DOI:
10.1039/D3AN00786C
(Paper)
Analyst, 2023,
148, 4020-4029
Influence of ventilatory parameters on the concentration of exhaled volatile organic compounds in mechanically ventilated patients†
Received
16th May 2023
, Accepted 3rd July 2023
First published on 20th July 2023
Abstract
Analysis of volatile organic compounds (VOC) within exhaled breath is subject to numerous sources of methodological and physiological variability. Whilst breathing pattern is expected to influence the concentrations of selected exhaled VOCs, it remains challenging to investigate respiratory rate and depth accurately in awake subjects. Online breath sampling was performed in 20 mechanically ventilated patients using proton transfer reaction time-of-flight mass spectrometry (PTR-ToF-MS). The effect of variation in respiratory rate (RR) and tidal volume (TV) on the VOC release profiles was examined. A panel of nineteen VOCs were selected, including isoprene, acetone, propofol, volatile aldehydes, acids and phenols. Variation in RR had the greatest influence on exhaled isoprene levels, with maximum and average concentrations being inversely correlated with RR. Variations in RR had a statistically significant impact on acetone, C3–C7 linear aldehydes and acetic acid. In comparison, phenols (including propofol), C8–C10 aldehydes and C3–C6 carboxylic acids were not influenced by RR. Isoprene was the only compound to be influenced by variation in TV. These findings, obtained under controlled conditions, provide useful guidelines for the optimisation of breath sampling protocols to be applied on awake patients.
Introduction
The analysis of volatile organic compounds (VOCs) within exhaled breath has emerged as a viable approach to the non-invasive detection of human disease. Previous studies have characterised the distinct VOCs signals of numerous conditions, including cancers,1 diabetes,2 gastrointestinal disorders,3,4 heart disease,5 liver disease,6 respiratory disease,7 neurogenerative disorders,8 organ transplantation rejection,9,10 oral health problems11 and kidney disease.12
A wide variety of analytical techniques have been employed for disease detection in breath, including mass spectrometry either after chromatographic separation or as direct injection technique,13 ion mobility spectrometry (IMS),14 optical and laser-based spectroscopy,15–17 electronic noses18 and nanomaterial-based gas sensors.19 The analytical technique used and the fraction of breath being sampled will influence the choice of breath sampling method. Breath can be sampled using bags,20 canisters21 or by taking advantage of bespoke solutions either developed in-house or commercial ones.22–24 When using gas chromatography-mass spectrometry (GC-MS), a step of pre-concentration is normally carried out by means of thermal desorption (TD), solid-phase microextraction (SPME) or needle trap devices (NTD).25–27 With direct injection methods, pre-concentration is instead not required.
A prerequisite of any diagnostic test before reaching wider clinical practice is an appreciation of inherent sources of physiological and methodological variability. Such knowledge is important for determining thresholds of (patho)physiological variance and the extent to which standardisation is required. This was exemplified by the discovery that exhaled nitric oxide is significantly influenced by expiratory flow and respiratory rate leading to the creation of international consensus guidelines for its measurement.28
In the context of exhaled VOCs, previous authors have studied the influence of respiratory variables (flow and respiratory rate),29,30 route of exhalation (oral vs. nasal),31–33 body posture,34 and oral cleansing.35 These studies identified that for certain VOCs variation in the conditions of breath sampling had a significant effect on their recorded concentration. A significant challenge faced by studies investigating the effect of respiratory parameters on exhaled VOC levels is the ability to accurately control those parameters in conscious subjects. There have been several previous reports detailing the analysis of exhaled VOCs in anaesthetised and mechanical ventilated patients.36–39 Such conditions offer a unique opportunity to study the effects of ventilatory parameters on VOC concentrations.
The current study aims to investigate the impact of both the rate and depth of breathing pattern on the levels of disease specific VOCs in patients who are anaesthetised and mechanically ventilated. The current study utilises Proton Transfer Reaction Time-of-Flight Mass Spectrometry (PTR-ToF-MS). This analytical technique generates data with high informational content with high time resolution, resulting therefore optimally suited to breath-by-breath online monitoring.
Methods
Study population
All enrolled patients were recruited from Imperial College Healthcare NHS Trust from June 2017 to August 2017. This study was conducted in accordance with the recommendations for physicians involved in research on human subjects adopted by the 18th World Medical Assembly, Helsinki 1964 and later revisions. Regional ethical approval for the study was granted by the Camden and Kings Cross Research Ethics Committee (REC ref: 15/LO/1140). All patients provided informed written consent prior to participation. The study population included both cancer and benign cases, with patients undergoing minor and major as well as open and laparoscopic operations. Intra-abdominal pressure during laparoscopic surgery was maintained throughout all cases at 12–15 mm Hg. Open surgery included gastrectomy from an abdominal approach and oesophagectomy from a right thoraco-laparotomy approach with one lung ventilation during the thoracotomy. Laparoscopic operation included staging laparoscopy, fundoplication, hiatus hernia repair, cholecystectomy and Hellers cardio-myotomy. The details of the study were explained to all eligible patients and fully informed and written consent was obtained prior to enrolment.
Ventilator preparation and general anaesthesia
Before breath sampling, all traces of volatile anaesthetic agents were removed from the ventilation circuit utilising the standard clinical procedure for ventilator preparation in cases of malignant hyperthermia. General anaesthesia was induced with alfentanil and propofol after patients were pre-oxygenated until their expired end-tidal oxygen concentration was over 90%. Total intravenous anaesthesia was maintained thereafter for the duration of the surgery.
VOC analysis
Breath VOC analysis was conducted using a commercial PTR-ToF-MS instrument (PTR-TOF 1000, Ionicon Analytik GmbH, Innsbruck, Austria) equipped with a commercial switch reagent ion (SRI) feature. The SRI feature allows to perform NO+ and O2+-based ionisation in addition to more commonly used H3O+. Optimal conditions for VOC identification and quantification were defined according to a previously described experimental workflow,40 dedicated to method optimization under breath-relevant conditions. Briefly, the workflow consisted of: (i) screening of reduced drift field conditions using different reagent ions, (ii) evaluating the impact of a change in humidity on branching ratios and (iii) gravimetric calibration using permeation or diffusion tubes.
