DOI:
10.1039/D2QI00520D
(Research Article)
Inorg. Chem. Front., 2022,
9, 2462-2469
KNa0.78Eu0.27In3.80B12S12: a novel hexanary thioborate featuring a B12S12 cluster and diverse InSx (x = 4, 5, 6) units†
Received
9th March 2022
, Accepted 13th April 2022
First published on 14th April 2022
Abstract
Thioborates combine the advantages of sulfides and borates, and are a new type of multifunctional inorganic material with versatile structural features. Here, a thioborate KNa0.78Eu0.27In3.80B12S12 crystallizing with a new type of compound was synthesized by a high-temperature flux method. Its structure features a rarely discovered B12S12 cluster and diverse InSx (x = 4, 5, 6) units built in a three-dimensional {[In3.80B12S12]2.6−}∞ polyanionic framework. The structural complexity and novelty are well elaborated. Its optical band gap was determined to be 2.28 eV, and a theoretical calculation of its electronic structure was also performed. This work not only enriches the structural chemistry of chalcogenoborates but also provides a new potential RE–B–Q family (RE = rare-earth metal; Q = S, Se). The latter may produce some interesting functionals.
1. Introduction
The boron element features structural complexity, electron deficiency, unusual bonding situations, and builds a rich boron chemistry.1 Boron and boron-based materials have important applications in modern industry, agriculture, biology, medicine and national defense.2–8 There are many variants of crystalline elemental boron, and all of them are built by the basic building unit B12 icosahedron. Each B12 icosahedron has three B–B σ single bonds and ten three-center two-electron (3c–2e) bonds.9 This specific bonding style yields a rich boron chemistry, in which the closo-polyhedral borane anions have drawn attention to these species as precursors of a new and rapidly expanding field of chemistry enriched with novel applications in contemporary materials research.10–12closo-borane [B12H12]2− is a cluster exhibiting a three-dimensional (3D) aromatic electron delocalization and a remarkable chemical stability.13–15 In contrast to the highly reactive borane with a small molecular weight, [B12H12]2−-based salts are the most stable molecules; this anion survives extended heating in strongly acidic or alkaline solutions.16,17
Attracted by the specialty of B12, many efforts have been made to enrich this special entity.18 Completely saturated with hydrogen, halogens, or even hydroxyl groups, the boron icosahedra can be obtained in solution.10,19–24 All of these bond with B12 to form an anion cluster. Different from this style, B12 icosahedron can also connect with twelve Q (Q = S, Se) atoms to form a unique [B12Q12]14− cluster.25–32 To date, around thirty chalcogenoborates containing B12Q12 cluster have been discovered. In their structures, there are two types of coordination environments around each B12 icosahedron. One is B12 saturated with six BQ3 units to form the [B12(BQ3)6]8− anion,25 and the other is B12 connected with six MQ4 and two M′Q6 units to build a [MB12(M′Q4)3]x− (x = 0, 2; M = Sm, Gd, Ga, In, Sn, Cr; M′ = Ga, In, Sn, Si) open framework.31,33–37 Specifically, the noncentrosymmetric (NCS) ones of the latter show Kleinman-forbidden second-harmonic generation activity.33–36
Chalcogenoborates containing B12Q12 clusters are very rare when compared with those containing B12X12, B12(OH)12 or B12H12 clusters.38–42 Encouraged by the structural diversity for these, it is necessary to explore more B12Q12-based ones to enrich their structural chemistry and explore their application potentials. Based on this consideration, our continuous investigation of the [B12Q12]14−-based system has led to the discovery of a novel hexanary thioborate, KNa0.78Eu0.27In3.80B12S12 (1). Its 3D structure features two types of B12 icosahedron and diverse InSx (x = 4, 5, 6) units, and B12 icosahedra are consolidated by eight or four InSx units, representing a new type of chalcogenoborate. Here, the synthesis, crystal structure, and diverse characterizations are reported.
2. Experimental section
2.1. Synthesis and analyses
A high-temperature solid-state method was used to synthesize the crystals of KNa0.78Eu0.27In3.80B12S12 (1). All the starting materials were used as received without further purification. Considerable efforts were devoted to synthesize 1, and eventually an optimized routine with a high yield was found. For the optimized synthetic route, a 500 mg mixture of Eu2O3 (99.9%), In2O3 (99.9%), S (99.95%) and B (99%) with 1
:
3
:
16
:
12 of Eu
:
In
:
B
:
S, and an additional 400 mg of NaI and 200 mg of KI (99%) as flux, were used as the starting materials, which were hand-ground in an agate mortar for 30 minutes. The obtained mixture was pressed into a pellet and then loaded into an evacuated quartz ampoule with a vacuum degree of 1 × 10−4 Torr. The quartz tube was put in a muffle furnace and heated to 950 °C in 25 h and kept for 7 days, and then cooled down to 300 °C in 5 days. The dark-red crystals of 1 are stable without any change after exposure to air for a few months at room temperature.
A semiquantitative microscopic elemental analysis on the as-prepared single-crystals of 1 was performed on a field-emission scanning electron microscope (FESEM, Zeiss-Supra55) equipped with an energy dispersive X-ray spectroscope (EDS, Bruker, Quantax), which confirmed the presence of K, Na, Eu, In and S. Specifically, the average ratio of In and Eu is 13, close to that of the single-crystal structure analysis, determined as 14.07. The B element could not be detected in view of its light mass (Table S1 and Fig. S1†).
