Spiers Memorial Lecture: CO2 utilization: why, why now, and how?
Received
22nd March 2021
, Accepted 12th April 2021
First published on 26th April 2021
Abstract
This introduction to the Faraday Discussion on carbon dioxide utilization (CDU) provides a framework to lay out the need for CDU, the opportunities, boundary conditions, potential pitfalls, and critical needs to advance the required technologies in the time needed. CDU as a mainstream climate-relevant solution is gaining rapid traction as measured by the increase in the number of related publications, the investment activity, and the political action taken in various countries.
Introduction
The need for carbon dioxide utilization
Decisive, rapid, and large-scale action is needed to address the escalating negative impacts of climate change. Massive efforts are required to deploy technologies, policies, and business models that quickly reduce the further release of carbon dioxide (CO2) into the atmosphere at large scales.1 Taking the post-2050 view for humanity, there will be processes where the formation and possible release of CO2 cannot be avoided, such as some heavy industry (steel, cement) and aviation.2 Also, there will always be products whose creation requires carbon, such as many chemicals, polymers, and more. These products will eventually end up as CO2 at the end of their life. Therefore, solutions are needed to handle the unavoidable CO2 emissions and satisfy the continued need for carbon without tapping into fossil sources that would inevitably increase the atmosphere’s carbon content.
Beyond that, it is also clear that the legacy of 200 years of industrial growth includes more than a trillion tons of excess CO2 in the atmosphere. Significant amounts of this CO2 must be removed to keep average atmospheric temperatures no more than 2 °C above pre-industrial levels.1,3
Emerging technologies exist that can capture CO2 from factories, power plants, and from the air.4 These technologies will have to be scaled and deployed quickly to handle the gigaton-scale CO2 removal projected in Fig. 1. Scenarios like the ones shown are abundant in the literature, and they all share the conclusion that climate stabilization requires large-scale CO2 removal. The successful scale-up of carbon removal will hinge upon well-timed investments in technology, energy, and capital.5–8
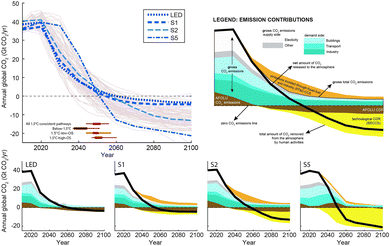 |
| Fig. 1 Projections for annual global CO2 emissions that would have to be achieved to limit the average atmospheric temperature increase to 1.5 °C over pre-industrial levels3 (reproduced with permission from IPCC). | |
The opportunities
Vast amounts of CO2 have to be disposed of permanently in underground caverns, aquifers, and spent oil and gas fields at a high financial cost.9 However, there is an additional pathway to handle some of the captured CO2. Captured CO2 can be used directly to create products that require a carbon feedstock, and through this, CO2 is repurposed without adding new carbon to the utilization cycle and the atmosphere.10 This also has the advantage of avoiding excessive land use if all carbon were to come from biological sources since that land may be needed to produce food and preserve natural habitats. In addition, since commercial products can be made from CO2 utilization, the outcomes will generate revenue projected to eventually exceed the cost of production.11
For long-term climate stability, CO2 emissions have to decrease rapidly,12 and in the long-run, the global carbon cycle has to be balanced to a net-zero scenario. Handling the inevitable emissions from some industries and consumer activities will require a combination of storage and utilization,13 as schematically shown in Fig. 2. For economic reasons alone, the utilization capabilities must be maximized, clearly identifying the reasons for CO2 utilization (CDU).
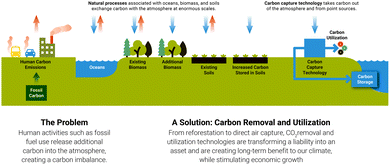 |
| Fig. 2 Schematic of major carbon dioxide fluxes. | |
CDU gaining rapid traction
Fig. 3 highlights how carbon dioxide utilization has found attention as an emerging field, measured by the number of annual publications indexed under “Carbon Dioxide Utilization” by the Web of Science. The increase in research publications during the second decade of the 21st century indicates the interest in CDU from funding agencies and the Academic Community. CDU is now being positioned towards market introduction, as observed previously in other technology fields.14,15
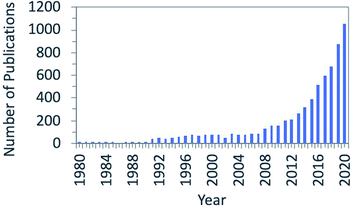 |
| Fig. 3 The Web of Science publication count based on the keyword “Carbon Dioxide Utilization” shows the typical fast increase in publications in an emerging field. | |
A 2016 study described a roadmap to a trillion-dollar market, highlighting the opportunities and documenting that at the time, less than 200 entities were engaged in commercial endeavors for CO2 utilization.11,16 That number has since increased incrementally, and some technologies have now achieved a sufficient maturity level to be deployed to markets, such as the production of chemicals, CO2-cured concrete, and aggregates. The potential for CO2 utilization, economically and environmentally, is demonstrated.
It is paramount to accelerate the transition from research laboratories to market introduction. However, as recently stated by an industry representative, “It seems to me that there is such a canyon between what the academic world considers complete and what the industry considers emerging – it is the old Valley of Death.”17
Measured on a technology readiness level (TRL) scale, university research readiness rarely exceeds levels 3–4, i.e., research at the level of proven feasibility and perhaps beginning technology development. Conversely, industrial interest, and that of many investors, begins at TRL 6 or higher, i.e., with pilot-sized, demonstrated technology ready to transition to full-scale engineering. This gap, the Valley of Death, is often a barrier that causes long delays in advancing inventions towards market introduction or literally leads to the death of a technology and termination of further pursuits. This valley is especially pronounced in developments that require complex and expensive hardware installation to advance the TRL. Shared incubators and collaborative partnerships can help to overcome the technological challenges.18,19 In addition, public policy plays a vital role in supporting the transition to higher TRL.20
Rapidly developing and deploying CO2 utilization technologies in a responsible manner requires an accelerated process to translate inventions from research laboratories to the market. Connecting and coordinating communities in academia, business, and government will be essential to address technical, financial, and societal questions in a responsible and impactful manner.21 The needs and opportunities align at this time, and CDU is expected to advance rapidly, even though many details have to be considered along the way.
Boundary conditions
The following questions need to be addressed to assist with decisions about CDU deployment.
• What to make from CO2?
• How much CO2 is permanently removed, or new release is avoided?
• What is the source of CO2?
• Do we have enough zero-carbon energy for CDU?
• Do we have enough zero-carbon hydrogen for CDU?
• How and when will CDU become financially competitive?
• What policies exist or are needed to deploy CDU?
• Are businesses prepared for CDU?
• What is the public sentiment towards CDU?
As a note, enhanced oil and gas recovery with CO2 can be considered a form of CO2 utilization and has been in use for decades already.22 The use of CO2 as a cooling or cutting fluid can equally be regarded as a significant use case with potential climate benefits through avoided emissions.23 However, neither of these use categories will be addressed in this paper.
CO2 product categories
Deciding the ‘what’, ‘how’, and ‘where’ of CDU is a multi-factorial process. Decisions will depend on location as well as on technology readiness, supply chain considerations, policies, and more. Each CDU project decision will have unique considerations, but all must fundamentally meet the following two criteria. First, the CO2-based product must have a better environmental footprint than the incumbent products, in particular a lower carbon footprint. Second, the economics of making and selling the product must be competitive with those of the incumbent product to engage the industry with the adaptation of the CO2-based product pathway.