Gravimetric calibration was performed by connecting the PTR-ToF-MS to a permeation unit (Eco Scientific Stroud, Gloucestershire UK). The permeation unit was used to keep different permeation/diffusion tubes at 60 °C, under a constant nitrogen flow and could be connected directly to the PTR-ToF-MS inlet. Since each permeation/diffusion tube had a known, constant diffusion rate (either because the device was supplied in the form of a certified reference standard or because the diffusion rate had been determined experimentally beforehand), the PTR-ToF-MS signal, typically expressed in normalised counts per second (ncps) could be converted into absolute concentrations (parts per billion or ppb).
The sample inlet consisted of a PEEK heated line (temperature 110 °C, length 1.2 m, inner diameter 0.04). Inlet flow rate was set to 40 ml min−1. NO+ was used as the reagent ion. This allowed to exploit different reaction channels for the different compound classes of interest and notably hydride transfer for aldehydes, charge transfer for phenols and adduct formation for ketones and carboxylic acids. Optimal conditions for the drift tube were: temperature 110 °C, pressure 2.30 mbar and voltage 350 V, resulting in an E/N of 84 Td (1 Townsend = 10−17 V cm2). Mass spectra were acquired in the 15–265 range of mass-to-charge ratio (m/z) at a rate of one spectrum per second. Analyte ions used in VOC determination are summarized in Table 1.
Table 1 Target compounds and their key properties, PTR-ToF-MS analyte ions and reaction mechanisms for the compounds measured in the study
Compound |
Log lH2O/gasa |
Bp (°C)b |
Log Pc |
Analyte ion (m/z) |
Product and reaction mechanism |
Log lH2O/gas: water/gas partitioning coefficients (https://www.henrys-law.org/henry/).
bp (°C): boiling points (PubChem database, https://pubchem.ncbi.nlm.nih.gov/).
Log P: octanol/water partitioning coefficients (PubChem database, https://pubchem.ncbi.nlm.nih.gov/).
Mixture of isomers, physical chemical data refer to 2-methyl-phenol.
Mixture of isomers, physical chemical data refer to 2-ethyl-phenol.
|
Isoprene |
−0.49 |
34 |
2.42 |
68.06 |
C5H8 + NO+ → C5H8+ + NO |
Acetone |
2.83 |
56 |
−0.24 |
88.03 |
C3H6O + NO+ → C3H6O [NO]+ |
Propanal |
2.39 |
48 |
0.39 |
57.03 |
C3H6O + NO+ → C3H5O+ + HNO |
Butanal |
2.37 |
75 |
0.88 |
71.04 |
C4H8O + NO+ → C4H7O+ + HNO |
Pentanal |
2.23 |
103 |
1.31 |
85.06 |
C5H10O + NO+ → C5H9O+ + HNO |
Hexanal |
1.90 |
131 |
1.78 |
99.06 |
C6H12O + NO+ → C6H11O+ + HNO |
Heptanal |
1.91 |
153 |
2.29 |
113.11 |
C7H14O + NO+ → C7H13O+ + HNO |
Octanal |
1.72 |
171 |
3.50 |
127.10 |
C8H16O + NO+ → C8H15O+ + HNO |
Nonanal |
1.39 |
191 |
3.27 |
141.10 |
C9H18O + NO+ → C9H17O+ + HNO |
Decanal |
1.03 |
208 |
3.76 |
155.13 |
C10H20O + NO+ → C10H19O+ + HNO |
Phenol |
4.82 |
182 |
1.46 |
94.05 |
C6H6O + NO+ → C6H6O+ + NO |
Methyl-phenold |
4.02 |
202 |
1.94 |
108.05 |
C7H8O + NO+ → C7H8O+ + NO |
Ethyl-phenole |
3.72 |
218 |
2.58 |
122.01 |
C8H10O + NO+ → C8H10O+ + NO |
Propofol |
4.07 |
256 |
3.79 |
178.08 |
C12H18O + NO+ → C12H18O+ + NO |
Acetic acid |
5.00 |
118 |
−0.17 |
90.03 |
C2H4O2 + NO+ → C2H4O2 [NO]+ |
Propanoic acid |
4.57 |
141 |
0.33 |
104.02 |
C3H6O2 + NO+ → C3H6O2 [NO]+ |
Butanoic acid |
4.38 |
164 |
0.79 |
118.03 |
C4H8O2 + NO+ → C4H8O2 [NO]+ |
Pentanoic acid |
4.76 |
186 |
1.39 |
132.01 |
C5H10O2 + NO+ → C5H10O2 [NO]+ |
Hexanoic acid |
4.51 |
202 |
1.92 |
146.03 |
C6H12O2 + NO+ → C6H12O2 [NO]+ |
VOC analysis focused on nineteen mass peaks, assigned to compounds previously associated with states of health and disease (Table 1). Isoprene and acetone were specifically included owing to their status as abundant endogenous breath VOCs. C3–C10 aldehydes, C4–C6 carboxylic acids, phenol, methyl-phenol and ethyl-phenol were selected in view of their importance as putative breath biomarkers of gastric adenocarcinoma41,42 and colorectal adenocarcinoma.43 The panel of volatile fatty acids was further extended to acetic acid and propanoic acid as they are found in elevated concentrations in the headspace of oesophago-gastric adenocarcinoma tissue samples.44 Propofol (2,6-bis(1-methylethyl)-phenol) was used as anaesthetic in all procedures, and was therefore included in the analysis.
Intraoperative breath sampling
Analysis of breath VOCs in response to varying respiratory parameters was performed shortly after induction of general anaesthesia prior to commencing the surgical procedure. Patients’ breath was continuously sampled into the inlet of the PTR-MS instrument via a T-piece placed immediately distal to the endotracheal tube. This set-up was adapted from the method previously developed for intraoperative breath analysis using Selected ion flow tube mass spectrometry (SIFT-MS).36 VOCs were measured under conditions of varying respiratory rate (RR) and tidal volume (TV) according to a defined protocol (Fig. 1). Baseline ventilation settings for all subjects were: FiO2 100%; RR 12 min−1, and TV 8 mL kg−1 calculated using ideal body weight. Ventilator parameters, including respiratory rate and tidal volume, were easily changed by the attending anaesthetist using the ventilator control panel. A 7-minute measurement conducted on a single patient under constant baseline conditions was employed to assess repeatability: measured %RSDs ranged between 2.7% and 21.2% according to the compound (Table S1, ESI†).