The inductively coupled plasma (ICP) spectroscopy of 1 was measured on an Optima 7300 DV spectrometer. Polytetrafluoroethylene(PTFE) beakers were used to dissolve the powder of 1 and blank samples were also measured to eliminate background effects. The results show that the mass ratios of Eu/In (14.67) and K/Na (1.26) are close to the theoretical values (Eu/In = 14.07 and K/Na = 1.33).
The powder X-ray diffraction measurement of 1 was carried out with a Bruker D8 Advance diffractometer at 40 kV and 100 mA for Cu-Kα radiation (λ = 1.5406 Å) at room temperature. The 2θ range was 10–60° with a scan step width of 2°. The calculated pattern was generated using the Mercury program with its single-crystal structure data. JANA2006 software was employed to perform the Rietveld refinement (Table S2† and Fig. 1).
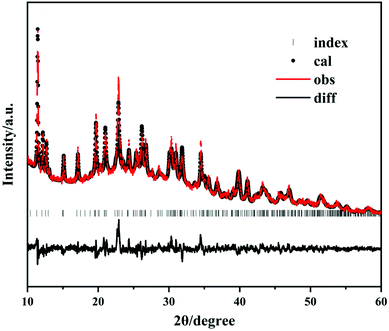 |
| Fig. 1 Rietveld refinement for the powder X-ray diffraction pattern of 1. | |
2.2. Crystal data
Several suitable single crystals of 1 were selected using an optical microscope for the single-crystal X-ray diffraction analysis. The diffraction data were collected by using a graphite-monochromated Mo-Kα radiation (λ = 0.71073 Å) at room temperature on a Bruker D8 QUEST X-ray diffractometer. The structure was solved by direct methods and refined on F2 by full-matrix least-squares methods using the Shelxtl2014 crystallographic software package.43 The crystallographic data are listed in Table 1, and the atom positions and anisotropic displacement parameters (ADP), and bond distances are listed in Tables S3 and S4,† respectively. The CIF document has also been deposited with the CCDC 2086848.†
Table 1 Crystal data and structure refinement parameters for 1
Chemical formula |
KNa0.78Eu0.27In3.80B12S12 |
R1 = ||Fo| − |Fc||/|Fo|.
wR2 = [w(Fo2 − Fc2)2]/[w(Fo2)2]1/2.
|
FW |
1048.21 |
T (K) |
296 |
Crystal system |
Monoclinic |
Space group |
C2/m |
Z
|
4 |
a (Å) |
15.2538(12) |
b (Å) |
10.4468(7) |
c (Å) |
14.6131(10) |
β (°) |
105.036(3) |
V (Å3) |
2248.9(3) |
D
calcd (g cm−3) |
3.096 |
μ (mm−1) |
5.888 |
F(000) |
1930.0 |
Crystal size/mm3 |
0.136 × 0.12 × 0.05 |
2θ range (°) |
4.78 to 50.942 |
Indep. reflns./Rint |
2108/0.0245 |
Data/restraints/parameters |
2108/0/164 |
GOF on F2 |
1.097 |
R1a (I > 2σ(I)) |
0.0306 |
wR2b (all data) |
0.0701 |
Considering that the structure of 1 is heavily disordered, involving partial occupation and spilt sites, it is necessary to make a detailed description about the structure solution process, which can be described as below. First, the EDS analysis result indicates that K, Na, Eu, In and S are the compositional elements in crystals of 1, and all these elements have nonnegligible contents according to the EDS analysis of more than 30 single-crystals. Second, all the B, S, and K sites are fully occupied with reasonable anisotropic displacement factors (ADPs). Third, two of the three In sites are fully occupied, while the third one (In(1)) is partially occupied, and the occupancy was freely refined to 0.80. Fourth, the occupancy of Eu(1) was freely refined to 0.27. Compared with the refinement results for the model with a full occupation of In(1) and Eu(1) atoms, the R1 value reduced from 0.1153 to 0.0492. Fifth, the Na(1) site was split over two neighboring sites, namely, Na(1A) on Wyckoff site m and Na(1B) on Wyckoff site 2/m. The occupancies for these two sites were refined to 0.56 for Na(1A) and 0.44 for Na(1B). The incomplete occupations of Eu and In atoms in other works were also discovered (Table S5†). Combining the above considerations together, the chemical formula of 1 is given as KNa0.78Eu0.27In3.80B12S12.
2.3. UV–vis–NIR diffuse reflectance spectroscopy
The UV-vis-NIR diffuse reflection spectrum was recorded at room temperature using a powder BaSO4 sample as a standard on a computer-controlled Cary 5000 UV–vis–NIR spectrometer equipped with a diffuse reflectance accessory at 200–2500 nm. The spectrum was calculated from the reflection spectrum by the Kubelka–Munk function:44
F(R) = (1 − R)2/(2R) = K/S |
where R is the reflectance, K is the absorption, and S is the scattering. In the (K/S) versus E plot, extrapolating the linear portion of the rising curve to zero gives rise to the onset of absorption.
2.4. Thermal analysis
Differential scanning calorimetry (DSC) analysis on 1 was performed by using a Mettler Toledo thermal analyzer under N2 atmosphere. The powder sample of 1 was put into a crucible and heated to 1000 °C and cooled back to room temperature at a rate of 10 °C min−1.
