DOI:
10.1039/D0QO00659A
(Review Article)
Org. Chem. Front., 2020,
7, 3027-3066
Recent advances in the synthesis of xanthones and azaxanthones
Received
3rd June 2020
, Accepted 25th July 2020
First published on 27th July 2020
Abstract
Xanthones are important O-heteroaromatic tricyclic molecules exhibiting a wide range of bioactivities and can represent a showcase to highlight the recent techniques and strategies in organic synthesis towards lead discovery and optimization. This review gives an insight into the recent literature disclosed to obtain the xanthone core, including the drug-like azaxanthones, isosteres of xanthones, by exploiting the optimization of well-known procedures as well as disruptive developed methodologies. With this review, it is expected to provide a useful chemical toolbox to synthetic medicinal chemistry focusing on these privileged structures, covering from 2012 to 2020, with some older examples of azaxanthones.
1 Introduction
Over the past few decades, strategic advances have been made in medicinal chemistry and organic synthesis that accelerated drug discovery. Diversity-oriented synthesis, photo-induced reactions, biorthogonal chemistry with new and eco-friendly reagents, late-stage diversification, and artificial intelligence are among the approaches involved in some of the recent breakthroughs in drug discovery.1,2 More than 85% of all biologically-active chemical entities contain a heterocycle which provides a useful tool for lead optimization.3,4 This current review aims to emphasize a particular class of heterocycles, xanthones; the use of these advances allow one to speed up their synthesis and lead optimization process.
Xanthones are naturally occurring compounds that can be found as secondary metabolites in diverse terrestrial and marine plants, fungi, and lichen.5–8 Chemically, xanthones (9H-xanthen-9-ones) comprise a family of compounds with an oxygen-containing dibenzo-γ-pyrone heterocyclic scaffold (Fig. 1).9,10 As a privileged structure, this family of compounds is able to provide a wide range of different substitutions modulating several biological responses, thus being considered a promising and interesting structure for drug development.11,12 Azaxanthones (Fig. 1) are molecules with one or more nitrogen atoms placed in the aromatic moiety of the xanthone chromophore. N-Heterocycles, present in both natural products and synthetic compounds, constitute important structural motifs in medicinal chemistry, capable of showing a plethora of biological activities.13 This allied to the privileged O-heterocyclic xanthone scaffold, which can give rise to new molecules, gifted with interesting activities and drug-likeness, and ultimately to the discovery of new lead compounds.14 Azaxanthones also have advantages over acridones, such as being better chromophores and presenting a higher solubility in water.15 In terms of the structural diversity of N-heterocycles, azaxanthones can be classified into a six-membered ring group, if one of the rings is a pyridine or a pyrimidine, or into a five-membered ring group, if one of the rings is a pyrazole or a triazole (Fig. 1).
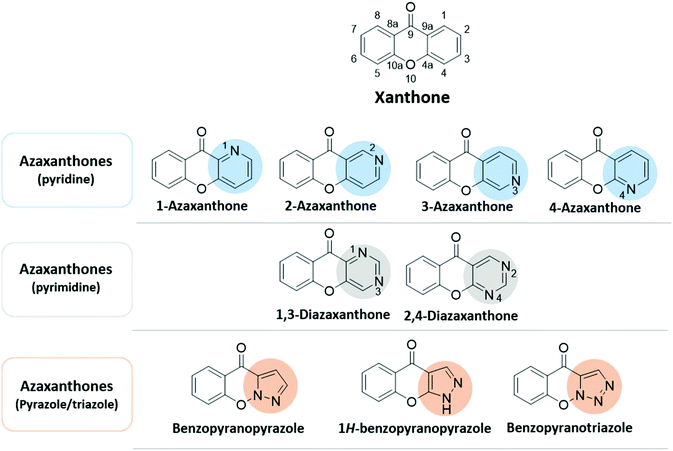 |
| Fig. 1 Xanthone and azaxanthone scaffolds. | |
In the last decade, both natural and synthetic xanthones have been reported with diverse biological and pharmacological properties,5,6,8,16 including antibacterial,8,17–27 antifungal,8,17,20,21,28–30 antiviral,31 antioxidant,32–37 antiobesity,38 anti-inflammatory,5,17,39–41 anticoagulant,42 and antitumour.36,43–58 Particularly, some xanthones’ molecular targets have been disclosed, including clinically important enzymes such as α-glucosidase,59–63 topoisomerase,64,65p-glycoprotein,66–68 and acetylcholinesterase33,69,70 and also protein–protein interactions, such as p53-MDM2.71–74 Similarly, a variety of biological activities was also disclosed for azaxanthones, depending on the side chains which can be found in their scaffolds. The activities described include antitumour,75,76 anti-allergic, bronchodilator, anti-inflammatory, and analeptic.77,78 In fact, one azaxanthone, amlexanox, was approved by the Food and Drug Administration (FDA) in 1996, as an antiallergic and anti-inflammatory immunomodulator, used for the treatment of aphthous ulcers.79 Although its mechanism of action is not fully understood, it is thought to inhibit the release of histamine and leukotrienes from white blood cells.80 Additionally, amlexanox and derivatives have shown potential as antitumour agents, particularly in tumour cells expressing one or more proteins of the S100 family, which include breast, colon, lung, pancreas, skin, oesophagus, bladder, and other tumours.
The first methods for the synthesis of xanthones were introduced by Michael and Kostanecki in 1883–189181,82 and in the 20th century and until 2012 several extensive reviews have reported the state of the art regarding the synthesis of xanthones.9,10,83,84 More recently reviews have focused on the synthesis of a very specific class of derivatives like carboxyxanthones,85 hydroxanthones,86 arylxanthones,87 and thioxanthones88 and, although azaxanthones have demonstrated therapeutic potential and interest as sensitizing chromophores,89–94 their reports in medicinal chemistry are still sparse in the literature. Therefore, in this review, the recent exploitation of both scaffolds was considered from a medicinal chemistry point of view, with advanced synthetic methodologies applied for obtaining bioactive derivatives being displayed in the sections below.
2 Synthesis of xanthones in one reaction step via condensation of two aryl building blocks
Methods for the synthesis of xanthones relying on a one-step methodology from readily available building blocks are still quite popular due to their simplicity and possibility of diverse substitution patterns. Four distinct approaches were developed and optimized over the last few years (DCXZ Scheme 1), involving the classical condensation of a salicylic acid with a phenol derivative (section 2.1), or involving an aryl aldehyde with a phenol derivative (section 2.2), a salicylaldehyde with 1,2-dihaloarenes (section 2.3), or an o-haloarenecarboxylic acid with arynes (section 2.4).
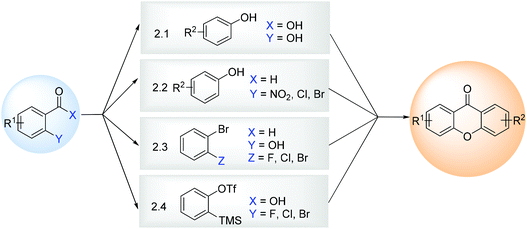 |
| Scheme 1 Methodologies for the synthesis of xanthones in one reaction step via condensation of two aryl building blocks. | |
2.1 Synthesis of xanthones by condensation of a salicylic acid with a phenol derivative
The classical Grover, Shah and Shah method95,96 constitutes a suitable approach for the synthesis of hydroxyxanthones from easily available salicylic acid derivatives and phenols heated along with zinc chloride in phosphoryl chloride.33,39,43,49,51,60,65,97–104 For the reaction to proceed directly to the desired xanthone, the 2,2′-dihydroxybenzophenone intermediate must contain an additional hydroxyl moiety ortho to the carbonyl group. Usually phloroglucinol is used in order to overcome this limitation; however, when other phenol derivatives like resorcinol are used, the synthetic pathway stops at the benzophenone form.53,105 Consequently, an additional cyclization step is required towards the conversion into xanthones. These and other limitations over this one-pot strategy towards simple xanthones led to modifications and development of new synthetic methods.9,10 Substitution of phosphorus oxychloride–zinc chloride by phosphorus pentoxide–methanesulfonic acid (Eaton's reagent) as an acylation catalyst generally led to better results.18,19,23,24,33,35,40,50,54,59,63,70,106–121 Since both of these methodologies had previously been extensively reviewed,9,10 they will not be discussed herein.
Rare earth metal triflates were used as unique Lewis acids to promote xanthone synthesis catalysed by Yb(OTf)3 hydrate from 2-substituted benzoic acids and differently substituted phenols under microwave irradiation (Scheme 2).122
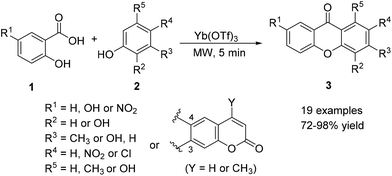 |
| Scheme 2 Synthesis of xanthones via a microwave assisted reaction catalysed by Yb(OTf)3. | |
Other metal triflates from the lanthanide series (e.g. lanthanum, europium, and gadolinium) led to the poorest yields, which can be explained by the fact that Yb3+ is the ‘hardest’ cation and thus the most oxophilic, due to its smaller ionic radius.122 Mechanistically, an initial Friedel–Crafts acylation can be considered towards the formation of a benzophenone intermediate, with further ring closure by displacement of the OH group coordinating the metal centre by the OH on the adjacent ring. Combination of salicylic, gentistic or 5-nitrosalicylic acids with m-cresol, orcinol, phloroglucinol, resorcinol, pyrogallol, p-nitrophenol, p-chlorophenol, 7-hydroxycoumarin or 4-methyl-7-hydroxycoumarin led to the corresponding xanthones 3 in high yields (19 examples, 72–98%).122
2.2 Synthesis of xanthones by condensation of an aryl aldehyde with a phenol derivative
Highly selective and efficient transition metals like copper and iron catalytic systems have been recently employed for the synthesis of xanthones due to the associated concept of eco-friendliness, considering atom economy, low toxicity and atom efficiency.123–126 In the scope of a study on the o-acylation of phenols with various aryl aldehydes using CuCl2 as the catalyst in the presence of triphenylphosphine, it was found that benzophenones were obtained when benzaldehyde or 3- or 4-substituted aryl aldehydes were employed. Nevertheless, when 2-substituted aryl aldehydes reacted with phenols, xanthones were obtained (Table 1, Method A), first via ortho-acylation of phenols with 2-substituted aryl aldehydes with further ring closure under basic conditions, with orto-substituents of the aryl aldehydes serving as leaving groups (LG).123 The scope of the reaction of 2-nitrobenzaldehydes and phenols was investigated after an initial screening of leaving groups, showing that electron-donating groups (EDG) (alkoxy, alkyl, aryl, and halides) were well tolerated on phenols, promoting the reaction. On the other hand, electron-withdrawing groups (EWG) (NO2 or CN) block the reaction completely, the main drawback of this methodology.123 Although these results are promising for eco-friendly reasons, they still employ ligands and expensive catalysts which are not recycled. To surpass these disadvantages, Menendéz et al.124 optimized the reaction conditions using a magnetic nanocatalyst consisting of CuNPs (NP = nanoparticles) on silica coated maghemite to catalyze the reaction between ortho-substituted benzaldehydes and phenols (Table 1, Method B). Provided with a high surface area, nanocatalysts were used to afford a more significant advancement in catalytic activity over conventional catalytic methods. This synthetic protocol was compatible with the presence of a variety of functional groups in the starting phenolic building blocks, affording the desired xanthones in good to excellent yields, with recovery of the catalyst.124 Ramani125 used Fe3O4 nanoparticles under ligand-free conditions to catalyze o-benzoylation of phenols with aryl aldehydes for the synthesis of xanthones, with lower yields than the reported for the latter two methodologies (Table 1, Method C). Later, Venkanna126 used magnetically separable nano-CuFe2O4 to catalyze o-acylation (Table 1, Method D). Xanthone derivatives were generally obtained in good yields from the condensation of diverse phenols with 2-nitrobenzaldehydes using nano-CuFe2O4 as the catalyst, K3PO4 and DMF at 80 °C, in an open flask, for 10 h. Comparing the four methodologies (Table 1), and looking at the characteristics of eco-friendliness, reaction conditions and yields, methods B and D seem to be more suitable for the synthesis of xanthones from 2-nitrobenzaldehydes and phenols, with a good tolerance for different substituent groups on the phenol moiety.
Table 1 Synthesis of xanthones via a one-step o-acylation of phenols with 2-substituted aldehydes
2.3 Synthesis of xanthones by condensation of a salicylaldehyde and 1,2-dihaloarenes
Aside the o-acylation of phenols with 2-substituted aldehydes, the xanthone core can also be constructed through a selective palladium-catalysed acylation/nucleophilic aromatic substitution (SNAr) approach of salicylaldehyde derivatives with aryl halides (Scheme 3).127,128 The first methodology reported consisted of the use of a bulky electron-rich trialkylphosphine ligand, n-BuPAd2, which prevents the decomposition of the palladium catalyst, observed with the use of other ligands.128 The scope of the reaction was extended to 22 different xanthones (41–81% yield); however, only activated salicylaldehydes were tolerated, long reaction times (12 h) were needed, and it was impractical to recover and reuse the catalyst. A rapid and convenient ligand-free alternative was later reported for this direct annulation using a recoverable palladium nanocatalyst supported on a green biochar under microwave irradiation, with a drastic reduction in reaction times.127 This eco-friendly strategy proceeded in very good yields (54–88%) and with high regioselectivity for a scope of 20 xanthone derivatives.
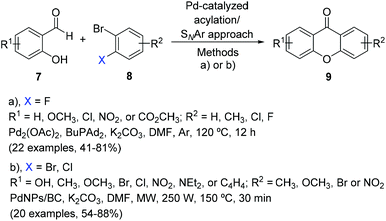 |
| Scheme 3 Synthesis of xanthones via the palladium-catalysed acylation/SNAr approach. | |
2.4 Synthesis of xanthones by condensation of o-haloarenecarboxylic acids with arynes
Dubrovskiy and Larock129 reported the synthesis of xanthones via an intermolecular C–O addition of o-halobenzoic acids 10 to arynes 11 employing CsF and THF at 125 °C for 24 h. Although o-chloro and o-iodo benzoic acids provided poor yields, o-fluorobenzoic acid afforded the desired xanthones 12 in good yields (Scheme 4). Diversification of substituents either in the benzoic acid building block or in the aryne moiety was well tolerated, and nine different xanthone derivatives were obtained, including an azaxanthone.
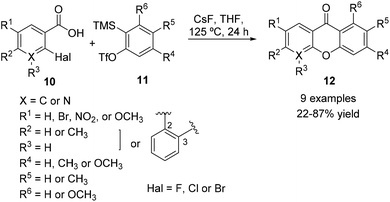 |
| Scheme 4 Synthesis of xanthones via an intermolecular C–O addition of o-halobenzoic acids to arynes. | |
3 Synthesis of (aza)xanthones via the benzophenone route
The limitations of some of the earlier one-step methodologies (e.g. Grover, Shah and Shah, section 2.1) led to the development of several multi-step approaches. The synthesis of xanthones via the benzophenone route is one of the most used approaches in which the benzophenone derivatives are easily accessed through (poly)oxygenated aromatic compounds with a benzoic acid derivative (section 3.1.1), followed by the cyclization of the benzophenone (section 3.1.2) into the xanthone scaffold (Scheme 5), usually achieved by base-catalysed intramolecular nucleophilic aromatic substitution. Considering the high versatility of this route, examples of the total synthesis of natural products using this strategy in the last decade130–136 are presented in Fig. 2.
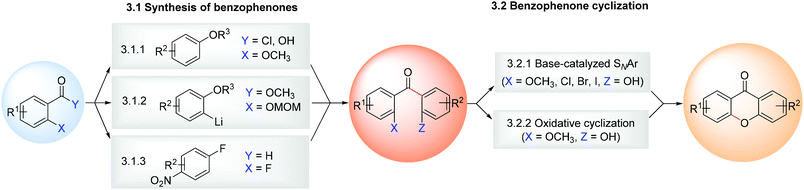 |
| Scheme 5 Strategies for the synthesis of xanthones via benzophenone. | |
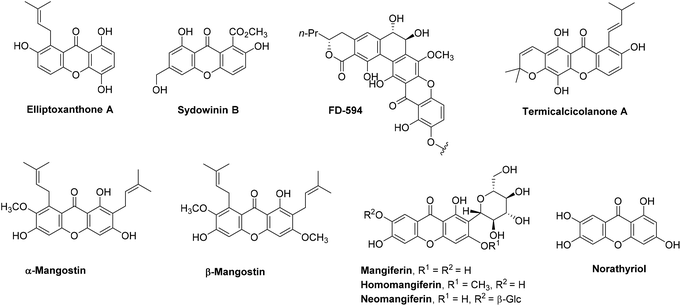 |
| Fig. 2 Recent examples of the total synthesis of natural products using the benzophenone route. | |
3.1 Synthesis of xanthones
3.1.1 Synthesis of benzophenones.
The Friedel–Crafts acylation continues to be the method of choice for the synthesis of the benzophenone moiety, when xanthones are obtained via a benzophenone route (section 3.1.1.1).28,47,66,71,137–140 Additional approaches for the synthesis of the benzophenone moiety include the halogen–lithium exchange (section 3.1.1.2) and NHC-catalysed aroylation (section 3.1.1.3) (Scheme 6).
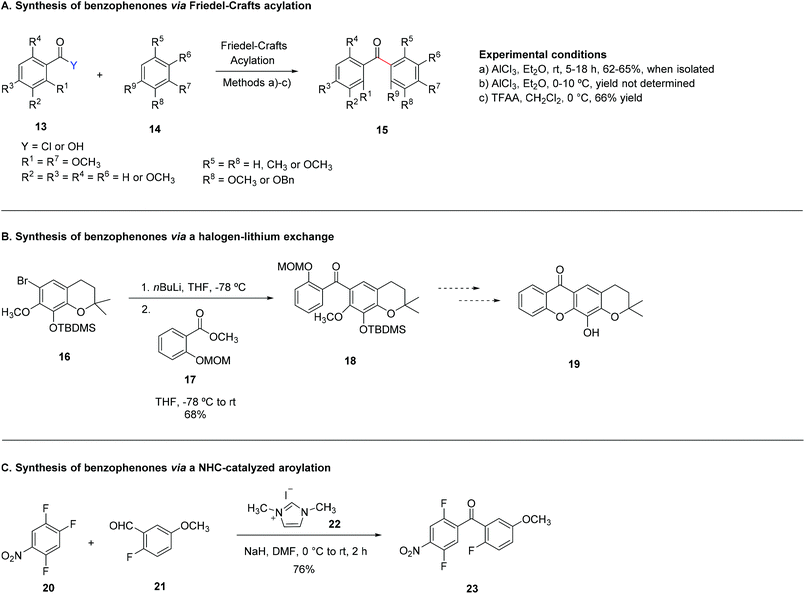 |
| Scheme 6 Synthesis of benzophenones. | |
Other methodologies were reported for the synthesis of benzephenones via an o-acylation of phenols using copper123 and iron-mediated125 approaches, or via a reductive decarbonylative coupling.141 However, further investigations led to the attainment of the corresponding xanthone in one step and, therefore, they are addressed in the appropriate sections (section 2.2 and section 7.1).
3.1.1.1 Synthesis of benzophenones via Friedel–Crafts acylation of (poly)oxygenated aromatic compounds with a benzoic acid derivative.
Over the last few years, several examples of the synthesis of benzophenones via Friedel–Crafts acylation have been reported, mainly via classical reaction conditions employing an acid chloride and AlCl3 as a Lewis acid catalyst (Scheme 6A).28,47,66,71,137–139When two methoxy groups are present in both R1 and R9 three different benzophenone derivatives can be formed: a benzophenone methoxylated at R1 and R9, and two benzophenone derivatives which result from the demethylation of the methoxy group in R1 or R9 (position peri-carbonyl). Hence, to avoid losses, usually the benzophenone derivatives are not isolated and the reaction crude is used directly towards cyclization to xanthones.28,66,71,138 Other interesting strategies have been developed in the last few years for the synthesis of benzophenones, worth highlighting being the one in which the carbonyl bridge is introduced via a mild Friedel–Crafts reaction using trifluoroacetic anhydride (TFAA) (Scheme 6A).140 This methodology was developed in the scope of a study to find novel PGAM1 inhibitors and involves the in situ formation of a mixed anhydride that results from the reaction of the benzoic acid derivative with TFAA, in order to make the carbonyl adjacent to the aromatic ring more susceptible to nucleophilic attack.140 Due to the limitations in the tolerated substituents that can be present at either the benzoic acid derivative or the (poly)oxygenated aromatic compound in order for cyclization to occur, this methodology is usually employed as the starting point for the synthesis of more complex xanthone derivatives.
3.1.1.2 Synthesis of benzophenones via a halogen–lithium exchange.
The halogen–lithium exchange benzophenone cyclization is not used as commonly as the Friedel–Crafts acylation, but can still be considered a valuable tool for the synthesis of benzophenone derivatives. Studies on the synthesis of 12-hydroxy-2,2-dimethyl-3,4-dihydropyran[3,2b]xanthene-6(2H)-one (19), a p53-MDM2 inhibitor,74 led to benzophenone 18, obtained from halogen–lithium exchange of the previously synthesized benzopyran 16 and O-protected MOM (methoxymethyl) benzyl salicylate 17 (Scheme 6B).46
3.1.1.3 Synthesis of benzophenones via a NHC-catalysed aroylation.
N-Heterocyclic carbenes (NHC) can be employed both as ligands for transition-metal systems or as small-molecule organocatalysts for chemical synthesis. In a nucleophilic aroylation catalysed by NHC 22, the 4-fluoro group of trifluoronitrobenzene (20) is replaced by the aroyl group originating from aldehyde 21 to afford the corresponding benzophenone 23 (Scheme 6C).133 This versatile methodology was applied in the total synthesis of termicalcicolanone A (Fig. 2), an anticancer natural xanthone.133
3.1.2 Benzophenone cyclization.
The base-catalysed intramolecular nucleophilic aromatic substitution is undoubtedly the most frequently used strategy for the cyclization of benzophenones to xanthones (section 3.1.2.1). This methodology involves the presence of a hydroxyl group in position 2 and a functional group, normally a methoxy, which can behave as a leaving group in position 2′ of the benzophenone, usually in the form of methanol. Other methodologies include oxidative cyclization (section 3.1.2.2) with new and greener reaction conditions being reported in the last few years.
3.1.2.1 Cyclization of benzophenones to xanthones via a base-catalysed intramolecular nucleophilic aromatic substitution.
Although the classical methodologies are widely used,130,132,137–140 some improvements, especially in terms of yields and shorter reaction times, were achieved with microwave-assisted organic synthesis (MAOS).28,46,47,66,71 Common bases used in this strategy are NaOH,28,47,66,71,138 tetramethylammonium hydroxide (TMAH),137,140 and K2CO3.132,139 A greener approach was also reported using a combination of base/H2O142 (Scheme 7A).