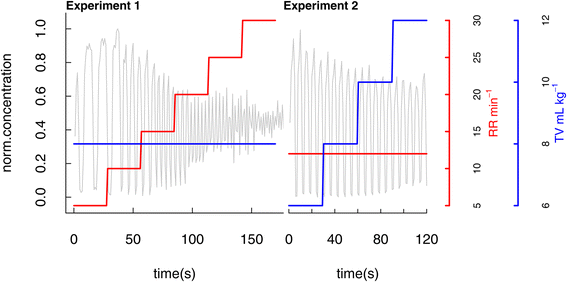 |
| Fig. 1 Schematic representation of the ventilator experimental protocol. Baseline (constant) ventilation settings were: RR 12 min−1 and TV 8 mL kg−1 calculated using ideal body weight. | |
In the first experiment RR was varied while keeping TV constant. Starting from the baseline ventilator settings, RR was first set to 5 min−1 and kept constant for 30 seconds. RR was subsequently increased in stepwise fashion to 10, 15, 20, 25 and 30 min−1 maintaining each rate constant for a 30 seconds period. Settings were then returned to baseline for a minimum of 60 seconds.
In the second experiment, TV was varied while maintaining a constant RR. TV was increased stepwise to 6, 8, 10, and 12 ml kg−1 with each step having a duration of 30 seconds. On completion of these experiments, ventilator settings were returned to baseline and thereafter optimised by the attending consultant anaesthetist.
Analysis of background VOCs within the ventilator circuit was performed at the start of each sampling day. Measurements were made for at least one minute using standard ventilator settings (O2 concentration 100%, tidal volume 550 ml min−1, respiratory rate 12 min−1) with a manual breath bag in the place of the patient's lung.
Data analysis
Data were extracted using PTR-MS viewer version 3.2.2.2 (Ionicon Analytik GmbH, Innsbruck, Austria). Additional data analysis was conducted using in-house generated scripts written using R programming language.45 To obtain a numeric description for each profile, four key parameters were defined for each set of conditions: the maximum (expressed as median intensity of the peak summits), the minimum (median intensity of the peak valleys), the amplitude (difference between maximum and minimum) and the area under the curve (AUC), corresponding to the sum of all values for the time window of interest. To reduce the impact of inter-individual differences, each profile was subjected to a normalisation process whereby the entire profile was rescaled to an arbitrary intensity scale ranging from 0 to 1. A graphical representation of a patient's isoprene profiles with the parameters extracted is reported in Fig. 2. The perusal of the time profile reveals that a few seconds of delay can sometimes be seen in the release pattern with respect to the change of ventilator settings; this was probably due to small operator-related delays in changing ventilator settings.
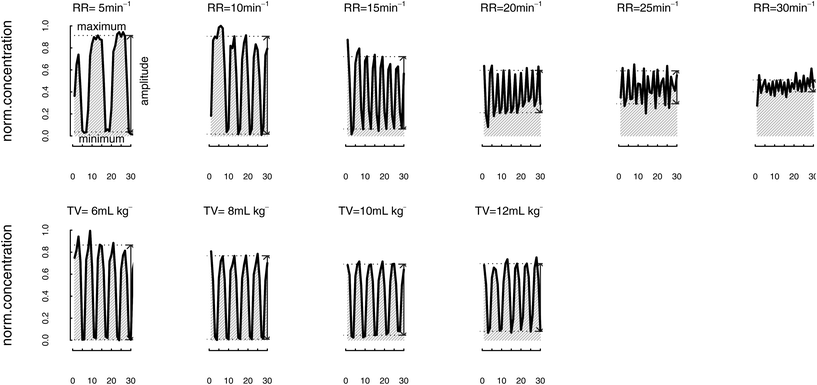 |
| Fig. 2 Breath profiles of isoprene (m/z = 68.06) in patient no.1. Measurements conducted at different values of respiratory rate (RR, top row) and tidal volume (TV, bottom row). Baseline (constant) ventilation settings were: RR 12 min−1 and TV 8 mL kg−1 calculated using ideal body weight. | |
Following a previously published protocol,39 a well-characterised mass peak (m/z 68.06, corresponding to isoprene) was used as ‘tracker’ to highlight the different phases of breathing has already been advised. Isoprene's mass peak was also used as reference for the extraction of breath profile parameters from all other masses. A dedicated R package (IsopreneR) was developed for the processing of the isoprene profiles and the source code is available at GitHub (aromano910/IsopreneR: Functions and packages for data processing in breath analysis (github.com). The ‘breath.tracer’ function, included within the package, allowed to find points corresponding to local maximums and minimums in all time profiles.
One-way ANOVA was conducted using parameter values as dependent variables and either RR or TV as independent variables. Statistical significance was established for p-value < 0.05, following correction for false discovery.46
Results
Intraoperative breath sampling was undertaken in twenty patients. Details of patient characteristics, including disease status and operation type are presented in Table 2.
Table 2 Demographic and clinical information of the study population
Date |
Case no. |
Disease |
Procedure type |
Procedure name |
26/06/17 |
1 |
Cancer |
Laparoscopic |
Staging laparoscopy |
26/06/17 |
2 |
Benign |
Laparoscopic |
Fundoplication |
03/07/17 |
3 |
Cancer |
Open |
Gastrectomy |
11/07/17 |
4 |
Cancer |
Laparoscopic |
Staging laparoscopy |
11/07/17 |
5 |
Cancer |
Laparoscopic |
Staging laparoscopy |
11/07/17 |
6 |
Benign |
Laparoscopic |
Cholecystectomy |
17/07/17 |
7 |
Cancer |
Laparoscopic |
Staging laparoscopy |
17/07/17 |
8 |
Cancer |
Laparoscopic |
Staging laparoscopy |
17/07/17 |
9 |
Benign |
Laparoscopic |
Fundoplication |
17/07/17 |
10 |
Benign |
Laparoscopic |
Oesopagomiotomy |
18/07/17 |
11 |
Cancer |
Laparoscopic |
Staging laparoscopy |
18/07/17 |
12 |
Cancer |
Laparoscopic |
Staging laparoscopy |
18/07/17 |
13 |
Cancer |
Open |
Gastrectomy |
24/07/17 |
14 |
Cancer |
Open |
Oesophagectomy |
25/07/17 |
15 |
Benign |
Laparoscopic |
Cholecystectomy |
25/07/17 |
16 |
Cancer |
Open |
Subtotal gastrectomy |
07/08/17 |
17 |
Cancer |
Open |
Oesophagectomy |
08/08/17 |
18 |
Cancer |
Laparoscopic |
Staging laparoscopy |
08/08/17 |
19 |
Cancer |
Laparoscopic |
Staging laparoscopy |
14/08/17 |
20 |
Benign |
Laparoscopic |
Fundoplication |
The signal of all nineteen VOCs and the corresponding mass peaks, were verified to be significantly lower in the background of the ventilator circuit compared to breath (Table S2, ESI†).