2.5. Calculation details
The electronic structure calculation, including band structure and density of states (DOS), was performed using the CASTEP module in Material Studio.45 A generalized gradient approximation (GGA) by PBE was adopted to describe the exchange–correlation energy. The electronic configurations for In, B, S, Na, K, and Eu were 5s and 5p, 2s and 2p, 3s and 3p, 3s, 4s, 5s/6s and 4f, respectively, and a cutoff energy of 700 eV was set. The numerical integration of the Brillouin zone was implemented by employing 2 × 2 × 4 Monkhorst–Pack k-point sampling. The Fermi level at 0 eV was chosen as the reference.
3. Results and discussion
3.1. Crystal structure
1 adopts a new monoclinic structure type with the space group C2/m (Pearson code mC119.4). There are three In, eight S, eight B, one K, one Na and one Eu sites in the crystallographically independent unit in the structure of 1 (Fig. 2a). Eight kinds of B atoms comprise two types of B12 icosahedra. For the sake of clarity, each B12 icosahedron is dummied into one sphere. Two B(2), two B(3), four B(4) and four B(7) link together to form a B12 icosahedron (type-I) with the B–B distances in the range 1.768(9)–1.806(8) Å. Meanwhile, two B(1), two B(5), four B(6) and four B(8) build a B12 (type-II) icosahedron, in which the B–B bond lengths are 1.764(8)–1.805(8) Å. In both cases, there are nine types of B–B distances, showing the B–B bond distance dispersity.
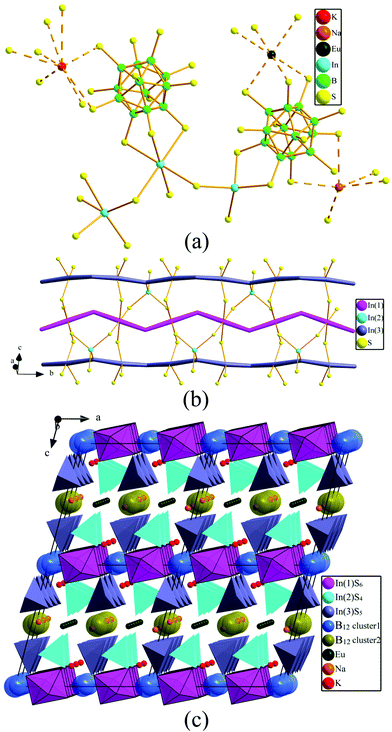 |
| Fig. 2 Crystal structure of 1: (a) Coordination geometry; (b) {[In3.80S12]12.6−}∞ polyanionic ribbons along the b axis; (C) 3D view of the crystal structure along the b direction. | |
Na, K, and Eu metal elements are surrounded with five, eight, and six S atoms to form a NaS5 trigonal bipyramid, KS8 cuboctahedron, and EuS6 octahedron, respectively. Each B12 icosahedron has twelve nearest S atoms to form the B12S12 cluster, in which each type-I B12 icosahedron is surrounded by two InS4 tetrahedra and four InS5 trigonal bipyramids by sharing edges. Whereas each type-II B12 icosahedron is surrounded by four InS6 octahedra through sharing faces, two InS4 tetrahedra and four InS5 trigonal bipyramids by sharing edges. Both types of B12S12 cluster are isolated, and they only connect with InSx polyhedra. The In(1)S6 octahedra and In(3)S5 trigonal bipyramids build two types of zig-zag chains and then connect with In(2)S4 tetrahedra to form {[In3.80S12]12.6−}∞ polyanionic ribbons extending along the b direction (Fig. 2b), which further connect with two types of B12 to build a 3D structure (Fig. 2c).
The simplified 3D structure graph of 1 viewing along the b and c directions is depicted in Fig. 3, where K, Na and Eu atoms are omitted for the sake of clarity, and the B12 icosahedron, In(1)S6 octahedra, In(2)S4 tetrahedra and In(3)S5 trigonal bipyramid are dummied to one atom, namely I-B12, O-In(1)S6, T-In(2)S4, TP-In(3)S5. The connections between the diverse polyhedra can be clearly observed. There are two-types of connections around I-B12, one with four O-In(1)S6, four TP-In(3)S5 and two T-In(2)S4, and the other with four TP-In(3)S5 and two T-In(2)S4. The I-B12 are isolated from each other, and they are connected by InSx polyhedra. Each O-In(1)S6 is surrounded by two I-B12, two T-In(2)S4, and two TP-In(3)S5, while each T-In(2)S4 is surrounded by two I-B12, TP-In(3)S5, and two O-In(1)S6; and each TP-In(3)S5 is surrounded by two I-B12, one T-In(2)S4, and one O-In(1)S6.