Carbon is a central element in human life, and carbon plays a direct or indirect role in most existing products. When holistically considering CO2 utilization as a climate mitigation tool, it is useful to group opportunities into product categories and examine these in terms of their environmental and economic potential. Table 1 presents a list of product categories that include those that are directly made from fossil carbon sources, such as fuels and chemicals, and products that aren’t directly linked to fossil carbon sources, such as construction materials like concrete, aggregates, and related inorganic materials. This table shows that, regardless of the current relationship with fossil carbon sources, a wide range of industries can leverage CDU.
Table 1 Categories of products that could be made with CO2 exhibit differences in use potential and permanence of CO2 removal
Product category |
CO2 use potential |
CO2 flow |
Construction materials |
Large |
Permanent removal |
Fuels |
Large |
Circular |
Chemicals |
Modest–large |
Circular |
Engineered materials |
Modest |
Circular–permanent removal |
Polymers |
Modest |
Circular |
Agriculture & food |
Modest–large |
Circular |
Some of the largest opportunities for CO2 utilization are in making construction materials, e.g., concrete and aggregate materials. This is especially interesting since these materials have the ability to keep CO2 out of the atmosphere indefinitely. For the other product categories, CO2 mostly becomes a circular source of carbon that is currently taken from fossil sources.
Market opportunities
Successful market introduction of CDU products will depend on their competitive advantages over the incumbent products, be those of technical, financial, or regulatory nature. In many cases, the CDU product will be indistinguishable from the incumbent product, and therefore, market introduction will be primarily driven by cost and policy requirements. The latter will be required to overcome initially higher costs to drive cost reductions based on economies of scale, as previously demonstrated when policies supported new technology fields, such as photovoltaics.24–26 However, such mandates may not lead to a pure cost advantage in the absence of indefinite policy support. Ultimately, if the economics do not work for a particular product, it will fail in the market, no matter the size of the environmental advantage.27Fig. 4 shows that the magnitudes and ranges of potential revenue and CO2 use are significant. This points to a favorable combination of economic prospects and significant environmental benefits to address climate change.
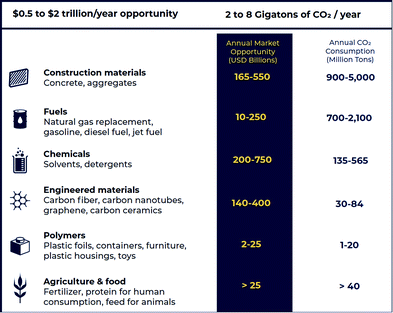 |
| Fig. 4 Projection of the economic and environmental potential for CDU products, based on a previous market assessment.11,16 | |
In this context, the overview in Table 2 points towards challenges with market introduction. Drop-in replacements are materials that are identical to the incumbents, and therefore they require either a lower cost or policy-supported procurement requirements for widespread market success. It appears unlikely that markets of that magnitude would be enabled by consumers choosing to pay a green-premium for the replacement product based on environmental concerns. The advantage of drop-in products, though, is that their functions and performances are known.
Table 2 CO2-based products may just be substitutes or become alternatives to incumbent products
Product category |
New vs. drop-in |
Construction materials |
New |
Fuels |
Drop-in and new |
Chemicals |
Drop-in |
Engineered materials |
New and drop-in |
Polymers |
Drop-in |
Agriculture & food |
New and drop-in |
CO2-based products, e.g., CO2-cured concrete materials, may be different from the incumbents and would therefore be considered new products, seeking to displace existing products. If such replacement products have superior properties not available in the incumbents, that competitive advantage can help to offset concerns about costs. On the other hand, regulatory requirements such as building codes and standards might complicate market introduction.
Exemplary products and technologies are briefly presented to illustrate the growing breadth and depth of the CDU field. While certainly considered an emerging field, the amount of literature available is already considerable, and so is the number of products that are being explored. This paper does not attempt to provide a comprehensive review of the entire field.
Products and technologies
Construction materials
The highest climate impact will be achieved with products that permanently remove the largest amount of CO2 and avoid the net release of large amounts of new CO2 into the atmosphere. Construction materials including cementitious materials, concrete, and aggregates play a critical role in this category because of their potential to permanently bind CO2 and their significant presence in global economies. Cement production alone accounts for 5–8% of global CO2 emissions, and at production rates of 10’s of gigatons, concrete is the second most widely used material next to water.28–30
Replacement materials and modifications for traditional cement,31–36 curing of concrete mixtures with CO2,37–42 and the use of waste materials or carbonated aggregates43–46 can all reduce the CO2 footprint of concrete. The use of new ingredients to make concrete and new curing methods can lead to substantially altered material properties. While this could lead to delays in market acceptance or require new procurement requirements, and building codes and standards, it is also an opportunity to expand the design space for cementitious construction materials.47 Previously introduced engineered cementitious composite materials,48 sometimes known as ‘bendable concrete’, offer substantially enhanced service lifetimes,49–51 self-healing properties (Fig. 5),52,53 as well as CO2-sequestering opportunities.37
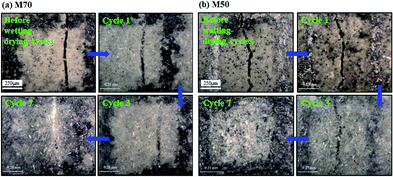 |
| Fig. 5 Self-healing properties of highly ductile carbonated Engineered Cementitious Composite (ECC)52 (reproduced with permission by the publisher). | |
Other construction materials, such as magnesium and calcium carbonates used to produce boards, panels, blocks, and gravel, can be made through carbonation of suitable precursor materials.54–57
Fuels and chemicals
Introducing CO2 as a source for carbon, in principle, opens up the entire value chain of organic chemistry.10,58–63 CO2 as a building block becomes much more interesting to industry.64
Technologies to produce fuels and chemicals from CO2 span a wide range of pathways that are often collectively called Power-to-X technologies.65 These can be based on syngas-based synthesis with Fischer–Tropsch processes,66 bio-organisms,67–70 and so-called artificial photosynthesis processes.71,72
These can help overcome some of the complexity of fuel synthesis from CO2 by integrating the harvesting of sunlight, splitting water and CO2 in artificial photosynthesis devices, labs-on-a-chip, to directly produce fuels such as methanol, methane, or hydrogen (Fig. 6).72
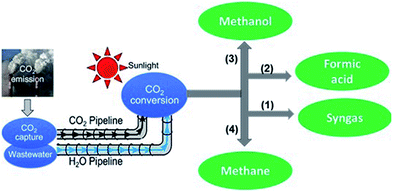 |
| Fig. 6 Schematic illustration of a solar refinery system for converting CO2 and wastewater into chemicals and fuels72 (reproduced with permission from Energy in Frontiers). | |
Converting CO2 into hydrocarbons for energy storage can cover large-scale fuel needs, especially in sectors where electrification is problematic, namely air and sea-based traffic, and seasonal storage of energy for intermittent, renewable energy sources, such as wind and solar systems.68,73–82 Particular challenges in this context are associated with the amount of energy and thus the cost required to transform CO2 into hydrocarbons. Careful consideration needs to be given to use cases where alternative energy carriers are not an option.59,83 The availability of cost-competitive green or blue hydrogen (if combined with carbon capture) is a key barrier to the growth of many Power-to-X technologies, and this requires equal attention as do the energy and CO2 footprint factors.84,85
Broadly, there is a rapidly growing number of photo- and electrochemical pathways to produce chemicals from CO2. The development of catalysts and catalytical systems requires particular attention.61,86–88 The integration of multiple reaction steps into one system, including CO2 capture, is of particular interest.61,89,90
Engineered materials
Fundamentally, the conversion of CO2 into carbon black and derived solid carbon products opens extensive market opportunities. The needs for carbon black are large and cover products such as toner for printing, fillers for polymers and concrete, and many more.91–95 Higher-value products such as carbon nanotubes96,97 or diamonds98 can also be made from CO2.
