column and are more heterogeneous (average variance 0.09). The relative abundance of AmPr-FPeSA-PrA in the unsaturated column may be due to transformation of this compound in the upper layers or displacement by other PFASs.
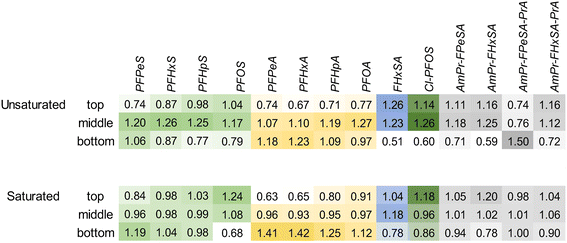 |
| Fig. 4 Illustration of relative media concentration by PFAS species in a black carbon amendment with a high AWI (unsaturated columns) vs. low AWI (saturated columns). Values are normalized to mean values for that compound and the column saturation type. Only PFASs with influent concentration above 20 ng L−1 are shown. | |
When the total mass of each PFAS applied to the columns is compared to the sum of estimated mass retained in the column and estimated mass in effluent, these mass balance data indicate close to full mass recovery for many of the PFASs included (Fig. 5) with all PFASs >0.7 times the influent load, apart from PFPeA. In the case of PFPeA, the poor mass recovery (45% saturated, 36% unsaturated) likely reflects the relative low concentration of PFPeA in the influent (31 ng L−1) and low (but significant in terms of relative influent mass) levels of PFPeA in the effluent (i.e., less than the LOQ of 5 to 10 ng L−1). In contrast, particularly for the unsaturated columns, the over-recovery of the sulfonamides FBSA and FHxSA likely reflect their potential formation from polyfluoroalkyl substances (i.e., precursors) with N-substituted sulfonamide head groups. For example, FHxSA is a commonly observed (and likely semi-stable57) intermediate of parent compounds such as AmPr-FHxSA.56,58 Such precursor transformation is expected and likely leads to more microbiologically inert and commonly regulated PFASs.59,60 Volatilization losses are not expected to contribute heavily to lack of mass recovery for most of the strongly acidic and anionic PFASs studied here,61 though some may volatilize when present in their neutral forms.62
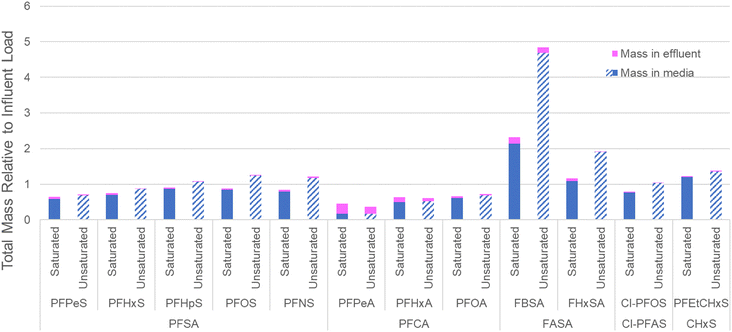 |
| Fig. 5 Mass balance. The ratio of soil and effluent sums of mass loads to the total influent mass load in each condition; a value of 1 means the total mass load in influent is equal to total recovered PFASs in effluent and media. Mass in effluent was calculated by summing all mass in all samples measured with linearly extrapolated masses from storm events that were not assessed. Mass in filter media was calculated by multiplying the media concentrations by the column mass. | |
Simulating hydraulic failure
One concern about relying on the AWI for retention of PFASs during stormwater treatment is the potential for significantly elevated releases of AWI-retained PFASs upon complete saturation, particularly given the transient nature of the AWI. However, data from the simulated inlet control failure (i.e., flushing event with clean synthetic stormwater) suggest that system failure for the unsaturated conditions would remobilize an equivalent mass of PFASs as the fully saturated conditions (Fig. 6), despite retention of greater masses of PFASs in the columns (Fig. 5). For most PFASs, no significant differences were apparent between the flush mass of PFASs released from the unsaturated and saturated conditions (p = 0.026 for target PFASs; data in Table S11†). More importantly, as a percentage of the total mass retained on the column, slightly less total mass was eluted from the unsaturated column during the flush, suggesting that if retention at the AWI was responsible for greater contaminant removal, the collapse of these interfaces would not threaten filter resiliency (see Table S11†). For example, during the flush, 0.9% of total retained PFOA mass eluted from saturated columns, while 0.8% eluted from unsaturated columns. Total flushed PFOS mass for saturated and unsaturated columns was 0.3% of mass retained in both conditions.