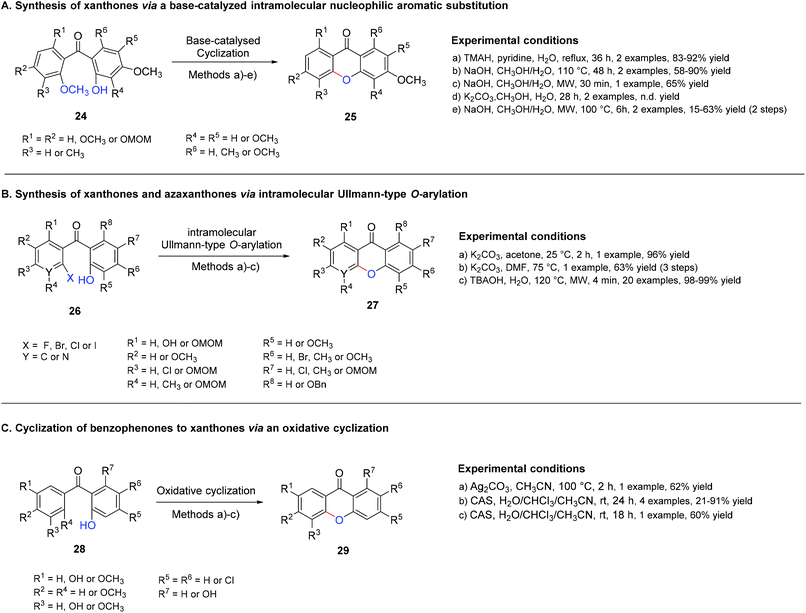 |
| Scheme 7 Synthesis of xanthones via benzophenone cyclization. | |
In the presence of 2-halo-2′-hydroxybenzophenones, a transition-metal-free intramolecular Ullmann-type O-arylation reaction (Scheme 7B) can be employed for the synthesis of the corresponding xanthones 27, either using K2CO3 or tetrabutylammonium hydroxide (TBAOH) as a base.143 The latter is an efficient, rapid, and green method which uses microwave irradiation, resulting in a short reaction time and excellent yields. The O-arylation reaction proceeds smoothly, regardless of the nature of the halogen leaving group present in the aromatic moiety, with several functional groups being well-tolerated in all the examples, and extendable to the synthesis of azaxanthones. Although the desired xanthones 27 were produced in comparatively lower yields when in the presence of 1-hydroxynapthalene, in 4 min under MW irradiation, quantitative yields could be obtained when the reaction time was extended to 8 min. Azaxanthone was prepared in two minutes with an excellent yield (98%).143
3.1.2.2 Cyclization of benzophenones to xanthones via an oxidative cyclization.
Despite not being used as often as the base-catalysed intramolecular nucleophilic aromatic substitution, methodologies based on the oxidative cyclization of the benzophenone are also employed for the synthesis of xanthone derivatives. Studies on the cyclization of the readily available model substrate 2,3′,5-trihydroxy benzophenone 28 revealed that the combination of Ag2CO3 and CH3CN, at 100 °C for 2 h gave the best results towards the cyclization product, with the hydroxyl group being crucial for cyclization that occurs via a single-electron transfer mechanism144 (Scheme 7C). To obtain 1,5,7-trisubstituted-3-pyridylxanthones with potential as insecticides, the target precursor 1,5,7-trihydroxy-3-chloroxanthone was cyclized using the optimized reaction conditions, in the route for the synthesis of several 1,5,7-trisubstituted-3-pyridylxanthones.144 Ceric ammonium sulfate (CAS)145,146 and ceric ammonium nitrate (CAN)146-mediated oxidative cyclization of benzophenones was also reported, although some limitations were observed with the formation of a xanthone-related dione. A possible mechanism for the formation of this secondary product was proposed with the formation of a radical cation with subsequent nucleophilic addition of the phenol, oxidation with CAN, and addition of water followed by elimination of methanol.145
3.2 Synthesis of azaxanthones
The synthesis of azaxanthones via the benzophenone route usually employs the preparation of the required benzophenone through Friedel–Crafts acylation (Scheme 8A), deprotocupration–aroylation (Scheme 8B), or even the formation of an intermediate alcohol via metal-mediated strategies, which is further oxidized to the corresponding ketone (Scheme 8C and D). Cyclization is typically achieved via SNAr.
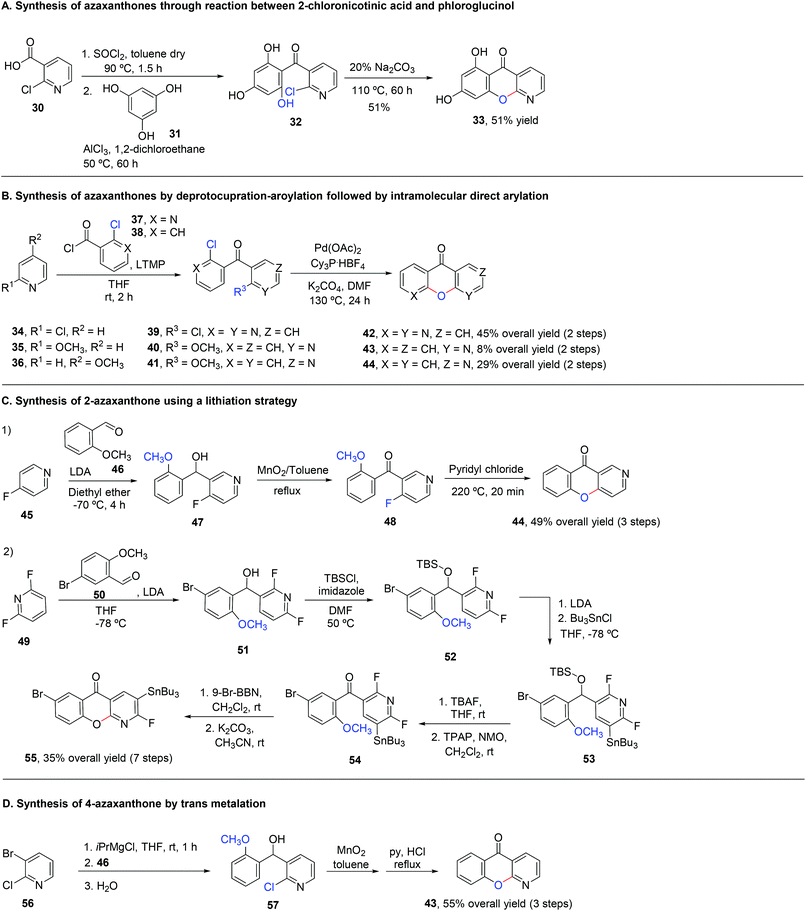 |
| Scheme 8 Synthesis of azaxanthones via the benzophenone route. | |
3.2.1 Synthesis of azaxanthones through the reaction between 2-chloronicotinic acid and phloroglucinol.
Kolokythas et al.76 have studied the potential of pyranoxanthenones and pyranothioxanthenones, as potential cytotoxic agents closely related to the broad spectrum antitumour alkaloid acronycine (Scheme 8A). As an outcome of this work, the carbocyclic moiety was replaced by the pyridine heterocycle isostere in the xanthone chromophore. Similarly to the work of Villani et al.,78 formation of the corresponding acyl chloride of 2-chloronicotinic acid (30) and further reaction with phloroglucinol 31 provided ketone 32 which was then cyclized to dihydroxyazaxanthone 33 in 51% yield.76
3.2.2 Synthesis of azaxanthones through deprotocupration–aroylation followed by intramolecular direct arylation.
Lithiocuprates, prepared from copper(II) chloride and lithium 2,2,6,6-tetramethylpiperidide (LTMP), can be used as bases for the deprotonative metalation of aromatic compounds. By using palladium as a catalyst for ring closure, it is possible to obtain not only the expected azafluorenones, but also azaxanthones, through cyclization, in the presence of oxygen-containing substituents or reagents.147 Synthesis of azaxanthones 42–44 was achieved by using this approach. 4,5-Diazaxanthone (42), was prepared from 2-chloropyridine (34) and LTMP, which was stirred for two hours at 0 °C in THF, before the addition of the corresponding aroyl chloride (Scheme 8B). The 4,5-diazaxanthone 42 was produced in 45% overall yield (2 steps) after treatment with palladium(II) acetate and triciclohexylphosphine tetrafluoroborate. The second azaxanthone, 4-azaxanthone (43), was synthesized similarly, starting from 2-methoxypyridine (35) and chlorobenzyl chloride in 8% overall yield (2 steps). The second step was slightly different, and although Pd(OAc)2 was used as a catalyst, tri-tert-butylphosphonium tetrafluoroborate was also employed (Scheme 8B). 2-Azaxanthone (44) was obtained through the same method as 4,5-diazaxanthone (42), using 4-methoxypyridine (36) and chlorobenzyl chloride (Scheme 8B), yielding 29% of the desired compound (overall yield for 2 steps).147
3.2.3 Synthesis of azaxanthones through metalation–lithiation.
Metalation of π-deficient heterocycles, such as pyridine, has proven to be a good strategy towards obtaining compounds that could serve as the starting materials for azaxanthones. This methodology can be a powerful functionalization approach, since electrophilic substitutions are frequently hard to achieve in such molecules. Based on the fact that halogens can induce o-lithiation of pyridine, Marsais et al.148 explored this route and one of their experiments led to the synthesis of 2-azaxanthone (44). The metalation of the starting material, 4-fluoropyridine (45), was achieved with complete chemoselectivity using lithium diisopropylamide (LDA) and o-anisaldehyde (46). The formation of butylated side products when using butyllithium attested the advantages of using LDA as a lithiation agent. The fact that the product, (4-fluoro-3-pyridyl)-2-methoxyphenylmethanol (47), precipitated with diethyl ether also constituted an advantage, as higher metalation yields could be reached. Oxidation of 47 with manganese dioxide led to (4-fluoro-3-pyridyl)-2-methoxyphenylmethanone (48) which when heated with pyridinium chloride yielded 2-azaxanthone (44), in 49% overall yield, obtained over 3 steps (Scheme 8C1).148 A similar approach was used for the synthesis of halo-azaxanthones, starting from 2,6-difluoropyridine (49) and 5-bromo-2-methoxybenzaldehyde (50). A tert-butyldimethyl silyl (TBS) ether protection of the formed hydroxyl moiety and installation of a Bu3Sn group after the second fluorine-directed o-lithiation of the pyridine were used as key steps in the synthesis of trisubstituted azaxanthone 55 in 35% yield (overall yield considering 7 steps) (Scheme 8C2).149
3.2.4 Synthesis of azaxanthones through transmetalation.
It is clear that metalation reactions are useful, with direct reactions with electrophiles or transmetalation, leading to cross-coupling reactions, being the most important. However, the lithiation reactions previously described require low temperatures, and, added to the fact that halogen–lithium exchange is extremely temperature-sensitive, that can be limiting, making them difficult to perform in an industrial setting.150 Therefore, the search for alternative reactions led to the development of pyridylmagnesium reagents that could either take part in electrophilic trapping or coupling reactions. In this scope, the halogen–magnesium exchange methodology was preferred over the Grignard reaction, as the direct access by oxidative addition of magnesium to the halopyridine is challenging to accomplish, even with magnesium activation.150 Studies on synthetic routes leading to new pyridine derivatives through bromine–magnesium exchange.148,150 revealed that isopropylmagnesium chloride (iPrMgCl) was the best exchange reagent, and THF was the best solvent. The fact that this reaction occurs at room temperature posed as an advantage. 4-Azaxanthone (43) was prepared starting from 3-bromo-2-chloropyridine (56) (Scheme 8D). Metalation with iPrMgCl, using THF as the solvent, at room temperature and further reaction with o-anisaldehyde (46) resulted in the formation of alcohol (57), which was then subject to oxidation and ring closure reactions affording 43 as described before, in 55% overall yield (3 steps).
4 Synthesis of (aza)xanthones via the diaryl ether route
The diaryl ether synthesis comprises the reaction between aryl halides and phenols which are further cyclized to the xanthone core. Like the benzophenone route, the diaryl ether route can then be divided into two steps: the synthesis of the diaryl ether (section 4.1.1) and the cyclization of the diaryl ether (section 4.1.2) (Scheme 9). This approach is particularly useful in the synthesis of 1,2-dioxygenated xanthones, when the pattern of substitution of the target xanthone cannot be obtained following the electrophilic aromatic substitution rules and/or is also dependent on the presence of bulky groups giving rise to isomeric mixtures. Nevertheless, due to generally higher yields obtained with intermolecular acylations when compared to the Ullmann ether syntheses, the recent examples of the total synthesis of natural products using the diaryl ether route are quite scarce, contrary to the existing ones via the benzophenone route (Fig. 3).
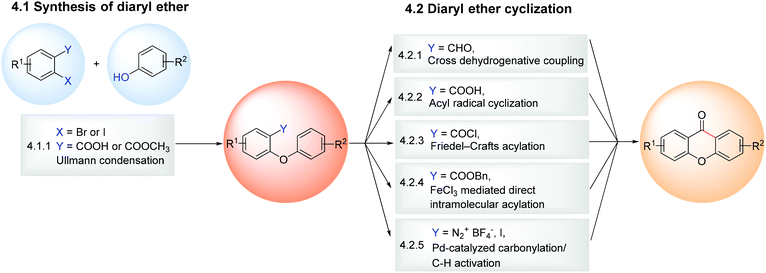 |
| Scheme 9 Strategies for the synthesis of xanthones via diaryl ether. | |
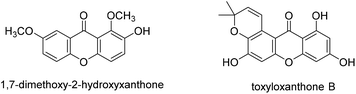 |
| Fig. 3 Recent examples of the total synthesis of natural products using the diaryl ether route. | |
4.1 Synthesis of xanthones
4.1.1 Diaryl ether synthesis.
With the extensive development of several methodologies towards xanthone synthesis, either through the benzophenone route (section 3) or even by one-step methodologies (section 2) or newer strategies (section 7), fewer examples involving other procedures have been described in recent times. When used, diaryl ether synthesis is usually achieved via the classic Ullmann condensation (section 4.1.1.1), which consists of the coupling of an aryl halide with a phenol (Scheme 9).
4.1.1.1 Synthesis of diaryl ether by coupling of an aryl halide with a phenol – Ullmann condensation.
While some modifications of the coupling of an aryl halide with a phenol using Ullmann conditions have been described in the last few years, copper continues to be the metal of choice for this coupling. Common bases for Ullmann type reactions are K2CO3 and Cs2CO3 and some variations in the copper catalyst include the use of CuI,45,151 CuO,152 CuCl153 or Cu/Cu2O.154 Since this methodology has already been extensively reviewed, recent examples are summarized in Scheme 10 and will not be widely discussed. However, it is worth mentioning a new copper-(I)-catalysed approach that, when compared to the classical Ullmann reaction protocols, requires only small amounts of copper catalyst and mild temperatures, with better overall yields, even when tested on a larger scale. The Buchwald's Cu(OTf)2–benzene complex proved to be extremely effective against the classic CuI when combined with 4-dimethylaminopyridine (4-DMAP), to give the desired diaryl ether in high yields.155 One of the synthesized xanthones showed typical xanthone fluorescence properties suitable for studying folding processes of polypeptides by triplet–triplet energy transfer (TTET).155
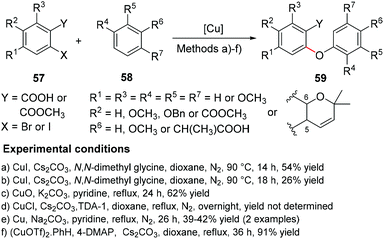 |
| Scheme 10 Synthesis of diaryl ether by Ullmann condensation. | |
4.1.2 Cyclization of the diaryl ether.
To be used as substrates in the synthesis of xanthones, diaryl ethers should contain certain molecular characteristics so that the next step, cyclization, can occur. Over the last few years, several approaches to the xanthone skeleton from a range of functionalized diaryl ethers via various mechanisms have been developed, depending largely on the functionality existing in the ortho position to the ether (Scheme 9, Y). When in the presence of an aldehyde derivative (Y = CHO), the most common methodology is via cross-dehydrogenative coupling (CDC, section 4.1.2.1). This strategy has received increasingly attention in recent years due to the fact that it does not require pre-functionalized coupling partners, can be extended to a wide scope of derivatives, and involves a few steps, and consequently high atom economy. In the presence of a carboxylic acid derivative (Y = COOH, COCl, COOBn), three approaches can be followed, depending on the nature of the derivative: an acyl radical cyclization via photoredox catalysis using methylene blue as a photosensitizer (section 4.1.2.2, Y = COOH), a metal-free variant of the intramolecular Friedel–Crafts acylation (section 4.1.2.3, Y = COCl), and a FeCl3 mediated direct intramolecular acylation of esters (section 4.1.2.4, Y = COOBn). However, the presence of a carboxylic acid derivative is not mandatory, and other substrates such as 2-iodo diaryl ethers (Y = I) or the corresponding diazonium salts (Y = N2+ BF4−) can be used for the synthesis of xanthones in a palladium-catalysed carbonylation/C–H activation (section 4.1.2.5). Other kinds of cyclization strategies have also been reported in which the xanthone core can be obtained through a Co(I)-catalysed Barbier reaction of aromatic halides with aromatic aldehydes and imines,156 a PhI(OAc)2–BF3–OEt2 mediated domino imine activation, intramolecular C–C bond formation and β-elimination,157 or a quaternary ammonium salt-promoted intramolecular dehydrogenative arylation of aldehydes.158
4.1.2.1 Cyclization of diaryl ethers to xanthones by a cross-dehydrogenative coupling.
After the pioneering work of Wang et al.159 in 2012 on the synthesis of xanthones via a rhodium-catalysed cross-dehydrogenative coupling (CDC) starting from 2-aryloxybenzaldehydes, alternative transition-metal catalysed strategies to construct the xanthone scaffold have been developed (Scheme 11)160–163 For example, Wertz et al.160 reported an elegant and straightforward procedure for the synthesis of the xanthone core through a FeCp2-catalysed CDC reaction via a base-promoted homolytic aromatic substitution. Under the optimized conditions, several 2-aryloxybenzaldehydes bearing both electron-donating and electron-withdrawing substituents were coupled efficiently to give the corresponding xanthones with yields ranging from 30 to 78% (Scheme 11). Later, Manna et al.161 reported an ortho C–H bond functionalization involving dichloro(p-cymene)ruthenium(II) dimer [[RuCl2(p-cymene)2]2 catalysed C–C bond formation. When compared to other metal-mediated CDC methodologies, this protocol does not require any expensive metal-catalyst and uses a commercially available cheap oxidant. The authors extended the scope of this reaction not only to xanthones, but also to thioxanthones and fluorenones in moderate to good yields.161 Recently, Bao et al.162 described a Cu(0)/Selectfluor system-catalysed intramolecular Csp2–H/Csp2–H bond CDC of 2-aryloxybenzaldehydes (Scheme 11).
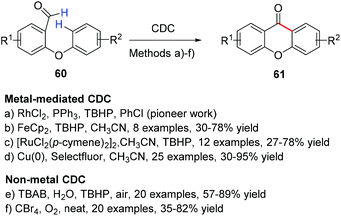 |
| Scheme 11 Methodologies for the synthesis of xanthones through intramolecular cross-dehydrogenative coupling (CDC) of 2-aryloxybenzaldehydes. | |
Interestingly, when in the presence of a substituent in the ortho position to the formyl group on the 2-aryloxybenzaldehyde, regioisomers were obtained. The presence of substituents at the ortho, meta, or para positions relatively to the formyl group are well tolerated, the products being obtained in good yields, except in the case of OPh, affording the desired product in lower yield (49%). Besides the clear advantages related to the use of an inexpensive and low-toxic copper catalyst and relatively mild reaction conditions, this procedure also accepts a wide variety of substituent groups such as F, Cl, Br, OCF3, CF3, Ph, OPh, iPr, and iBu, which provides opportunities for further molecular modifications. The authors were able to expand the use of this methodology for the synthesis of 9H-thioxanthen-9-one and 10,10-dioxide and phenanthridin-6(5H)-one derivatives162 and, interestingly, this process could be conducted successfully on a gram scale for a simple oxygenated xanthone (80% yield). A plausible mechanism for the [RuCl2(p-cymene)2]2-catalysed cyclization reaction was proposed and involves the formation of a cyclic seven membered transition state by addition of TBHP followed by reductive elimination to afford the desired compounds 61.161
Over the last few years, the development of green CDC methodologies has sprout in order to compete with these transition-metal catalysed approaches (Scheme 11) and to address some drawbacks of their use in the pharmaceutical industry (metal harsh elimination and consequent toxicity). Rao et al.164 reported a metal-free tetrabutylammonium bromide (TBAB)-promoted intramolecular annulation in aqueous medium, via oxidative coupling of C–H bond/aromatic C–H of several 2-aryloxybenzaldehydes. After reaction condition optimization, this reaction tolerated both electron-donating and electron-withdrawing functional groups (Scheme 11). Interestingly, when 2-(4′-phenoxyphenoxy)-benzaldehyde was employed, the formyl C–H bond selectively coupled with the 2-phenoxy part to give the phenoxy-9H-xanthen-9-one while leaving the 4′-phenoxy part intact, attesting that the intramolecular route is favored by the oxidative coupling protocol.
In a related investigation, Tang et al.165 developed a straightforward ring closure protocol for the synthesis of xanthones from 2-aryloxybenzaldehydes through a simple and practical carbon tetrabromide promoted intramolecular aerobic oxidative dehydrogenative coupling reaction (Scheme 11). The scope and versatility of this reaction were also investigated, by experimenting different solvents, halogen reagents, and substituents, with good tolerance for different functional groups, being inclusively extended for the synthesis of fluorenones by using 2-arylbenzaldehydes as substrates. After preliminary studies on the reaction mechanism, the authors also suggested that the reaction may proceed through a radical pathway.165
4.1.2.2 Cyclization of diaryl ethers to xanthones by an acyl radical cyclization.
Although CDC is the most frequently used methodology for the synthesis of xanthones from diaryl ethers, other less explored methodologies, with substrates containing a carboxylic acid derivative moiety, have recently been reported. An efficient intramolecular radical cyclization reaction via photoredox catalysis was developed for the synthesis of dibenzocycloketone derivatives using methylene blue as a photosensitizer (Scheme 12A).166 This strategy relies on the fact that carboxylic acids are attractive and widely used synthons to prepare acyl radicals by decarboxylation through photoredox catalysis. The authors used 2-(2-phenoxyacetyl) benzoic acid as a model substrate to obtain the corresponding eight-membered dibenzocycloketones and, after reaction condition optimization, the scope of the reaction was extended to other six, seven and eight-membered dibenzocycloketones. Xanthone (63) was obtained in 75% yield through a reaction of 2-phenoxybenzoic acid (62) with methylene blue, PPh3 and 2,4,6-collidine in 2 mL DMA and irradiated with LED white light at 25 °C for 24 h.166
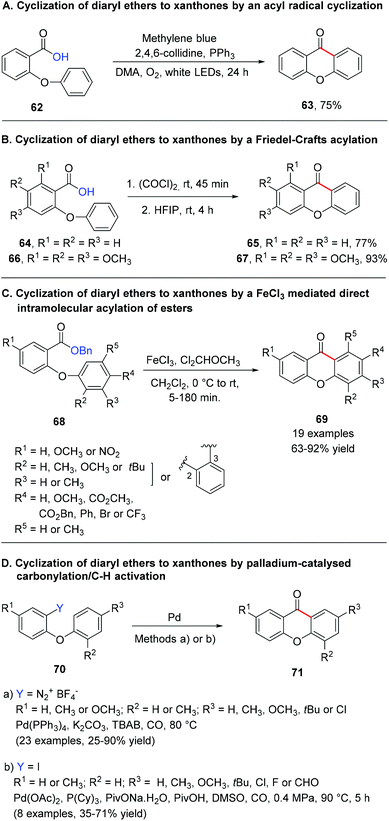 |
| Scheme 12 Methodologies used for the cyclization of diaryl ethers to xanthones. | |
4.1.2.3 Cyclization of diaryl ethers to xanthones by a Friedel–Crafts acylation.
A promising metal-free variant of the intramolecular Friedel–Crafts acylation reaction, which employs mild conditions and avoid excesses of harsh acids, was described by Motiwala et al.167 This simple strategy consists of dissolving readily available acid chlorides in 1,1,1,3,3,3-hexafluorpropan-2-ol (HFIP), a strong hydrogen-bond-donating solvent, and allows the reaction to stir at room temperature for 4 h (Scheme 12B). The reaction was optimized using 4-(3,4-dimethoxyphenyl)butanoic acid as a model against solvent, HFIP loading, and reaction time to obtain 6,7-dimethoxy-1-tetralone. After the optimal conditions were obtained, the scope was extended to a range of structurally diverse carboxylic acids; the formation of six-membered rings was generally favored over five- and seven-membered rings, xanthone 65 being obtained in 77% yield and 1,2,3-trimethoxy-9H-xanthen-9-one (67) in 93% yield (Scheme 12B).