Polymers
Building on the analog in nature, CO2 has been investigated intensely as a building block for polymers.99,100 High-volume materials, such as polyurethanes, can be made with substantial fractions of CO2
101 and thus CO2 as a raw material opens pathways to green chemistry for polymer production.102 Introducing CO2 into polymer synthesis had already been described in the 1960’s.103 The production of polyurethane for foam manufacturing can serve a wide range of industries (Fig. 7).104 The versatility of CO2 as a renewable feedstock for polymers is considered an important element of the circular carbon economy.105
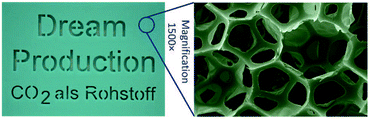 |
| Fig. 7 Polyurethane foam made from polycarbonates that were synthesized with CO2.104 (Reproduced with permission by the publisher). | |
Food and agriculture
Perhaps the most prominent example of CO2 use in this category is the production of urea, a key chemical for the fertilizer industry that can make use of the CO2 released during the production of NH3, the second key ingredient in the synthesis of urea.106 The use of CO2 to fumigate greenhouses to promote plant growth is established, though only recently have efforts started to use CO2 captured directly from the air instead of deliberately burning fossil fuels to generate the necessary CO2. Another novel method of turning CO2 into food is based on technologies that combine electrochemical processes with microbes to produce proteins as a source of food.107–109
Key factors for success
A new paradigm of energy use
Most methods of CO2 utilization are enormously energy-intensive. Therefore, CDU’s success will depend on the widespread availability of carbon-free energy to meet demand sufficiently. Undoubtedly, the energy requirements are substantial. A scenario for what is called ‘emergency CO2 direct air capture’ projects the need to use 9–14% of electricity and 53–83% of natural gas available by 2075 just to capture the CO2 that needs to be removed to stabilize the climate.5 Related to this, a study examining the electricity needs to operate the entire production of key chemicals from CO2 projects the need to double the current world production of electricity.59 It is thus imperative to develop processes that minimize energy needs, but it is equally important to consider a new paradigm for energy conversion and use. While it will always be important to use any resource as efficiently as possible, in terms of energy input to CO2 utilization (storage), the more important factor is avoiding adding new CO2 to the atmosphere. In the transition to such a scenario, it is clear that at present times, the available energy mix will render most CO2 utilization projects not helpful in mitigating the carbon problem or making it even worse. On the other hand, assuming future energy mixes, scenario planning will identify the full potential of new technologies.59,110 Thus, the path to carbon-free energy conversion and the development and deployment of CDU will have to go hand-in-hand.
Policy and other incentives
Undeniably, CDU is a portfolio of expensive technologies, but these technologies will become more affordable following the typical economies of scale. However, deployments will be slow without additional incentives, which may come in the form of taxes, subsidies, or mandates that require the purchase of CO2-based products.21,111–113 Policies, often local, regional, or national, will lead to a fragmented landscape where incentives at one location will promote CDU while elsewhere CDU will fail.114 An example is the production of ethanol from industrial waste gases which would be counted as a renewable fuel in the European Union due to the Renewable Energy Directive II (REDII). In contrast, in the United States, renewable fuels have to be bio-based, and therefore this CDU ethanol production would not receive benefits. On the other hand, legislation is being discussed in some US states that would create procurement requirements for low embodied carbon concrete (more below). These examples show that opportunities for CDU products can be region- and product-specific.
CDU is appealing as it provides a way to a new, truly circular carbon economy, coupled with new employment opportunities.115,116 Additionally, the United Nations Sustainable Development Goals are a suitable existing framework to assess the societal implications of CDU.117 Support for launching new industries in the form of procurement incentives or requirements has helped in the past, e.g., renewable portfolio standards for the solar energy field. Developing state procurement policies like the Low Embodied Carbon Concrete Leadership Act (LECCLA), which is currently being considered in the New York State and New Jersey legislatures, and Buy Clean California are examples that could help drive demand and build capacity for CDU products. Carbon offset markets will eventually look for opportunities to acquire offsets, and therefore, CDU offers business options to create suitable offsets for sale. Demand and supply forces for sources and uses of CO2
118,119 will then be expanded by these additional drivers.
The rapidly growing number of corporate commitments towards carbon neutrality will increase CDU deployments since offsets alone are not sustainable. As more demand for offsets is created, availability will decrease, costs will rise, and sooner rather than later, offset opportunities from agricultural or forestry efforts as well as selling credits that are not used will be used up.
In support of developing solutions, examples include the Shopify Sustainability Fund and Stripe’s Climate program which allocate and generate support to create a carbon removal market; the Carbon Capture XPrize, funded by Elon Musk; Microsoft’s commitment to offset all their emissions retroactively to when the company was founded and then go beyond to become carbon negative; Breakthrough Energy Ventures; the Oil & Gas Climate Initiative; Amazon’s Climate Pledge, and in addition Jeff Bezos’s personal pledge; Unilever’s commitment to stop using fossil carbon sources by 2030; similar commitments by Covestro; and so forth. Similarly, for power companies and cement plants, achieving net-zero emissions gets more and more difficult for the last 10’s of percent of their carbon emissions. CDU can offer an opportunity to reach the ambitious, yet necessary, goals.
Life cycle assessments and techno-economic assessments
CO2 utilization, in contrast to CO2 storage, has the advantage that the resulting products can, fundamentally, achieve financial profitability and sustainability with far less, if any, subsidies. The cost of CO2 itself is a key cost factor for many CDU products,120 but these costs vary widely across product categories11 and based on the source of CO2.9
Despite the urgency of developing and deploying CO2 utilization, it is paramount that CDU practitioners comprehensively assess the impact and opportunities of new technologies to ensure environmental and economic gains will be achieved. Complete life cycle assessments (LCA) and techno-economic assessments (TEA) are essential in guiding research, development, and deployment. As an example, while CO2 curing of concrete should provide a sink for CO2, the actual outcome strongly depends on the design and execution of the processes used, and in the worst case, the CO2 footprint is higher than for traditionally made concrete.42 The production of methanol is another prominent example that illustrates the importance of assessing technologies in a context that allows comparison across production methods, available energy mixes, and other factors (Fig. 8).88 Concepts such as a carbon return on investment to evaluate CDU technology’s benefits will be guided by LCA.121
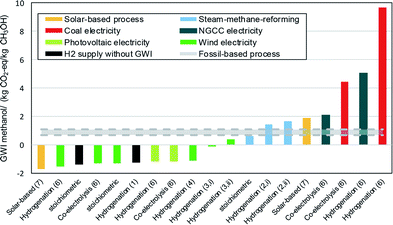 |
| Fig. 8 The assessed CO2 footprint of methanol production from CO2 strongly depends on the technologies used and the details of the assessment methods.88 (Reproduced with permission by the publisher). | |
The entire value chain must be optimized as a system for the best environmental impact – so that breakthrough technologies can be brought closer to market readiness and environmentally costly mistakes will be avoided. One is reminded of what Sir Harry Ricardo said in 1922 about direct-injection engines in that “working with a stratified charge … is possible and the high efficiency theoretically obtainable from it can be approached. The worst feature about it is that, if not just right, it may be very wrong; a small change in form or dimension may upset the whole system.”122
Transparent, complete, and uniform assessments for the life cycle emissions of product production and use have to be available for rigorous and impartial evaluation for the continuation of research work, scale-up work, investment in emerging companies, and certification for possible tax consideration.