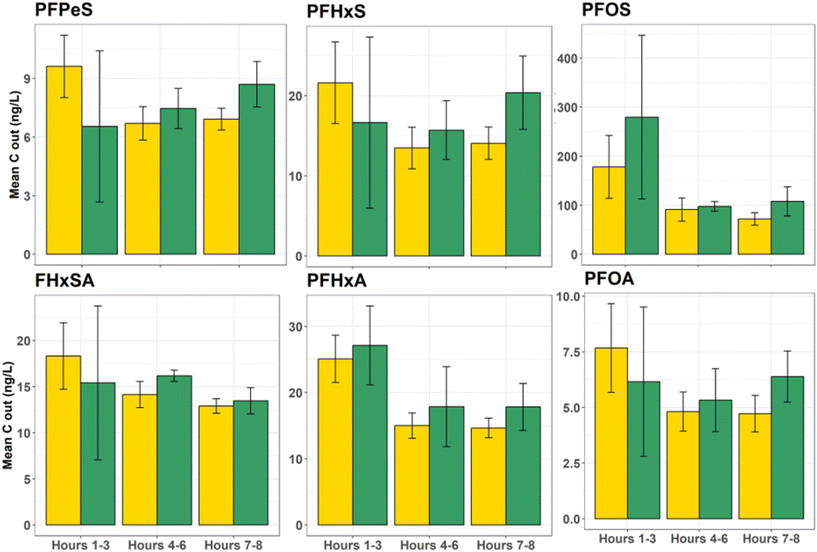 |
| Fig. 6 Flush event demonstrating similar PFAS release between saturated and unsaturated columns when fully saturated and then allowed to freely drain. | |
The greater removal by the unsaturated columns during simulated storm events and yet comparable leaching in both conditions during the flushing event was somewhat surprising. The premise of the study was that any significantly greater mass being retained under the unsaturated flow conditions would be a result of air–water interfacial retention, which, in theory, would be readily released upon re-saturation (i.e., a collapse of AWIs that had been maintained throughout the 24 simulated storms because of the hydraulic inlet controls). The lack of a “pulse” release due to the collapse of these interfaces suggests that although initial retention may have been due to this interfacial partitioning, the additional time spent in the engineered media (enabled by the AWI retention) allowed for greater uptake of PFASs into the sorbent media. In other words, PFAS adsorption to the AWI in these unsaturated pores may have allowed for more time for the PFASs absorbed to that interface to sorb into the biochar, increasing the effective contact time for PFASs and other compounds that interact with the air–water interface. Typically, PFAS sorption to black carbon is kinetically limited in faster-flow applications such as those required by higher flow rate stormwater control measures,35 as was observed in previous saturated experiments conducted with a face velocity of 20 cm h−1.33 PFAS adsorption kinetics may vary based on the presence of co-contaminants, sorbent porosity, and the functional groups present on the sorbent.63 Various sorption models can be used to model PFAS sorption to black carbon amendments;64,65 the results here encourage closer scrutiny on PFAS–AWI interactions in unsaturated systems during transport and retention modeling.