4.1.2.4 Cyclization of diaryl ethers to xanthones by a FeCl3 mediated direct intramolecular acylation of esters.
Benzyl esters have also shown to be suitable building blocks for the synthesis of xanthone derivatives. This serendipitous example of cyclization of diaryl ethers bearing a carboxylic acid moiety emerged as a way to dodge the disadvantages sometimes associated with the classical methods.168 After an initial screening concerning several catalysts, dichloromethyl methyl ether loading, and reaction times, and with the optimal conditions in hand, the scope of the reaction was investigated and it was observed that the acylation could tolerate various functional groups such as OCH3, NO2, CO2CH3, Br, and Cl, affording the desired xanthones in good yields (Scheme 12C). Mechanistic studies indicated that the formation of an intermediate, which results from the cooperative activation of benzyl esters by FeCl3 and Cl2CHOCH3, plays a key role in the acylation of esters.
4.1.2.5 Cyclization of diaryl ethers to xanthones by palladium-catalysed carbonylation/C–H activation.
Although the previous examples approached the use of diaryl ethers bearing a carboxylic acid moiety, this is not mandatory and the carbonyl bridge between the two aryl rings can be formed with the aid of carbon monoxide. Thus, palladium catalysed carbonylations/C–H activation reactions emerged as an alternative methodology for the synthesis of xanthones in the last few years. The employment of a palladium catalyst (and ligand), base/additive, and carbon monoxide proceeds smoothly when using 2-iodo diaryl ethers or the corresponding diazonium salts to form the desired xanthones, with great tolerance for different functional groups (Scheme 12D). The proposed mechanism of the intramolecular cyclization is similar in both strategies and proceeds through the initial oxidative addition of Pd, insertion of CO, activation of an ortho aromatic C–H with the assistance of the base, subsequent reductive elimination to afford the intended xanthone, and regeneration of Pd for the next catalytic cycle.
4.2 Synthesis of azaxanthones
The synthesis of azaxanthones via the diaryl ether route requires the synthesis of an intermediate, typically a cyanopyridyl phenyl ether (Scheme 13) or a phenoxynicotinic acid derivative (Scheme 14). Cyanopyridyl phenyl ether derivatives can be obtained either through SNAr of aromatic halides with hydroxypyridine or phenol derivatives. Phenoxynicotinic acids are also achieved via SNAr, from building blocks containing the carboxylic acid moiety or other functionalities (e.g. methyl) that are easily converted into the desired carboxylic acid. Other strategies include the use of quinolinimide as the starting material, which can be further transformed into the corresponding carboxylic acid (Scheme 14). An excess of protic acid (often as solvent) such as sulfuric acid or polyphosphoric acid (PPA) is usually the reagent of choice for the intramolecular Friedel–Crafts cyclization reaction.
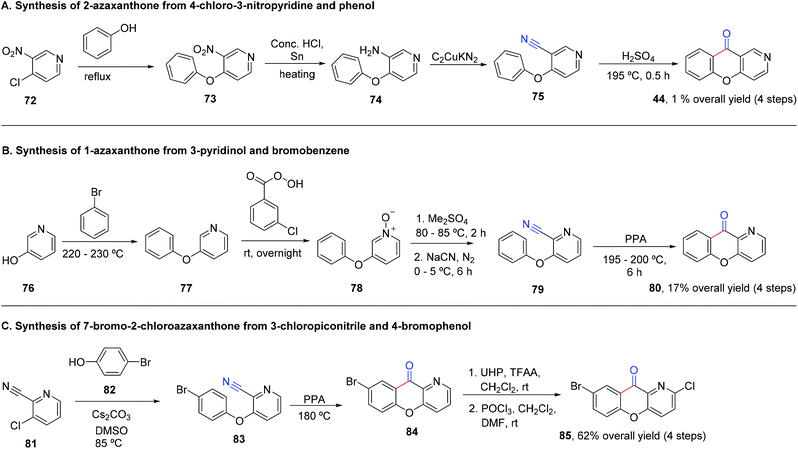 |
| Scheme 13 Synthesis of azaxanthones via cyanopyridyl phenyl ether derivatives (diaryl ether route). | |
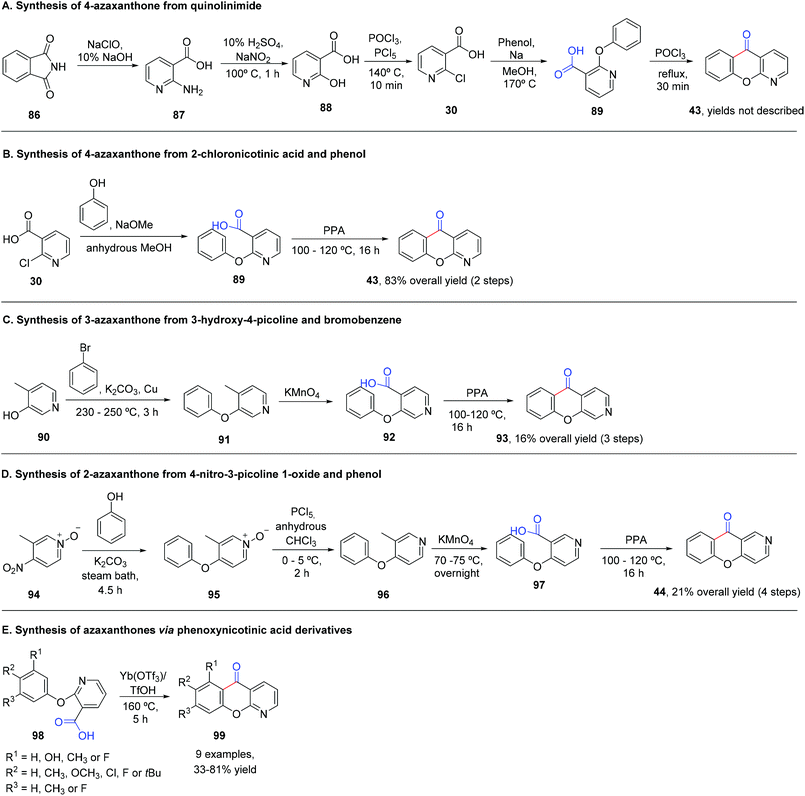 |
| Scheme 14 Synthesis of azaxanthones via phenoxynicotinic acid derivatives (diaryl ether route). | |
4.2.1 Synthesis of azaxanthones via cyanopyridyl phenyl ether.
The synthesis of azaxanthones via cyanopyridyl phenyl ether is a very straightforward methodology, since the cyclization step is easily achieved due to the presence of a pendent cyano moiety. 2-Azaxanthone (44) was synthesized via this methodology by Kruger et al.,169 starting from the condensation of 4-chloro-3-nitropyridine (72) with phenol to yield intermediary 3-nitro-pyridyl phenyl ether (73) (Scheme 13A). Reduction of the nitro group, oxidative conversion of the amine to the corresponding nitrile, and further cyclization with sulfuric acid at 195 °C led to 2-azaxanthone (44), in very low yield (1% over 4 steps), probably due to the formation of sulfonated phenoxy derivatives that were soluble in water. A similar strategy was used for the synthesis of 1-azaxanthone (80)78 (Scheme 13B). 3-Phenoxypyridine (77) was used as the starting material, previously obtained from the reaction between 3-pyridinol (76) and bromobenzene. N-Oxide formation with m-chloroperbenzoic acid, and the subsequent reaction with dimethyl sulfate produced N-methoxymethyl sulfate salts. Due to the fact that the resulting salts are generally hygroscopic and difficult to handle, a reaction with sodium cyanide under a nitrogen atmosphere yielded stable 2-cyano-3-phenoxypyridine (79). 1-Azaxanthone (80) was obtained via direct ring closure in 17% overall yield in four steps from 3-pyridinol (76). A di-halogen-substituted azaxanthone was also synthesized via cyanopyridyl phenyl ether from 3-chloropiconitrile (81) and 4-bromophenol (82) (Scheme 13C). The diaryl ether 83 was obtained through SNAr and further intramolecular Friedel–Crafts reaction in PPA and an in situ hydrolysis yielded the 1-azaxanthone core 84. Nitrogen oxidation and treatment with phosphoryl chloride originated the desired chloride 85 with 62% overall yield, considering 4 reaction steps (Scheme 13).149
4.2.2 Synthesis of azaxanthones via phenoxynicotinic acid derivatives.
4-Azaxanthone (43), also known as 9-oxa-4-aza-anthrone or 5H-[1]benzopyrano[2,3-b]pyridin-5-one, was the first azaxanthone to be synthesized ever, following a methodology involving phenoxynicotinic acid derivatives (Scheme 14A). Preparation of 2-aminonicotinic acid (87) via the Hofmann reaction on quinolinimide 86 previously obtained from 8-hydroxiquinoline and further treatment of 87 with nitrous oxide yielded 2-hydroxynicotinic acid (88). Transformation into 2-chloronicotinic acid (30) and condensation of the corresponding sodium salt with sodium phenoxide yielded 2-phenoxynicotinic acid (89) which after cyclization afforded compound 43.170 Another method for the synthesis of azaxanthones was developed by Villani and collaborators,78 taking phenoxypyridinecarboxylic acids as the starting materials, with the nitrogen in different positions, according to the desired azaxanthone. The reactions employed allowed the synthesis of azaxanthones previously described in better yields. For the synthesis of 4-azaxanthone (43, Scheme 14B), 2-chloronicotinic acid (30) reacted with phenol in the presence of sodium methoxide to yield 2-phenoxynicotinic acid (89). The ring closure was achieved with the use of phenylphosphonic acid (PPA), in 83% overall yield [2 steps from 2-chloronicotinic acid (30). For the synthesis of 3-azaxanthone (93, Scheme 14C), the starting materials used were 3-hydroxy-4-picoline (90) and bromobenzene, which through the Ullmann condensation led to 3-phenoxy-4-picoline (91). 3-Phenoxyisonicotinic acid (92) was obtained by oxidation of 91 and heating of 92 with PPA, similarly to the previous reaction, led to 3-azaxanthone (93) in 16% overall yield, in three steps from 3-hydroxy-4-picoline (90).78 On the other hand, 2-azaxanthone (44) was prepared by using 4-nitro-3-picoline 1-oxide (94) and phenol as the starting materials. Displacement of the nitro group by phenoxide in the presence of K2CO3 gave phenyl ether 95 that was N-deoxygenated to the pyridyl ether 96. Oxidation gave the desired 4-phenoxynicotinic acid (97) which after ring closure gave 2-azaxanthone (44) in 21% overall yield in 4 steps from 4-nitro-3-picoline 1-oxide (94) and phenol (Scheme 14D).78 Worth highlighting is a simple and clean methodology for the synthesis of azaxanthones and azathioxanthones. Li et al.171 developed a synthetic route that did not use expensive materials and had solvent-free conditions, starting from phenoxypyridine acids. In order to obtain the best catalyst, studies with a series of metal triflates were carried out. It was concluded that ytterbium(III) trifluoromethansulfonate was the best catalyst, and that the use of a Brønsted acid would slightly improve the yield in the synthesis of 4-azaxanthone and derivatives (Scheme 14E).
4.2.3 Synthesis of azaxanthones via amide derivatives.
In an effort to find new β-amyloid precursor protein cleaving enzyme 1 (BACE1) inhibitors, new azaxanthones were synthesized.149 Compared to previously synthesized xanthones, the introduction of a nitrogen could improve in vitro potency, cardiovascular safety, pharmacokinetics, and drug metabolism, and modulate central nervous system penetration.172 These features could also be evidenced by a fluorine-substitution at the 3- or 4-position. The combination of these features would ultimately lead to an overall improvement of the compounds as BACE1 inhibitors. Since these molecules had never been synthesized before, different synthetic approaches were attempted. For the synthesis of 3-aza-4-fluoroxanthone 104 (Scheme 15), the first step was the regioselective copper-catalysed Ullmann coupling between 2,5-dibromobenzoic acid (100) and 2-fluoro-hydroxypyridine (101), which afforded a diaryl ether 102. This intermediate 102 was treated with diethylamine and 2-(1H-benzotriazole-1-yl)-1,1,3,3-tetramethylaminium tetrafluoro-borate (TBTU) to give amide 103. An amide-directed lithiation on the pyridine, followed by an in situ cyclization furnished a fluorinated azaxanthone 104 [30% overall yield in 3 reaction steps from 2,5-dibromobenzoic acid (100)]. From the same amide intermediate 103, N-oxidation was performed on the pyridine nitrogen (105) and subsequent treatment with phosphoryl chloride afforded a chloride derivative 106, which produced a tri-halogenated azaxanthone 107 after a tandem lithiation/cyclization (Scheme 15), with an overall yield of 9% in five steps from 2,5-dibromobenzoic acid (100) and 2-fluoro-hydroxypyridine (101) (Scheme 15).149
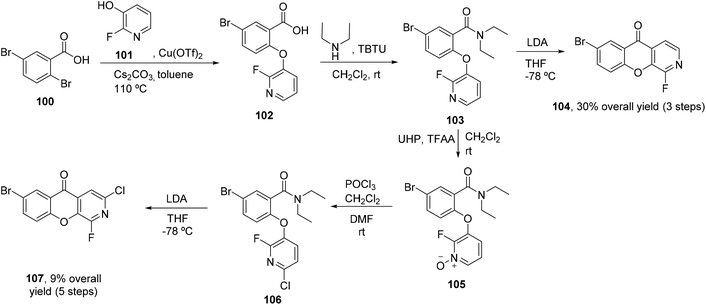 |
| Scheme 15 Synthesis of azaxanthones via amide derivatives (diaryl ether route). | |
5 Synthesis of (aza)xanthones via chromone derivatives
The chromone core constitutes a versatile building block that can be used for the synthesis of xanthone derivatives. To form an additional aromatic ring, several strategies can be applied, mainly the traditional Diels–Alder or other cycloaddition reactions. Depending on the substitution pattern of the chromone derivative, this section will be sub-divided into three subsections: synthesis of xanthones from 2-substituted (section 5.1), 3-substituted (section 5.2) and 2,3-disubstituted chromones (section 5.3) (Scheme 16).
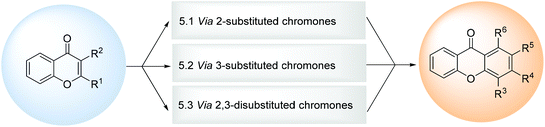 |
| Scheme 16 Synthesis of xanthones via chromones. | |
5.1 Synthesis of xanthones from 2-substituted chromones
5.1.1
Via Diels–Alder reaction.
The high versatility and efficiency of the Diels–Alder reactions have also been extended to the synthesis of xanthones from 2-substituted chromones. Xanthone-1,2,3-triazole dyads were obtained by two different approaches, both starting from (E)-2-(4-arylbut-1-en-3-yn-1-yl)chromones, previously obtained by a base-catalysed aldol reaction of 2-methylchromone and arylpropargyl aldehydes.173 While the first approach adopted a methodology in which the xanthone core is primarily constructed using chromones as dienes in the Diels–Alder reaction, followed by the construction of the triazole ring, in the second strategy the process was inverted, staring with the triazole ring followed by the construction of the xanthone core (Scheme 17A). Thus, in the first methodology, a Diels–Alder reaction of the chromone derivatives 108 with N-methylmaleimide under microwave irradiation gave Diels–Alder adducts 109. Oxidation of the obtained adducts with 2,3-dichloro-5,6-dicyano-1,4-benzoquinone (DDQ) gave xanthone derivatives 110 which upon reacting with sodium azide in refluxing DMF provided the desired xanthone-1,2,3-triazole dyads 111 in excellent yields (88–90%). The second approach consisted of a 1,3-cycloaddition reaction of chromones 108 with sodium azide to form the triazole derivatives (112) followed by methylation of the triazole NH group prior to the Diels–Alder reaction with N-methylmaleimide and oxidation with DDQ to provide the xanthone-1,2,3-triazole dyads (111) in moderate to good yields (57–80%).173 (E)-2′-Propargyloxy-2-styrylchromones (115) were used as substrates in a microwave-assisted intramolecular Diels–Alder reaction, affording chromeno[3,4-b]-xanthones 116 (Scheme 17B).174 During optimization processes, besides the variation of solvent, Lewis acid, temperature, and reaction time, different oxidizing agents were also tested in order to push the reaction towards the oxidized product 116, without the formation of secondary products that are not fully oxidized. Optimal conditions for MAOS of chromeno[3,4-b]xanthones are the use of 1,2,4-trichlorobenzene (1,2,4-TCB) as a solvent, under MW irradiation at 200 °C for 60 min and then the addition of the oxidizing agent chloranil to the reaction crude, under MW irradiation at 80 °C for 30 min.174
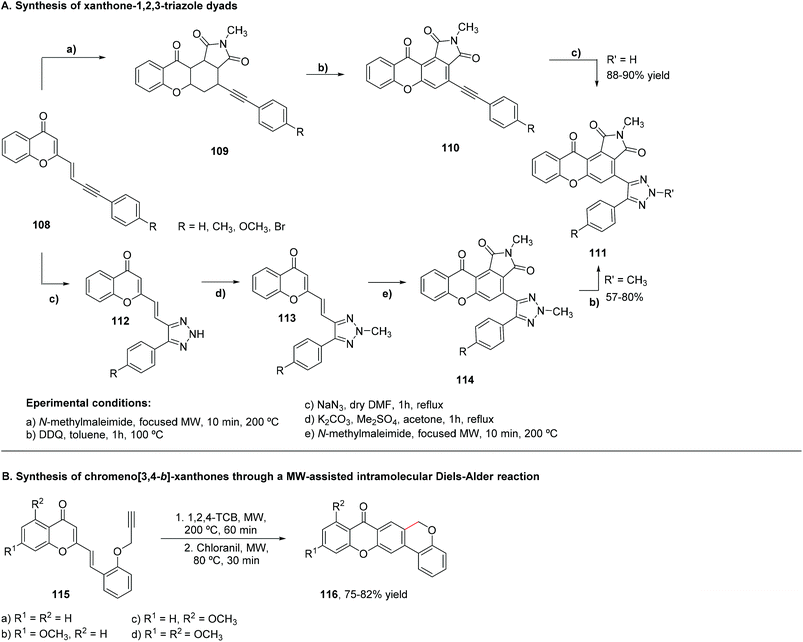 |
| Scheme 17 Synthesis of xanthones from 2-substituted chromones via the Diels–Alder reaction. | |
5.1.2
Via electrocyclization.
Another example of transforming 2-substituted chromones into xanthone derivatives is through an electrocyclization and subsequent oxidation (Scheme 18). The substrates 2-[(1E,3E)-4-arylbuta-1,3-dien-1-yl]-4H-chromone 117 are previously obtained in good yields by condensation of 2-methyl-4H-chromones with cinnamaldehydes in the presence of sodium ethoxide. Thus, the target 1-aryl-9H-xanthen-9-ones 118 are obtained under refluxing 1,2,4-TCB in the presence of a catalytic amount of iodine and subsequent demethylation with BBr3, in moderate to good yields.175 This study also disclosed that some of the tested compounds had improved scavenging activity when compared with previously reported analogues, being promising pharmacophores with potential therapeutic applications associated with oxidative stress disorders.175
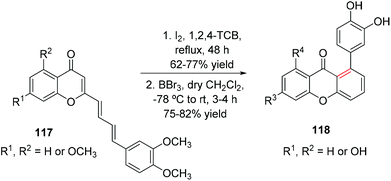 |
| Scheme 18 Synthesis of xanthones through an electrocyclization and subsequent oxidation. | |
5.2 Synthesis of xanthones from 3-substituted chromones
5.2.1
Via a tandem [4 + 1]-[4 + 2] cycloaddition.
Bornadiego et al.176–178 reported the synthesis of 4-aminoxanthones via a tandem [4 + 1]-[4 + 2] cycloaddition of 3-carbonylchromones, isocyanides and dienophiles (Scheme 19A). The authors were able to extend the reaction scope to different dienophiles, either symmetric N-phenyl- and N-methylmaleimide, maleimide, and maleic anhydride,176 or asymmetric ones like acrylonitrile or methyl vinyl ketone.177 Additionally, they were also able to extend this methodology to carbonylchromones containing both electron-withdrawing and electron-donating groups.178 Mechanistically, 4-aminoxanthone is obtained through a [4 + 1] cycloaddition of a 3-carbonylchromone 119 with an isocyanide 120 to give an iminolactone intermediate 121. Tautomerization to aminofuran 122, which undergoes a [4 + 2] cycloaddition with the dienophile 93, leads to 7-oxabicyclo [2.2.1]heptane (124). This is readily transformed to 1-hydroxydihydroxanthone 125 by the opening of the oxygen bridge assisted by the nitrogen lone pair. Dehydration finally affords the aromatic 4-aminoxanthone 126 (Scheme 19A).178
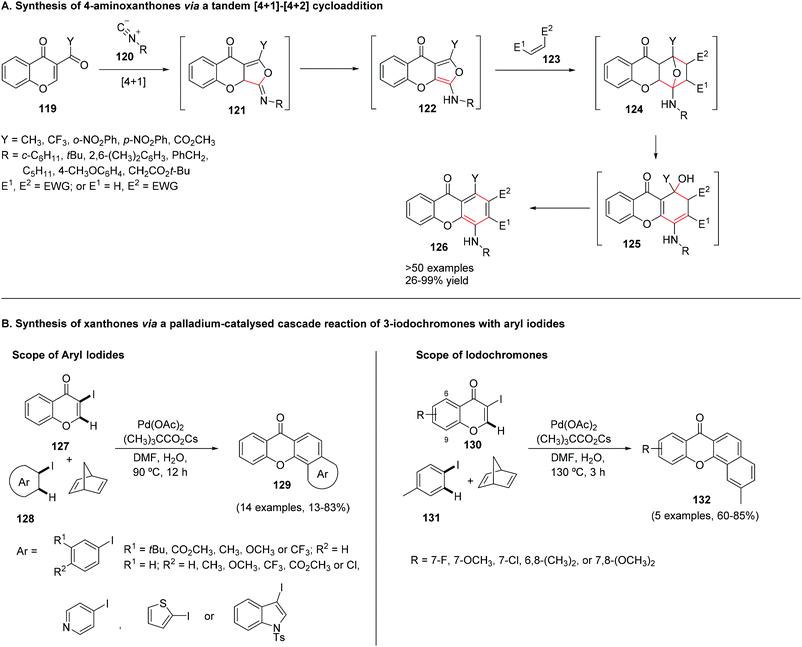 |
| Scheme 19 Synthesis of xanthones from 3-substituted chromones. | |
5.2.2
Via a tandem Heck reaction/double C–H activation/retro Diels–Alder pathway.