Assessments, both TEA and LCA, rarely provide a unique and straightforward answer; rather, several factors need to be considered to draw a conclusion and make decisions about continuation of research, scale-up, deployment, policy support, etc.123,124
ISO standards 14040 and 14044 are available for LCA in general. Applying these standards for CDU, though, left key assumptions and procedures to the user’s discretion. As a result, LCA results were difficult to compare against each other. The need to provide further specification and guidance for LCA and TEA was identified, and additional guidance specifications for CDU were developed.125–129 Building on these and other efforts, further work is underway to harmonize guidance for CDU assessments130 and provide guidance for decision makers.131,132
Information about the environmental and economic benefits and risks would ideally be available early in the research and development process. Unfortunately, the assessment of low TRL efforts suffers from considerable uncertainty in the data or even lack of required data. Therefore, LCA and TEA of low TRL processes require particular attention to produce meaningful outcomes.133,134
Equally challenging is the joint interpretation of LCA and TEA if conducted separately, possibly not using shared data and/or functional units for the analysis. Therefore, combined or integrated LCA and TEA should be preferred to achieve outcomes that allow accurate interpretation and meaningful decisions for action.135,136
While the technical, economic, and environmental feasibility of CDU product manufacturing and use is essential for deploying related technologies, social acceptance and social justice factors are critically important. Deployment of CDU technologies will, literally, be visible with large-scale installations, debates about the best use of resources, concerns about environmental factors, and more. Given the relatively new field of CDU, public familiarity, understanding, and acceptance are not yet established, and efforts are required to ensure societal compatibility.137–144
Digitalization
Implementing a carbon economy built around CO2 as a feedstock requires building up entirely new supply and value chains. This new carbon economy can be structured around current technological infrastructure to improve efficiency and real-time communication. In designing and operating new industrial processes, digital tools can optimize additional factors, including globally minimized CO2 emissions, social equity, and global resources management. None of that will work without the fullest deployment of digitalization tools across the CO2 utilization field. Continuing enhancements and implementation of digitalization will be a technological enabler to optimize systems for minimal CO2 emissions. The key is that reaching net-zero emissions requires fully considering any action’s impact on the system response. Fully integrated digital systems will allow one to transparently track emissions, to record certification of carbon credits, carbon dividends, tax obligations, and other financial instruments essential to managing ambitious environmental goals.
Digital tools are already being used to react to the worst impacts of climate change.145 Big data and artificial intelligence are being used to respond to fires, floods, and other climate disasters. They have shown their potential to save lives and minimize economic losses. However, these tools must be used for more than adaptation; climate regeneration industries must embrace digital tools in the toolkit needed to fight climate change. Among other means, artificial intelligence and digitalization tools must be brought to a level of performance and reliability to accurately predict the system-level impact of proposed climate action in all aspects: climate-related, financial or societal.
Capture of carbon dioxide
Above, many reasons and opportunities for the fast and large-scale growth of CDU were given. However, a key barrier to faster deployment of CDU is the cost of CO2 itself. The financial and energy cost of capture technology, both in terms of installations and operation, are still too high.7,9,146,147 Depending on the source of the CO2, the associated carbon footprint of capture and post-processing will vary substantially.148 Continued efforts will develop enhanced sorbents and processes that will capture more CO2 per specific amount of sorbent, that are more stable, and that require less energy for desorption.149–155
The present amount of captured CO2 is on the order of 77 million tons a year, and only a small fraction of that is used in CDU processes. This is a far cry from where the activity level needs to be, based on climate models and business opportunities, as shown in Fig. 9.156
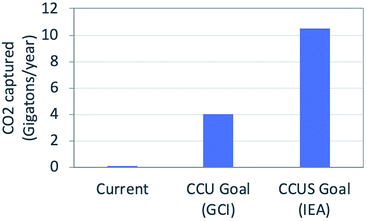 |
| Fig. 9 The current CO2 capture capacity is far less than what IPCC projections and IEA state is required for a net-zero carbon economy.157 It is less than 1% of the amount that could be used for CDU products.11,27 | |
Conclusions
The conditions for further developing, scaling, and deploying CO2 utilization (CDU) are such that it is clear that these technologies are needed now. These technologies must rapidly be brought to market, though many questions remain.
Fundamentally, climate model predictions consistently show that CO2 must be removed from the atmosphere at rates of 10’s of gigatons per year. Even once the current excess level of CO2 in the atmosphere has been brought back down to tolerable levels, there is a continuing need for CO2 capture, utilization, and storage to handle emissions that cannot be avoided. Simultaneously, there will be a continued need for carbon-based products and these could be made from CO2. Thus, utilizing CO2 to create economically sustainable products becomes both a source for essential products and a process to achieve and maintain a net-zero carbon economy. Its contribution is through the permanent sequestration of CO2 into rock-like materials, such as concrete, and as an alternative source of carbon for products traditionally made from fossil carbon. Thereby, CDU avoids the addition of new CO2 to the atmosphere and contributes substantially to a circular carbon economy.
The climate scenarios predicted by integrated assessment models show the urgency at which action is needed, prompting some to call for war-like efforts.5 At this time, CDU has the potential to become an additional ‘wedge’ in efforts towards a net-zero carbon economy158 and advances are being made.159
Much of the work described above is enabled by and developed in parallel with the rapid increase in available carbon-free energy and growing industry that can produce and deliver hydrogen in an environmentally friendly manner. In this context, the use of energy has to follow a new paradigm, one where it is still necessary to push for the highest possible efficiencies and lowest overall energy use. However, the need to avoid net CO2 addition to the atmosphere supersedes some of these needs.
The case has been made for CDU based on environmental, technological, and economic grounds. Avoiding a climate-related disaster requires decisive, fast, and large-scale action. Some solutions are available and new technologies are emerging, while work on additional efforts needs to intensify.160 The prospects of economic growth can be a powerful enabler. The timing might be perfect now since a growing number of current and emerging business leaders are beginning to see management as a calling in service to society.161 But much work is still needed to create public awareness and political support for CDU.
Conflicts of interest
There are no conflicts to declare.
Acknowledgements
The author is grateful for support from the Global CO2 Initiative and the College of Engineering, University of Michigan’s BlueSky program. The author would also like to acknowledge helpful discussions with Dr Hannelore Lillich, Dr Lucca Henrion, and Dr Kristine Kattapong.
References
- O. Hoegh-Guldberg, D. Jacob, M. Taylor, T. Guillen Bolanos, M. Bindi, S. Brown, I. A. Camilloni, A. Diedhiou, R. Djalante, K. Ebi, F. Engelbrecht, J. Guiot, Y. Hijioka, S. Mehrotra, C. W. Hope, A. J. Payne, H. O. Portner, S. I. Seneviratne, A. Thomas, R. Warren and G. Zhou, Science, 2019, 365, eaaw6974 Search PubMed.
-
Energy Transitions Commission, Mission Possible: Reaching Net-Zero Carbon Emissions From Harder-To-Abate Sectors by Mid-Century, London, UK, 2018 Search PubMed.