Lifetime estimate
Lifetime estimates were based on a previous modeling effort considering kinetic limitations in contaminant transport65 trained and validated with similarly composed filters, synthetic stormwater, and contaminant loading with a continuous flow model. A saturated 0.3 m-depth filter operated at a 20 cm h−1 flow rate was estimated to remove PFOS at 55 ng L−1 (an industrial runoff concentration from MN, USA selected as a chronic benchmark for the modeling66) to below the California, USA notification level of 6.5 ng L−1 for an estimated 13.9 ± 4.0 years; this represents approximately 12% breakthrough. In this study, PFOS, spiked at 4 μg L−1, experienced 12% breakthrough at storm event 12 in saturated systems and storm event 22 in unsaturated systems, an 83% slower breakthrough. Assuming the ratio of time to breakthrough for the saturated and unsaturated filters remains constant at the two levels of influent concentrations, one might expect up to an 83% increase in filter lifetime under unsaturated conditions. At NWSSB, this corresponds to a lifetime of 25.4 years. This improvement in filter lifetime for PFOS may not change the lifetime for other contaminants such as metals and pesticides, but as PFOS is often a limiting contaminant due to low regulatory limits, increasing PFOS lifetime increases the overall filter lifetime, as long as filter maintenance occurs regularly. A disproportionately longer lifetime might be achieved by extending filter depth.65
More detailed, mechanistic modeling as well as kinetic experiments to determine the minimum duration necessary for maintaining the AWI to achieve enhanced sorption may be helpful in estimating the impact of unsaturated conditions on filter lifetime. However, other stormwater models that are currently developed32,65 are set up for continuous flow or saturated conditions, while this study involved noncontinuous, intermittent flow and unsaturated conditions (i.e., the presence of an AWI). As the AWI-based retention appears to reduce kinetic limitations for PFAS sorption to the biochar instead of being a final sink (as indicated by the flush experiment), different sorption kinetics are likely in effect in the filters. To be more robust, a model should consider the resultant effect on kinetics from a system maintaining AWI (i.e., removing or reducing kinetic limitations from the sorption model); however, this modeling effort is beyond the scope of this study. Ultimately, though, antecedent dry days cannot be controlled in the field, and thus such efforts must be viewed solely in the context of understanding the mechanisms responsible for the enhanced removal observed here. Regardless, the breakthrough data from this experiment implies that filter lifetime can be extended by (possibly) removing kinetic limitations through unsaturated flow and the presence of an AWI.
In the context of remobilization of PFASs from the top layer of sediment, unsaturated biofilters can be advantageous as well. As shown in Fig. 4, the unsaturated system concentrated most PFASs below the top 5 cm; first flush and high energy storms may not reintroduce PFASs from these systems into the water column in the same way as saturated filters. Finally, the unsaturated columns in our study show promise for reduced maintenance relative to saturated columns; during the course of this experiment, the unsaturated columns clogged much less frequently in the barrier sand at the top of the column, requiring less maintenance to restore flow. Presumably, this may transfer to better field performance, increased filter longevity, and reduced cost.
Implications
Remediation of stormwater at AFFF-impacted sites is a priority, and this study shows performance improvements at these sites may be as simple as adding inlet controls to existing black carbon-amended infiltration stormwater control measures, or adding volumetric storage with regulated, unsaturated biofilter polishing using black carbon-amended media. Saturated filters, such as those with an internal water storage zone, are known to improve stormwater effluent quality for some constituents;67 including an unsaturated zone could improve removal of PFASs, likely to be the most difficult-to-remove contaminants. Both saturated and unsaturated column configurations provided substantial water quality improvement with respect to PFASs, Hi-TOrCs, and metals for the duration of the experiment. Across all PFASs analyzed, unsaturated conditions outperformed saturated conditions, likely due to their preferential sorption to the AWI under unsaturated conditions. While there were insufficient data to observe and compare the removal of Hi-TOrCs in this experiment due to exceedingly efficacious sorption by the biochar amendment, data for metals indicated saturated conditions performed better for Ni removal, while unsaturated conditions performed better for Cu removal; all other metals performed similarly in each condition. The results have implications for bioretention system design for PFAS removal in contaminated catchment areas. Introducing designs (e.g., inlet flow controls) that maintain an unsaturated biofilter containing specialized treatment media may be an acceptable lower-cost method for treating low to moderate levels of PFASs, while increasing treatment performance longevity.
Author contributions
Kathleen Mills Hawkins – conceptualization, formal analysis, investigation, methodology, software, visualization, writing – original draft. James Conrad Pritchard – conceptualization, formal analysis, methodology, validation, writing – review & editing. Yeo-Myoung Cho – conceptualization, methodology, project administration, writing – review & editing. Scott Struck – conceptualization, methodology, writing – review & editing. Richard Luthy – conceptualization, funding acquisition, methodology, project administration, resources, writing – review & editing. Chris Higgins – conceptualization, funding acquisition, methodology, project administration, resources, supervision, writing – review & editing.