Influence of respiratory rate and tidal volume
Of the nineteen VOCs that were studied, isoprene was the most affected with a significant change in all the assessed metrics (signal amplitude, AUC, maximum and minimum signal) in response to varying RR. Furthermore, only isoprene (signal amplitude) was significantly affected by alteration in TV (Fig. 3). Besides isoprene, eight more VOCs showed a significant change in one or more parameters in response to varying RR. Affected VOCs included acetone, C3–C7 aldehydes, acetic acid and propanoic acid (Tables S3 and S4, ESI†). Other compounds of supposedly endogenous origin (C8–10 aldehydes, C4–6 volatile fatty acid and phenols) as well as the anaesthetic agent propofol were not significantly influenced by changing RR and TV.
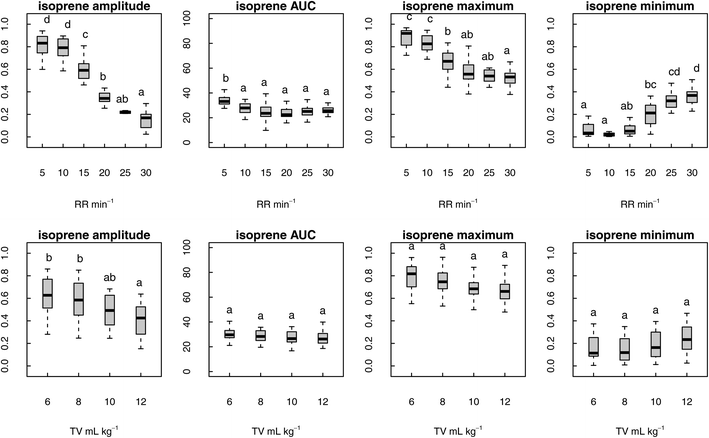 |
| Fig. 3 Variation of isoprene breath profile parameters at different values of RR (top row) and TV (bottom row). Statistically significant differences are established by means of one-way ANOVA, followed by Tukey's post-hoc (letter notation highlights differences between conditions, p < 0.05). | |
The data regarding maximum values obtained for all the nineteen VOCs are summarised in Table 3. Six VOCs showed significant differences in maximum: isoprene, acetone, propanal, pentanal, hexanal and acetic acid. Isoprene (graphically represented in Fig. 3) was the most affected with maximum values reaching the highest level for RR = 5 min−1 and continuously decreasing until RR = 30 min−1.
Table 3 Effect of respiratory rate (RR) on maximum parameters extracted from breath profiles of selected compounds. Statistically significant differences are established by means of one-way ANOVA, followed by Tukey's post-hoc (letter notation highlights differences between conditions, p < 0.05)
Compound |
RR = 5 min−1 |
RR = 10 min−1 |
RR = 15 min−1 |
RR = 20 min−1 |
RR = 25 min−1 |
RR = 30 min−1 |
p-Value |
Isoprene |
0.86 ± 0.11c |
0.81 ± 0.10c |
0.65 ± 0.14b |
0.57 ± 0.10ab |
0.55 ± 0.11ab |
0.53 ± 0.10a |
0.000 |
Acetone |
0.79 ± 0.15b |
0.68 ± 0.18ab |
0.57 ± 0.21a |
0.58 ± 0.14a |
0.60 ± 0.15a |
0.60 ± 0.14a |
0.005 |
Propanal |
0.68 ± 0.20c |
0.60 ± 0.20bc |
0.48 ± 0.15ab |
0.44 ± 0.12a |
0.49 ± 0.10ab |
0.57 ± 0.14ac |
0.001 |
Butanal |
0.57 ± 0.21a |
0.54 ± 0.18a |
0.45 ± 0.13a |
0.45 ± 0.13a |
0.50 ± 0.14a |
0.60 ± 0.16a |
0.372 |
Pentanal |
0.49 ± 0.22ab |
0.52 ± 0.17ab |
0.42 ± 0.14a |
0.40 ± 0.13a |
0.48 ± 0.13ab |
0.61 ± 0.13b |
0.021 |
Hexanal |
0.56 ± 0.15b |
0.53 ± 0.14b |
0.41 ± 0.11a |
0.40 ± 0.10a |
0.38 ± 0.11a |
0.39 ± 0.13a |
0.000 |
Heptanal |
0.46 ± 0.13a |
0.47 ± 0.12a |
0.39 ± 0.08a |
0.43 ± 0.08a |
0.43 ± 0.10a |
0.49 ± 0.08a |
1.000 |
Octanal |
0.53 ± 0.13a |
0.51 ± 0.11a |
0.47 ± 0.12a |
0.47 ± 0.10a |
0.48 ± 0.16a |
0.48 ± 0.11a |
1.000 |
Nonanal |
0.60 ± 0.10a |
0.62 ± 0.10a |
0.57 ± 0.08a |
0.55 ± 0.08a |
0.56 ± 0.07a |
0.54 ± 0.08a |
1.000 |
Decanal |
0.53 ± 0.14a |
0.55 ± 0.12a |
0.51 ± 0.15a |
0.53 ± 0.15a |
0.58 ± 0.15a |
0.62 ± 0.10a |
1.000 |
Acetic acid |
0.56 ± 0.13c |
0.54 ± 0.12bc |
0.49 ± 0.11ac |
0.46 ± 0.09ac |
0.46 ± 0.10ab |
0.43 ± 0.09a |
0.032 |
Propanoic acid |
0.55 ± 0.17a |
0.47 ± 0.16a |
0.46 ± 0.16a |
0.42 ± 0.12a |
0.42 ± 0.12a |
0.39 ± 0.10a |
0.328 |
Butanoic acid |
0.54 ± 0.13a |
0.48 ± 0.12a |
0.41 ± 0.14a |
0.43 ± 0.11a |
0.41 ± 0.11a |
0.40 ± 0.10a |
0.063 |
Pentanoic acid |
0.34 ± 0.16a |
0.28 ± 0.18a |
0.28 ± 0.19a |
0.22 ± 0.19a |
0.25 ± 0.15a |
0.20 ± 0.16a |
1.000 |
Hexanoic acid |
0.33 ± 0.22a |
0.32 ± 0.21a |
0.29 ± 0.21a |
0.28 ± 0.20a |
0.26 ± 0.21a |
0.27 ± 0.16a |
1.000 |
Phenol |
0.44 ± 0.13a |
0.47 ± 0.11a |
0.41 ± 0.15a |
0.41 ± 0.11a |
0.38 ± 0.11a |
0.41 ± 0.07a |
1.000 |
Methyl-phenol |
0.54 ± 0.15a |
0.45 ± 0.18a |
0.47 ± 0.09a |
0.40 ± 0.16a |
0.43 ± 0.13a |
0.42 ± 0.12a |
1.000 |
Ethyl-phenol |
0.28 ± 0.21a |
0.34 ± 0.13a |
0.29 ± 0.19a |
0.30 ± 0.18a |
0.28 ± 0.19a |
0.30 ± 0.15a |
1.000 |
Propofol |
0.52 ± 0.29a |
0.52 ± 0.26a |
0.51 ± 0.28a |
0.53 ± 0.27a |
0.60 ± 0.23a |
0.58 ± 0.25a |
1.000 |
The results relative to the remaining five VOCs are graphically represented in Fig. 4. Like isoprene, acetone, hexanal and acetic acid showed a descending trend in maximum with the progressive increase in RR. For these three VOCs, maximum was significantly higher for RR = 5–10 min−1, stable for RR = 15–25 min−1 and significantly lower for R = 30 min−1 (acetic acid only). Short-chain aldehydes propanal and pentanal showed a different behaviour: the highest concentrations were reached at the two extremes of the assessed RR range, whereas the lowest concentrations were observed for RR = 20 min−1.