-
V. Masson-Delmotte, P. Zhai, H.-O. Pörtner, D. Roberts, J. Skea, P. R. Shukla, A. Pirani, W. Moufouma-Okia, C. Péan, R. Pidcock, S. Connors, J. B. R. Matthews, Y. Chen, X. Zhou, M. I. Gomis, E. Lonnoy, T. Maycock, M. Tignor and T. Waterfield, Global Warming of 1.5 °C. An IPCC Special Report on the impacts of global warming of 1.5 °C above pre-industrial levels and related global greenhouse gas emission pathways, in the context of strengthening the global response to the threat of climate change, sustainable development, and efforts to eradicate poverty, Intergovernmental Panel on Climate Change, Geneva, Switzerland, 2018 Search PubMed.
-
J. Wilcox, Carbon Capture, Springer, 2012 Search PubMed.
- R. Hanna, A. Abdulla, Y. Xu and D. G. Victor, Nat. Commun., 2021, 12, 368 Search PubMed.
- S. D. Supekar, T.-H. Lim and S. J. Skerlos, Environ. Res. Lett., 2019, 14, 084013 Search PubMed.
-
K. Lebling, N. McQueen, M. Pisciotta and J. Wilcox, 2021, 2021, https://www.wri.org/blog/2021/01/direct-air-capture-definitioncost-considerations.
-
R. D. Aines, G. Amador, W. Anderegg, E. Belmont, A. Bergman, H. J. Buck, D. Cullenward, J. Davids, M. Desmond, G. Dipple, S. Fuss, J. Freeman, K. Gomes, S. Hovorka, R. Jacobson, P. Kelemen, K. Kian, B. Kolosz, F. Kraxner, S. Liguori, C. McCormick, S. McCoy, N. McQueen, K. Paustian, H. Pilorgé, M. Pisciotta, P. Psarras, A. Ramirez, P. Renforth, A. Rinberg, D. L. Sanchez, V. Sick, P. Smith, M. Torn, T. Troxler, M. V. d. Spek, J. Wilcox, C. M. Woodall and G. C. Wu, Carbon Dioxide Removal Primer, 2021, https://cdrprimer.org/ Search PubMed.
-
A. Baylin-Stern and N. Berghout, 2021, https://www.iea.org/commentaries/is-carbon-capture-tooexpensive.
-
Carbon Dioxide Utilisation: Closing the Carbon Cycle, ed. P. Styring, E. A. Quadrelli and K. Armstrong, Elsevier, 2015 Search PubMed.
-
CO2 Sciences, Global Roadmap for Implementing CO2 Utilization, University of Michigan, Ann Arbor, MI, USA, 2016 Search PubMed.
-
V. Ramanathan, R. Aines, M. Auffhammer, M. Barth, J. Cole, F. Forman, H. Han, M. Jacobsen, D. Pellow, K. Pezzoli, D. Press, E. Rignot, S. Samuelsen, W. Silver, G. Solomon, R. Somerville, M. E. Tucker, D. Victor, D. Zaelke and S. Friese, Bending the Curve: Climate Change Solutions, 2019, https://escholarship.org/uc/item/6kr8p5rq Search PubMed.
-
Intergovernmental Panel on Climate Change, Special Report: Global Warming of 1.5 °C, 2018 Search PubMed.
- L. F. Chanchetti, D. R. Leiva, L. I. Lopes de Faria and T. T. Ishikawa, Int. J. Hydrogen Energy, 2020, 45, 5356–5366 Search PubMed.
- Y. Warsi, V. Kabanov, P. Zhou and A. Sinha, Front. Energy Res., 2020, 8, 574147 Search PubMed.
-
Lux Research, Global Roadmap Study of CO2U Technologies, University of Michigan, Ann Arbor, MI, USA, 2016 Search PubMed.
- Anonymous, personal communication..
-
M. Sefton, I. Rönkkö and M. Bellgran, in Sps2020, 2020, DOI:10.3233/atde200175.
- P. Ellwood, C. Williams and J. Egan, Technovation, 2020 DOI:10.1016/j.technovation.2020.102162.
- J. Bonnin Roca and E. O’Sullivan, Technovation, 2020 DOI:10.1016/j.technovation.2020.102157.
- S. J. Friedmann, Front. Clim., 2019, 1, 3 Search PubMed.
- V. Núñez-López and E. Moskal, Front. Clim., 2019, 1, 5 Search PubMed.
- A. F. Clarens, J. B. Zimmerman, G. A. Keoleian, K. F. Hayes and S. J. Skerlos, Environ. Sci. Technol., 2008, 42, 8534–8540 Search PubMed.
- B. A. Andersson and S. Jacobsson, Energy Policy, 2000, 28, 1037–1049 Search PubMed.
- V. Babich, R. Lobel and Ş. Yücel, Manuf. Serv. Oper. Manag., 2020, 22, 1148–1164 Search PubMed.
- X. Zhang, L. Zhang, K. Y. Fung, G. P. Rangaiah and K. M. Ng, Comput. Chem. Eng., 2018, 118, 118–131 Search PubMed.
- C. Hepburn, E. Adlen, J. Beddington, E. A. Carter, S. Fuss, N. Mac Dowell, J. C. Minx, P. Smith and C. K. Williams, Nature, 2019, 575, 87–97 Search PubMed.
-
International Energy Agency, World Energy Outlook, International Energy Agency, Paris, France, 2018 Search PubMed.
-
International Energy Agency, Technology Roadmap: Low-Carbon Transition in the Cement Industry, 2018 Search PubMed.
- R. M. Andrew, Earth Syst. Sci. Data, 2019, 11, 1675–1710 Search PubMed.
- S. A. Miller, A. Horvath and P. J. M. Monteiro, Environ. Res. Lett., 2016, 11, 074029 Search PubMed.
- B. Lothenbach, K. Scrivener and R. D. Hooton, Cem. Concr. Res., 2011, 41, 1244–1256 Search PubMed.
- C. Shi, J. Mater. Civ. Eng., 2004, 16, 230–236 Search PubMed.
- D. Zhang, X. Cai and Y. Shao, J. Mater. Civ. Eng., 2016, 04016127 Search PubMed.
- K. L. Scrivener, V. M. John and E. M. Gartner, Cem. Concr. Res., 2018, 114, 2–26 Search PubMed.
- K. Scrivener, F. Martirena, S. Bishnoi and S. Maity, Cem. Concr. Res., 2018, 114, 49–56 Search PubMed.
- H.-L. Wu, D. Zhang, B. R. Ellis and V. C. Li, Constr. Build. Mater., 2018, 191, 23–31 Search PubMed.
- W. Ashraf, Constr. Build. Mater., 2016, 120, 558–570 Search PubMed.
- S. Monkman and M. MacDonald, J. Cleaner Prod., 2017, 167, 365–375 Search PubMed.
- S. Monkman, M. MacDonald, R. D. Hooton and P. Sandberg, Cem. Concr. Compos., 2016, 74, 218–224 Search PubMed.
- K. Vance, M. Aguayo, T. Oey, G. Sant and N. Neithalath, Cem. Concr. Compos., 2013, 39, 93–103 Search PubMed.
- D. Ravikumar, D. Zhang, G. Keoleian, S. Miller, V. Sick and V. Li, Nat. Commun., 2021, 12, 855 Search PubMed.
- X. Huang, R. Ranade, W. Ni and V. C. Li, Constr. Build. Mater., 2013, 44, 757–764 Search PubMed.
- B. Zhan, C. Poon and C. Shi, Cem. Concr. Compos., 2013, 42, 1–8 Search PubMed.
- Z. Cao, R. J. Myers, R. C. Lupton, H. Duan, R. Sacchi, N. Zhou, T. Reed Miller, J. M. Cullen, Q. Ge and G. Liu, Nat. Commun., 2020, 11, 3777 Search PubMed.