Conflicts of interest
There are no conflicts to declare.
Acknowledgements
The authors would like to acknowledge the contributions of Ciara O'Grady, Madi Deter, Cooper Rockwell, Aidan Borgo, Sophia Nelson, Stefanie Shea, Sarah Choyke, Brittnee Halpin, Nick Gonda, Creighton Hawkins, Cecil Hawkins. Fig. 1 and Table of Contents entry created with https://www.Biorender.com. Some of the work was performed in the following core facility, which is a part of Colorado School of Mines' Shared Instrumentation Facility Liquid Chromatography Mass Spectrometry: RRID:SCR_022052. This work was supported by the Strategic Environmental Research and Development Program (SERDP ER18-1145) and the National Science Foundation Engineering Research Center Program for Reinventing the Nation's Urban Water Infrastructure (ReNUWIt NSF ERC 1028968 Project N3.8).
References
- N. A. Procopio, R. Karl, S. M. Goodrow, J. Maggio, J. B. Louis and T. B. Atherholt, Occurrence and source identification of perfluoroalkyl acids (PFAAs) in the Metedeconk River Watershed, New Jersey, Environ. Sci. Pollut. Res., 2017, 24, 27125–27135 CrossRef CAS PubMed.
- Y. Zushi, T. Takeda and S. Masunaga, Existence of nonpoint source of perfluorinated compounds and their loads in the Tsurumi River basin, Japan, Chemosphere, 2008, 71, 1566–1573 CrossRef CAS PubMed.
- J. J. Sansalone and S. G. Buchberger, Partitioning and First Flush of Metals in Urban Roadway Storm Water, J. Environ. Eng., 1997, 123, 134–143 CrossRef CAS.
- A. Burant, W. Selbig, E. T. Furlong and C. P. Higgins, Trace organic contaminants in urban runoff: Associations with urban land-use, Environ. Pollut., 2018, 242, 2068–2077 CrossRef CAS PubMed.
- M. Murakami and H. Takada, Perfluorinated surfactants (PFSs) in size-fractionated street dust in Tokyo, Chemosphere, 2008, 73, 1172–1177 CrossRef CAS PubMed.
- M. Kotthoff, J. Müller, H. Jürling, M. Schlummer and D. Fiedler, Perfluoroalkyl and polyfluoroalkyl substances in consumer products, Environ. Sci. Pollut. Res., 2015, 22, 14546–14559 CrossRef CAS PubMed.
- P. K. Thai, J. T. McDonough, T. A. Key, J. Thompson, P. Prasad, S. Porman and J. F. Mueller, Release of perfluoroalkyl substances from AFFF-impacted concrete in a firefighting training ground (FTG) under repeated rainfall simulations, Journal of Hazardous Materials Letters, 2022, 3, 100050 CrossRef CAS.
- Y. Zushi and S. Masunaga, First-flush loads of perfluorinated compounds in stormwater runoff from Hayabuchi River basin, Japan served by separated sewerage system, Chemosphere, 2009, 76, 833–840 CrossRef CAS PubMed.
- L. Zhao, M. Zhou, T. Zhang and H. Sun, Polyfluorinated and Perfluorinated Chemicals in Precipitation and Runoff from Cities Across Eastern and Central China, Arch. Environ. Contam. Toxicol., 2013, 64, 198–207 CrossRef CAS PubMed.
- C. Baduel, C. J. Paxman and J. F. Mueller, Perfluoroalkyl substances in a firefighting training ground (FTG), distribution and potential future release, J. Hazard. Mater., 2015, 296, 46–53 CrossRef CAS PubMed.
- B. T. Rushton, Low-Impact Parking Lot Design Reduces Runoff and Pollutant Loads, J. Water Resour. Plan. Manag., 2001, 127, 172–179 CrossRef.
-
US EPA, Fact Sheet: Draft 2022 Aquatic Life Ambient Water Quality Criteria for Perfluorooctanoic acid (PFOA) and Perfluorooctane Sulfonic Acid (PFOS), 2022 Search PubMed.