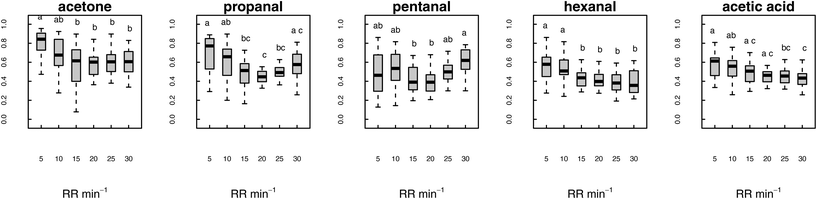 |
| Fig. 4 Effect of respiratory rate (RR) on maximum values extracted from breath profiles of selected compounds. Statistically significant differences are established by means of one-way ANOVA, followed by Tukey's post-hoc (letter notation highlights differences between conditions, p < 0.05). | |
Discussion
This work presents original data, showing the impact of a change in ventilatory parameters on the time-resolved profiles of selected VOCs of clinical interest. For the majority of these VOCs this is the first report of their measurement in patients who are intubated and ventilated. The ability to evaluate effects in patients who are mechanically ventilated offers a unique opportunity to achieve fine control over the rate and depth of subjects breathing that historically has not been possible in awake patients. Furthermore, breath sampling via an endotracheal tube offers a chance to analyse VOCs that are of systemic (endogenous) origin reducing the potential influence of oral and ambient contaminants.
The principal findings of this study were: (i) for half of assessed endogenous VOCs variation of respiratory parameters did not appear to affect signals levels, (ii) with the exception of isoprene, variation in TV had negligible influence on the levels of selected VOCs and; (iii) a variation in RR affected maximum concentrations of six VOCs: of the four extracted parameters, maximum appears to be the most significant as it will correspond to the actual VOC concentration in exhaled breath.
Exhaled isoprene constitutes one of the most studied VOCs in breath research. Isoprene is thought to be linked to cholesterol biosynthesis through the mevalonate pathway and should therefore be ubiquitous in breath. Notwithstanding there are reports of both children47 and adults48 who have undetectable levels of breath isoprene. Isoprene is highly volatile and hydrophobic (log
P = 2.42, bp = 34 °C) with high tendency to partition into the gas phase (log
P = −0.49). A close correlation between blood and alveolar concentrations and a rapid response to a change in breathing and hemodynamic parameters may therefore to be expected. A prompt reduction in end-tidal isoprene levels was observed in response to increased respiratory rate during physical exercise on an ergometer,49 as well as during pulmonary recruitment on mechanically ventilated patients.37,39 In the current study, isoprene signal maximum showed an inverse proportionality to respiratory rate across the whole RR range reflecting a progressively quicker depletion of the airway compartment.
Acetone is also widely studied, due to its high relative abundance in breath, where it is believed to be released as by-product of lipid degradation and ketogenesis. Compared to isoprene, acetone has a relatively high polarity and higher blood
:
air partition coefficient. For acetone the exchange mechanisms are more complex and involve interactions with the airway mucus layer. Our results show that acetone breath profiles are affected by a change in RR. This is in agreement with previous observations.37,49
Aldehydes were studied as they appear to be consistently enriched in the breath of oesophago-gastric and colorectal cancer patients.41–43 In the case of oesophageal adenocarcinoma, a link has also been established between aldehyde accumulation and a deficiency in aldehyde detoxification.50 When evaluating the influence of ventilator parameters, volatile C3–C7 aldehydes are all partially affected by a change in RR. In particular, a decrease in maximum is displayed by propanal, pentanal and hexanal: as observed for isoprene, it is likely that faster rates result in a quicker depletion of the airway compartments. This sensitivity to changes in RR decreases with the reduction in volatility. C8–C10 aldehydes are in comparison not affected: these compounds are the least volatile and might respond less rapidly to a change in ventilation; moreover, for hydrophobic compounds other compartments (endothelial lining, muscle and fat tissue) might have a buffering action on breath VOC levels. For propanal and pentanal a statistically significant increase in maximum has been observed at the highest respiratory rate values. This is in agreement with recent studies carried out on ventilated patients51 and animal models,52 showing a positive correlation between breath pentanal and mechanical ventilation pressure, probably because of an increase in lipid peroxidation and oxidation stress.
Phenols (phenol, methyl-phenol and ethyl-phenol) and C2–C6 carboxylic acids have also been linked to oesophago-gastric cancer.41,42,44 None of these VOCs were significantly impacted by changes in respiratory parameters and this is not surprising considering these compounds are all polar, less volatile and have high blood
:
air partitioning coefficients. The only exception was acetic acid: as seen for other classes of compounds, faster airway depletion when RR is increased is probably favoured by a higher volatility. Since acetic acid has a high blood
:
air partitioning coefficient, exchanges are likely taking place in the airways and not at the alveolar level.
Propofol was also monitored during the experiments due to its relevance for surgical procedures. At the moment, no method is available for online propofol monitoring: the possibility to measure propofol in breath in real time has already been shown and propofol concentrations in blood, blood headspace and breath are correlated.38 The fact that rapid modifications in ventilation parameters has no short-term effects on breath propofol levels would appear to support further research in to the use of breath analysis for propofol monitoring during surgery.