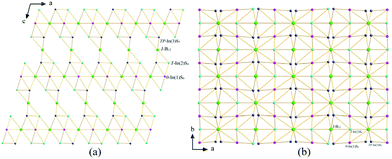 |
| Fig. 3 Simplified open-framework of 1 viewed along the b (a) and c (b) directions. | |
Specifically, the coordination diversity of In atoms is fully exhibited in this structure. In(1), In(2) and In(3) atoms coordinate with six, four and five S atoms to form octahedral In(1)S6, tetrahedral In(2)S4 and trigonal bipyramidal In(3)S5 units. The distances from In to the three equatorial and one of the apical S atoms are short (2.4596(14)–2.5778(9) Å); while the distance to the other apical S atom is considerably longer (2.9409(8) Å) (Fig. S2†). This longer In–S distance is comparable with those in NaIn3S5, Ba18F18In8S21, Fe1.5Pb5.5In10S22, Ca0.76In2.84S5 and La4Ag2In4S13 (Table S6†). In other In-containing chalcogenides, tetrahedral and octahedral coordination geometries are normally found for the In atom; in contrast, examples of trigonal bipyramidal geometry are very few, including but not limited to γ-In2Se3,46 GaxIn2−xSe3,47 BaRE2In2Q7 (Q = S, Se)48 and K5In3P6Se19.49 In general, a structure containing three In coordination geometries simultaneously is very rare. At present, it's only discovered in K5In3P6Se19.49 Hence, 1 is the first chalcogenoborate and the second chalcogenide containing three InSx coordination geometries.
When referring to the different In–S polyhedra, the connection between In and S atoms can be described as a highly distorted In(3)S5 trigonal bipyramid, slightly distorted In(2)S4 tetrahedron and normal In(1)S6 octahedron with gradually reduced calculated dipole moments of 18.8529, 9.2955, and 3.9815 D, respectively. They connect with each other to form {[In3.80S12]12.6−}∞ polyanionic ribbons (Fig. 2b), which represent a new In–S slab. Differently, the three InSex coordination geometries in K5In3P6Se19 are connected with each other by Se(1) atoms to form the [In3Se13]17− anion (Fig. S2†).49
Differently from the arrangement of other B12Q12 compounds,25,34 the B12S12 clusters in 1 are arranged in a cubic closest-packed manner with the center to center distance within 8.987 to 11.857 Å (Fig. S3†).50 The cluster anions exhibit their centers of gravity at the Wyckoff positions 2a (for type-I B12) and 2d (for type-II B12), with distances of almost 1.7 Å to the twelve boron atoms, providing them an inner diameter of 3.4 Å, which is close to that of other inorganic compounds containing B12 clusters (Table 2) and indicates the high stability of B12 clusters.
Table 2 Different B12 clusters in known chalcogenoborates
Compound |
d (B–B)/Å |
d (C–B)/Å |
ID |
The CE of B12 |
Note: d (C–B), ID and CE represent the average values of the B12 gravity's center to B atoms, inner diameter of B12, and chemical environments of B12, respectively. |
K3Cl[InB12(InSe4)3] 36 |
1.79 |
1.70 |
3.40 |
6[InSe4], 4[InSe6] |
Ag2B12Cl12 50 |
1.78 |
1.70 |
3.40 |
6[AgCl6] |
Sn4B12Se15 31 |
1.79 |
1.70 |
3.40 |
6[SnSe4], 2[SnSe6] |
Cs8B12(BSe3)6 25 |
1.78 |
1.70 |
3.39 |
6[BSe3] |
1 (type-I B12) |
1.79 |
1.71 |
3.41 |
4[InS5], 2[InS4] |
1 (type-II B12) |
1.79 |
1.70 |
3.40 |
4[InS6], 2[InS4], 4[InS5] |
As far as we know, there are two-types of connection around B12 icosahedra: one is surrounded by six BS3 units to form [B12(BS3)6]8− polyanion (Fig. 4a), while the other one is connected with two InS6 and six InS4 units to construct the [In8B12S30]26− polyanion (Fig. 4b).26,35 Different from these two styles, the B12 icosahedron in this work adopts a new connection mode with diverse InSx units to form the [In7.1B12S29]26.7− anion in 1 (Fig. 4c). Among the two known structure types, a family of multinary (A3X)[MB12(M′Q4)3] (A = alkali metal; X = halogen element; M = Ga, In, rare-earth metal; M′ = Ga, In) compounds have the maximum relevance to the structure of 1.33–36 (K3I)[InB12(InS4)3] crystalizes in the NCS hexagonal space group P6322 featuring a 3D honeycomb-like open-framework, providing channels occupied by face-sharing IK6 octahedral chains.35 Accordingly, 1 features {[In3.80S12]12.6−}∞ polyanionic ribbons constructed by diverse InSx units, which further connect with two types of B12 icosahedra to form the 3D structure (Fig. 4c). Unlike 1 with two types of B12 icosahedra, (K3I)[InB12(InS4)3] has only one type of B12 icosahedron with two unique B atoms, and each B12 icosahedron is surrounded by two In(1)S6 octahedra via sharing faces and six In(2)S4 tetrahedra via sharing edges (Fig. 4b and c).