- R. G. Luthy, S. Sharvelle and P. Dillon, Urban Stormwater to Enhance Water Supply, Environ. Sci. Technol., 2019, 53, 5534–5542 CrossRef CAS PubMed.
- B. C. Crone, T. F. Speth, D. G. Wahman, S. J. Smith, G. Abulikemu, E. J. Kleiner and J. G. Pressman, Occurrence of per- and polyfluoroalkyl substances (PFAS) in source water and their treatment in drinking water, Crit. Rev. Environ. Sci. Technol., 2019, 49, 2359–2396 CrossRef PubMed.
- D. M. Wanninayake, Comparison of currently available PFAS remediation technologies in water: A review, J. Environ. Manage., 2021, 283, 111977 CrossRef CAS PubMed.
- E. C. Porse, Stormwater Governance and Future Cities, Water, 2013, 5, 29–52 CrossRef.
- A. Borthakur, B. K. Cranmer, G. P. Dooley, J. Blotevogel, S. Mahendra and S. K. Mohanty, Release of soil colloids during flow interruption increases the pore-water PFAS concentration in saturated soil, Environ. Pollut., 2021, 286, 117297 CrossRef CAS PubMed.
- F. Dixit, B. Barbeau, S. G. Mostafavi and M. Mohseni, PFOA and PFOS removal by ion exchange for water reuse and drinking applications: role of organic matter characteristics, Environ. Sci.: Water Res. Technol., 2019, 5, 1782–1795 RSC.
- H. McIntyre, V. Minda, E. Hawley, R. Deeb and M. Hart, Coupled photocatalytic alkaline media as a destructive technology for per- and polyfluoroalkyl substances in aqueous film-forming foam impacted stormwater, Chemosphere, 2022, 291, 132790 CrossRef CAS PubMed.
- J. L. Webber, T. Fletcher, R. Farmani, D. Butler and P. Melville-Shreeve, Moving to a future of smart stormwater management: A review and framework for terminology, research, and future perspectives, Water Res., 2022, 218, 118409 CrossRef CAS PubMed.
- L. Hoang and R. A. Fenner, System interactions of stormwater management using sustainable urban drainage systems and green infrastructure, Urban Water J., 2016, 13, 739–758 CrossRef.
- B. A. Ulrich, M. Loehnert and C. P. Higgins, Improved contaminant removal in vegetated stormwater biofilters amended with biochar, Environ. Sci.: Water Res. Technol., 2017, 3, 726–734 RSC.
- M. A. Cruz, J. Xu, J. W. Foppen, S. Pérez, E. Vázquez-Suñé and M. Teixidó, Transport and removal of stormwater vehicle-related mobile organic contaminants in geomedia-amended sand columns, Sci. Total Environ., 2023, 892, 164264 CrossRef CAS PubMed.
- N. Belkouteb, V. Franke, P. McCleaf, S. Köhler and L. Ahrens, Removal of per- and polyfluoroalkyl substances (PFASs) in a full-scale drinking water treatment plant: Long-term performance of granular activated carbon (GAC) and influence of flow-rate, Water Res., 2020, 182, 115913 CrossRef CAS PubMed.
- B. Cantoni, A. Turolla, J. Wellmitz, A. S. Ruhl and M. Antonelli, Perfluoroalkyl substances (PFAS) adsorption in drinking water by granular activated carbon: Influence of activated carbon and PFAS characteristics, Sci. Total Environ., 2021, 795, 148821 CrossRef CAS PubMed.
- K. A. Thompson, K. K. Shimabuku, J. P. Kearns, D. R. U. Knappe, R. S. Summers and S. M. Cook, Environmental Comparison of Biochar and Activated Carbon for Tertiary Wastewater Treatment, Environ. Sci. Technol., 2016, 50, 11253–11262 CrossRef CAS PubMed.
- H. A. Alhashimi and C. B. Aktas, Life cycle environmental and economic performance of biochar compared with activated carbon: A meta-analysis, Resour., Conserv. Recycl., 2017, 118, 13–26 CrossRef.