A limitation of this work resides in the choice of one spectrum s−1 as PTR-ToF-MS acquisition speed: a quicker acquisition speed might have provided better definition and more accurate parameter estimation, even though at the expense of sensitivity. It therefore remains unclear whether variation in VOC primarily in response to increasing RR is as a consequence of a true physiological effect relation to their partition between the airways and breath or alternatively as result of limitation in settings. The fact that findings obtained on isoprene and acetone agree with previous findings supports the validity of our approach.
Whilst RR and TV were carefully controlled during this study we did not record exact minute ventilation under experimental conditions. Discrepancy between ventilator setting and the ventilation delivered may have existed. Small sample size and the limited number of VOCs that could be assessed are other acknowledge limitation of this work. Finally, in remains uncertain whether these findings would be the same in situations where breath samples are collected off-line and what effect if any prior breathing pattern may have.
In conclusion, findings presented herein identify that for selected VOC variation in respiratory parameters, principally respiratory rate, can have a significant impact on the levels at which they are detected in breath. Whilst acknowledging that it is not a requirement for all exhaled VOCs, it would nevertheless appear prudent to advocate greater standardisation for breath sampling in future research. Subjects should avoid strenuous activity, be rested prior to sampling and breathe slowly with a resting tidal volume. In awake patients, this could be achieved with the aid of visual or audible cues, such as a metronome.
Author contributions
Andrea Romano: data curation, software, formal analysis, validation, investigation, visualization, writing – original draft. Matyas Fehervari: data curation, investigation, writing – review and editing. Piers Boshier: conceptualisation, supervision, investigation, methodology, project administration, writing – review and editing.
Conflicts of interest
There are no conflicts of interest to declare.
References
- G. B. Hanna, P. R. Boshier, S. R. Markar and A. Romano, Accuracy and Methodologic Challenges of Volatile Organic Compound–Based Exhaled Breath Tests for Cancer Diagnosis: A Systematic Review and Meta-analysis, JAMA Oncol., 2019, 5, e182815, DOI:10.1001/jamaoncol.2018.2815.
- T. D. C. Minh, D. R. Blake and P. R. Galassetti, The clinical potential of exhaled breath analysis for diabetes mellitus, Diabetes Res. Clin. Pract., 2012, 97, 195–205, DOI:10.1016/j.diabres.2012.02.006.
- C. Timms, P. S. Thomas and D. H. Yates, Detection of gastro-oesophageal reflux disease (GORD) in patients with obstructive lung disease using exhaled breath profiling, J. Breath Res., 2012, 6, 016003, DOI:10.1088/1752-7155/6/1/016003.
- K. Dryahina, P. Španěl, V. Pospíšilová, K. Sovová, L. Hrdlička, N. Machková, M. Lukáš and D. Smith, Quantification of pentane in exhaled breath, a potential biomarker of bowel disease, using selected ion flow tube mass spectrometry: Quantification of pentane in exhaled breath, Rapid Commun. Mass Spectrom., 2013, 27, 1983–1992, DOI:10.1002/rcm.6660.
- F. G. Marcondes-Braga, G. L. Batista, F. Bacal and I. Gutz, Exhaled Breath Analysis in Heart Failure, Curr. Heart Failure Rep., 2016, 13, 166–171, DOI:10.1007/s11897-016-0294-8.
- A. De Vincentis, U. Vespasiani-Gentilucci, A. Sabatini, R. Antonelli-Incalzi and A. Picardi, Exhaled breath analysis in hepatology: State-of-the-art and perspectives, World J. Gastroenterol., 2019, 25, 4043–4050, DOI:10.3748/wjg.v25.i30.4043.
- P. Finamore, S. Scarlata and R. A. Incalzi, Breath analysis in respiratory diseases: state-of-the-art and future perspectives, Expert Rev. Mol. Diagn., 2019, 19, 47–61, DOI:10.1080/14737159.2019.1559052.
-
S. Patsiris, A. Karpouza, T. Exarchos and P. Vlamos, Exhaled Breath Analysis in Neurodegenerative Diseases, in Handb. Comput. Neurodegener, ed. P. Vlamos, I. S. Kotsireas and I. Tarnanas, Springer International Publishing, Cham, 2021, pp. 1–12. DOI:10.1007/978-3-319-75479-6_67-1.
- S. M. Studer, J. B. Orens, I. Rosas, J. A. Krishnan, K. A. Cope, S. Yang, J. V. Conte, P. B. Becker and T. H. Risby, Patterns and significance of exhaled-breath biomarkers in lung transplant recipients with acute allograft rejection, J. Heart Lung Transplant., 2001, 20, 1158–1166, DOI:10.1016/S1053-2498(01)00343-6.
- P. A. Sobotka, D. K. Gupta, D. M. Lansky, M. R. Costanzo and E. J. Zarling, Breath pentane is a marker of acute cardiac allograft rejection, J. Heart Lung Transplant., 1994, 13, 224–229 CAS.
- M. Nakhleh, M. Quatredeniers and H. Haick, Detection of halitosis in breath: Between the past, present, and future, Oral Dis., 2018, 24, 685–695, DOI:10.1111/odi.12699.
- B. Grabowska-Polanowska, J. Faber, M. Skowron, P. Miarka, A. Pietrzycka, I. Śliwka and A. Amann, Detection of potential chronic kidney disease markers in breath using gas chromatography with mass-spectral detection coupled with thermal desorption method, J. Chromatogr. A, 2013, 1301, 179–189, DOI:10.1016/j.chroma.2013.05.012.
- R. Su, T. Yang, X. Zhang, N. Li, X. Zhai and H. Chen, Mass spectrometry for breath analysis, TrAC, Trends Anal. Chem., 2023, 158, 116823, DOI:10.1016/j.trac.2022.116823.
- V. Ruzsanyi, J. I. Baumbach, S. Sielemann, P. Litterst, M. Westhoff and L. Freitag, Detection of human metabolites using multi-capillary columns coupled to ion mobility spectrometers, J. Chromatogr. A, 2005, 1084, 145–151, DOI:10.1016/j.chroma.2005.01.055.
- A. Karpf, Y. Qiao and G. N. Rao, Ultrasensitive, real-time trace gas detection using a high-power, multimode diode laser and cavity ringdown spectroscopy, Appl. Opt., 2016, 55, 4497, DOI:10.1364/AO.55.004497.