Another strategy towards the construction of the xanthone core from 3-substituted chromones is via a tandem Heck reaction/double C–H activation/retro Diels–Alder pathway. The use of readily available 3-iodochromones 127 and 130, aryl iodides 128 and 131, and norbornadiene as the starting materials in this palladium-catalysed cascade reaction enables access to diverse annulated xanthones (129 and 132, Scheme 19B).179 This process is initiated by oxidative addition of Pd(0) to the chromone ring system, followed by norbornadiene insertion and C–H activation of the 2-position of the chromone ring. A second oxidative addition of the aryl iodide, subsequent reductive elimination, and second C–H activation formed the desired xanthones.179
5.2.3 Synthesis of azaxanthones by reaction of carbonitrile chromones and oximes.
To better understand the condensation reactions of chromone-3-carbonitriles, Ghosh et al.180 have performed reactions that yielded, among other compounds, azaxanthones. The starting carbonitrile chromone 133 reacted with acetylacetone (134), in the presence of piperidine, furnishing a 4-azaxanthone derivative 135 in 80% yield. The mechanism through which this reaction occurs was investigated, and it was concluded that 134 undergoes Michael addition to the α,β-unsaturated ketone. Concomitantly, the pyrone ring opens, yielding an intermediate which undergoes, probably in a concerted manner, a double cyclisation (Scheme 20A). The same study also contemplated the synthesis of the same compound (135), taking an oxime (136) as the starting material (Scheme 20A). Given that chromone-3-nitriles are prepared from oximes, their use for preparing azaxanthones shortens the synthetic route, although the yield, 67%, was not as good as when the nitrile was used as the starting material.180
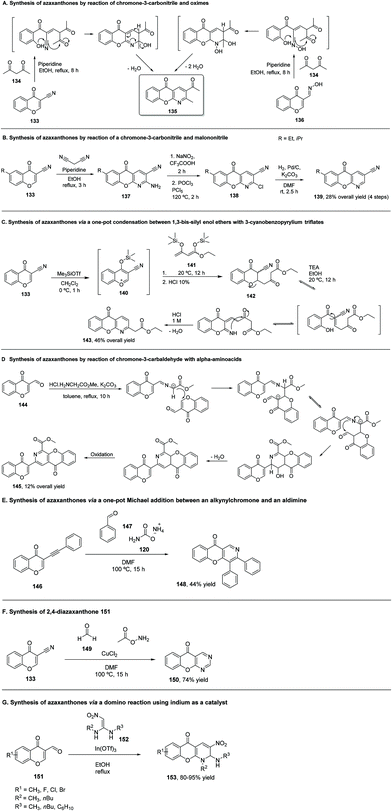 |
| Scheme 20 Synthesis of azaxanthones from 3-substituted chromones. | |
5.2.4 Synthesis of azaxanthones by reaction of a cyano chromone and malononitrile.
A reaction of cyano chromone 133 with malononitrile led to 137 which was then deaminated by sodium nitrite in trifluoroacetic acid. Further chlorination using phosphoryl chloride and phosphorus pentachloride afforded 138 in 38% yield, which was converted to compound 139 by catalytic hydrogenation (Scheme 20B), in 28% overall yield from cyano chromone 133 in four reaction steps.181
5.2.5 Synthesis of azaxanthones via a one-pot condensation between 1,3-bis-silyl enol ethers with 3-cyanobenzopyrylium triflates.
Azaxanthone derivatives could also be attained by domino reactions in a one-pot process. The approach used by Langer and Appel182 consisted of the condensation of 1,3-bis-silyl enol ethers (141), unsymmetrical acetoacetone, with 3-cyanobenzopyrylium triflates (140). This yielded an open-chained product (142), which was treated with triethylamine, to give an azaxanthone (143) in 46% overall yield, through a domino “retro-Michael-lactonization-aldol” reaction (Scheme 20C).
5.2.6 Synthesis of azaxanthones by reaction of chromone-3-carbaldehyde with α-amino acids.
In an attempt to synthesize (hydroxybenzoyl)pyrroles, Figueiredo et al.183 have discovered an azaxanthone, from a chromone moiety. The reaction started with a chromone aldehyde 144 and ethyl glycinate hydrochloride, and depending on the reaction conditions, several compounds were formed. For the azaxanthone derivative 145 to be synthesized, in 12% overall yield, one equivalent of methyl glycinate and 0.5 equivalent of potassium carbonate were required, in refluxing toluene. The authors proposed a mechanism for the formation of the azaxanthone intermediate, which comes from the oxidation of the dihydroxanthenone (Scheme 20D).
5.2.7 Synthesis of azaxanthones via a one-pot Michael addition between an alkynylchromone and an aldimine.
One-pot approaches were also an efficient method of obtaining azaxanthones. In this scope, the starting material chosen was 2-(1-alkynyl)-2-alken-1-ones (Scheme 20E), which are interesting for multicomponent reactions, as they are prone to transition metal, Lewis acid, and electrophile-induced cascade cyclization reactions promoted by nucleophiles. Since these starting compounds have previously been reported to be involved in reactions initiated by Michael addition of nitrogen, it was postulated that N-unsubstituted aryl aldimines would act as nucleophiles in Michael addition reactions with 3-(1-alkynyl)chromones. Subsequently, a cascade sequence would be initiated, ultimately leading to the production of azaxanthones.14 The optimal conditions for this reaction were also investigated. DMF was found to be the best solvent, against ethanol, DMSO, toluene, and dioxane. As for the base, it was shown that three equivalents of ammonium acetate provided the optimal conditions, but the reaction also occurred with ammonium carbamate, ammonium bicarbonate, and ammonium formate. In the opposite way, ammonium chloride and ammonium sulfate did not yield the desirable product.14 The same study also described the synthesis of 2,4-diazaxanthones (150) through the same methodology (Scheme 20F). In an effort to extend the cascade reaction to other Michael acceptors, 3-cyanochromone (133) was chosen as the starting material. After testing different reaction conditions, it was demonstrated that the use of copper(II) chloride as an oxidant would lead to yields up to 85%. For the production of the unsubstituted 2,4-diazaxanthone (150), the aldehyde employed was paraformaldehyde (149), and the yield was 74% (Scheme 20F).14
5.2.8 Synthesis of azaxanthones via a domino reaction using indium as a catalyst.
A green approach for the formation of azaxanthones was proposed by Poomathi et al.184 This group managed to synthesize azaxanthones through a domino reaction using a small amount of catalyst and ethanol as the solvent. Studies on the catalyst showed that indium triflate was the best choice for a Lewis acid for this reaction, and that it could be recovered after the reaction and maintain its catalytic efficiency for, at least, five reactions.
In order to obtain azaxanthones (153), 3-formylchromones (151) reacted with N,N′-dibutyl-2-nitroethene-1,1-diamines (152), using In(OTf)3 in ethanol, in reflux, as can be seen in Scheme 20G. This reaction allowed the synthesis of a series of derivatives, whose yields ranged from 80% to 95%.184 The reaction mechanism hypothesized involves a Henry reaction between 3-formylchromone (151) and N,N-dibutyl-2-nitroethene-1,1-diamine (152) (Scheme 20G). Elimination of water and further 6π-electrocyclization yield azaxanthones 153.184
5.3 Synthesis of xanthones from 2,3-disubstituted chromones
5.3.1
Via photo-induced tandem cyclization of 3-iodoflavones with five membered heteroarenes.
An excellent example that provides rapid access to polycyclic xanthones without the requirement of any transition-metal-catalyst and/or oxidant additives is a tandem cyclization via the coupling of 3-iodoflavones 154 with five-membered heteroarenes.185 This procedure allowed the preparation of a broad variety of novel polycyclic xanthone frameworks with excellent regioselectivity in good yields under mild and environmentally friendly reaction conditions. Mechanistic studies unveiled that the reaction proceeds via two consecutive C–C bond formations after irradiation of the substrate and N-methylpyrrole in CH3CN using a high-pressure mercury lamp (500 W) at room temperature for 7 h (Scheme 20A). It was possible to observe that the obtained yields were lower when in the presence of electron-donating groups on both R1 and R2 and higher when in the presence of only one electron-donating group at either R1 or R2. The substrate scope was also extended to other five-membered heteroarenes, with the desired xanthone derivatives 155 being obtained in lower yields.185
5.3.2
Via intramolecular [4 + 2] cyclization.
Another procedure that allows the preparation of xanthone derivatives without the need for a transition metal catalyst consists of a phase transfer reagent promoted tandem ring-opening and ring-closing reaction of 3-(hepta-1,6-diyn-1-yl)chromone derivatives 156 followed by a intramolecular [4 + 2] cyclization.186 The use of t-BuOK, n-Bu4NCl and phenylacetonitrile as an anion transfer reagent in DMSO at 110 °C for 20 min under MW irradiation proceeded smoothly for the preparation of several xanthone derivatives 157 (Scheme 21B), with the exception of R2 = H, and R1 = Ph, the latter probably due to the steric effects of two phenyl groups at the ortho position.186
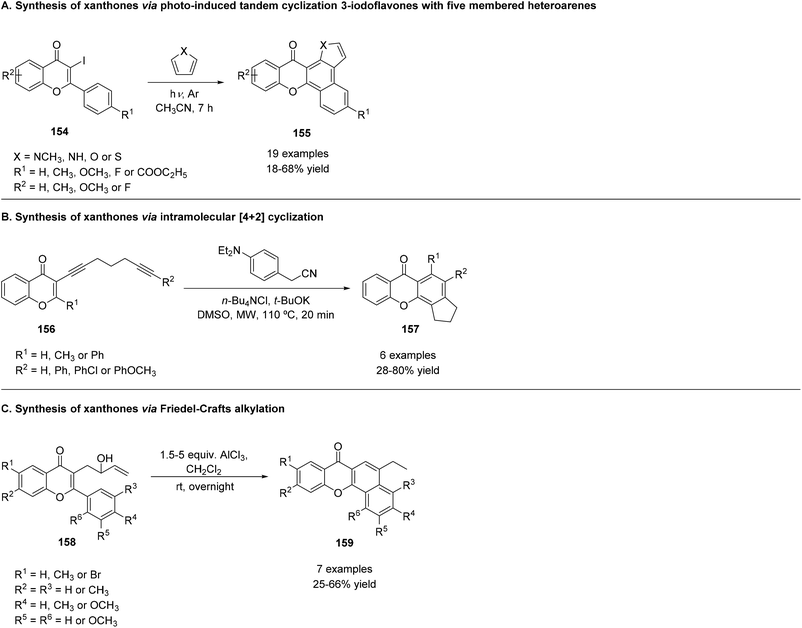 |
| Scheme 21 Synthesis of xanthones from 2,3-disubstituted chromones. | |
5.3.3
Via Friedel–Crafts alkylation.
An intramolecular Friedel–Crafts alkylation strategy using AlCl3 in dichloromethane was used to smoothly synthesize the corresponding benzoxanthones 159 in low to moderate yields (Scheme 21C), with the more electron rich aromatic systems in the 2-position of the chromone 158 being obtained with increased efficiency.187
5.3.4 Synthesis of azaxanthones by reaction of chromone-3-carbonitriles and aminoacrylate or ethyl propiolate.
While performing studies on potential anti-anaphylactic agents, Nohara et al. applied a series of different methods to synthesize azaxanthone derivatives, inspired by xanthone-2-carboxylic acids bearing a tetrazole group, which have proven to show oral antiallergic activity.181,188 The authors converted chromone-3-carbonitriles 133 into 2-aminochromone-3-carbaldehydes 160 which were found to be good starting materials for the synthesis of some types of heterocycles. After the synthesis of these intermediates, some different synthetic methodologies were applied to produce different azaxanthones (Scheme 22).
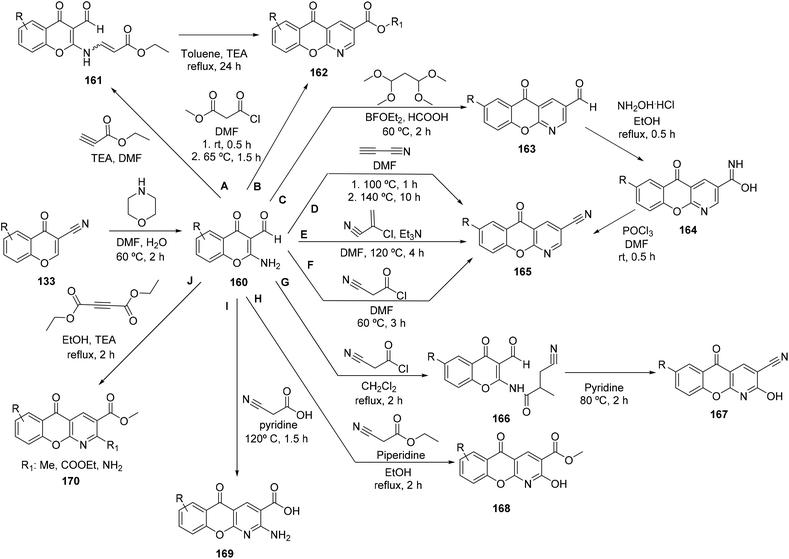 |
| Scheme 22 Synthesis of azaxanthones starting from 2-aminochromone-3-carbaldehyde 160. | |
The first method used was the reaction of 160 with ethyl propiolate with triethylamine (TEA) in DMF, yielding an aminoacrylate 161 (Scheme 22A), which was then converted by further heating to the desired azaxanthone 162, in 19% yield. A similar product could be obtained by a one-step reaction, using methyl malonyl chloride (Scheme 22B), in 36% yield.181 A different approach was the reaction of 160 with cyanoacetylene, yielding 3-cyano derivatives (165), in 36% yield (Scheme 22D). In this reaction, no basic catalyst was needed. The search for an alternative to this methodology, since cyanoacetylene is unstable and sublimates at low temperatures, among other undesirable properties, led to the synthesis of the same type of compound 165 in 33% yield, using α-chloroacrylonitrile with triethylamine (Scheme 22E). A second alternative to prepare 165 is the treatment of 160 with DMF and cyanoacetyl chloride, which produced the desired compound in 49% yield (Scheme 22F).181 The change of DMF for dichloromethane, in the reaction with cyanoacetyl chloride, produced an amide intermediate (166) (Scheme 22G), which could be converted into an azaxanthone 167 by heating with pyridine.
In this case, the azaxanthone obtained was 2-hydroxy substituted (167), in 22% yield.181 The same group used another synthetic strategy towards the synthesis of azaxanthones, which was the condensation of 160 with malonaldehyde. As such, a derivative of 160 was chosen to react with malonaldehyde bis(dimethyl acetal), in the presence of formic acid in boron trifluoride etherate, affording compound 163 in 61% yield (Scheme 22C). Afterwards, an oxime (164) was prepared through the reaction of 163 with hydroxylamine, with 95% yield. Finally, a nitrile (166) was obtained by the reaction with phosphoryl chloride in DMF (Scheme 22), yielding 36% of the desired compound.181 The same group also performed reactions where the final product would be a 2-substituted azaxanthone. The methods used ethyl cyanoacetate and piperidine (Scheme 22H, 95% yield), cyanoacetic acid and pyridine (Scheme 22I, 55%) and diethyl acetylenedicarboxylate (Scheme 22J, 91%), yielding azaxanthone derivatives 168, 169 and 170, respectively.181
5.3.5 Synthesis of azaxanthones via a modified Friedländer reaction.
The synthesis of azaxanthones can also be achieved through modification of the Friedländer reaction. Using o-aminoaromatic aldehydes and unmodified ketones, Dormer et al. (2003) proceeded to synthesize 2-alkylsubstituted products, in a regioselective manner. The main objective was to evaluate the efficiency of several catalysts in terms of regioselectivity, conversion ratio and reaction conditions, and it was concluded that 1,3,3-trimethyl-6-azabicyclo[3.2.1]octane (TABO) was the most effective. Slow addition of the ketone had a positive impact on regioselectivity, and reaction temperature also played a key role.189 For the preparation of an azaxanthone using this method, 2-amino-3-formylchromone (160) and 2-hexanone (171) were chosen as the starting materials. The chromone was slowly added to a solution of the ketone, sulfuric acid, and the catalyst in ethanol. Out of all the reactions this group performed, this was the one with the lowest regioselectivity [80% 3-substituted (172) (79% overall): 20% 2,3,-disubstituted (173) (20% overall)] (Scheme 23A). Curiously, this reaction did not occur under the conventional Friedländer conditions.189
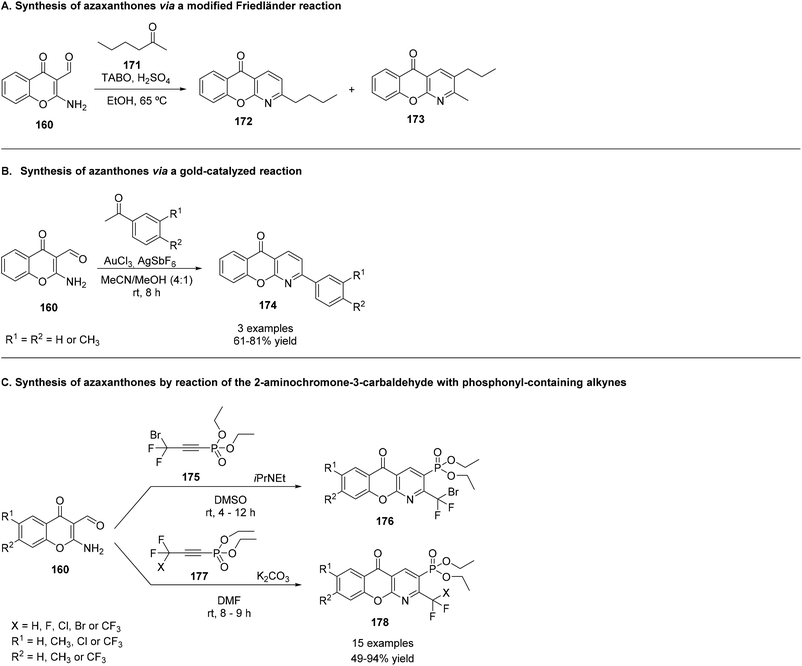 |
| Scheme 23 Synthesis of azaxanthones from 2,3-disubstituted chromones. | |
5.3.6 Synthesis of azanthones via a gold-catalysed reaction.
A collection of N-heterocycles, including azaxanthones, was synthesized through gold catalysed synthesis. The condensation of 2-amino-3-formylchromone 160 and acetophenone derivatives, using gold(III) chloride and silver hexafluoroantimonate(V), and a mixture of acetonitrile and methanol as solvents, gave aryl substituted azaxanthones 174 with good yield (Scheme 23B).190
5.3.7 Synthesis of azaxanthones by reaction of the chromone-carbaldehyde with phosphonyl-containing alkynes.
The synthesis of the azaxanthone scaffold was also described by a different route in an attempt to prepare 2-difluoromethyl-azaxanth-3-ylphosphonates. Duda et al.191 showed a synthetic method that led to these compounds through 2-amino-3-formylchromone (160) which reacted with unsymmetrical substituted electron-poor halogen-CF2 and phosphonyl-containing alkynes 175 and 177. The reaction occurred with DMSO as a solvent and a basic catalyst, at room temperature. It was proven that the best conditions for the synthesis of fluorine, chlorine, difluoromethyl and trifluoromethyl derivatives 176 was DMSO as the solvent and iPrNEt as the catalyst, while for derivatives 178, the best basic catalyst was potassium carbonate and DMF was the best solvent (Scheme 23C). In the formulated hypothesis of the reaction mechanism, the base-activated 2-aminochromone-3-carbaldehydes reacts in a regioselective manner through a Michael-type addition with the carbon containing the halogen-CF2, which is the most electrophilic. Formation of a dipolar allene, and subsequent intramolecular cyclization into the dihydropyridine derivative, leads, after dehydration, to azaxanthone. The polar solvent has utmost importance in this reaction, serving as a stabilizer and possibly interacting with the allene intermediate. An interpretation of the reaction mechanism is depicted in Scheme 23C.191
6 Other methodologies
Other important and conceptually different approaches, which do not belong to any of the categories described above, were also reported, manly focusing in tandem and/or rearrangement reactions. Although some of them start from readily available substrates,192 other require complex starting materials that usually need to be prepared prior to the reaction,193,194 but also capable of reaching unusual substituents/patterns of substitution in the xanthonic core.
6.1 Synthesis of xanthones via decarbonylative coupling
Salicylaldehydes can also be used as substrates in rhodium-catalysed reductive decarbonylative couplings to give symmetrically substituted xanthones 180 (Scheme 24A), containing formyl, bromo, chloro, fluoro, methyl, acetyl, nitro, hydroxyl, and trimethoxy groups (10 examples).141 Interestingly, this reaction revealed to be site-selective since when 4-hydroxyisophthalaldehyde was used as a substrate, the corresponding 9-oxo-9H-xanthene-2,7-dicarbaldehyde was obtained, indicating the preferential reaction of the formyl group ortho to hydroxyl during the decarbonylative homo-coupling process.141
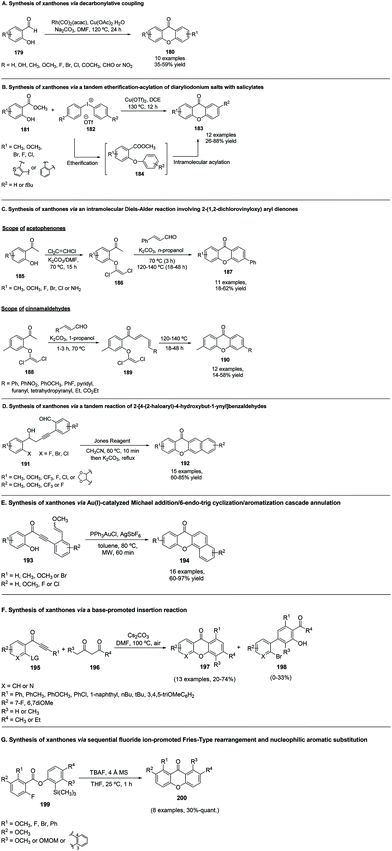 |
| Scheme 24 Other methodologies for the synthesis of xanthones and azaxanthones. | |
6.2 Synthesis of xanthones via a tandem etherification–acylation of diaryliodonium salts with salicylates
Salicylates can also be employed for the synthesis of substituted xanthones 183, when combined with diaryliodonium salts.195 An intermolecular etherification–acylation in the presence of Cu(OTf)2 as a catalyst in dichloroethane (DCE) produced the desired xanthones in good yields (Scheme 24B). Mechanistically, the reactions are initiated by the etherification of diaryliodonium salts with salicylates, followed by an intramolecular acylation.