- A. Boehm, C. D. Bell, N. J. M. Fitzgerald, E. Gallo, C. P. Higgins, T. S. Hogue, R. G. Luthy, A. C. Portmann, B. A. Ulrich and J. M. Wolfand, Biochar-augmented biofilters to improve pollutant removal from stormwater – can they improve receiving water quality?, Environ. Sci.: Water Res. Technol., 2020, 6, 1520–1537 RSC.
- R. A. Tirpak, A. N. Afrooz, R. J. Winston, R. Valenca, K. Schiff and S. K. Mohanty, Conventional and amended bioretention soil media for targeted pollutant treatment: A critical review to guide the state of the practice, Water Res., 2021, 189 DOI:10.1016/j.watres.2020.116648.
- J. M. Wolfand, C. Seller, C. D. Bell, Y.-M. Cho, K. Oetjen, T. S. Hogue and R. G. Luthy, Occurrence of Urban-Use Pesticides and Management with Enhanced Stormwater Control Measures at the Watershed Scale, Environ. Sci. Technol., 2019, 53, 3634–3644 CrossRef CAS PubMed.
- A. C. Portmann, G. H. Lefevre, R. Hankawa, D. Werner and C. P. Higgins, The regenerative role of biofilm in the removal of pesticides from stormwater in biochar-amended biofilters, Environ. Sci.: Water Res. Technol., 2022, 8, 1092–1110 RSC.
- B. A. Ulrich, E. A. Im, D. Werner and C. P. Higgins, Biochar and activated carbon for enhanced trace organic contaminant retention in stormwater infiltration systems, Environ. Sci. Technol., 2015, 49, 6222–6230 CrossRef CAS PubMed.
- J. C. Pritchard, K. M. Hawkins, Y.-M. Cho, S. Spahr, S. D. Struck, C. P. Higgins and R. G. Luthy, Black Carbon-Amended Engineered Media Filters for Improved Treatment of Stormwater Runoff, ACS Environ. Au, 2023, 3(1), 34–46 CrossRef CAS PubMed.
- L. Kim, H. Kang and W. Bae, Treatment of metals from expressway stormwater runoff using zeolite filtration, Desalin. Water Treat., 2010, 19, 97–104 CrossRef CAS.
- X. Xiao, B. A. Ulrich, B. Chen and C. P. Higgins, Sorption of Poly- and Perfluoroalkyl Substances (PFASs) Relevant to Aqueous Film-Forming Foam (AFFF)-Impacted Groundwater by Biochars and Activated Carbon, Environ. Sci. Technol., 2017, 51, 6342–6351 CrossRef CAS PubMed.
- J. A. K. Silva, W. A. Martin, J. L. Johnson and J. E. McCray, Evaluating air-water and NAPL-water interfacial adsorption and retention of Perfluorocarboxylic acids within the Vadose zone, J. Contam. Hydrol., 2019, 223 DOI:10.1016/j.jconhyd.2019.03.004.
- C. E. Schaefer, V. Culina, D. Nguyen and J. Field, Uptake of Poly- and Perfluoroalkyl Substances at the Air–Water Interface, Environ. Sci. Technol., 2019, 53, 12442–12448 CrossRef CAS PubMed.
- C. E. Schaefer, D. Nguyen, E. Christie, S. Shea, C. P. Higgins and J. A. Field, Desorption of Poly- and Perfluoroalkyl Substances from Soil Historically Impacted with Aqueous Film-Forming Foam, J. Environ. Eng., 2021, 147, 06020006 CrossRef CAS.
- H. Sharifan, M. Bagheri, D. Wang, J. G. Burken, C. P. Higgins, Y. Liang, J. Liu, C. E. Schaefer and J. Blotevogel, Fate and transport of per- and polyfluoroalkyl substances (PFASs) in the vadose zone, Sci. Total Environ., 2021, 771, 145427 CrossRef CAS PubMed.
- A. Nickerson, A. C. Maizel, C. E. Schaefer, J. F. Ranville and C. P. Higgins, Effect of geochemical conditions on PFAS release from AFFF-impacted saturated soil columns, Environ. Sci.: Processes Impacts, 2023, 25, 405–414 RSC.