- T. Tomberg, M. Vainio, T. Hieta and L. Halonen, Sub-parts-per-trillion level sensitivity in trace gas detection by cantilever-enhanced photo-acoustic spectroscopy, Sci. Rep., 2018, 8, 1848, DOI:10.1038/s41598-018-20087-9.
- L. Lechevallier, R. Grilli, E. Kerstel, D. Romanini and J. Chappellaz, Simultaneous detection of C2H6, CH4, and δ13C-CH4 using optical feedback cavity-enhanced absorption spectroscopy in the mid-infrared region: towards application for dissolved gas measurements, Atmos. Meas. Tech., 2019, 12, 3101–3109, DOI:10.5194/amt-12-3101-2019.
- W. Hu, L. Wan, Y. Jian, C. Ren, K. Jin, X. Su, X. Bai, H. Haick, M. Yao and W. Wu, Electronic Noses: From Advanced Materials to Sensors Aided with Data Processing, Adv. Mater. Technol., 2018, 1800488, DOI:10.1002/admt.201800488.
- M. Velumani, A. Prasanth, S. Narasimman, A. Chandrasekhar, A. Sampson, S. R. Meher, S. Rajalingam, E. Rufus and Z. C. Alex, Nanomaterial-Based Sensors for Exhaled Breath Analysis: A Review, Coatings, 2022, 12, 1989, DOI:10.3390/coatings12121989.
- S. Ghimenti, T. Lomonaco, F. G. Bellagambi, S. Tabucchi, M. Onor, M. G. Trivella, A. Ceccarini, R. Fuoco and F. Di Francesco, Comparison of sampling bags for the analysis of volatile organic compounds in breath, J. Breath Res., 2015, 9, 047110, DOI:10.1088/1752-7155/9/4/047110.
- A. B. Lindstrom and J. D. Pleil, A review of the USEPA's single breath canister (SBC) method for exhaled volatile organic biomarkers, Biomarkers, 2002, 7, 189–208, DOI:10.1080/13547500110119750.
- J. Kwak, M. Fan, S. Harshman, C. Garrison, V. Dershem, J. Phillips, C. Grigsby and D. Ott, Evaluation of Bio-VOC Sampler for Analysis of Volatile Organic Compounds in Exhaled Breath, Metabolites, 2014, 4, 879–888, DOI:10.3390/metabo4040879.
- A. Di Gilio, J. Palmisani, G. Ventrella, L. Facchini, A. Catino, N. Varesano, P. Pizzutilo, D. Galetta, M. Borelli, P. Barbieri, S. Licen and G. De Gennaro, Breath Analysis: Comparison among Methodological Approaches for Breath Sampling, Molecules, 2020, 25, 5823, DOI:10.3390/molecules25245823.
- Y. L. Pham, J. Beauchamp, A. Clement, F. Wiegandt and O. Holz, 3D-printed mouthpiece adapter for sampling exhaled breath in medical applications, 3D Print. Med., 2022, 8, 27, DOI:10.1186/s41205-022-00150-y.
- K. Westphal, D. Dudzik, M. Waszczuk-Jankowska, B. Graff, K. Narkiewicz and M. J. Markuszewski, Common Strategies and Factors Affecting Off-Line Breath Sampling and Volatile Organic Compounds Analysis Using Thermal Desorption-Gas Chromatography-Mass Spectrometry (TD-GC-MS), Metabolites, 2023, 13 DOI:10.3390/metabo13010008.
- H. Ma, X. Li, J. Chen, H. Wang, T. Cheng, K. Chen and S. Xu, Analysis of human breath samples of lung cancer patients and healthy controls with solid-phase microextraction (SPME) and flow-modulated comprehensive two-dimensional gas chromatography (GC × GC), Anal. Methods, 2014, 6, 6841, 10.1039/C4AY01220H.
- W. Filipiak, A. Filipiak, C. Ager, H. Wiesenhofer and A. Amann, Optimization of sampling parameters for collection and preconcentration of alveolar air by needle traps, J. Breath Res., 2012, 6, 027107, DOI:10.1088/1752-7155/6/2/027107.
- ATS/ERS Recommendations for Standardized Procedures for the Online and Offline Measurement of Exhaled Lower Respiratory Nitric Oxide and Nasal Nitric Oxide, 2005, Am. J. Respir. Crit. Care Med., 2005, 171, 912–930, DOI:10.1164/rccm.200406-710ST.
- P. R. Boshier, O. H. Priest, G. B. Hanna and N. Marczin, Influence of respiratory variables on the on-line detection of exhaled trace gases by PTR-MS, Thorax, 2011, 66, 919–920, DOI:10.1136/thx.2011.161208.
- P. Sukul, J. K. Schubert, K. Zanaty, P. Trefz, A. Sinha, S. Kamysek and W. Miekisch, Exhaled breath compositions under varying respiratory rhythms reflects ventilatory variations: translating breathomics towards respiratory medicine, Sci. Rep., 2020, 10, 14109, DOI:10.1038/s41598-020-70993-0.
- G. Slingers, R. Goossens, H. Janssens, M. Spruyt, E. Goelen, E. M. Vanden, M. Raes and G. Koppen, Real-time selected ion flow tube mass spectrometry to assess short- and long-term variability in oral and nasal breath, J. Breath Res., 2020, 14, 036006, DOI:10.1088/1752-7163/ab9423.
- P. Sukul, P. Oertel, S. Kamysek and P. Trefz, Oral or nasal breathing? Real-time effects of switching sampling route onto exhaled VOC concentrations, J. Breath Res., 2017, 11, 027101, DOI:10.1088/1752-7163/aa6368.
- T. Wang, A. Pysanenko, K. Dryahina, P. Španěl and D. Smith, Analysis of breath, exhaled via the mouth and nose, and the air in the oral cavity, J. Breath Res., 2008, 2, 037013, DOI:10.1088/1752-7155/2/3/037013.
- P. Sukul, P. Trefz, S. Kamysek, J. K. Schubert and W. Miekisch, Instant effects of changing body positions on compositions of exhaled breath, J. Breath Res., 2015, 9, 047105, DOI:10.1088/1752-7155/9/4/047105.
- B. Vadhwana, I. Belluomo, P. R. Boshier, C. Pavlou, P. Španěl and G. B. Hanna, Impact of oral cleansing strategies on exhaled volatile organic compound levels, Rapid Commun. Mass Spectrom., 2020, 34, e8706, DOI:10.1002/rcm.8706.