- I. Rahmouni, G. Promis, A. R’Mili, H. Beji and O. Limam, Constr. Build. Mater., 2019, 197, 241–250 Search PubMed.
- J. J. Biernacki, J. W. Bullard, G. Sant, N. Banthia, K. Brown, F. P. Glasser, S. Jones, T. Ley, R. Livingston, L. Nicoleau, J. Olek, F. Sanchez, R. Shahsavari, P. E. Stutzman, K. Sobolev and T. Prater, J. Am. Ceram. Soc., 2017, 100, 2746–2773 Search PubMed.
-
V. C. Li, Engineered Cementitious Composites (ECC): Bendable Concrete for Sustainable and Resilient Infrastructure, Springer, 2019 Search PubMed.
- H. Liu, Q. Zhang, C. Gu, H. Su and V. C. Li, Cem. Concr. Compos., 2016, 72, 104–113 Search PubMed.
- H. Zhang, M. D. Lepech, G. A. Keoleian, S. Qian and V. C. Li, J. Infrastruct. Syst., 2010, 16, 299–309 Search PubMed.
- G. A. Keoleian, A. Kendall, J. E. Dettling, V. M. Smith, R. F. Chandler, M. D. Lepech and V. C. Li, J. Infrastruct. Syst., 2005, 11, 51–60 Search PubMed.
- D. Zhang, H. Wu, V. C. Li and B. R. Ellis, Constr. Build. Mater., 2020, 238, 117672 Search PubMed.
- L. L. Kan, H. S. Shi, A. R. Sakulich and V. C. Li, ACI Mater. J., 2010, 107, 617–642 Search PubMed.
- F. P. Glasser, G. Jauffret, J. Morrison, J.-L. Galvez-Martos, N. Patterson and M. S.-E. Imbabi, Front. Energy Res., 2016, 4, 3 Search PubMed.
- A.-H. A. Park, R. Jadhav and L.-S. Fan, Can. J. Chem. Eng., 2003, 81, 885–890 Search PubMed.
- S. Lin, T. Kiga, Y. Wang and K. Nakayama, Energy Procedia, 2011, 4, 356–361 Search PubMed.
- Y. Katsuyama, A. Yamasaki, A. Iizuka, M. Fujii, K. Kumagai and Y. Yanagisawa, Environ. Prog., 2005, 24, 162–170 Search PubMed.
- P. R. Yaashikaa, P. Senthil Kumar, S. J. Varjani and A. Saravanan, J. CO2 Util., 2019, 33, 131–147 Search PubMed.
- A. Kätelhön, R. Meys, S. Deutz, S. Suh and A. Bardow, Proc. Natl. Acad. Sci. U. S. A., 2019, 116, 11187–11194 Search PubMed.
- A. Mustafa, B. G. Lougou, Y. Shuai, Z. Wang and H. Tan, J. Energy Chem., 2020, 49, 96–123 Search PubMed.
- N. W. Kinzel, C. Werle and W. Leitner, Angew. Chem., Int. Ed., 2021, 60, 2–61 Search PubMed.
- J. B. Zimmerman, P. T. Anastas, H. C. Erythropel and W. Leitner, Science, 2020, 367, 397 Search PubMed.
- M. Poliakoff, W. Leitner and E. S. Streng, Faraday Discuss., 2015, 183, 9–17 Search PubMed.
- S. Dabral and T. Schaub, Adv. Synth. Catal., 2019, 361, 223–246 Search PubMed.
- B. Rego de Vasconcelos and J. M. Lavoie, Front. Chem., 2019, 7, 392 Search PubMed.
- R. Cuéllar-Franca, P. García-Gutiérrez, I. Dimitriou, R. H. Elder, R. W. K. Allen and A. Azapagic, Appl. Energy, 2019, 253, 113560 Search PubMed.
- J. Daniell, M. Köpke and S. Simpson, Energies, 2012, 5, 5372–5417 Search PubMed.
- M. Köpke, C. Mihalcea, J. C. Bromley and S. D. Simpson, Curr. Opin. Biotechnol., 2011, 22, 320–325 Search PubMed.
- G. Hu, Y. Li, C. Ye, L. Liu and X. Chen, Trends Biotechnol., 2019, 37, 532–547 Search PubMed.
- S. A. Razzak, M. M. Hossain, R. A. Lucky, A. S. Bassi and H. de Lasa, Renewable Sustainable Energy Rev., 2013, 27, 622–653 Search PubMed.
- D. Gust, T. A. Moore and A. L. More, Acc. Chem. Res., 2009, 42, 1890–1898 Search PubMed.
- Z. Mi and V. Sick, Front. Energy Res., 2020, 8, 153 Search PubMed.
- B. Yao, T. Xiao, O. A. Makgae, X. Jie, S. Gonzalez-Cortes, S. Guan, A. I. Kirkland, J. R. Dilworth, H. A. Al-Megren, S. M. Alshihri, P. J. Dobson, G. P. Owen, J. M. Thomas and P. P. Edwards, Nat. Commun., 2020, 11, 6395 Search PubMed.
- P. Styring and G. R. M. Dowson, Johnson Matthey Technol. Rev., 2021, 65(2), 170–179 Search PubMed.
- P. De Luna, C. Hahn, D. Higgins, S. A. Jaffer, T. F. Jaramillo and E. H. Sargent, Science, 2019, 364, 350 Search PubMed.
-
Sustainable synthetic carbon based fuels for transport, The Royal Society, London, UK, 2019 Search PubMed.
- T. Pregger, G. Schiller, F. Cebulla, R.-U. Dietrich, S. Maier, A. Thess, A. Lischke, N. Monnerie, C. Sattler, P. L. Clercq, B. Rauch, M. Köhler, M. Severin, P. Kutne, C. Voigt, H. Schlager, S. Ehrenberger, M. Feinauer, L. Werling, V. P. Zhukov, C. Kirchberger, H. K. Ciezki, F. Linke, T. Methling, U. Riedel and M. Aigner, Energies, 2019, 13, 138 Search PubMed.
- H. A. Daggash, C. F. Patzschke, C. F. Heuberger, L. Zhu, K. Hellgardt, P. S. Fennell, A. N. Bhave, A. Bardow and N. Mac Dowell, Sustainable Energy Fuels, 2018, 2, 1153–1169 Search PubMed.
-
P. J. Hall, I. A. G. Wilson and A. Rennie, in Carbon Dioxide Utilisation, ed. P. Styring, E. A. Quadrelli and K. Armstrong, Elsevier, Amsterdam, 2015, pp. 33–44, DOI:10.1016/b978-0-444-62746-9.00003-7.
-
L. J. France, P. P. Edwards, V. L. Kuznetsov and H. Almegren, in Carbon Dioxide Utilisation, ed. P. Styring, E. A. Quadrelli and K. Armstrong, Elsevier, Amsterdam, 2015, pp. 161–182, DOI:10.1016/b978-0-444-62746-9.00010-4.
- I. Dimitriou, P. García-Gutiérrez, R. H. Elder, R. M. Cuéllar-Franca, A. Azapagic and R. W. K. Allen, Energy Environ. Sci., 2015, 8, 1775–1789 Search PubMed.
- S. W. Sheehan, E. R. Cave, K. P. Kuhl, N. Flanders, A. L. Smeigh and D. T. Co, Chem, 2017, 3, 3–7 Search PubMed.
- J. Newman, P. G. Hoertz, C. A. Bonino and J. A. Trainham, J. Electrochem. Soc., 2012, 159, A1722–A1729 Search PubMed.