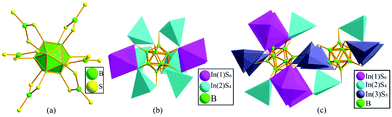 |
| Fig. 4 The chemical environment around one B12 icosahedron: [B12(BS3)6]8− (a); [In8B12S30]26− (b); [In7.1B12S29]26.7− (c). | |
The In(1)–S bond distances in the InS6 unit are 2.680(6) Å and the In(2)–S bond distances in the InS4 unit are 2.399(6)–2.492(7) Å in (K3I)[InB12(InS4)3], indicating that In(1)S6 octahedra are normal and centrosymmetric (CS). In(1)S6 octahedra arrange in a parallel manner and In(2)S4 tetrahedra settle orderly at a certain angle. Although all the coordination polyhedra in 1 are distorted, it crystallizes in the CS structure, since all the InSx units in its structure stack in a back-to-back style, which cancels out their hyperpolarizabilities.51,52 Their NCS/CS structures can also be explained by analyzing their structural symmetry. Considering their unit-cell centers, the In atom coordinates with four S atoms to form a highly distorted InS4 tetrahedron in (K3I)[InB12(InS4)3] with side lengths of 3.854–4.181 Å, whereas in the symmetric center of 1, Na and S atoms connect with each other to form two parallelograms with Na–S distances of 3.261(6) and 3.007(5) Å (Fig. 5).
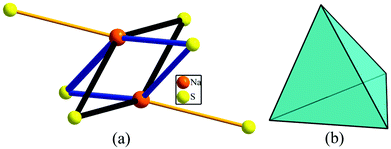 |
| Fig. 5 The center of the unit cell: (a) parallelograms formed by Na and S in 1; (b) highly distorted InS4 tetrahedron in (K3I)[InB12(InS4)3]. | |
3.2. Optical properties
The optical band gap of 1 was determined to be approximately 2.26 eV from the result of UV-vis-NIR diffuse-reflectance spectroscopy (Fig. 6), which is consistent with its dark-red color.
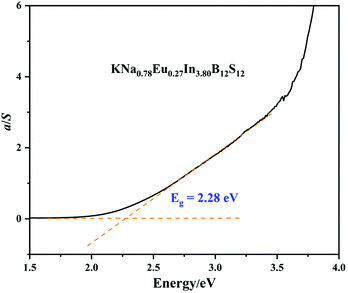 |
| Fig. 6 UV–vis–NIR diffuse reflectance spectrum of 1. | |
3.3. Thermal behavior
The differential scanning calorimetry (DSC) result shows that it undergoes melting upon heating and crystallization upon cooling events (Fig. 7).53,54 The inherently high kinetic stability of the B12 icosahedron makes it resistant to decomposition reactions at elevated temperatures, and it is thermally stable up to 739 °C.
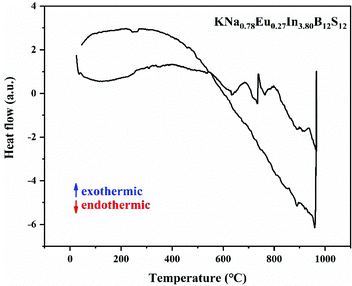 |
| Fig. 7 DSC curve for 1 measured from room temperature to 1000 °C under a N2 atmosphere. | |
3.4. Theoretical calculations
To better understand its structure, an electronic structure calculation for 1 was performed. The calculated band gap of 1 is 1.08 eV (Fig. 8a). Usually, the calculated band gap is underestimated compared with its experimental one. The density of states (DOS) calculation was carried out to observe the orbitals’ contribution near the Fermi level (Fig. 8b). Total and Partial DOS show that the top of the valence band is mainly composed of S-3p and minor B-2p orbitals, while the bottom of the conduction band is primarily constituted of In-5s and S-3p orbitals, suggesting that charge transfer between the frontier orbitals is primarily from S 3p to In 5s orbitals. Recalling the case for (K3I)[InB12(InS4)3],34 similar calculation results are available and also verify that these calculations are reliable.
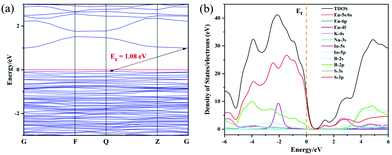 |
| Fig. 8 Calculated electronic structure, including band structure (a) and DOS (b). 0 eV is selected as the Fermi level. | |
4. Conclusion
In summary, a novel hexanary thioborate KNa0.78Eu0.27In3.80B12S12 (1) was obtained and systematically studied. Its structure features an unprecedented {[In3.80S12]12.6−}∞ polyanionic ribbon, two types of B12 icosahedra, and diverse InSx units. 1 is the first thioborate and the second chalcogenide to include InS4, InS5 and InS6 polyhedra in one structure. This work not only provides a novel type of thioborate with a special structure, but also enriches both B and In chemistry. Next, it is proposed to explore its potential structural tolerance for diverse derivatives and physical properties. We hope this work can evoke increasing interest on chalcogenoborates, which really represents an amazing while insufficiently explored material system.
Conflicts of interest
There are no conflicts to declare.
Acknowledgements
The authors would like to acknowledge the financial support by the national natural science foundation of China (21771159, 22071212), and the Qinglan project from Yangzhou University.
References
- B. Albert and H. Hillebrecht, Boron: Elementary Challenge for Experimenters and Theoreticians, Angew. Chem., Int. Ed., 2009, 48, 8640–8668 CrossRef CAS PubMed.
- Y. Chi, J. Xu, H. G. Xue, Y. P. Zhang, X. L. Chen, M. H. Whangbo, S. P. Guo and S. Q. Deng, Triple-Kagome-Layer Slabs of Mixed-Valence Rare-Earth Ions Exhibiting Quantum Spin Liquid Behaviors: Synthesis and Characterization of Eu9MgS2B20O41, J. Am. Chem. Soc., 2019, 141, 9533–9536 CrossRef CAS PubMed.