- P. R. Boshier, J. R. Cushnir, V. Mistry, A. Knaggs, P. Španěl, D. Smith and G. B. Hanna, On-line, real time monitoring of exhaled trace gases by SIFT-MS in the perioperative setting: a feasibility study, Analyst, 2011, 136, 3233, 10.1039/c1an15356k.
- B. Brock, S. Kamysek, J. Silz, P. Trefz, J. K. Schubert and W. Miekisch, Monitoring of breath VOCs and electrical impedance tomography under pulmonary recruitment in mechanically ventilated patients, J. Breath Res., 2017, 11, 016005, DOI:10.1088/1752-7163/aa53b2.
- C. Hornuss, S. Praun, J. Villinger, A. Dornauer, P. Moehnle, M. Dolch, E. Weninger, A. Chouker, C. Feil, J. Briegel, M. Thiel and G. Schelling, Real-time Monitoring of Propofol in Expired Air in Humans Undergoing Total Intravenous Anesthesia, Anesthesiology, 2007, 106, 665–674, DOI:10.1097/01.anes.0000264746.01393.e0.
- P. Trefz, M. Schmidt, P. Oertel, J. Obermeier, B. Brock, S. Kamysek, J. Dunkl, R. Zimmermann, J. K. Schubert and W. Miekisch, Continuous Real Time Breath Gas Monitoring in the Clinical Environment by Proton-Transfer-Reaction-Time-of-Flight-Mass Spectrometry, Anal. Chem., 2013, 85, 10321–10329, DOI:10.1021/ac402298v.
- A. Romano and G. B. Hanna, Identification and quantification of VOCs by proton transfer reaction time of flight mass spectrometry: An experimental workflow for the optimization of specificity, sensitivity, and accuracy, J. Mass Spectrom., 2018, 53, 287–295, DOI:10.1002/jms.4063.
- S. Kumar, J. Huang, N. Abbassi-Ghadi, H. A. Mackenzie, K. A. Veselkov, J. M. Hoare, L. B. Lovat, P. Španěl, D. Smith and G. B. Hanna, Mass Spectrometric Analysis of Exhaled Breath for the Identification of Volatile Organic Compound Biomarkers in Esophageal and Gastric Adenocarcinoma, Ann. Surg., 2015, 262, 981–990, DOI:10.1097/SLA.0000000000001101.
- S. R. Markar, T. Wiggins, S. Antonowicz, S.-T. Chin, A. Romano, K. Nikolic, B. Evans, D. Cunningham, M. Mughal, J. Lagergren and G. B. Hanna, Assessment of a Noninvasive Exhaled Breath Test for the Diagnosis of Oesophagogastric Cancer, JAMA Oncol., 2018, 4, 970, DOI:10.1001/jamaoncol.2018.0991.
- S. R. Markar, S.-T. Chin, A. Romano, T. Wiggins, S. Antonowicz, P. Paraskeva, P. Ziprin, A. Darzi and G. B. Hanna, Breath Volatile Organic Compound Profiling of Colorectal Cancer Using Selected Ion Flow-tube Mass Spectrometry, Ann. Surg., 2019, 269, 903–910, DOI:10.1097/SLA.0000000000002539.
- M. E. Adam, M. Fehervari, P. R. Boshier, S.-T. Chin, G.-P. Lin, A. Romano, S. Kumar and G. B. Hanna, Mass spectrometry analysis of mixed breath, isolated bronchial breath and gastric endoluminal volatile fatty acids in oesophagogastric cancer, Anal. Chem., 2019, 91(5), 3740–3746, DOI:10.1021/acs.analchem.9b00148.
-
R Core Team, R: A Language and Environment for Statistical Computing, R Foundation for Statistical Computing, Vienna, Austria, 2022. https://www.R-project.org/.
- Y. Benjamini and Y. Hochberg, Controlling the False Discovery Rate: A Practical and Powerful Approach to Multiple Testing, J. R. Stat. Soc., Series B Methodol., 1995, 57, 289–300, DOI:10.1111/j.2517-6161.1995.tb02031.x.
- D. Smith, P. Španěl, B. Enderby, W. Lenney, C. Turner and S. J. Davies, Isoprene levels in the exhaled breath of 200 healthy pupils within the age range 7–18 years studied using SIFT-MS, J. Breath Res., 2010, 4, 017101, DOI:10.1088/1752-7155/4/1/017101.
- P. Sukul, A. Richter, J. K. Schubert and W. Miekisch, Deficiency and absence of endogenous isoprene in adults, disqualified its putative origin, Heliyon, 2021, 7, e05922, DOI:10.1016/j.heliyon.2021.e05922.
- J. King, A. Kupferthaler, K. Unterkofler, H. Koc, S. Teschl, G. Teschl, W. Miekisch, J. Schubert, H. Hinterhuber and A. Amann, Isoprene and acetone concentration profiles during exercise on an ergometer, J. Breath Res., 2009, 3, 027006, DOI:10.1088/1752-7155/3/2/027006.
- S. Antonowicz, Z. Bodai, T. Wiggins, S. R. Markar, P. R. Boshier, Y. M. Goh, M. E. Adam, H. Lu, H. Kudo, F. Rosini, R. Goldin, D. Moralli, C. M. Green, C. J. Peters, N. Habib, H. Gabra, R. C. Fitzgerald, Z. Takats and G. B. Hanna, Endogenous aldehyde accumulation generates genotoxicity and exhaled biomarkers in esophageal adenocarcinoma, Nat. Commun., 2021, 12, 1454, DOI:10.1038/s41467-021-21800-5.
- L. M. Müller-Wirtz, D. Kiefer, J. Knauf, M. A. Floss, J. Doneit, B. Wolf, F. Maurer, D. I. Sessler, T. Volk, S. Kreuer and T. Fink, Differential Response of Pentanal and Hexanal Exhalation to Supplemental Oxygen and Mechanical Ventilation in Rats, Molecules, 2021, 26, 2752, DOI:10.3390/molecules26092752.
- L. M. Müller-Wirtz, D. Kiefer, S. Ruffing, T. Brausch, T. Hüppe, D. I. Sessler, T. Volk, T. Fink, S. Kreuer and F. Maurer, Quantification of Volatile Aldehydes Deriving from In Vitro Lipid Peroxidation in the Breath of Ventilated Patients, Molecules, 2021, 26, 3089, DOI:10.3390/molecules26113089.
|
This journal is © The Royal Society of Chemistry 2023 |
Click here to see how this site uses Cookies. View our privacy policy here.