-
M. Alvéra, Generation H: Healing the climate with hydrogen, Mondadori Electa S.p.A., Milano, 2019 Search PubMed.
-
International Energy Agency, The future of hydrogen, International Energy Agency, Paris, France, 2019 Search PubMed.
- B. Zhou, P. Ou, N. Pant, S. Cheng, S. Vanka, S. Chu, R. T. Rashid, G. Botton, J. Song and Z. Mi, Proc. Natl. Acad. Sci. U. S. A., 2020, 117, 1330–1338 Search PubMed.
- D. G. Montjoy, H. Hou, J. H. Bahng, A. Eskafi, R. Jiang and N. A. Kotov, ACS Nano, 2021, 15(3), 4226–4234 Search PubMed.
- J. Artz, T. E. Müller, K. Thenert, J. Kleinekorte, R. Meys, A. Sternberg, A. Bardow and W. Leitner, Chem. Rev., 2018, 118, 434–504 Search PubMed.
- S. Overa, T. G. Feric, A.-H. A. Park and F. Jiao, Joule, 2021, 5, 8–13 Search PubMed.
- R. K. Singh, R. Singh, D. Sivakumar, S. Kondaveeti, T. Kim, J. Li, B. H. Sung, B.-K. Cho, D. R. Kim, S. C. Kim, V. C. Kalia, Y.-H. P. J. Zhang, H. Zhao, Y. C. Kang and J.-K. Lee, ACS Catal., 2018, 8, 11085–11093 Search PubMed.
- Y. Fan, G. D. Fowler and M. Zhao, J. Cleaner Prod., 2020, 247, 119115 Search PubMed.
- N. J. Hardman, Reinf. Plast., 2017, 61, 145–148 Search PubMed.
-
J.-B. Donnet, Carbon Black: Science and Technology, Marcel Dekker, Inc., New York, 2nd edn, 1993 Search PubMed.
- C. Li, X. Zhang, K. Wang, F. Su, C.-M. Chen, F. Liu, Z.-S. Wu and Y. Ma, J. Energy Chem., 2021, 54, 352–367 Search PubMed.
- C. Liang, Y. Chen, M. Wu, K. Wang, W. Zhang, Y. Gan, H. Huang, J. Chen, Y. Xia, J. Zhang, S. Zheng and H. Pan, Nat. Commun., 2021, 12, 119 Search PubMed.
- X. Wang, F. Sharif, X. Liu, G. Licht, M. Lefler and S. Licht, J. CO2 Util., 2020, 40, 101218 Search PubMed.
- X. Wang, G. Licht, X. Liu and S. Licht, Sci. Rep., 2020, 10, 21518 Search PubMed.
- Z. Lou, Q. Chen, Y. Zhang, Y. Qian and W. Wang, J. Phys. Chem. B, 2004, 108, 4239–4241 Search PubMed.
- E. J. Beckman, Science, 1999, 283, 946 Search PubMed.
-
M. Carus, L. Dammer, A. Raschka and P. Skoczinski, Renewable Carbon – Key to a Sustainable and Future-Oriented Chemical and Plastic Industry, Nova Institute, Hürth, Germany, 2020 Search PubMed.
-
J. Langanke, A. Wolf and M. Peters, in Carbon Dioxide Utilisation, ed. P. Styring, E. A. Quadrelli and K. Armstrong, Elsevier, Amsterdam, 2015, pp. 59–71, DOI:10.1016/b978-0-444-62746-9.00005-0.
- C. Hussong, J. Langanke and W. Leitner, Green Chem., 2020, 22, 8260–8270 Search PubMed.
- S. Inoue, H. Koinuma and T. Tsuruta, Macromol. Chem. Phys., 1969, 130, 210–220 Search PubMed.
- J. Langanke, A. Wolf, J. Hofmann, K. Böhm, M. A. Subhani, T. E. Müller, W. Leitner and C. Gürtler, Green Chem., 2014, 16, 1865–1870 Search PubMed.
- B. Grignard, S. Gennen, C. Jerome, A. W. Kleij and C. Detrembleur, Chem. Soc. Rev., 2019, 48, 4466–4514 Search PubMed.
- J. Meessen, Chem. Ing. Tech., 2014, 86, 2180–2189 Search PubMed.
- J. Sillman, L. Nygren, H. Kahiluoto, V. Ruuskanen, A. Tamminen, C. Bajamundi, M. Nappa, M. Wuokko, T. Lindh, P. Vainikka, J.-P. Pitkänen and J. Ahola, Glob. Food Sec., 2019, 22, 25–32 Search PubMed.
- A. Mishra, J. N. Ntihuga, B. Molitor and L. T. Angenent, Joule, 2020, 4, 1142–1147 Search PubMed.
- B. Pander, Z. Mortimer, C. Woods, C. McGregor, A. Dempster, L. Thomas, J. Maliepaard, R. Mansfield, P. Rowe and P. Krabben, Engineering Biology, 2020, 4, 21–24 Search PubMed.
- D. Ravikumar, G. Keoleian and S. Miller, Appl. Energy, 2020, 279, 115770 Search PubMed.
-
B. G. Rabe, M. E. Kraft and S. Kamieniecki, Can We Price Carbon?, MIT Press, 2018 Search PubMed.
-
J. Friedmann, A. Zapantis, B. Page, C. Consoli, Z. Fan, H. Liu, E. R. Ochu, N. Raji, D. Rassool, H. Sheerazi and A. Townsend, Net-Zero and Geospheric Return: Actions Today for 2030 and Beyond, Columbia University, Center on Global Energy Policy, 2020 Search PubMed.
-
J. Larsen, N. Kaufman, P. Marsters, W. Herndon, H. Kolus and B. King, Expanding the reach of a carbon tax emissions impacts of pricing combined with additional climate actions, Columbia University, Center on Global Energy Policy, New York, 2020 Search PubMed.
- F. Schenuit, R. Colvin, M. Fridahl, B. McMullin, A. Reisinger, D. L. Sanchez, S. M. Smith, A. Torvanger, A. Wreford and O. Geden, Front. Clim., 2021, 3, 638805 Search PubMed.
-
J. Larsen, W. Herndon, G. Hiltbrand and B. King, The Economic Benefits of Industrial Carbon Capture: Investment and Employment Opportunities for Eastern and Western States, 2021 Search PubMed.
-
J. Larsen, W. Herndon, G. Hiltbrand and B. King, The Economic Benefits of Carbon Capture: Investment and Employment Estimates for the Contiguous United States, Rhodium Group, 2020 Search PubMed.
- B. Olfe-Kräutlein, Front. Energy Res., 2020, 8, 198 Search PubMed.
- H. Naims, J. Ind. Ecol., 2020, 24(5), 1126–1139 Search PubMed.
- H. Naims, Environ. Sci. Pollut. Res. Int., 2016, 23, 22226–22241 Search PubMed.
- N. Mac Dowell, P. S. Fennell, N. Shah and G. C. Maitland, Nat. Clim. Change, 2017, 7, 243–249 Search PubMed.
- U. Singh and L. M. Colosi, Appl. Energy, 2021, 285, 116394 Search PubMed.
- H. R. Ricardo, SAE Trans., 1922, 14, 30–32 Search PubMed.
- G. Centi, S. Perathoner, A. Salladini and G. Iaquaniello, Front. Energy Res., 2020, 8, 567986 Search PubMed.
- R. M. Cuéllar-Franca and A. Azapagic, J. CO2 Util., 2015, 9, 82–102 Search PubMed.