- A. Nickerson, A. E. Rodowa, D. T. Adamson, J. A. Field, P. R. Kulkarni, J. J. Kornuc and C. P. Higgins, Spatial Trends of Anionic, Zwitterionic, and Cationic PFASs at an AFFF-Impacted Site, Environ. Sci. Technol., 2021, 55, 313–323 CrossRef CAS PubMed.
- A. C. Maizel, S. Shea, A. Nickerson, C. Schaefer and C. P. Higgins, Release of Per- and Polyfluoroalkyl Substances from Aqueous Film-Forming Foam Impacted Soils, Environ. Sci. Technol., 2021, 55, 14617–14627 CrossRef CAS PubMed.
- I. Wallis, J. Hutson, G. Davis, R. Kookana, J. Rayner and H. Prommer, Model-based identification of vadose zone controls on PFAS mobility under semi-arid climate conditions, Water Res., 2022, 225, 119096 CrossRef CAS PubMed.
- D. Muschalla, B. Vallet, F. Anctil, P. Lessard, G. Pelletier and P. A. Vanrolleghem, Ecohydraulic-driven real-time control of stormwater basins, J. Hydrol., 2014, 511, 82–91 CrossRef.
- Public Information Map, https://lacounty.maps.arcgis.com/apps/PublicInformation/index.html?appid=cd5ec68b636f4e47bbba5b8e9307be1e, (accessed 8 December 2022).
- O. Gibert, M. Hernández, E. Vilanova and O. Cornellà, Guidelining protocol for soil-column experiments assessing fate and transport of trace organics, Demeau, 2014, 3, 54 Search PubMed.
- S. Hao, Y.-J. Choi, B. Wu, C. P. Higgins, R. Deeb and T. J. Strathmann, Hydrothermal Alkaline Treatment for Destruction of Per- and Polyfluoroalkyl Substances in Aqueous Film-Forming Foam, Environ. Sci. Technol., 2021, 55, 3283–3295 CrossRef CAS PubMed.
- A. E. Rodowa, E. Christie, J. Sedlak, G. F. Peaslee, D. Bogdan, B. DiGuiseppi and J. A. Field, Field Sampling Materials Unlikely Source of Contamination for Perfluoroalkyl and Polyfluoroalkyl Substances in Field Samples, Environ. Sci. Technol. Lett., 2020, 7, 156–163 CrossRef CAS.
- O. US EPA, National Stormwater Calculator, https://www.epa.gov/water-research/national-stormwater-calculator, (accessed 19 July 2023).
- O. US EPA, Compliance Inspection Manual for National Pollutant Discharge Elimination System, https://www.epa.gov/compliance/compliance-inspection-manual-national-pollutant-discharge-elimination-system, (accessed 21 June 2023).
- C. C. Murray, H. Vatankhah, C. A. McDonough, A. Nickerson, T. T. Hedtke, T. Y. Cath, C. P. Higgins and C. L. Bellona, Removal of per- and polyfluoroalkyl substances using super-fine powder activated carbon and ceramic membrane filtration, J. Hazard. Mater., 2019, 366, 160–168 CrossRef CAS PubMed.
- A. Nickerson, A. C. Maizel, P. R. Kulkarni, D. T. Adamson, J. J. Kornuc and C. P. Higgins, Enhanced Extraction of AFFF-Associated PFASs from Source Zone Soils, Environ. Sci. Technol., 2020, 54, 4952–4962 CrossRef CAS PubMed.
- O. US EPA, Method 200.7, https://www.epa.gov/esam/method-2007-determination-metals-and-trace-elements-water-and-wastes-inductively-coupled, (accessed 24 July 2023).
- Y. Chen, H. Zhang, Y. Liu, J. A. Bowden, T. M. Tolaymat, T. G. Townsend and H. M. Solo-Gabriele, Evaluation of per- and polyfluoroalkyl substances (PFAS) in leachate, gas condensate, stormwater and groundwater at landfills, Chemosphere, 2023, 318, 137903 CrossRef CAS PubMed.