6.3 Synthesis of xanthones via an intramolecular Diels–Alder reaction involving 2-(1,2-dichlorovinyloxy) aryl dienones
An intramolecular Diels–Alder reaction where both of the pyrone and the second aromatic rings are forged in a single step was reported for the synthesis of xanthones.192 The scope of the route to several substituted xanthones was tested by using different 2′-hydroxyacetophenones (185) to prepare the corresponding 1,2-dichlorovinyloxy acetophenones (186 and 188) that react in situ with cinnamaldehyde (Scheme 24C). Similarly, the scope of the reaction was also examined using a variety of commercially available α,β-unsaturated aldehydes (Scheme 24C) with the expected xanthones 187 and 190 being obtained in modest yields (23 examples, 18–62%).192
6.4 Synthesis of xanthones via a tandem reaction of 2-[4-(2-haloaryl)-4-hydroxybut-1-ynyl]benzaldehydes
12H-Benzo[b]xanthen-12-ones 192 were synthesized using Jones reagent and K2CO3 promoted tandem reaction of 2-(4-(2-haloaryl)-4-hydroxubut-1-ynyl)benzaldehydes 191 (Scheme 24D).193 The scope and limitation of this reaction were studied and it was found that in addition to a 2-bromo substituted substrate, 2-fluoro or 2-chloro derivatives could also be obtained. Due to fluoride's great inductive effect, which accelerates the rate-determining step of the nucleophilic aromatic substitution step, it was observed that fluoro substituted substrates reacted faster than bromo or chloro. However, a bromo substituted substrate was more reactive than 2-chloro since, as is well known, Br is a better leaving group than Cl. It was proposed that the overall transformation presumably proceeds through an initial tandem reaction of 191 with the formation of a 3-acyl-2-naphtol key intermediate, which should be simultaneously transformed into the corresponding phenoxide in the presence of K2CO3. Further intramolecular O-arylation occurs to give the expected 12H-benzo[b]xanthen-12-ones 192.
6.5 Synthesis of xanthones via Au(I)-catalysed Michael addition/6-endo-trig cyclization/aromatization cascade annulation
Another strategy used for the synthesis of 7H-benzo[c]xanthen-7-ones 194 is through a Michael addition/6-endo cascade cyclization/aromatization, extensible to the synthesis of benzoacridones (Scheme 24E).194 While the presence of electron-withdrawing groups on the methoxyvinyl phenyl ring led to production of the target xanthones in higher yields, when they were present in the phenol ring poor yields were obtained, regardless of the substitutions on the methoxyvinyl phenyl ring.194 The observed outcome of the reaction could be explained by the formation of a complex with the gold catalyst, activating the 1,3-diphenylprop-2-yn-1-one which is then attacked by the hydroxyl group in a 6-endo-dig manner. Photodeauration of the formed intermediate and further attack of the newly formed enol ether to the electron-rich methoxyl vinyl ether gave the final xanthone after isomerization and aromatization through the release of methanol.
6.6 Synthesis of xanthones via a base-promoted insertion reaction
In a completely different approach, xanthones and azaxanthones were obtained through a base-promoted insertion and transition-metal-free reaction of isolated internal alkynes with β-diketone compounds (Scheme 24F).196 Studies on the scope of the reaction revealed that different leaving groups on the acetylenic ketone 195 were well tolerated, although 1-(2-bromophenyl)-3-phenylprop-2-yn-1-one (LG = Br) produced the target xanthone 197 in the highest yield and greatest chemoselectivity. The corresponding acetyl aroyl derivative 198 was obtained as a secondary product (10% yield) of the reaction, which increased when an electron-withdrawing substituent (R2 = 7-F) was employed.
6.7 Synthesis of xanthones via sequential fluoride ion-promoted Fries-type rearrangement and nucleophilic aromatic substitution
A mild and efficient reaction promoted by fluoride ions was used to prepare 1,8-disubstituted xanthones 200 from phenyl benzoate derivatives 199 (Scheme 24G).197 The reaction proceeded via a Fries-type rearrangement to generate a benzophenone intermediate that immediately cyclizes into the expected xanythones due to the presence of phenoxide and fluorine moieties at the C-2 and C-2′ positions. This methodology is particularly useful for the synthesis of xanthones with steric hindrance around C-9, which are difficult to obtain through other methodologies.
6.8 Synthesis of azaxanthone-derivatives: the case of five-membered ring azaxanthones
Different types of azaxanthones have been synthesized. Noteworthy for their biological activities are the five-membered ring azaxanthones. The incorporation of a pyrazole and a triazole moiety in the xanthone core can be achieved by two different methods. In the first method (Scheme 25A), an intramolecular SNAr would be responsible for the construction of the central ring; in the second method (Scheme 25B), an anionic intramolecular ring closure takes place. Concerning the first method, 1-(benzyloxy)pyrazole (201) and 1-benzyloxy-1,2,3-triazole (202) were metalated, using butyllithium in THF, at −78 °C. The second step was slightly different for the two lithiated compounds: for the pyrazole derivative, transmetalation with zinc(II) iodide or chloride was performed, followed by cross-coupling 2-fluorobenzoyl chloride and tetrakis(triphenylphosphine)palladium(0) in THF at 20 °C. The next step was debenzylation, using hydrochloric acid.198 On the other hand, for the triazole derivative, the transmetalation step occurred with tributylstannane, and the debenzylation with H2/Pd.199 The ring closure proceeded under mild conditions, using potassium carbonate in DMF, at 50 °C.200 The overall yield was 79% for the benzopyranopyrazole (203) and 70% for the benzopyranotriazole (204). Scheme 25A shows the reactions used to obtain this kind of azaxanthone.
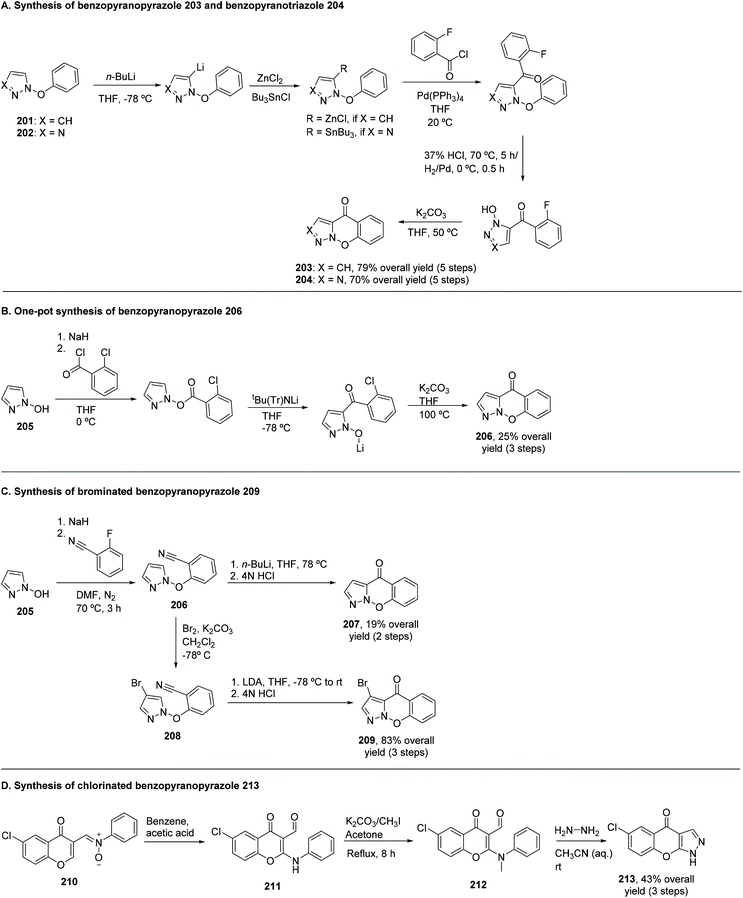 |
| Scheme 25 Other methodologies for the synthesis of xanthones. | |
A one-pot approach was also achieved, which represented a faster way of obtaining a pyrazol-containing azaxanthone, albeit with a more modest yield of 25% (Scheme 25B). In this reaction, 1-hydroxypyroxazol (205) was used as the starting material, and was dissolved in THF, at 0 °C, under a nitrogen atmosphere, and sodium hydride was added. Afterwards, 2-chlorobenzoyl chloride was added and, after the reaction vessel was cooled to −78 °C, lithium t-butyltritylamide was added. Then, the mixture was allowed to heat to room temperature and potassium carbonate was added, followed by heating to 100 °C for 10 hours, yielding the desired azaxanthone (206) (Scheme 25B).200
The second route was aimed to construct the O-aryl bond before closing the ring. As such, efforts were placed towards the synthesis of a cyano diaryl-ether (206), since the cyano group constitutes an activating group in nucleophilic aromatic substitution (Scheme 25C). 1-Hydroxypyroxazol (205) reacted with 2-fluorobenzonitrile, in the presence of sodium hydride, in DMF, under a nitrogen atmosphere, for three hours, at 70 °C. n-Buthyllithium was used for the lithiation, in THF, at −78 °C. Addition of hydrochloric acid led to the desired azaxanthone 207, with a low yield of approximately 20%. Studies demonstrated that the 4-bromo derivative of the cyano precursor (208) would give the desired azaxanthone 209 in a more satisfactory 83% overall yield (3 steps), only changing the lithiation reagent to LDA, in order to avoid halogen-metal exchange (Scheme 25C).200
Another method towards obtaining five membered ring azaxanthones was proposed by Singh et al.201 Starting from a nitrone 210, 2-anilino-6-chloro-3-formylchromone (211) was formed in dry benzene, with a few drops of acetic acid (Scheme 25D). A reaction with methyl-iodide in the presence of potassium carbonate in acetone yielded 6-chloro-2-(N-methylanilino)-3-formylchromone (212), which upon reaction with hydrazine hydrate in aqueous acetonitrile overnight afforded the azaxanthone 213 (Scheme 25D) in 43% overall yield.
Besides the synthesis of bioactive azaxanthones, the synthesis of thioazaxanthones and their use as photosensitizers15,202 have been reported as well as the synthesis of more complex pyranoazaxanthones, fused ring azaxanthones203–205 and others.89,206–218
7 Diversification on the xanthone core
Hydroxyl groups are undoubtedly one of the most versatile functional groups when it comes to structural modifications (Scheme 26). A myriad of examples have been described in the literature, including the reaction with alkyl halides for the transformation into alkoxy,43,59,69,219–223 haloalkoxy,18,51,69,99,224 methyl alkyl- or aryl-piperazine moieties,225 epoxypropanoxy65,103,220–222 oxirane221, oxypropanolamines,103 benzenesulfonamides,226 and propargyloxy.45,52,54,63,106,118,173,227,228 When reacted with other compounds that are not alkyl halides, several other functionalities can be achieved like alkoxy,19,23,24,33,97 which in certain situations can be converted into chlorosulfonyl24 or amine,33 ethylenedioxy,100 and allyl.39,115,138 Other transformations include the introduction of glycosyl,55,110,132,135,229,230 ester,17,62,227 amino,151 and even prenyl23,39,40,45,46,49,50,52,53,97,98,111–114,117,130,133,221,231–233 moieties, the latter being further used for the synthesis of caged xanthones39,49,105,138,234 or pyranoxanthones.40,45,46 Ultimately, hydroxyl groups can be converted into triflates which provide easy access towards amines,227,235 imines,116,140,235 aryl,119,236 and alkyl xanthones,155 or even converted into tosyl groups for further transformations110 for the introduction of amines. Aminoxanthones can be obtained through reduction of the nitro group.54,60,108,227,237,238 Further transformation of the amino moiety can be used for the synthesis of oxazol,60 amide,108,237–239 and propargyl54 xanthone derivatives or even transformed into hydroxyl, chloro, iodo or arylxanthones via diazonium salts.219 Carboxyxanthone derivatives can be coupled with amines to obtain the corresponding amides47,151 or esters.240 Although the methyl group is usually not considered a highly versatile group, after a simple bromination66,71,219,241 it is possible to obtain several other functionalities. For example, imidazolylmethyl,219 methylhydroxy,66 methylamine241 or formyl,66,71 the latter being further transformed into methylamine,33,71 acid,240 ester240 and aldoxime240 moieties. The presence of a halogen substituent at the xanthone core also provides an opportunity for further transformations. Recent examples include iodo137 or bromo197 to aryl via arylboronic acids, or bromo to alkynyl xanthones via Sonogashira coupling.197 On the other hand fluoro can undergo SNAr reactions with pyrrolidine and sodium ethoxide197 or installation of the isoprenyl moiety via isoprenyl Grignard reagent.231
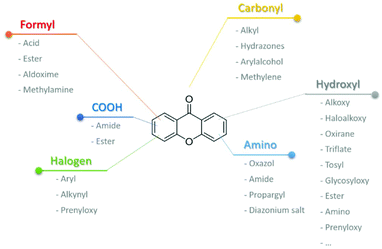 |
| Scheme 26 Most common molecular diversifications on substituted xanthones. | |
8 Conclusions
The importance of xanthones and azaxanthones in medicinal chemistry led to the employment of advanced synthetic methodologies in these heterocyclic scaffolds. For obtaining xanthones and azaxanthones three starting points are generally used: the benzophenone, the diaryl ether or the chromone.
The benzophenone route consists of a multi-step approach using a benzoic or nicotinic acid derivative, followed by a cyclization, finally leading to the xanthone scaffold. The diaryl ether route focuses on a classic Ullmann condensation between an aryl halide and a phenol derivative. The cyclisation can be achieved by a wide range of methodologies, being also a multi-step approach. On the other hand, the chromone route is usually a one-step strategy. These reactions are not exclusive to the (aza)xanthone scaffold, being transversal to the synthesis of many heterocycles, such as flavones, coumarins, acridines, among others which expand the scope of the reaction conditions presented herein.
It can also be observed that many approaches are being revisited and recent advances made with the aim of developing greener methods, such as one-pot reactions, MAOS, solvent- and metal-free strategies. Nanocatalysts represent a significant advancement in catalytic activity over conventional catalytic methods in several synthetic approaches with a diversity of catalysts being employed, such as palladium and magnetically separable nano-CuFe2O4 allowing also high throughputs in purification procedures.
New reagents for transition-metal systems are emerging and include pyridylmagnesium reagents and N-heterocyclic carbenes with unique reactivities that have been used in a broad range of organometallic transformations and are expected to increase in the following years. Also, metal-free conditions are being applied such as metal-free variants of the intramolecular Friedel–Crafts acylation, with quaternary ammonium salts like tetrabutylammonium bromide (TBAB)-promoted intramolecular annulation in aqueous medium standing out. Concerning atom economy, one-pot approaches rule with multicomponent and domino/tandem reactions being often applied in the cyclization reactions of (aza)xanthones. Solvent-free conditions and more efficient catalysts are being disclosed such as indium triflate, ytterbium(III) trifluoromethansulfonate, Cu(0)/Selectfluor system, dichloro(p-cymene)ruthenium(II), and also in palladium catalysed carbonylations/C–H activation reactions. Recent trends are the radical cyclization via photoredox catalysis using photosensitizers and the cross-dehydrogenative reaction that involves a few steps and can be extended to a wide scope of derivatives.
This review also shows that the xanthone scaffold still gathers a lot of attention not only from a synthetic point of view but due to the diversity of bioactive derivatives being disclosed, the case-studies of this study. New biological applications are being proposed for xanthones beyond their pharmacological properties with relevance for chemical biology as sensitizing chromophores. Therefore, it is useful to know the vast array of approaches being used, as they can become a valuable toolbox in the search of novel bioactive heterocycles, emphasizing the importance of this privileged scaffold. Moreover, the site-selective late-stage functionalization approaches highlighted herein can guide medicinal chemists in the lead optimization not only of (aza)xanthones, but also of isosters like thioxanthones, acridones, cromones, flavones, among others. This review can help guide medicinal chemists working with heterocycles by providing chemical tools and reaction conditions to both target and diversity-oriented synthetic approaches.
Conflicts of interest
There are no conflicts to declare.
Acknowledgements
This research was supported by national funds through FCT – Foundation for Science and Technology within the scope of UIDB/04423/2020, UIDP/04423/2020, and under the project PTDC/SAU-PUB/28736/2017 (reference POCI-01-0145-FEDER-028736), co-financed by COMPETE 2020, Portugal 2020 and the European Union through the ERDF and by FCT through national funds, as well as CHIRALBIOACTIVE-PI-3RL-IINFACTS-2019. F. D. and M. M. also acknowledges FCT for their PhD grants (SFRH/BD/114681/2019 and SFRH/BD/146211/2019, respectively).
References
- G. Wu, T. Zhao, D. Kang, J. Zhang, Y. Song, V. Namasivayam, J. Kongsted, C. Pannecouque, E. De Clercq, V. Poongavanam, X. Liu and P. Zhan, Overview of Recent Strategic Advances in Medicinal Chemistry, J. Med. Chem., 2019, 62, 9375–9414 CrossRef CAS PubMed.
- J. J. Vanden Eynde, A. A. Mangoni, J. Rautio, J. Leprince, Y.-T. Azuma, A. T. García-Sosa, C. Hulme, J. Jampilek, R. Karaman, W. Li, P. A. C. Gomes, D. Hadjipavlou-Litina, R. Capasso, A. Geronikaki, L. Cerchia, J.-M. Sabatier, R. Ragno, T. Tuccinardi, A. Trabocchi, J.-Y. Winum, F. J. Luque, K. Prokai-Tatrai, M. Spetea, M. Gütschow, I. Kosalec, C. Guillou, M. H. Vasconcelos, G. Kokotos, G. Rastelli, M. E. de Sousa, C. Manera, S. Gemma, S. Mangani, C. Siciliano, S. Galdiero, H. Liu, P. J. H. Scott, C. de los Ríos, L. A. Agrofoglio, S. Collina, R. C. Guedes and D. Muñoz-Torrero, Breakthroughs in Medicinal Chemistry: New Targets and Mechanisms, New Drugs, New Hopes-6, Molecules, 2019, 25, 119 CrossRef PubMed.
- A. P. Taylor, R. P. Robinson, Y. M. Fobian, D. C. Blakemore, L. H. Jones and O. Fadeyi, Modern advances in heterocyclic chemistry in drug discovery, Org. Biomol. Chem., 2016, 14, 6611–6637 RSC.
- J. Jampilek, Heterocycles in Medicinal Chemistry, Molecules, 2019, 24, 3839 CrossRef CAS PubMed.
- Z. Feng, X. Lu, L. Gan, Q. Zhang and L. Lin, Xanthones, A Promising Anti-Inflammatory Scaffold: Structure, Activity, and Drug Likeness Analysis, Molecules, 2020, 25, 598 CrossRef CAS PubMed.
- Z. Salman, J. Yu-Qing, L. Bin, P. Cai-Yun, C. M. Iqbal, R. Atta-ur and W. Wei, Antioxidant Nature Adds Further Therapeutic Value: An Updated Review on Natural Xanthones and Their Glycosides, Digital Chin. Med., 2019, 2, 166–192 CrossRef.
- H. R. El-Seedi, D. M. H. El-Ghorab, M. A. El-Barbary, M. F. Zayed, U. Göransson, S. Larsson and R. Verpoorte, Naturally Occurring Xanthones; Latest Investigations: Isolation, Structure Elucidation and Chemosystematic Significance, Curr. Med. Chem., 2009, 16, 2581–2626 CrossRef CAS PubMed.
- D. R. P. Loureiro, J. X. Soares, J. C. Costa, Á. F. Magalhães, C. M. G. Azevedo, M. M. M. Pinto and C. M. M. Afonso, Structures, Activities and Drug-Likeness of Anti-Infective Xanthone Derivatives Isolated from the Marine Environment: A Review, Molecules, 2019, 24, 243 CrossRef PubMed.
- M. E. Sousa and M. M. M. Pinto, Synthesis of Xanthones: An Overview, Curr. Med. Chem., 2005, 12, 2447–2479 CrossRef CAS PubMed.
- A. Carlos Miguel Gonçalves, A. Carlos Manuel Magalhães and P. Madalena Maria Magalhães, Routes to Xanthones: An Update on the Synthetic Approaches, Curr. Org. Chem., 2012, 16, 2818–2867 CrossRef.
- A. S. Gomes, P. Brandão, C. S. G. Fernandes, M. R. P. C. Silva, E. Sousa and M. M. M. Pinto, Drug-like Properties and ADME of Xanthone Derivatives: The Antechamber of Clinical Trials, Curr. Med. Chem., 2016, 23, 3654–3686 CrossRef CAS PubMed.
- Á. Santos, J. X. Soares, S. Cravo, M. E. Tiritan, S. Reis, C. Afonso, C. Fernandes and M. M. M. Pinto, Lipophilicity assessement in drug discovery: Experimental and theoretical methods applied to xanthone derivatives, J. Chromatogr.
B: Anal. Technol. Biomed. Life Sci., 2018, 1072, 182–192 CrossRef PubMed.
- E. Vitaku, D. T. Smith and J. T. Njardarson, Analysis of the Structural Diversity, Substitution Patterns, and Frequency of Nitrogen Heterocycles among U.S. FDA Approved Pharmaceuticals, J. Med. Chem., 2014, 57, 10257–10274 CrossRef CAS PubMed.
- J. Yan, M. Cheng, F. Hu and Y. Hu, Direct synthesis of functional azaxanthones by using a domino three-component reaction, Org. Lett., 2012, 14, 3206–3209 CrossRef CAS PubMed.
- P. Atkinson, K. S. Findlay, F. Kielar, R. Pal, D. Parker, R. A. Poole, H. Puschmann, S. L. Richardson, P. A. Stenson, A. L. Thompson and J. Yu, Azaxanthones and azathioxanthones are effective sensitisers for europium and terbium luminescence, Org. Biomol. Chem., 2006, 4, 1707–1722 RSC.
- M. M. M. Pinto, M. E. Sousa and M. S. J. Nascimento, Xanthone Derivatives: New Insights in Biological Activities, Curr. Med. Chem., 2005, 12, 2517–2538 CrossRef CAS PubMed.
- X. Chen, J. Leng, K. P. Rakesh, N. Darshini, T. Shubhavathi, H. K. Vivek, N. Mallesha and H. L. Qin, Synthesis and molecular docking studies of xanthone attached amino acids as potential antimicrobial and anti-inflammatory agents, MedChemComm, 2017, 8, 1706–1719 RSC.
- A. Das, M. M. Shaikh and S. Jana, Design, synthesis, and in vitro antibacterial screening of some novel 3-pentyloxy-1-hydroxyxanthone derivatives, Med. Chem. Res., 2014, 23, 436–444 CrossRef CAS.
- T. Kodama, T. Ito, D. F. Dibwe, S. Y. Woo and H. Morita, Syntheses of benzophenone-xanthone hybrid polyketides and their antibacterial activities, Bioorg. Med. Chem. Lett., 2017, 27, 2397–2400 CrossRef CAS PubMed.
- J. Araujo, C. Fernandes, M. Pinto and M. E. Tiritan, Chiral derivatives of Xanthones with antimicrobial activity, Molecules, 2019, 24, 314 CrossRef PubMed.
- A. K. Kumar, V. Sunitha, B. Shankar, T. M. Krishna, C. A. Lincoln and P. Jalapathi, Synthesis and antimicrobial screening of novel 9-{2- (1H-1,2,3-triazol-4-yl)methoxy phenyl}-3,3,6,6-tetramethyl-3,4,5,6,7,9-hexahydro-1H-xanthene-1,8(2H)-dione derivatives, Russ. J. Gen. Chem., 2017, 87, 2011–2020 CrossRef.