-
A. W. Zimmermann, J. Wunderlich, G. Buchner, L. Müller, K. Armstrong, S. Michailos, A. Marxen, H. Naims, F. Mason, G. Stokes and E. Williams, Techno-Economic Assessment & Life-Cycle Assessment Guidelines for CO2 Utilization, G. C. Initiative, University of Michigan, Ann Arbor, MI, USA, 2018 Search PubMed.
-
NETL, Life Cycle Analysis (LCA) of Energy Technology and Pathways, https://www.netl.doe.gov/LCA, accessed 8/28/2019, 2019 Search PubMed.
- G. Garcia-Garcia, M. C. Fernandez, K. Armstrong, S. Woolass and P. Styring, ChemSusChem, 2021, 14, 995–1015 Search PubMed.
- L. J. Müller, A. Kätelhön, M. Bachmann, A. Zimmermann, A. Sternberg and A. Bardow, Front. Energy Res., 2020, 8, 15 Search PubMed.
- A. W. Zimmermann, J. Wunderlich, L. Müller, G. A. Buchner, A. Marxen, S. Michailos, K. Armstrong, H. Naims, S. McCord, P. Styring, V. Sick and R. Schomäcker, Front. Energy Res., 2020, 8, 5 Search PubMed.
- V. Sick, K. Armstrong, G. Cooney, L. Cremonese, A. Eggleston, G. Faber, G. Hackett, A. Kätelhön, G. Keoleian, J. Marano, J. Marriott, S. McCord, S. A. Miller, M. Mutchek, B. Olfe-Kräutlein, D. Ravikumar, L. K. Roper, J. Schaidle, T. Skone, L. Smith, T. Strunge, P. Styring, L. Tao, S. Völker and A. Zimmermann, Energy Technol., 2020, 1901034, DOI:10.1002/ente.201901034.
-
L. Cremonese, B. Olfe-Kräutlein, T. Strunge, H. Naims, A. Zimmermann, T. Langhorst, L. Müller, S. McCord, V. Sick, S. Saccani and S. Jahilo, Making Sense of Techno-Economic Assessment & Life Cycle Assessment Studies for CO2 Utilization: A guide on how to commission, understand, and derive decisions from TEA and LCA studies, University of Michigan, Ann Arbor, MI, USA, 2020 Search PubMed.
- G. Zang, P. Sun, A. Elgowainy, A. Bafana and M. Wang, Environ. Sci. Technol., 2021, 55(6), 3888–3897 Search PubMed.
- A. W. Zimmermann and R. Schomäcker, Energy Technol., 2017, 5, 850–860 Search PubMed.
- K. Roh, A. Bardow, D. Bongartz, J. Burre, W. Chung, S. Deutz, D. Han, M. Heßelmann, Y. Kohlhaas, A. König, J. S. Lee, R. Meys, S. Völker, M. Wessling, J. H. Lee and A. Mitsos, Green Chem., 2020, 22, 3842–3859 Search PubMed.
- A. Kendall, G. A. Keoleian and G. E. Helfand, J. Infrastruct. Syst., 2008, 14, 214–222 Search PubMed.
- C. Fernández-Dacosta, M. van der Spek, C. R. Hung, G. D. Oregionni, R. Skagestad, P. Parihar, D. T. Gokak, A. H. Strømman and A. Ramirez, J. CO2 Util., 2017, 21, 405–422 Search PubMed.
- K. S. Wolske, K. T. Raimi, V. Campbell-Arvai and P. S. Hart, Clim. Change, 2019, 152, 345–361 Search PubMed.
- K. T. Raimi, A. Maki, D. Dana and M. P. Vandenbergh, Environmental Communication, 2019, 13, 300–319 Search PubMed.
- V. Campbell-Arvai, P. S. Hart, K. T. Raimi and K. S. Wolske, Clim. Change, 2017, 143, 321–336 Search PubMed.
- L. Engelmann, K. Arning, A. Linzenich and M. Ziefle, Front. Energy Res., 2020, 8, 579814 Search PubMed.
- J. Offermann-van Heek, K. Arning, A. Sternberg, A. Bardow and M. Ziefle, Energy Policy, 2020, 143, 111586 Search PubMed.
- K. Arning, J. Offermann-van Heek, A. Sternberg, A. Bardow and M. Ziefle, Environ. Innov. Soc. Transit., 2020, 35, 292–308 Search PubMed.
- C. R. Jones, B. Olfe-Kräutlein, H. Naims and K. Armstrong, Front. Energy Res., 2017, 5, 11 Search PubMed.
- C. R. Jones, R. L. Radford, K. Armstrong and P. Styring, J. CO2 Util., 2014, 7, 51–54 Search PubMed.
-
Council on Foreign Relations, Digital Decarbonization: Promoting Digital Innovations to Advance Clean Energy Systems, 2018 Search PubMed.
- L. Joos, J. M. Huck, V. Van Speybroeck and B. Smit, Faraday Discuss., 2016, 192, 391–414 Search PubMed.
- S. Deutz and A. Bardow, Nat. Energy, 2021, 6, 203–213 Search PubMed.
- L. Müller, A. Kätelhön, S. Bringezu, S. McCoy, S. Suh, R. Edwards, V. Sick, S. Kaiser, R. Cuellar-Franca, A. El Khamlichi, J. H. Lee, N. von der Assen and A. Bardow, Energy Environ. Sci., 2020, 13(9), 2979–2992 Search PubMed.
- J.-T. Anyanwu, Y. Wang and R. T. Yang, Ind. Eng. Chem. Res., 2020, 59, 7072–7079 Search PubMed.
- C. Rosu, S. H. Pang, A. R. Sujan, M. A. Sakwa-Novak, E. W. Ping and C. W. Jones, ACS Appl. Mater. Interfaces, 2020, 12, 38085–38097 Search PubMed.
- V. Y. Mao, P. J. Milner, J. H. Lee, A. C. Forse, E. J. Kim, R. L. Siegelman, C. M. McGuirk, L. B. Porter-Zasada, J. B. Neaton, J. A. Reimer and J. R. Long, Angew. Chem., Int. Ed., 2020, 59, 19468–19477 Search PubMed.
- D. W. Keith, G. Holmes, D. St. Angelo and K. Heidel, Joule, 2018, 2, 1573–1594 Search PubMed.
- S. Jin, M. Wu, R. G. Gordon, M. J. Aziz and D. G. Kwabi, Energy Environ. Sci., 2020, 13, 3706–3722 Search PubMed.
- M. T. Ho, G. W. Allinson and D. E. Wiley, Ind. Eng. Chem. Res., 2008, 47, 1562–1568 Search PubMed.
- L. Legrand, O. Schaetzle, R. C. F. de Kler and H. V. M. Hamelers, Environ. Sci. Technol., 2018, 52, 9478–9485 Search PubMed.
-
M. Blakeslee, Global Carbon Capture Activity, University of Michigan, 2021 Search PubMed.
-
International Energy Agency, CCUS in Clean Energy Transitions, International Energy Agency, Paris, France, 2020 Search PubMed.
- S. Pacala and R. Socolow, Science, 2004, 305, 968–972 Search PubMed.
- Z. Zhang, T. Wang, M. J. Blunt, E. J. Anthony, A.-H. A. Park, R. W. Hughes, P. A. Webley and J. Yan, Appl. Energy, 2020, 278, 115627 Search PubMed.
-
B. Gates, How to avoid a climate disaster: the solutions we have and the breakthroughs we need, Alfred A. Knopf, New York, 2021 Search PubMed.
-
A. J. Hoffman, Management as a calling, Stanford University Press, 2021 Search PubMed.
|
This journal is © The Royal Society of Chemistry 2021 |
Click here to see how this site uses Cookies. View our privacy policy here.