-
N. S. Hosmane, Boron Science: New Technologies and Applications, CRC Press, 2011 Search PubMed.
- Y. Chi, H. G. Xue and S. P. Guo, Designing Sulfide Borate as a Novel Type of Second-Order Nonlinear-Optical Material, Inorg. Chem., 2020, 59, 1547–1555 CrossRef CAS PubMed.
- Z. H. Zhang, E. S. Penev and B. I. Yakobson, Two-Dimensional Boron: Structures, Properties and Applications, Chem. Soc. Rev., 2017, 46, 6746–6763 RSC.
- F. Ali, N. S. Hosmane and Y. Zhu, Boron Chemistry for Medical Applications, Molecules, 2020, 25, 828–852 CrossRef CAS PubMed.
- Y. Zhu and N. S. Hosmane, Recent Advances and Applications in Boron Chemistry, Coord. Chem. Rev., 2015, 293, 357–367 CrossRef.
- Z. H. Shi, Y. Chi, M. Yang, W. L. Liu and S. P. Guo, A Series of Chalcogenide Borates RE6Ta2MgQB8O26 (RE = Sm, Eu, Gd; Q = S, Se) Featuring a B4O10 Cluster, Inorg. Chem., 2020, 59, 3532–3536 CrossRef CAS PubMed.
- M. Fujimori, T. Nakata, T. Nakayama, E. Nishibori, K. Kimura, M. Takata and M. Sakata, Peculiar Covalent Bonds in α-Rhombohedral Boron, Phys. Rev. Lett., 1999, 82, 4452–4455 CrossRef CAS.
- T. Peymann, C. B. Knobler, S. I. Khan and M. F. Hawthorne, Dodecahydroxy-closo-Dodecaborate(2−), J. Am. Chem. Soc., 2001, 123, 2182–2185 CrossRef CAS PubMed.
- O. Conrad, C. Jansen and B. Krebs, Boron-Sulfur and Boron-Selenium Compounds-From Unique Molecular Structural Principles to Novel Polymeric Materials, Angew. Chem., Int. Ed., 1998, 37, 3208–3218 CrossRef PubMed.
- W. L. Li, X. Chen, T. Jian, T. T. Chen, J. Li and L. S. Wang, From Planar Boron Clusters to Borophenes and Metalloborophenes, Nat. Rev. Chem., 2017, 1, 0071–0080 CrossRef CAS.
-
M. F. Hawthorne, Advances in Boron Chemistry: Special Publication No. 201, Royal Society of Chemistry, London, 1997 Search PubMed.
- J. J. Aihara, Three-Dimensional Aromaticity of Polyhedral Boranes, J. Am. Chem. Soc., 1878, 100, 3339–3342 CrossRef.
- R. B. King, Three-Dimensional Aromaticity in Polyhedral Boranes and Related Molecules, Chem. Rev., 2001, 101, 1119–1152 CrossRef CAS PubMed.
- E. L. Muetterties, J. H. Balthis, Y. T. Chia, W. H. Knoth and H. C. Miller, Chemistry of Boranes. VIII. Salts and Acids of B10H102− and B12H122−, Inorg. Chem., 1964, 3, 444–451 CrossRef CAS.
- A. A. Kamin and M. A. Juhasz, Exhaustive Cyanation of the Dodecaborate Dianion: Synthesis, Characterization, and X-ray Crystal Structure of [B12(CN)12]2−, Inorg. Chem., 2020, 59, 189–192 CrossRef CAS PubMed.
- I. B. Sivaev, V. I. Bregadze and S. Sjöberg, Chemistry of closo-Dodecaborate Anion [B12H12]2−: A Review, Collect. Czech. Chem. Commun., 2002, 67, 679–727 CrossRef CAS.
- T. Peymann, A. Herzog, C. B. Knobler and M. F. Hawthorne, Aromatic Polyhedral Hydroxyborates: Briding Boron Oxides and Boron Hydrides, Angew. Chem., Int. Ed., 1999, 38, 1061–1064 CrossRef CAS PubMed.
- I. Tiritiris and T. Schleid, Single Crystals of the Dodecaiodo-closo-Dodecaborate Cs2[B12I12]·2CH3CN (≡Cs-(NCCH3)2[B12I12]) from Acetonitrile, Z. Anorg. Allg. Chem., 2001, 627, 2568–2570 CrossRef.
- E.
V. Bukovsky, D. V. Peryshkov, H. Wu, W. Zhou, W. S. Tang, W. M. Jones, V. Stavila, T. J. Udovic and S. H. Strauss, Comparison of the Coordination of B12F122−, B12Cl122−, and B12H122− to Na+ in the Solid State: Crystal Structures and Thermal Behavior of Na2(B12F12), Na2(H2O)4(B12F12), Na2(B12Cl12), and Na2(H2O)6(B12Cl12), Inorg. Chem., 2017, 56, 4369–4379 CrossRef CAS PubMed.
- M. Mayer, M. Rohdenburg, V. van Lessen, M. C. Nierstenhöfer, E. Aprà, S. Grabowsky, K. R. Asmis, C. Jenne and J. Warneke, First Steps Towards a Stable Neon Compound: Observation and Bonding Analysis of [B12(CN)11Ne]−, Chem. Commun., 2020, 56, 4591–4594 RSC.