- G. Liao, J. Fan, L. Ludwig-Radtke, K. Backhaus and S. M. Li, Increasing Structural Diversity of Natural Products by Michael Addition with ortho-Quinone Methide as the Acceptor, J. Org. Chem., 2020, 85, 1298–1307 CrossRef CAS PubMed.
- S. M. Lin, J. J. Koh, T. T. Aung, F. H. Lim, J. G. Li, H. X. Zou, L. Wang, R. Lakshminarayanan, C. Verma, Y. J. Wang, D. T. H. Tan, D. R. Cao, R. W. Beuerman, L. Ren and S. P. Liu, Symmetrically Substituted Xanthone Amphiphiles Combat Gram-Positive Bacterial Resistance with Enhanced Membrane Selectivity, J. Med. Chem., 2017, 60, 1362–1378 CrossRef CAS PubMed.
- M. Malekpoor, S. Gharaghani, A. Sharifzadeh, S. N. Mirsattari and A. R. Massah, Synthesis and antibacterial evaluation of novel xanthone sulfonamides, J. Chem. Res., 2015, 433–437, DOI:10.3184/174751915x14373971129805.
- A. C. Micheletti, N. K. Honda, N. C. P. Carvalho, D. P. de Lima and A. Beatriz, Design, Synthesis and in vitro Antimicrobial Activity Evaluation of Novel Hybrids of Lichexantone-THC Derivatives, Orbital: Electron. J. Chem., 2015, 7, 301–307 CAS.
- H. X. Zou, J. J. Koh, J. G. Li, S. X. Qiu, T. T. Aung, H. F. Lin, R. Lakshminarayanan, X. P. Dai, C. Tang, F. H. Lim, L. Zhou, A. L. Tan, C. Verma, D. T. H. Tan, H. S. O. Chan, P. Saraswathi, D. R. Cao, S. P. Liu and R. W. Beuerman, Design and Synthesis of Amphiphilic Xanthone-Based, Membrane-Targeting Antimicrobials with Improved Membrane Selectivity (vol 56, pg 2359, 2013), J. Med. Chem., 2013, 56, 3747–3747 CrossRef CAS.
- L. J. Bessa, A. Palmeira, A. S. Gomes, V. Vasconcelos, E. Sousa, M. Pinto and P. M. d. Costa, Synergistic Effects Between Thioxanthones and Oxacillin Against Methicillin-Resistant Staphylococcus aureus, Microb. Drug Resist., 2015, 21, 404–415 CrossRef CAS PubMed.
- D. Resende, P. Pereira-Terra, A. S. Inacio, P. M. da Costa, E. Pinto, E. Sousa and M. M. M. Pinto, Lichen Xanthones as Models for New Antifungal Agents, Molecules, 2018, 23, 2617 CrossRef PubMed.
- E. Pinto, C. Afonso, S. Duarte, L. Vale-Silva, E. Costa, E. Sousa and M. Pinto, Antifungal Activity of Xanthones: Evaluation of their Effect on Ergosterol Biosynthesis by High-performance Liquid Chromatography, Chem. Biol. Drug Des., 2011, 77, 212–222 CrossRef CAS PubMed.
- A. Pedro Gonçalves, N. Silva, C. Oliveira, D. J. Kowbel, N. L. Glass, A. Kijjoa, A. Palmeira, E. Sousa, M. Pinto and A. Videira, Transcription profiling of the Neurospora crassa response to a group of synthetic (thio)xanthones and a natural acetophenone, Genomics Data, 2015, 4, 26–32 CrossRef PubMed.
- R. T. Lima, H. Seca, A. Palmeira, M. X. Fernandes, F. Castro, M. Correia-da-Silva, M. S. J. Nascimento, E. Sousa, M. Pinto and M. H. Vasconcelos, Sulfated Small Molecules Targeting EBV in Burkitt Lymphoma: From In Silico Screening to the Evidence of In Vitro Effect on Viral Episomal DNA, Chem. Biol. Drug Des., 2013, 81, 631–644 CrossRef CAS PubMed.
- E. V. Buravlev, O. G. Shevchenko, A. A. Anisimov and K. Y. Suponitsky, Novel Mannich bases of alpha- and gamma-mangostins: Synthesis and evaluation of antioxidant and membrane-protective activity, Eur. J. Med. Chem., 2018, 152, 10–20 CrossRef CAS PubMed.
- I. Cruz, P. Puthongking, S. Cravo, A. Palmeira, H. Cidade, M. Pinto and E. Sousa, Xanthone and Flavone Derivatives as Dual Agents with Acetylcholinesterase Inhibition and Antioxidant Activity as Potential Anti-Alzheimer Agents, J. Chem., 2017, 2017, 8587260 Search PubMed.
- J. M. Khurana, A. Chaudhary, A. Lumb and B. Nand, Efficient one-pot syntheses of dibenzo a,i xanthene-diones and evaluation of their antioxidant activity, Can. J. Chem. – Rev. Roum. Chim., 2012, 90, 739–746 CrossRef CAS.
- E. Yuanita, H. D. Pranowo, D. Siswanta, R. T. Swasono, Mustofa, A. K. Zulkarnain, J. Syahri and Jumina, One-pot synthesis, antioxidant activity and toxicity evaluation of some hydroxyxanthones, Chem. Chem. Technol., 2018, 12, 290–295 CrossRef CAS.
- B. D. Zhou, L. L. Zeng, Y. G. Tong, J. Y. Fang, Z. P. Ruan, X. Y. Zeng, Y. Y. Fang, G. F. Xu and D. B. Hu, Synthesis and antitumor, antityrosinase, and antioxidant activities of xanthone (vol 20, pg 467, 2018), J. Asian Nat. Prod. Res., 2019, 21, 688–688 CrossRef PubMed.
- H. Cidade, V. Rocha, A. Palmeira, C. Marques, M. E. Tiritan, H. Ferreira, J. S. Lobo, I. F. Almeida, M. E. Sousa and M. Pinto, In silico and in vitro antioxidant and cytotoxicity evaluation of oxygenated xanthone derivatives, Arabian J. Chem., 2020, 13, 17–26 CrossRef CAS.
- R. Urbatzka, S. Freitas, A. Palmeira, T. Almeida, J. Moreira, C. Azevedo, C. Afonso, M. Correia-da-Silva, E. Sousa, M. Pinto and V. Vasconcelos, Lipid reducing activity and toxicity profiles of a library of polyphenol derivatives, Eur. J. Med. Chem., 2018, 151, 272–284 CrossRef CAS PubMed.
- O. Chantarasriwong, A. T. Milcarek, T. H. Morales, A. L. Settle, C. O. Rezende Jr., B. D. Althufairi, M. A. Theodoraki, M. L. Alpaugh and E. A. Theodorakis, Synthesis, structure-activity relationship and in vitro pharmacodynamics of A-ring modified caged xanthones in a preclinical model of inflammatory breast cancer, Eur. J. Med. Chem., 2019, 168, 405–413 CrossRef CAS PubMed.
- C. T. Yen, K. Nakagawa-Goto, T. L. Hwang, S. L. Morris-Natschke, K. F. Bastow, Y. C. Wu and K. H. Lee, Design and synthesis of gambogic acid analogs as potent cytotoxic and anti-inflammatory agents, Bioorg. Med. Chem. Lett., 2012, 22, 4018–4022 CrossRef CAS PubMed.
- D. Zelaszczyk, A. Lipkowska, N. Szkaradek, K. Sloczynska, A. Gunia-Krzyzak, T. Librowski and H. Marona, Synthesis and preliminary anti-inflammatory evaluation of xanthone derivatives, Heterocycl. Commun., 2018, 24, 231–236 CAS.
- M. Correia-da-Silva, E. Sousa, B. Duarte, F. Marques, F. Carvalho, L. M. Cunha-Ribeiro and M. M. M. Pinto, Polysulfated Xanthones: Multipathway Development of a New Generation of Dual Anticoagulant/Antiplatelet Agents, J. Med. Chem., 2011, 54, 5373–5384 CrossRef CAS PubMed.
- R. Shen, W. H. Wang and G. L. Yang, DNA Binding Property and Antitumor Evaluation of Xanthone with Dimethylamine Side Chain, J. Fluoresc., 2014, 24, 959–966 CrossRef CAS PubMed.
- F. M. Soliman, M. M. Said, M. Youns and S. A. Darwish, Chemistry of phosphorus ylides 32: synthesis of phosphoranylidene-pyrano- and -cyclobutyl-xanthenones with potential antitumor activity, Monatsh. Chem., 2012, 143, 965–973 CrossRef CAS.
- C. M. G. Azevedo, C. M. M. Afonso, J. X. Soares, S. Reis, D. Sousa, R. T. Lima, M. H. Vasconcelos, M. Pedro, J. Barbosa, L. Gales and M. M. M. Pinto, Pyranoxanthones: Synthesis, growth inhibitory activity on human tumor cell lines and determination of their lipophilicity in two membrane models, Eur. J. Med. Chem., 2013, 69, 798–816 CrossRef CAS PubMed.
- C. M. G. Azevedo, C. M. M. Afonso, D. Sousa, R. T. Lima, M. H. Vasconcelos, M. Pedro, J. Barbosa, A. G. Correa, S. Reis and M. M. M. Pinto, Multidimensional optimization of promising antitumor xanthone derivatives, Bioorg. Med. Chem., 2013, 21, 2941–2959 CrossRef CAS PubMed.
- C. Fernandes, K. Masawang, M. E. Tiritan, E. Sousa, V. de Lima, C. Afonso, H. Bousbaa, W. Sudprasert, M. Pedro and M. M. Pinto, New chiral derivatives of xanthones: Synthesis and investigation of enantioselectivity as inhibitors of growth of human tumor cell lines, Bioorg. Med. Chem., 2014, 22, 1049–1062 CrossRef CAS PubMed.
- W. L. Lan, J. C. Wei, J. K. Qin, Z. M. Yang, G. F. Su and Z. K. Dai, Synthesis and Biological Evaluation of 1, 3-Dihydroxyxanthone Mannich Base Derivatives as Potential Antitumor Agents, Lett. Drug Des. Discovery, 2013, 10, 689–698 CAS.
- X. Li, X. J. Zhang, X. J. Wang, N. G. Li, C. J. Lin, Y. Gao, Z. Q. Yu, Q. L. Guo and Q. D. You, Synthesis and Anti-tumor Evaluation of B-ring Modified Caged Xanthone Analogues of Gambogic Acid, Chin. J. Chem., 2012, 30, 35–42 CrossRef CAS.
- C. K. Lim, L. Y. Tho, Y. M. Lim, S. A. A. Shah and J. F. F. Weber, Synthesis of 1,3,6-Trioxygenated Prenylated Xanthone Derivatives as Potential Antitumor Agents, Lett. Org. Chem., 2012, 9, 549–555 CrossRef CAS.
- L. Luo, J. K. Qin, Z. K. Dai and S. H. Gao, Synthesis and biological evaluation of novel benzo b xanthone derivatives as potential antitumor agents, J. Serb. Chem. Soc., 2013, 78, 1301–1308 CrossRef CAS.
- X. J. Zhan, X. Li, H. P. Sun, X. J. Wang, L. Zhao, Y. Gao, X. R. Liu, S. L. Zhang, Y. Y. Wang, Y. R. Yang, S. Zeng, Q. L. Guo and Q. D. You, Garcinia Xanthones as Orally Active Antitumor Agents, J. Med. Chem., 2013, 56, 276–292 CrossRef PubMed.
- X. J. Zhang, X. Li, S. F. Ye, Y. Zhang, L. Tao, Y. Gao, D. D. Gong, M. Y. Xi, H. Y. Meng, M. Q. Zhang, W. L. Gao, X. L. Xu, Q. L. Guo and Q. D. You, Synthesis, SAR and Biological Evaluation of Natural and Non-natural Hydroxylated and Prenylated Xanthones as Antitumor Agents, Med. Chem., 2012, 8, 1012–1025 CAS.
- Y. Zou, Q. J. Zhao, H. G. Hu, L. N. Hu, S. C. Yu, M. J. Xu and Q. Y. Wu, Synthesis and in vitro antitumor activities of xanthone derivatives containing 1,4-disubstituted-1,2,3-triazole moiety, Arch. Pharmacal Res., 2012, 35, 2093–2104 CrossRef CAS PubMed.
- A. Alves, J. Campos, D. Ferreira, P. C. Costa, A. Alves, M. Correia-da-Silva, E. Sousa, M. Pinto, M. Correia-da-Silva, E. Sousa, H. Bousbaa, M. Pinto, C. Nunes, P. M. A. Silva, H. Bousbaa and F. Rodrigues, Discovery of a New Xanthone against Glioma: Synthesis and Development of (Pro)liposome Formulations, Molecules, 2019, 24, 409 CrossRef PubMed.
- J. Barbosa, R. T. Lima, D. Sousa, A. S. Gomes, A. Palmeira, H. Seca, K. Choosang, P. Pakkong, H. Bousbaa, M. M. Pinto, E. Sousa, M. H. Vasconcelos and M. Pedro, Screening a Small Library of Xanthones for Antitumor Activity and Identification of a Hit Compound which Induces Apoptosis, Molecules, 2016, 21, 81 CrossRef PubMed.
- C. Elisangela, S. Emilia, N. Nair, S. J. N. Maria and M. M. P. Madalena, Synthesis of Xanthones and Benzophenones as Inhibitors of Tumor Cell Growth, Lett. Drug Des. Discovery, 2010, 7, 487–493 CrossRef.
- A. Palmeira, A. Paiva, E. Sousa, H. Seca, G. M. Almeida, R. T. Lima, M. X. Fernandes, M. Pinto and M. H. Vasconcelos, Insights into the In Vitro Antitumor Mechanism of Action of a New Pyranoxanthone, Chem. Biol. Drug Des., 2010, 76, 43–58 CrossRef CAS PubMed.
- P. S. Bairy, A. Das, L. M. Nainwal, T. K. Mohanta, M. K. Kumawat, P. K. Mohapatra and P. Parida, Design, synthesis and anti-diabetic activity of some novel xanthone derivatives targeting alpha-glucosidase, Bangladesh J. Pharmacol., 2016, 11, 308–318 CrossRef.
- S. M. Ding, T. Lan, G. J. Ye, J. J. Huang, Y. Hu, Y. R. Zhu and B. Wang, Novel oxazolxanthone derivatives as a new type of alpha-glucosidase inhibitor: synthesis, activities, inhibitory modes and synergetic effect, Bioorg. Med. Chem., 2018, 26, 3370–3378 CrossRef CAS PubMed.
- Z. Heydari, M. Mohammadi-Khanaposhtani, S. Imanparast, M. A. Faramarzi, M. Mandavi, P. R. Ranjbar and B. Larijani, Pyrano 3,2-c quinoline Derivatives as New Class of alpha-glucosidase Inhibitors to Treat Type 2 Diabetes: Synthesis, in vitro Biological Evaluation and Kinetic Study, Med. Chem., 2019, 15, 8–16 CrossRef CAS PubMed.
- G. L. Li, C. Y. Cai, J. Y. He, L. Rao, L. Ma, Y. Liu and B. Wang, Synthesis of 3-acyloxyxanthone derivatives as alpha-glucosidase inhibitors: A further insight into the 3-substituents’ effect, Bioorg. Med. Chem., 2016, 24, 1431–1438 CrossRef CAS PubMed.
- G.-J. Ye, T. Lan, Z.-X. Huang, X.-N. Cheng, C.-Y. Cai, S.-M. Ding, M.-L. Xie and B. Wang, Design and synthesis of novel xanthone-triazole derivatives as potential antidiabetic agents: α-Glucosidase inhibition and glucose uptake promotion, Eur. J. Med. Chem., 2019, 177, 362–373 CrossRef CAS PubMed.
- P. F. Cheng, L. J. Zhu, W. Guo, W. F. Liu, J. Z. Yao, G. Q. Dong, Y. Q. Zhang, C. L. Zhuang, C. Q. Sheng, Z. Y. Miao and W. N. Zhang, Synthesis of novel benzoxanthone analogues as non-Camptothecin topoisomerase I inhibitors, J. Enzyme Inhib. Med. Chem., 2012, 27, 437–442 CrossRef CAS PubMed.
- S. Park, E. Hong, S. Y. Kwak, K. Y. Jun, E. S. Lee, Y. Kwon and Y. Na, Synthesis and biological evaluation of C1-O-substituted-3-(3-butylamino-2-hydroxy-propoxy)-xanthen-9-one as topoisomerase II alpha catalytic inhibitors, Eur. J. Med. Chem., 2016, 123, 211–225 CrossRef CAS PubMed.
- E. Martins, V. Silva, A. Lemos, A. Palmeira, P. Puthongking, E. Sousa, C. Rocha-Pereira, C. I. Ghanem, H. Carmo, F. Remiao and R. Silva, Newly synthesized oxygenated xanthones as potential P-glycoprotein activators: in vitro, ex vivo, and in silico studies, Molecules, 2019, 24, 707 CrossRef CAS PubMed.
- R. Silva, E. Sousa, H. Carmo, A. Palmeira, D. J. Barbosa, M. Gameiro, M. Pinto, M. de Lourdes Bastos and F. Remião, Induction and activation of P-glycoprotein by dihydroxylated xanthones protect against the cytotoxicity of the P-glycoprotein substrate paraquat, Arch. Toxicol., 2014, 88, 937–951 CrossRef CAS PubMed.
- E. Sousa, A. Palmeira, A. S. Cordeiro, B. Sarmento, D. Ferreira, R. T. Lima, M. Helena Vasconcelos and M. Pinto, Bioactive xanthones with effect on P-glycoprotein and prediction of intestinal absorption, Med. Chem. Res., 2013, 22, 2115–2123 CrossRef CAS.
- M. S. Alawi, T. A. Awad, M. A. Mohamed, A. Khalid, E. M. O. Ismail, F. Alfatih, S. Naz and Z. Ul-Haq, Insights into the molecular basis of acetylcholinesterase inhibition by xanthones: an integrative in silico and in vitro approach, Mol. Simul., 2020, 46, 253–261 CrossRef CAS.
- C. A. Menendez, B. Biscussi, S. Accordino, A. P. Murray, D. C. Gerbino and G. A. Appignanesi, Design, synthesis and biological evaluation of 1,3-dihydroxyxanthone derivatives: Effective agents against acetylcholinesterase, Bioorg. Chem., 2017, 75, 201–209 CrossRef CAS PubMed.
- A. Lemos, A. S. Gomes, J. B. Loureiro, P. Brandao, A. Palmeira, M. M. M. Pinto, L. Saraiva and M. E. Sousa, Synthesis, biological evaluation, and in silico studies of novel aminated xanthones as potential p53-activating agents, Molecules, 2019, 24, 1975 CrossRef PubMed.
- S. Gomes, L. Raimundo, J. Soares, J. B. Loureiro, M. Leão, H. Ramos, M. N. Monteiro, A. Lemos, J. Moreira, M. Pinto, P. Chlapek, R. Veselska, E. Sousa and L. Saraiva, New inhibitor of the TAp73 interaction with MDM2 and mutant p53 with promising antitumor activity against neuroblastoma, Cancer Lett., 2019, 446, 90–102 CrossRef CAS PubMed.
- M. Leão, S. Gomes, J. Pedraza-Chaverri, N. Machado, E. Sousa, M. Pinto, A. Inga, C. Pereira and L. Saraiva, α-Mangostin and Gambogic Acid as Potential Inhibitors of the p53−MDM2 Interaction Revealed by a Yeast Approach, J. Nat. Prod., 2013, 76, 774–778 CrossRef PubMed.
- M. Leão, C. Pereira, A. Bisio, Y. Ciribilli, A. M. Paiva, N. Machado, A. Palmeira, M. X. Fernandes, E. Sousa, M. Pinto, A. Inga and L. Saraiva, Discovery of a new small-molecule inhibitor of p53−MDM2 interaction using a yeast-based approach, Biochem. Pharmacol., 2013, 85, 1234–1245 CrossRef PubMed.
-
A. Semov, G. Pietrzynski and V. Alakhov, Method of treating tumors with azaxanthones, Supratek Pharma Inc, US Pat., US7772255B2, 2010 Search PubMed.
- G. Kolokythas, I. K. Kostakis, N. Pouli, P. Marakos, D. Kletsas and H. Pratsinis, Synthesis and cytotoxic activity of some new azapyranoxanthenone aminoderivatives, Bioorg. Med. Chem., 2003, 11, 4591–4598 CrossRef CAS PubMed.
-
M. M. Perez, R. M. Docampo, J. M. P. Codina, J. R. Moliner and J. S. Solá, 1-azaxanthone for use as therapeutic agent, processes for its production and pharmaceutical compositions, Lacer SA, European Pat., 0075607B1, 1985 Search PubMed.
- F. J. Villani, T. A. Mann, E. A. Wefer, J. Hannon, L. L. Larca, M. J. Landon, W. Spivak, D. Vashi and S. Tozzi, Benzopyranopyridine derivatives. 1. Aminoalkyl derivatives of the azaxanthenes as bronchodilating agents, J. Med. Chem., 1975, 18, 1–8 CrossRef CAS PubMed.
- W. Meng, Y. Dong, J. Liu, Z. Wang, X. Zhong, R. Chen, H. Zhou, M. Lin, L. Jiang, F. Gao, T. Xu, Q. Chen and X. Zeng, A clinical evaluation of amlexanox oral adhesive pellicles in the treatment of recurrent aphthous stomatitis and comparison with amlexanox oral tablets: a randomized, placebo controlled, blinded, multicenter clinical trial, Trials, 2009, 10, 30–30 CrossRef PubMed.
-
D. Rakel, in Integrative Medicine, ed. D. Rakel, Elsevier, 4th edn, 2018, pp. 747–752, DOI:10.1016/B978-0-323-35868-2.00075-X.
- A. Michael, On the action of aromatic oxy-acids on phenols, Ber. Dtsch. Chem. Ges., 1883, 5, 81 Search PubMed.
- S. V. Kostanecki and B. Nessler, Synthesen von Oxyxanthonen, Ber. Dtsch. Chem. Ges., 1891, 24, 1894–1897 CrossRef.
-
F. M. Dean, in The Total Synthesis of Natural Products, ed. J. Apsimon, 1973, pp. 534–562 Search PubMed.
- M. Afzal and J. M. Al-Hassan, Synthesis and Biosynthesis of Phytoxanthones, Heterocycles, 1980, 14, 1173–1205 CrossRef CAS.
- J. Ribeiro, C. Veloso, C. Fernandes, M. E. Tiritan and M. M. M. Pinto, Carboxyxanthones: bioactive agents and molecular scaffold for synthesis of analogues and derivatives, Molecules, 2019, 24, 180 CrossRef PubMed.
- R. Khanna, A. Dalal, R. Kumar and R. C. Kamboj, Synthesis of Xanthenones: A Review, ChemistrySelect, 2016, 1, 840–851 CrossRef CAS.
- C. M. M. Santos, D. Pinto, V. L. M. Silva and A. M. S. Silva, Arylxanthones and arylacridones: a synthetic overview, Pure Appl. Chem., 2016, 88, 579–594 CAS.
- A. M. Paiva, M. M. Pinto and E. Sousa, A Century of Thioxanthones: Through Synthesis and Biological Applications, Curr. Med. Chem., 2013, 20, 2438–2457 CrossRef CAS PubMed.