- A. C. Ellis, C. J. Liu, Y. Fang, T. H. Boyer, C. E. Schaefer, C. P. Higgins and T. J. Strathmann, Pilot study comparison of regenerable and emerging single-use anion exchange resins for treatment of groundwater contaminated by per- and polyfluoroalkyl substances (PFASs), Water Res., 2022, 223, 119019 CrossRef CAS PubMed.
- Y. J. Choi, D. E. Helbling, J. Liu, C. I. Olivares and C. P. Higgins, Microbial biotransformation of aqueous film-forming foam derived polyfluoroalkyl substances, Sci. Total Environ., 2022, 824, 153711 CrossRef CAS PubMed.
- L. A. D'Agostino and S. A. Mabury, Aerobic biodegradation of 2 fluorotelomer sulfonamide–based aqueous film–forming foam components produces perfluoroalkyl carboxylates, Environ. Toxicol. Chem., 2017, 36, 2012–2021 CrossRef PubMed.
- E. K. Cook, C. I. Olivares, E. H. Antell, S. Yi, A. Nickerson, J. Choi, C. P. Higgins, D. L. Sedlak and L. Alvarez-Cohen, Biological and Chemical Transformation of the Six-Carbon Polyfluoroalkyl Substance N-Dimethyl Ammonio Propyl Perfluorohexane Sulfonamide (AmPr-FHxSA), Environ. Sci. Technol., 2022, 56(22), 15478–15488 CrossRef CAS PubMed.
- J. S.-C. Liou, B. Szostek, C. M. DeRito and E. L. Madsen, Investigating the biodegradability of perfluorooctanoic acid, Chemosphere, 2010, 80, 176–183 CrossRef CAS PubMed.
- K. Prevedouros, I. T. Cousins, R. C. Buck and S. H. Korzeniowski, Sources, Fate and Transport of Perfluorocarboxylates, Environ. Sci. Technol., 2006, 40, 32–44 CrossRef CAS PubMed.
- S.-H. Seo, M.-H. Son, E.-S. Shin, S.-D. Choi and Y.-S. Chang, Matrix-specific distribution and compositional profiles of perfluoroalkyl substances (PFASs) in multimedia environments, J. Hazard. Mater., 2019, 364, 19–27 CrossRef CAS PubMed.
- T. P. Bastow, G. B. Douglas and G. B. Davis, Volatilization Potential of Per- and Poly-fluoroalkyl Substances from Airfield Pavements and during Recycling of Asphalt, Environ. Toxicol. Chem., 2022, 41, 2202–2208 CrossRef CAS PubMed.
- S. Deng, Q. Yu, J. Huang and G. Yu, Removal of perfluorooctane sulfonate from wastewater by anion exchange resins: Effects of resin properties and solution chemistry, Water Res., 2010, 44, 5188–5195 CrossRef CAS PubMed.
- M. L. Brusseau, N. Yan, S. Van Glubt, Y. Wang, W. Chen, Y. Lyu, B. Dungan, K. C. Carroll and F. O. Holguin, Comprehensive retention model for PFAS transport in subsurface systems, Water Res., 2019, 148, 41–50 CrossRef CAS PubMed.
- J. C. Pritchard, Y.-M. Cho, K. M. Hawkins, S. Spahr, C. P. Higgins and R. G. Luthy, Predicting PFAS and Hydrophilic Trace Organic Contaminant Transport in Black Carbon-Amended Engineered Media Filters for Improved Stormwater Runoff Treatment, Environ. Sci. Technol., 2023, 57, 14417–14428 CrossRef CAS PubMed.
- F. Xiao, M. F. Simcik and J. S. Gulliver, Perfluoroalkyl acids in urban stormwater runoff: Influence of land use, Water Res., 2012, 46, 6601–6608 CrossRef CAS PubMed.
- B. K. Biswal, K. Vijayaraghavan, D. L. Tsen-Tieng and R. Balasubramanian, Biochar-based bioretention systems for removal of chemical and microbial pollutants from stormwater: A critical review, J. Hazard. Mater., 2022, 422, 126886 CrossRef CAS PubMed.
|
This journal is © The Royal Society of Chemistry 2024 |
Click here to see how this site uses Cookies. View our privacy policy here.