- R. T. Boeré, J. Derendorf, C. Jenne, S. Kacprzak, M. Keßler, R. Riebau, S. Riedel, T. L. Roemmele, M. Rühle, H. Scherer, T. Vent-Schmidt, J. Warneke and S. Weber, On the Oxidation of the Three-Dimensional Aromatics [B12X12]2− (X=F, Cl, Br, I), Chem. – Eur. J., 2014, 20, 4447–4459 CrossRef PubMed.
- H. F. T. Klare and M. Oestreich, Silylium Ions in Catalysis, Dalton Trans., 2010, 39, 9176–9184 RSC.
- J. Küper, O. Conrad and B. Krebs, Selenoboratoborates [B12(BSe3)6]8−: A New Class of Chalcogen-Substituted Icosahedral Boron Clusters, Angew. Chem., Int. Ed. Engl., 1997, 36, 1903–1904 CrossRef.
- J. Kuchinke, A. Hammerschmidt and B. Krebs, Rb8[B12(BS3)6] and Cs8[B12(BS3)6]: The First Thioborato-closo-Dodecaborates, Solid State Sci., 2003, 5, 189–196 CrossRef CAS.
- A. Hammerschmidt, A. Lindemann, C. Köster and B. Krebs, Na6[B18Se17]: The First Selenoborato-closo-Dodecaborate with a Polymeric Chain Anion, Z. Anorg. Allg. Chem., 2001, 627, 2121–2126 CrossRef CAS.
- A. Hammerschmidt, A. Lindemann, M. Döch and B. Krebs, A Novel Selenoborato-closo-Dodecaborate with Different Anion Substitution Patterns, Z. Anorg. Allg. Chem., 2003, 629, 1249–1255 CrossRef CAS.
- A. Hammerschmidt, M. Döch, S. Pütz and B. Krebs, Na8[B12(BSe3)6]: A Novel Selenoborato-closo-Dodecaborate, Z. Anorg. Allg. Chem., 2004, 630, 2299–2303 CrossRef CAS.
- A. Hammerschmidt, M. Döch, S. Pütz and B. Krebs, Na2[B18Se16]: The First 3D Polymeric Selenoborato-closo-Dodecaborate, Z. Anorg. Allg. Chem., 2005, 631, 1125–1128 CrossRef CAS.
- D. G. Chica, I. Spanopoulos, S. Hao, C. Wolverton and M. G. Kanatzidis, Sn4−δ,B12Se12[Qx], Q = Se, Te, a B12 Cluster Tunnel Framework Hosting Neutral Chalcogen Chains, Chem. Mater., 2021, 33, 1723–1730 CrossRef CAS.
- A. Hammerschmidt, M. Döch, A. Lindemann, S. Pütz and B. Krebs, Sodium Selenoborato-closo-Dodecaborates: Structures and Properties, Phys. Chem. Glasses: Eur. J. Glass Sci. Technol., Part B, 2006, 47, 377–380 CAS.
- S. P. Guo, G. C. Guo, M. S. Wang, J. P. Zou, H. Y. Zeng, L. Z. Cai and J. S. Huang, A Facile Approach to Hexanary Chalcogenoborate Featuring a 3-D Chiral Honeycomb-Like Open-framework Constructed from Rare-Earth Consolidating Thiogallate-closo-Dodecaborate, Chem. Commun., 2009, 4366–4368 RSC.
- S. P. Guo, Y. Chi, B. W. Liu and G. C. Guo, Synthesis, Crystal Structure and Second-Order Nonlinear Optical Property of a Novel Pentanary Selenide (K3I)[InB12(InSe4)]3, Dalton Trans., 2016, 45, 10459–10465 RSC.
- Z. D. Sun, Y. Chi, H. G. Xue and S. P. Guo, A Series of Pentanary Inorganic Supramolecular Sulfides (A3X)[MB12(MS4)3] (A = K, Cs; X = Cl, Br, I; M = Ga, In, Gd) Featuring B12S12 Clusters, Inorg. Chem. Front., 2017, 4, 1841–1847 RSC.
- S. S. Han, W. D. Yao, S. X. Yu, Y. Sun, A. Gong and S. P. Guo, Salt-Inclusion Chalcogenoborates Containing a B12Q12 (Q = S, Se) Cluster Exhibiting a Kleinman-Forbidden Frequency-Doubling Effect, Inorg. Chem., 2021, 60, 3375–3383 CrossRef CAS PubMed.
- M. Sugimori, H. Fukuoka, H. Imoto and T. Saito, Preparation and Crystal Structure of Chromium Silicon Selenide Containing a B12 Icosahedron with a Tunnel Structure, J. Organomet. Chem., 2000, 611, 547–550 CrossRef CAS.
- H. Lin, W. B. Wei, H. Chen, X. T. Wu and Q. L. Zhu, Ration Design of Infrared Nonlinear Optical Chalcogenides by Chemical Substitution, Coord. Chem. Rev., 2020, 406, 213150–213173 CrossRef CAS.
- M. Y. Ran, Z. J. Ma, H. Chen, B. X. Li, X. T. Wu, H. Lin and Q. L. Zhu, Partial Isovalent Anion Substitution to Access Remarkable Second-Harmonic Generation Response: a Generic and Effective Strategy for Design of Infrared Nonlinear Optical Materials, Chem. Mater., 2020, 32, 5890–5896 CrossRef CAS.