- D. Parker, J. W. Walton, L. Lamarque and J. M. Zwier, Comparative Study of the Luminescence Properties and Relative Stability of a Series of Europium(III) Complexes Bearing One to Four Coordinated Azaxanthone Groups, Eur. J. Inorg. Chem., 2010, 2010, 3961–3966 CrossRef.
- C. Coenjarts and J. C. Scaiano, Reaction Pathways Involved in the Quenching of the Photoactivated Aromatic Ketones Xanthone and 1-Azaxanthone by Polyalkylbenzenes, J. Am. Chem. Soc., 2000, 122, 3635–3641 CrossRef CAS.
- S. Corrent, L. J. Martínez, J. C. Scaiano, H. García and V. Fornés, Intrazeolite Photochemistry. Photochemistry of 1-Azaxanthone in Zeolites in the Presence of Hydrogen Donors, Electron Donors, and Energy Acceptors, J. Phys. Chem. B, 1999, 103, 8097–8103 CrossRef CAS.
- L. J. Martínez and J. C. Scaiano, The Photochemistry of 1-Azaxanthone in Aqueous Solutions and in Micellar Environments, J. Phys. Chem. A, 1999, 103, 203–208 CrossRef.
- M. Sakamoto, X. Cai, M. Hara, S. Tojo, M. Fujitsuka and T. Majima, Anomalous Fluorescence from the Azaxanthone Ketyl Radical in the Excited State, J. Am. Chem. Soc., 2005, 127, 3702–3703 CrossRef CAS PubMed.
- J. C. Scaiano, D. Weldon, C. N. Pliva and L. J. Martínez, Photochemistry and Photophysics of 1-Azaxanthone in Organic Solvents, J. Phys. Chem. A, 1998, 102, 6898–6903 CrossRef CAS.
- P. K. Grover, G. D. Shah and R. C. Shah, Xanthones. Part IV. A new synthesis of hydroxyxanthones and hydroxybenzophenones, J. Chem. Soc., 1955, 3982–3985, 10.1039/JR9550003982.
- P. K. Gover, G. D. Shah and R. C. Shah, Xanthones. Part III – A New Synthesis of Hydroxyxanthones and Hydrozybenzophenones. Part 111, J. Sci. Ind. Res., 1954, 13, 175 Search PubMed.
- J. K. Qin, W. L. Lan, Z. Liu, J. Huang, H. Tang and H. S. Wang, Synthesis and biological evaluation of 1, 3-dihydroxyxanthone mannich base derivatives as anticholinesterase agents, Chem. Cent. J., 2013, 7, 78 CrossRef PubMed.
- Q. R. Shen, Y. Dai, G. H. Wang, F. Yao, Y. H. Duan, H. F. Chen, W. G. Zhang, X. K. Zhang and X. S. Yao, Total synthesis and RXR alpha-mediated transcription studies of neriifolone B and related compounds, Bioorg. Med. Chem., 2014, 22, 2671–2677 CrossRef CAS PubMed.
- Z. M. Yang, J. Huang, J. K. Qin, Z. K. Dai, W. L. Lan, G. F. Su, H. Tang and F. Yang, Design, synthesis and biological evaluation of novel 1-hydroxyl-3-aminoalkoxy xanthone derivatives as potent anticancer agents, Eur. J. Med. Chem., 2014, 85, 487–497 CrossRef CAS PubMed.
- N. Guo, J. Liu, L. Qin, D. Jiang, X. You, K. Lu, Y. O. Teng and P. Yu, Synthesis and antitumor activity evaluation of a novel series of xanthone derivatives, J. Asian Nat. Prod. Res., 2015, 17, 377–383 CrossRef CAS PubMed.
- J. Liu, J. R. Zhang, H. L. Wang, Z. J. Liu, C. Zhang, Z. L. Jiang and H. R. Chen, Synthesis of xanthone derivatives and studies on the inhibition against cancer cells growth and synergistic combinations of them, Eur. J. Med. Chem., 2017, 133, 50–61 CrossRef CAS PubMed.
- Z. L. Jiang, J. Liu, F. Zhou, J. R. Zhang, Z. J. Liu, C. Zhang and H. R. Chen, One-step Construction of Xanthone Scaffold Assisted by Microwave Irradiation to Optimize the Synthesis of DMXAA, Chem. Res. Chin. Univ., 2018, 34, 918–922 CrossRef CAS.
- O. Goshain and B. Ahmed, Antihypertensive activity, toxicity and molecular docking study of newly synthesized xanthon derivatives (xanthonoxypropanolamine), PLoS One, 2019, 14, e0220920 CrossRef CAS PubMed.
- J. Liu, H. Bao, H. Wang, Q. Luo, J. Zuo, Z. Liu, S. Qiu, X. Sun and X. Liu, Synthesis of xanthone derivatives and anti-hepatocellular carcinoma potency evaluation: induced apoptosis, RSC Adv., 2019, 9, 40781–40791 RSC.
- X. J. Zhang, X. Li, H. P. Sun, Z. Y. Jiang, L. Tao, Y. Gao, Q. L. Guo and Q. D. You, Synthesis and evaluation of novel aza-caged Garcinia xanthones, Org. Biomol. Chem., 2012, 10, 3288–3299 RSC.
- M. Dai, X. Yuan, Z. J. Zhu, L. Shan, R. H. Liu, Q. Y. Sun and W. D. Zhang, Efficient Total Synthesis and Biological Activities of 6-Deoxyisojacareubin, Arch. Pharm., 2013, 346, 314–320 CrossRef CAS PubMed.
- S. S. Mao, H. H. Zheng, C. T. Luo and H. R. Chen, Concise Syntheses of 1,3-Dihydroxylxanthones and Their Clearance Effections against Nitrite, Chin. J. Org. Chem., 2014, 34, 515–521 CrossRef CAS.
- F. L. Qu, G. J. Bai, W. S. Dong, Y. Yang, Y. S. Jin, Q. G. Meng, Q. Y. Wu, W. Guo and S. C. Yu, Synthesis and Evaluation of Novel Xanthone Derivatives as Potent AChE Inhibitors, Asian – J. Chem., 2014, 26, 3496–3498 CrossRef CAS.
- X. Li, Y. Zou, Q. J. Zhao, Y. Yang, M. C. Wu, T. Huang, H. G. Hu and Q. Y. Wu, Synthesis, Biological Evaluation, and Molecular Docking Studies of Xanthone Sulfonamides as ACAT Inhibitors, Chem. Biol. Drug Des., 2015, 85, 394–403 CrossRef CAS PubMed.
- G. P. Song, S. M. Li, H. Z. Si, Y. B. Li, Y. S. Li, J. H. Fan, Q. Q. Liang, H. B. He, H. M. Ye and Z. N. Cui, Synthesis and bioactivity of novel xanthone and thioxanthone L-rhamnopyranosides, RSC Adv., 2015, 5, 36092–36103 RSC.
- J. J. Koh, H. X. Zou, S. M. Lin, H. F. Lin, R. T. Soh, F. H. Lim, W. L. Koh, J. G. Li, R. Lakshminarayanan, C. Verma, D. T. H. Tan, D. R. Cao, R. W. Beuerman and S. P. Liu, Nonpeptidic Amphiphilic Xanthone Derivatives: Structure-Activity Relationship and Membrane-Targeting Properties, J. Med. Chem., 2016, 59, 171–193 CrossRef CAS PubMed.
- K. D. Reichl, M. J. Smith, M. K. Song, R. P. Johnson and J. A. Porco, Biomimetic Total Synthesis of (+/−)-Griffipavixanthone via a Cationic Cycloaddition-Cyclization Cascade, J. Am. Chem. Soc., 2017, 139, 14053–14056 CrossRef CAS PubMed.
- J. Syahri, E. Yuanita, B. A. Nurohmah, M. H. Wathon, R. Syafri, R. Armunanto and B. Purwono, Xanthone as Antimalarial: QSAR Analysis, Synthesis, Molecular Docking and In vitro Antimalarial Evaluation, Orient. J. Chem., 2017, 33, 29–40 CrossRef CAS.
- X. Q. Chi, C. T. Zi, H. M. Li, L. Yang, Y. F. Lv, J. Y. Li, B. Hou, F. C. Ren, J. M. Hu and J. Zhou, Design, synthesis and structure-activity relationships of mangostin analogs as cytotoxic agents, RSC Adv., 2018, 8, 41377–41388 RSC.
- T. Khammee, W. Jongsu, M. Kuno and S. Suksanrarn, Allylxanthone Derivatives as Xanthine Oxidase Inhibitors: Synthesis, Biological Evaluation and Molecular Docking Study, Orient. J. Chem., 2018, 34, 38–44 CrossRef CAS.
- P. H. Wang, L. L. Jiang, Y. Cao, D. Y. Ye and L. Zhou, The Design and Synthesis of N-Xanthone Benzenesulfonamides as Novel Phosphoglycerate Mutase 1 (PGAM1) Inhibitors, Molecules, 2018, 23, 1396 CrossRef PubMed.
- P. F. Zhou, A. J. Hou and Y. Wang, Total Synthesis of Cudratricusxanthone B, Chin. J. Org. Chem., 2018, 38, 156–161 CrossRef CAS.
- J. Wu, J. Dai, Y. Zhang, J. Wang, L. Huang, H. Ding, T. Li, Y. Zhang, S. Yu, J. Wu, J. Dai, Y. Zhang, T. Li, Y. Zhang and J. Mao, Synthesis of Novel Xanthone Analogues and Their Growth Inhibitory Activity Against Human Lung Cancer A549 Cells, Drug Des., Dev. Ther., 2019, 13, 4239–4246 CrossRef CAS PubMed.
- L. Yu, L. Chen, G. Luo, L. Liu, W. Zhu, P. Zhang, C. Zhang, W. Wu, L. Chen and P. Yan, Study on Synthesis and Biological Evaluation of 3-Aryl Substituted Xanthone Derivatives as Novel and Potent Tyrosinase Inhibitors, Chem. Pharm. Bull., 2019, 67, 1232–1241 CrossRef CAS.
- E. Yuanita, H. D. Pranowo, M. Mustofa, R. T. Swasono, J. Syahri and J. Jumina, Synthesis, Characterization and Molecular Docking of Chloro-substituted Hydroxyxanthone Derivatives, Chem. J. Mold., 2019, 14, 68–76 CAS.
- X. D. Kou, L. L. Song, Y. H. Wang, Q. Yu, H. Ju, A. H. Yang and R. Shen, Design, synthesis and anti-Alzheimer’s disease activity study of xanthone derivatives based on multi-target strategy, Bioorg. Med. Chem. Lett., 2020, 30, 126927 CrossRef CAS PubMed.
- S. Genovese, S. Fiorito, M. C. Specchiulli, V. A. Taddeo and F. Epifano, Microwave-assisted synthesis of xanthones promoted by ytterbium triflate, Tetrahedron Lett., 2015, 56, 847–850 CrossRef CAS.
- J. Hu, E. A. Adogla, Y. Ju, D. Fan and Q. Wang, Copper-catalyzed ortho-acylation of phenols with aryl aldehydes and its application in one-step preparation of xanthones, Chem. Commun., 2012, 48, 11256–11258 RSC.
- C. A. Menendéz, F. Nador, G. Radivoy and D. C. Gerbino, One-Step Synthesis of Xanthones Catalyzed by a Highly Efficient Copper-Based Magnetically Recoverable Nanocatalyst, Org. Lett., 2014, 16, 2846–2849 CrossRef PubMed.
- T. Ramani, P. Umadevi, K. L. Prasanth and B. Sreedhar, Synthesis of ortho-Hydroxybenzophenones Catalyzed by Magnetically Retrievable Fe3O4 Nanoparticles under Ligand-Free Conditions, Eur. J. Org. Chem., 2013, 2013, 6021–6026 CrossRef CAS.
- A. Venkanna, P. V. K. Goud, P. V. Prasad, M. Shanker and P. V. Rao, One-Step Synthesis of Xanthones and Thio-Xanthones by Using Magnetically Separable Copper ferrite Nanoparticles as a Catalyst under Ligand Free Conditions, ChemistrySelect, 2016, 1, 2271–2276 CrossRef CAS.
- H. S. Steingruber, P. Mendioroz, A. S. Diez and D. C. Gerbino, A Green Nanopalladium-Supported Catalyst for the Microwave-Assisted Direct Synthesis of Xanthones, Synthesis, 2020, 52, 619–628 CrossRef CAS.
- C. R. Shen and X. F. Wu, Selective Preparation of Xanthones from 2-Bromofluorobenzenes and Salicylaldehydes via Palladium-Catalyzed Acylation-SNAr Approach, Synlett, 2016, 27, 1269–1273 CrossRef CAS.
- A. V. Dubrovskiy and R. C. Larock, Intermolecular C-O addition of carboxylic acids to arynes: synthesis of o-hydroxyaryl ketones, xanthones, 4-chromanones, and flavones, Tetrahedron, 2013, 69, 2789–2798 CrossRef CAS PubMed.
- Y. Fujimoto, H. Yanai and T. Matsumoto, Concise Total Synthesis of Elliptoxanthone A by Utilizing Aromatic Oxy-Cope Rearrangement for Efficient C-Isoprenylation of Xanthone Skeleton, Synlett, 2016, 27, 2229–2232 CrossRef CAS.
- A. Little and J. A. Porco, Total Syntheses of Graphisin A and Sydowinin B, Org. Lett., 2012, 14, 2862–2865 CrossRef CAS PubMed.
- T. Xie, C. Zheng, K. Chen, H. He and S. Gao, Asymmetric Total Synthesis of the Complex Polycyclic Xanthone FD-594, Angew. Chem., Int. Ed., 2020, 59, 4360 CrossRef CAS PubMed.
- S. Ito, T. Kitamura, S. Arulmozhiraja, K. Manabe, H. Tokiwa and Y. Suzuki, Total Synthesis of Termicalcicolanone A via Organocatalysis and Regioselective Claisen Rearrangement, Org. Lett., 2019, 21, 2777–2781 CrossRef CAS PubMed.
- D. D. Xu, Y. Nie, X. Z. Liang, L. Ji, S. Y. Hu, Q. D. You, F. Wang, H. C. Ye and J. X. Wang, A Concise and Efficient Total Synthesis of alpha-Mangostin and beta-Mangostin from Garcinia mangostana, Nat. Prod. Commun., 2013, 8, 1101–1103 CAS.
- X. Wei, D. L. Liang, Q. Wang, X. B. Meng and Z. J. Li, Total synthesis of mangiferin, homomangiferin, and neomangiferin, Org. Biomol. Chem., 2016, 14, 8821–8831 RSC.
- Q. X. Qin, J. Yang, B. Yang, M. Liu, D. Xiao and X. M. Yang, A facile synthesis of norathyriol, J. Chem. Res., 2013, 38–39, DOI:10.3184/174751912x13547158602399.
- M. Dai, X. Yuan, J. Kang, Z. J. Zhu, R. C. Yue, H. Yuan, B. Y. Chen, W. D. Zhang, R. H. Liu and Q. Y. Sun, Synthesis and biological evaluation of phenyl substituted polyoxygenated xanthone derivatives as anti-hepatoma agents, Eur. J. Med. Chem., 2013, 69, 159–166 CrossRef CAS.
- K. M. Elbel, G. Guizzunti, M. A. Theodoraki, J. Xu, A. Batova, M. Dakanali and E. A. Theodorakis, A-ring oxygenation modulates the chemistry and bioactivity of caged Garcinia xanthones, Org. Biomol. Chem., 2013, 11, 3341–3348 RSC.
- C. M. Liu, M. Zhang, Z. H. Zhang, S. B. Zhang, S. M. Yang, A. Zhang, L. J. Yin, S. Swarts, S. Vidyasagar, L. R. Zhang and P. Okunieff, Synthesis and anticancer potential of novel xanthone derivatives with 3,6-substituted chains, Bioorg. Med. Chem., 2016, 24, 4263–4271 CrossRef CAS PubMed.
- P. H. Wang, L. L. Jiang, Y. Cao, X. D. Zhang, B. J. Chen, S. Y. Zhang, K. Huang, D. Y. Ye and L. Zhou, Xanthone derivatives as phosphoglycerate mutase 1 inhibitors: Design, synthesis, and biological evaluation, Bioorg. Med. Chem., 2018, 26, 1961–1970 CrossRef CAS PubMed.
- M. L. N. Rao and B. S. Ramakrishna, Rh-catalyzed direct synthesis of 2,2 ‘-dihydroxybenzophenones and xanthones, RSC Adv., 2016, 6, 75505–75511 RSC.
- X. J. Zhang, S. F. Ye, Y. Zhang, H. Y. Meng, M. Q. Zhang, W. L. Gao and Q. D. You, Microwave-assisted efficient and green synthesis of hydroxyxanthone in water, Synth. Commun., 2012, 42, 2952–2958 CrossRef CAS.
- X. J. Zhang, L. Yang, Y. Wu, J. Y. Du, Y. L. Mao, X. Wang, S. J. Luan, Y. H. Lei, X. Li, H. P. Sun and Q. D. You, Microwave-assisted transition-metal-free intramolecular Ullmann-type O-arylation in water for the synthesis of xanthones and azaxanthones, Tetrahedron Lett., 2014, 55, 4883–4887 CrossRef CAS.
- S. Fuse, K. Matsumura, K. Johmoto, H. Uekusa, H. Tanaka, T. Hirose, T. Sunazuka, S. Omura and T. Takahashi, The Design, Synthesis, and Evaluation of 1,5,7-Trisubstituted-3-Pyridyl-Xanthones for Use as Insecticides Starting from PyripyropeneA, Chem. – Eur. J., 2016, 22, 18450–18455 CrossRef CAS PubMed.
- M. M. Johnson, J. M. Naidoo, M. A. Fernandes, E. M. Mmutlane, W. A. L. van Otterlo and C. B. de Koning, CAN-Mediated Oxidations for the Synthesis of Xanthones and Related Products (vol 75, pg 8701, 2010), J. Org. Chem., 2019, 84, 472–472 CrossRef CAS PubMed.
- J. Dam, M. L. Bode and C. B. de Koning, Ceric Ammonium Sulfate (CAS) Mediated Oxidations of Benzophenones Possessing a Phenolic Substituent for the Synthesis of Xanthones and Related Products, J. Org. Chem., 2019, 84, 150–160 CrossRef CAS PubMed.
- N. Marquise, P. Harford, F. Chevallier, T. Roisnel, V. Dorcet, A.-L. Gagez, S. Sablé, L. Picot, V. Thiéry, A. Wheatley, P. Gros and F. Mongin, Synthesis of azafluorenones and related compounds using deprotocupration-aroylation followed by intramolecular direct arylation, Tetrahedron, 2013, 69, 10123 CrossRef CAS.
- F. Marsais, F. Trécourt, P. Bréant and G. Quéguiner, Directed lithiation of 4-halopyridines: Chemoselectivity, regioselectivity and application to synthesis, J. Heterocycl. Chem., 1988, 25, 81–87 CrossRef CAS.
- W. Qian, J. Brown, J. J. Chen and Y. Cheng, Regioselective synthesis of multiply halogenated azaxanthones, Tetrahedron Lett., 2014, 55, 7229–7232 CrossRef CAS.
- F. Trécourt, G. Breton, V. Bonnet, F. Mongin, F. Marsais and G. Quéguiner, New Syntheses of Substituted Pyridines via Bromine–Magnesium Exchange, Tetrahedron, 2000, 56, 1349–1360 CrossRef.
- C. Fernandes, L. Oliveira, M. E. Tiritan, L. Leitao, A. Pozzi, J. B. Noronha-Matos, P. Correia-de-Sa and M. M. Pinto, Synthesis of new chiral xanthone derivatives acting as nerve conduction blockers in the rat sciatic nerve, Eur. J. Med. Chem., 2012, 55, 1–11 CrossRef CAS PubMed.
- W. J. Liu, D. S. Mei and W. H. Duan, Synthesis of 1,7-dimethoxy-2-hydroxyxanthone, a natural product with potential activity on erectile dysfunction, Chin. Chem. Lett., 2013, 24, 515–517 CrossRef CAS.
- N. Fukushima, N. Ieda, M. Kawaguchi, K. Sasakura, T. Nagano, K. Hanaoka, N. Miyata and H. Nakagawa, Development of photo-controllable hydrogen sulfide donor applicable in live cells, Bioorg. Med. Chem. Lett., 2015, 25, 175–178 CrossRef CAS PubMed.
- P. Lorenz, A. Heller, M. Bunse, M. Heinrich, M. Berger, J. Conrad, F. C. Stintzing and D. R. Kammerer, Structure Elucidation of the Main Tetrahydroxyxanthones of Hypericum Seeds and Investigations into the Testa Structure, Chem. Biodiversity, 2018, 15, e1800035 CrossRef.
- C. Hoppmann, U. Alexiev, E. Irran and K. Ruck-Braun, Synthesis and fluorescence of xanthone amino acids, Tetrahedron Lett., 2013, 54, 4585–4587 CrossRef CAS.
- M. Presset, J. Paul, G. N. Cherif, N. Ratnam, N. Laloi, E. Leonel, C. Gosmini and E. Le Gall, CoI-Catalyzed Barbier Reactions of Aromatic Halides with Aromatic Aldehydes and Imines, Chem. – Eur. J., 2019, 25, 4491–4495 CrossRef CAS PubMed.
- S. Sarkar and N. Tadigoppula, PhI(OAc)(2)-BF3-OEt2 mediated domino imine activation, intramolecular C-C bond formation and beta-elimination: new approach for the synthesis of fluorenones, xanthones and phenanthridines, RSC Adv., 2014, 4, 40964–40968 RSC.
- Z. Z. Shi and F. Glorius, Synthesis of fluorenones via quaternary ammonium salt-promoted intramolecular dehydrogenative arylation of aldehydes, Chem. Sci., 2013, 4, 829–833 RSC.
- P. Wang, H. Rao, R. Hua and C.-J. Li, Rhodium-Catalyzed Xanthone Formation from 2-Aryloxybenzaldehydes via Cross-Dehydrogenative Coupling (CDC), Org. Lett., 2012, 14, 902–905 CrossRef CAS PubMed.
- S. Wertz, D. Leifert and A. Studer, Cross Dehydrogenative Coupling via Base-Promoted Homolytic Aromatic Substitution (BHAS): Synthesis of Fluorenones and Xanthones, Org. Lett., 2013, 15, 928–931 CrossRef CAS PubMed.
- S. K. Manna, S. L. K. Manda and G. Panda, [RuCl2(p-cymene)2]2 catalyzed cross dehydrogenative coupling (CDC) toward xanthone and fluorenone analogs through intramolecular C–H bond functionalization reaction, Tetrahedron Lett., 2014, 55, 5759–5763 CrossRef CAS.
- H. Bao, X. Hu, J. Zhang and Y. Liu, Cu(0)/Selectfluor system-catalyzed intramolecular Csp2-H/Csp2-H cross-dehydrogenative coupling (CDC), Tetrahedron, 2019, 75, 130533 CrossRef.
- J. Chen and F. R. Sheykhahmad, Intramolecular cross-dehydrogenative coupling of benzaldehyde derivatives: A novel and efficient route to benzocyclic ketones, J. Chin. Chem. Soc., 2020, 67, 638–645 CrossRef CAS.