- M. M. Chen, Z. Ma, B. X. Li, W. B. Wei, X. T. Wu, H. Lin and Q. L. Zhu, M2As2Q5 (M = Ba, Pb; Q = S, Se): A Source of Infrared Nonlinear Optical Materials with Excellent Overall Performance Activated by Multiple Discrete Arsenate Anions, J. Mater. Chem. C, 2021, 9, 1156–1163 RSC.
- H. Chen, W. B. Wei, H. Lin and X. T. Wu, Transition-metal-based chalcogenides: A rich source of infrared nonlinear optical materials, Coord. Chem. Rev., 2021, 448, 214154–214192 CrossRef CAS.
- M. M. Chen, S. H. Zhou, W. B. Wei, X. T. Wu and Q. L. Zhu, Phase Matchability Transformation in the Infrared Nonlinear Optical Materials with Diamond-Like Frameworks, Adv. Opt. Mater., 2021, 10, 2102123–2102131 CrossRef.
- O. V. Dolomanov, L. J. Bourhis, R. J. Gildea, J. A. K. Howard and H. Puschmann, OLEX2: A Complete Structure Solution, Refinement and Analysis Program, J. Appl. Crystallogr., 2009, 42, 339–341 CrossRef CAS.
-
(a)
W. W. Wendlandt and H. G. Hecht, Reflectance pectroscopy, Interscience Publishers, New York, 1966 Search PubMed;
(b)
G. Kortüm, Reflectance Spectroscopy, Springer, 1969 CrossRef.
- S. J. Clark, M. D. Segall, C. J. Pickard, P. J. Hasnip, M. I. Probert, K. Refson and M. C. Payne, First Principles Methods Using CASTEP, Z. Kristallogr. – Cryst. Mater., 2005, 220, 567–570 CrossRef CAS.
- Y. Chi, Z. D. Sun, Q. T. Xu, H. G. Xue and S. P. Guo, Hexagonal In2Se3: A Defect Wurtzite-Type Infrared Nonlinear Optical Material with Moderate Birefringence Contributed by Unique InSe5 Unit, ACS Appl. Mater. Interfaces, 2020, 12, 17711–17717 Search PubMed.
- Q. T. Xu, Y. Chi, X. H. Li, W. L. Liu and S. P. Guo, Structural Chemistry and Excellent Nonlinear Optical Properties of a Series of Ternary Selenides Gax,In2−xSe3, Inorg. Chem., 2021, 61, 431–438 CrossRef PubMed.
- W. L. Yin, A. K. Iyer, X. Lin, C. Li, J. Y. Yao and A. Mar, A. Quaternary Chalcogenides BaRE2In2Ch7 (RE = La-Nd; Ch = S, Se) Containing InCh5 Trigonal Bipyramids, Dalton Trans., 2016, 45, 12329–12337 RSC.
- A. Rothenberger, H. H. Wang, D. Chung and M. G. Kanatzidis, Structural Diversity by Mixing Chalcogen Atoms in the Chalcophosphate System K/In/P/Q (Q = S, Se), Inorg. Chem., 2010, 49, 1144–1151 CrossRef CAS PubMed.
- I. Tiritiris and T. Schleid, The Crystal Structure of Solvent-Free Silver Dodecachloro-closo-Dodecaborate Ag2[B12Cl12] from Aqueous Solution, Z. Anorg. Allg. Chem., 2003, 629, 581–583 CrossRef CAS.
- B. W. Liu, X. M. Jiang, H. Y. Zeng and G. C. Guo, [ABa2Cl][Ga4S8] (A = Rb, Cs): Wide-Spectrum Nonlinear Optical Materials Obtained by Polycation-Substitution-Induced Nonlinear Optical (NLO)-Functional Motif Ordering, J. Am. Chem. Soc., 2020, 142, 10641–10645 CrossRef CAS PubMed.
- Z. T. Lu, Z. D. Sun, Y. Chi, H. G. Xue and S. P. Guo, Balanced Second-Order Nonlinear Optical Properties of Adducts CHI3·(S8)3 and AsI3·(S8)3: A Systematic Survey, Inorg. Chem., 2019, 58, 4619–4625 CrossRef CAS PubMed.
- B. W. Liu, H. Y. Zeng, X. M. Jiang, G. E. Wang, S. F. Li, L. Xu and G. C. Guo, [A3X][Ga3PS8] (A = K, Rb; X = Cl, Br): Promising IR Non-Linear Optical Materials Exhibiting Concurrently Strong Second-Harmonic Generation and High Laser Induced Damage Thresholds, Chem. Sci., 2016, 7, 6273–6277 RSC.
- J. N. Li, W. D. Yao, X. H. Li, W. L. Liu, H. G. Xue and S. P. Guo, A Novel Promising Infrared Nonlinear Optical Selenide KAg3Ga8Se14 Designed from Benchmark AgGaQ2 (Q = S, Se), Chem. Commun., 2021, 57, 1109–1112 RSC.
Footnote |
† Electronic supplementary information (ESI) available: Crystal data tables, crystal photographs, EDS and other tables. CCDC 2086848. For ESI and crystallographic data in CIF or other electronic format see DOI: https://doi.org/10.1039/d2qi00520d |
|
This journal is © the Partner Organisations 2022 |
Click here to see how this site uses Cookies. View our privacy policy here.