- H. Rao, X. Ma, Q. Liu, Z. Li, S. Cao and C.-J. Li, Metal-Free Oxidative Coupling: Xanthone Formation via Direct Annulation of 2-Aryloxybenzaldehyde using Tetrabutylammonium Bromide as a Promoter in Aqueous Medium, Adv. Synth. Catal., 2013, 355, 2191–2196 CrossRef CAS.
- J. Tang, S. J. Zhao, Y. Y. Wei, Z. J. Quan and C. D. Huo, CBr4 promoted intramolecular aerobic oxidative dehydrogenative arylation of aldehydes: application in the synthesis of xanthones and fluorenones, Org. Biomol. Chem., 2017, 15, 1589–1592 RSC.
- H. Jiang, G. Mao, H. Wu, Q. An, M. Zuo, W. Guo, C. Xu, Z. Sun and W. Chu, Synthesis of dibenzocycloketones by acyl radical cyclization from aromatic carboxylic acids using methylene blue as a photocatalyst, Green Chem., 2019, 21, 5368–5373 RSC.
- H. F. Motiwala, R. H. Vekariya and J. Aubé, Intramolecular Friedel–Crafts Acylation Reaction Promoted by 1,1,1,3,3,3-Hexafluoro-2-propanol, Org. Lett., 2015, 17, 5484–5487 CrossRef CAS PubMed.
- N. Jiang, S.-Y. Li, S.-S. Xie, H. Yao, H. Sun, X.-B. Wang and L.-Y. Kong, FeCl3 and ether mediated direct intramolecular acylation of esters and their application in efficient preparation of xanthone and chromone derivatives, RSC Adv., 2014, 4, 63632–63641 RSC.
- S. Kruger and F. G. Mann, Xanthones and thioxanthones. Part VI. The preparation and properties of 9-thia-3-aza-anthrone, J. Chem. Soc., 1955, 2755–2763, 10.1039/JR9550002755.
- F. G. Mann and J. H. Turnbull, Xanthones and thioxanthones. Part III. The synthesis of 9-oxa-1-aza-anthrone (4-azaxanthone), J. Chem. Soc., 1951, 761–762, 10.1039/JR9510000761.
- J. Li, C. Jin and W. Su, Synthesis of 1-Azaxanthones and 1-Azathioxanthones by Yb(OTf)3/TfOH Co-Catalyzed Intramolecular Friedel–Crafts Reaction, Heterocycles, 2010, 81, 2555–2567 CrossRef CAS.
- O. Epstein, M. C. Bryan, A. C. Cheng, K. Derakhchan, T. A. Dineen, D. Hickman, Z. Hua, J. B. Human, C. Kreiman, I. E. Marx, M. M. Weiss, R. C. Wahl, P. H. Wen, D. A. Whittington, S. Wood, X. M. Zheng, R. T. Fremeau, R. D. White and V. F. Patel, Lead Optimization and Modulation of hERG Activity in a Series of Aminooxazoline Xanthene β-Site Amyloid Precursor Protein Cleaving Enzyme (BACE1) Inhibitors, J. Med. Chem., 2014, 57, 9796–9810 CrossRef CAS PubMed.
- H. M. T. Albuquerque, C. M. M. Santos, J. A. S. Cavaleiro and A. M. S. Silva, (E)-2-(4-Arylbut-1-en-3-yn-1-yl)chromones as Synthons for the Synthesis of Xanthone-1,2,3-triazole Dyads, Eur. J. Org. Chem., 2015, 2015, 4732–4743 CrossRef CAS.
- H. M. T. Albuquerque, C. M. M. Santos, J. A. S. Cavaleiro and A. M. S. Silva, First intramolecular Diels-Alder reactions using chromone derivatives: synthesis of chromeno 3,4-b xanthones and 2-(benzo c chromenyl)-chromones, New J. Chem., 2018, 42, 4251–4260 RSC.
- C. Proenca, H. M. T. Albuquerque, D. Ribeiro, M. Freitas, C. M. M. Santos, A. M. S. Silva and E. Fernandes, Novel chromone and xanthone derivatives: Synthesis and ROS/RNS scavenging activities, Eur. J. Med. Chem., 2016, 115, 381–392 CrossRef CAS PubMed.
- A. Bornadiego, J. Diaz and C. F. Marcos, Synthesis of 4-Aminoxanthones by an Uncatalyzed, Multicomponent Reaction, Adv. Synth. Catal., 2014, 356, 718–722 CrossRef CAS.
- A. Bornadiego, J. Diaz and C. F. Marcos, Regioselective Tandem 4+1 - 4+2 Synthesis of Amino-Substituted Dihydroxanthones and Xanthones, J. Org. Chem., 2015, 80, 6165–6172 CrossRef CAS PubMed.
- A. Bornadiego, J. Diaz and C. F. Marcos, Tandem synthesis of 4-aminoxanthones is controlled by a water-assisted tautomerization: A general straightforward reaction, Org. Biomol. Chem., 2019, 17, 1410–1422 RSC.
- M. Cheng, J. Yan, F. Hu, H. Chen and Y. Hu, Palladium-catalyzed cascade reactions of 3-iodochromones with aryl iodides and norbornadiene leading to annulated xanthones, Chem. Sci., 2013, 4, 526–530 RSC.
- C. Ghosh, D. K. SinhaRoy and K. K. Mukhopadhyay, Heterocyclic systems. Part 6. Reactions of 4-oxo-4H-[1]benzopyran-3-carbonitriles with hydrazine, phenylhydrazine, hydroxylamine, and some reactive methylene compounds, J. Chem. Soc., Perkin Trans. 1, 1979, 1964–1968, 10.1039/P19790001964.
- A. Nohara, T. Ishiguro, K. Ukawa, H. Sugihara, Y. Maki and Y. Sanno, Studies on Antianaphylactic Agents. 7. Synthesis of Antiallergic 5-Oxo-5H-[1]benzopyrano[2,3-b]pyridines, J. Med. Chem., 1985, 28, 559–568 CrossRef CAS PubMed.
- P. Langer and B. Appel, Efficient synthesis of benzopyrano[2,3-b]pyridines
by sequential reactions of 1,3-bis-silyl enol ethers with 3-cyanobenzopyrylium triflates, Tetrahedron Lett., 2003, 44, 5133–5135 CrossRef CAS.
- A. G. P. R. Figueiredo, A. C. Tomé, A. M. S. Silva and J. A. S. Cavaleiro, Reaction of chromone-3-carbaldehyde with α-amino acids—syntheses of 3- and 4-(2-hydroxybenzoyl)pyrroles, Tetrahedron, 2007, 63, 910–917 CrossRef CAS.
- N. Poomathi, P. T. Perumal and S. Ramakrishna, An efficient and eco-friendly synthesis of 2-pyridones and functionalized azaxanthone frameworks via indium triflate catalyzed domino reaction, Green Chem., 2017, 19, 2524–2529 RSC.
- Q. Yang, R. Wang, J. Han, C. C. Li, T. Wang, Y. Liang and Z. T. Zhang, Photo-induced tandem cyclization of 3-iodoflavones with electron rich five-membered heteroarenes, RSC Adv., 2017, 7, 43206–43211 RSC.
- Y. Liu, S. Jin, L. Huang and Y. Hu, Phase Transfer Reagent Promoted Tandem Ring-Opening and Ring-Closing Reactions of Unique 3-(1-Alkynyl) Chromones, Org. Lett., 2015, 17, 2134–2137 CrossRef CAS PubMed.
- S. A. French, M. R. Clark, R. J. Smith, T. Brind and B. C. Hawkins, Expedient synthesis of xanthones and multi-functionalized chromones from 1,1-diacyl cyclopropanes, Tetrahedron, 2018, 74, 5340–5350 CrossRef CAS.
- K. Goto, M. Terasawa and Y. Maruyama, Anti-Allergic Activities of a New Benzopyranopyridine Derivative Y-12,141 in Rats, Int. Arch. Allergy Appl. Immunol., 1979, 59, 13–19 CrossRef CAS PubMed.
- P. G. Dormer, K. K. Eng, R. N. Farr, G. R. Humphrey, J. C. McWilliams, P. J. Reider, J. W. Sager and R. P. Volante, Highly regioselective Friedlander annulations with unmodified ketones employing novel amine catalysts: syntheses of 2-substituted quinolines, 1,8-naphthyridines, and related heterocycles, J. Org. Chem., 2003, 68, 467–477 CrossRef CAS PubMed.
- H. Waldmann, G. V. Karunakar and K. Kumar, Gold(III)-Mediated Aldol Condensations Provide Efficient Access to Nitrogen Heterocycles, Org. Lett., 2008, 10, 2159–2162 CrossRef CAS PubMed.
- B. Duda, S. N. Tverdomed and G. V. Roschenthaler, CF2-containing acetylenephosphonates in heterocyclization reactions: the first synthesis of 2-difluoromethyl azaxanth-3-ylphosphonates, Org. Biomol. Chem., 2011, 9, 8228–8232 RSC.
- K. Otrubova, A. E. Fitzgerald and N. S. Mani, A novel entry to xanthones by an intramolecular Diels-Alder reaction involving 2-(1,2-dichlorovinyloxy) aryl dienones, Tetrahedron, 2018, 74, 5715–5724 CrossRef CAS.
- Y. He, S. H. Guo, X. S. Fan, C. H. Guo and X. Y. Zhang, One-pot synthesis of 12H-benzo b xanthen-12-ones and naphtha 2,3-b furans from non-naphthalene substrates, Tetrahedron Lett., 2014, 55, 4747–4752 CrossRef CAS.
- Z. L. Xiong, X. H. Zhang, Y. M. Li, X. S. Peng, J. Y. Fu, J. J. Guo, F. K. Xie, C. G. Jiang, B. Lin, Y. X. Liu and M. S. Cheng, Syntheses of 12H-benzo a xanthen-12-ones and benzo a acridin-12(7H)-ones through Au(I)-catalyzed Michael addition/6-endo-trig cyclization/aromatization cascade annulation, Org. Biomol. Chem., 2018, 16, 7361–7374 RSC.
- G. X. Z. Liu, C. Wu, B. F. Chen, R. He and C. Chen, Concise synthesis of xanthones by the tandem etherification-Acylation of diaryliodonium salts with salicylates, Chin. Chem. Lett., 2018, 29, 985–988 CrossRef CAS.
- X. C. Cheng, Y. Y. Zhou, F. F. Zhang, K. Zhu, Y. Y. Liu and Y. Z. Li, Base-Promoted Tandem Reaction Involving Insertion into Carbon-Carbon sigma-Bonds: Synthesis of Xanthone and Chromone Derivatives, Chem. – Eur. J., 2016, 22, 12655–12659 CrossRef CAS PubMed.
- Y. Fujimoto, R. Itakura, H. Hoshi, H. Yanai, Y. Ando, K. Suzuki and T. Matsumoto, Novel One-Pot Synthesis of Xanthones via Sequential Fluoride Ion-Promoted Fries-Type Rearrangement and Nucleophilic Aromatic Substitution, Synlett, 2013, 24, 2575–2580 CrossRef CAS.
- J. Kristensen, M. Begtrup and P. Vedsø, Preparation of 5-Acyl- and 5-Aryl-Substituted 1-(Benzyloxy)pyrazoles via Directed Ortho-Lithiation/Transmetalation and Palladium Catalyzed Cross-Coupling, Synthesis, 1998, 1604–1608 CrossRef CAS.
- J. Felding, P. Uhlmann, J. Kristensen, P. Vedsø and M. Begtrup, Palladium-Catalyzed Arylation and Acylation of 1-Benzyloxy-1,2,3-triazole Through a Directed Ortho Lithiation-Transmetalation Strategy, Synthesis, 1998, 1181–1184 CrossRef CAS.
- J. L. Kristensen, P. Vedsø and M. Begtrup, Synthesis of novel azaxanthones derived from N-hydroxyazoles, Tetrahedron, 2002, 58, 2397–2404 CrossRef CAS.
- G. Singh, R. Singh, N. K. Girdhar and M. P. S. Ishar, A versatile route to 2-alkyl-/aryl-amino-3-formyl- and hetero-annelated-chromones, through a facile nucleophilic substitution at C2 in 2-(N-methylanilino)-3-formylchromones, Tetrahedron, 2002, 58, 2471–2480 CrossRef CAS.
- F. Mann and J. Reid, Xanthones and thioxanthones. Part IV. The preparation and properties of 9-oxa-1-aza-anthrone and 9-thia-1-aza-anthrone, J. Chem. Soc., 1952, 2057–2062 RSC.
- G. Kolokythas, N. Pouli, P. Marakos, H. Pratsinis and D. Kletsas, Design, synthesis and antiproliferative activity of some new azapyranoxanthenone aminoderivatives, Eur. J. Med. Chem., 2006, 41, 71–79 CrossRef CAS.
- D. E. Daia, C. D. Gabbutt, B. M. Heron, J. D. Hepworth, M. B. Hursthouse and K. M. A. Malik, Conjugate additions of lithium dialkynylcuprates [(RC·C)2CuLi] to activated chromones. Unexpected formation of the 6H-bis[1]benzopyrano[2,3-b:3′,4′-e]pyridine system, Tetrahedron Lett., 2003, 44, 1461–1464 CrossRef CAS.
- Z. N. Siddiqui, One pot synthesis of new benzopyranopyridines via Friedlander condensation, Tetrahedron Lett., 2012, 53, 4974–4978 CrossRef CAS.
- P. Biswas, J. Ghosh, T. Sarkar, D. Jana and C. Bandyopadhyay, Design and synthesis of dichromeno[2,3-b;3′,2′-e]pyridine-12,14-dione to evaluate its optical properties, New J. Chem., 2017, 41, 3887–3893 RSC.
- C. K. Ghosh, K. Bhattacharya and C. Ghosh, Benzopyrans - XXXIII. [4+2]Cycloaddition of N,N-dimethylhydrazones and anils of 2-unsubstituted and 2-methyl-4-oxo-4H-1-benzopyran-3-carboxaldehyde with N-phenylmaleimide, Tetrahedron, 1994, 50, 4905–4912 CrossRef CAS.
- T. U. Kumar, D. Roy and A. Bhattacharya, Iron(III) catalyzed direct C–H functionalization at the C-3 position of chromone for the synthesis of fused chromeno-quinoline scaffolds, Tetrahedron Lett., 2019, 60, 1895–1898 CrossRef CAS.
- T. Schurreit, 4-Hydroxy-2H-[1]benzopyran-2-on als Baustein zur Synthese von Bisbenzopyranopyridinen, Arch. Pharm., 1987, 320, 500–506 CrossRef CAS.
- Y. Panneerselvam and B. S. R. Reddy, One pot synthesis of 1-azaxanthones via Friedlander condensation, Indian J. Chem., Sect. B: Org. Chem. Incl. Med. Chem., 2016, 55, 83–87 Search PubMed.
- C. P. Montgomery, D. Parker and L. Lamarque, Effective and efficient sensitisation of terbium luminescence at 355 nm with cell permeable pyrazoyl-1-azaxanthone macrocyclic complexes, Chem. Commun., 2007, 3841–3843, 10.1039/B709805G.
- R. Martín, L. B. Jiménez, M. Álvaro, J. C. Scaiano and H. García, Photoinduced Formation and Characterization of Electron–Hole Pairs in Azaxanthylium-Derivatized Short Single-Walled Carbon Nanotubes, Chem. – Eur. J., 2009, 15, 8751–8759 CrossRef PubMed.
- H.-J. Cho, M.-J. Jung, Y. Kwon and Y. Na, Oxiranylmethyloxy or thiiranylmethyloxy-azaxanthones and -acridone analogues as potential topoisomerase I inhibitors, Bioorg. Med. Chem. Lett., 2009, 19, 6766–6769 CrossRef CAS PubMed.
- T. Ghosh and C. Bandyopadhyay, Reactions of 2-Amino-4H-1-benzopyran-4-one with 4-Oxo-4H-1-benzopyran-3-carbaldehydes, J. Heterocycl. Chem., 2006, 43, 1431–1434 CrossRef CAS.
- D. Imperio, G. B. Giovenzana, G.-L. Law, D. Parker and J. W. Walton, Synthesis and comparative anion binding profiles of two di-aqua Eu(III) complexes, Dalton Trans., 2010, 39, 9897–9903 RSC.
- G.-L. Law, R. Pal, L. O. Palsson, D. Parker and K.-L. Wong, Responsive and reactive terbium complexes with an azaxanthone sensitiser
and one naphthyl group: applications in ratiometric oxygen sensing in vitro and in regioselective cell killing, Chem. Commun., 2009, 7321–7323, 10.1039/B920222F.
- H. Nouri, C. Cadiou, L. M. Lawson-Daku, A. Hauser, S. Chevreux, I. Dechamps-Olivier, F. Lachaud, R. Ternane, M. Trabelsi-Ayadi, F. Chuburu and G. Lemercier, A modified cyclen azaxanthone ligand as a new fluorescent probe for Zn2+, Dalton Trans., 2013, 42, 12157–12164 RSC.
- A. Rampa, A. Bisi, P. Valenti, M. Recanatini, A. Cavalli, V. Andrisano, V. Cavrini, L. Fin, A. Buriani and P. Giusti, Acetylcholinesterase Inhibitors: Synthesis and Structure−Activity Relationships of ω-[N-Methyl-N-(3-alkylcarbamoyloxyphenyl)- methyl]aminoalkoxyheteroaryl Derivatives, J. Med. Chem., 1998, 41, 3976–3986 CrossRef CAS PubMed.
- S. Gobbi, Q. Z. Hu, M. Negri, C. Zimmer, F. Belluti, A. Rampa, R. W. Hartmann and A. Bisi, Modulation of Cytochromes P450 with Xanthone-Based Molecules: From Aromatase to Aldosterone Synthase and Steroid 11 beta-Hydroxylase Inhibition, J. Med. Chem., 2013, 56, 1723–1729 CrossRef CAS.
- N. Szkaradek, A. Rapacz, K. Pytka, B. Filipek, D. Zelaszczyk, P. Szafranski, K. Sloczynska and H. Marona, Cardiovascular activity of the chiral xanthone derivatives, Bioorg. Med. Chem., 2015, 23, 6714–6724 CrossRef CAS PubMed.
-
N. Szkaradek, D. Sypniewski, A. M. Waszkielewicz, A. Gunia-Krzyzak, A. Galilejczyk, S. Galka, H. Marona and I. Bednarek, Synthesis and in vitro Evaluation of the Anticancer Potential of New Aminoalkanol Derivatives of Xanthone, Anti-Cancer Agents Med. Chem., 2016, 16, 1587–1604 Search PubMed.
- M. Kubacka, N. Szkaradek, S. Mogilski, K. Panczyk, A. Siwek, A. Grybos, B. Filipek, P. Zmudzki, H. Marona and A. M. Waszkielewicz, Design, synthesis and cardiovascular evaluation of some aminoisopropanoloxy derivatives of xanthone, Bioorg. Med. Chem., 2018, 26, 3773–3784 CrossRef CAS PubMed.
- N. Szkaradek, A. Rapacz, K. Pytka, B. Filipek, A. Siwek, M. Cegla and H. Marona, Synthesis and preliminary evaluation of pharmacological properties of some piperazine derivatives of xanthone, Bioorg. Med. Chem., 2013, 21, 514–522 CrossRef CAS PubMed.
- M. Franceschin, D. Nocioni, A. Biroccio, E. Micheli, S. Cacchione, C. Cingolani, A. Venditti, P. Zizza, A. Bianco and A. Altieri, Design and synthesis of a new dimeric xanthone derivative: enhancement of G-quadruplex selectivity and telomere damage, Org. Biomol. Chem., 2014, 12, 9572–9582 RSC.
- D. Zelaszczyk, M. Jakubczyk, K. Pytka, A. Rapacz, M. Walczak, P. Janiszewska, K. Panczyk, H. Marona, A. M. Waszkielewicz, P. Zmudzki and K. Sloczynska, Design, synthesis and evaluation of activity and pharmacokinetic profile of new derivatives of xanthone and piperazine in the central nervous system, Bioorg. Med. Chem. Lett., 2019, 29, 126679 CrossRef PubMed.
- S. Motavallizadeh, A. Fallah-Tafti, S. Maleki, A. N. Shirazi, M. Pordeli, M. Safavi, S. K. Ardestani, S. Asd, R. Tiwari, D. Oh, A. Shafiee, A. Foroumadi, K. Parang and T. Akbarzadeh, Synthesis and evaluation of antiproliferative activity of substituted N-(9-oxo-9H-xanthen-4-yl)benzenesulfonamides, Tetrahedron Lett., 2014, 55, 373–375 CrossRef CAS PubMed.
- X. Fei, M. Jo, B. Lee, S. B. Han, K. Lee, J. K. Jung, S. Y. Seo and Y. S. Kwak, Synthesis of xanthone derivatives based on alpha-mangostin and their biological evaluation for anti-cancer agents, Bioorg. Med. Chem. Lett., 2014, 24, 2062–2065 CrossRef CAS PubMed.
- D. Giallombardo, A. C. Nevin, W. Lewis, C. C. Nawrat, R. R. A. Kitson and C. J. Moody, Synthesis of toxyloxanthone B, Tetrahedron, 2014, 70, 1283–1288 CrossRef CAS.
- T. S. Ehianeta, S. Laval and B. Yu, Bio- and chemical syntheses of mangiferin and congeners, BioFactors, 2016, 42, 445–458 CrossRef CAS PubMed.
- X. Wei, D. L. Liang, M. H. Ning, Q. Wang, X. B. Meng and Z. J. Li, Semi-synthesis of neomangiferin from mangiferin, Tetrahedron Lett., 2014, 55, 3083–3086 CrossRef CAS.
- Y. Fujimoto, Y. Watabe, H. Yanai, T. Taguchi and T. Matsumoto, An Efficient Isoprenylation of Xanthones at the C1 Position by Utilizing Anion-Accelerated Aromatic Oxy-Cope Rearrangement, Synlett, 2016, 27, 848–853 CrossRef CAS.
- O. Talhi and A. M. S. Silva, Organic Synthesis of C-Prenylated Phenolic Compounds, Curr. Org. Chem., 2013, 17, 1067–1102 CrossRef CAS.
- R. S. Wang, R. D. Chen, J. H. Li, X. Liu, K. B. Xie, D. W. Chen, Y. Peng and J. G. Dai, Regiospecific Prenylation of Hydroxyxanthones by a Plant Flavonoid Prenyltransferase, J. Nat. Prod., 2016, 79, 2143–2147 CrossRef CAS PubMed.
- X. Li, Y. Wu, Y. Y. Wang, Q. D. You and X. J. Zhang, ‘Click Chemistry’ Synthesis of Novel Natural Product-Like Caged Xanthones Bearing a 1,2,3-Triazole Moiety with Improved Druglike Properties as Orally Active Antitumor Agents, Molecules, 2017, 22, 1834 CrossRef.
- G. A. Kraus, T. Guney, A. Kempema, J. M. Hyman and B. Parvin, Efficient synthesis of fluorescent rosamines: multifunctional platforms for cellular imaging, Tetrahedron Lett., 2014, 55, 1549–1551 CrossRef CAS.
- O. A. Akrawi, H. H. Mohammed, T. Patonay, A. Villinger and P. Langer, Synthesis of arylated xanthones by site-selective Suzuki-Miyaura reactions of the bis(triflate) of 1,3-dihydroxyxanthone, Tetrahedron, 2012, 68, 6298–6304 CrossRef CAS.
- M. M. Lakouraj, G. Rahpaima and S. M. Mohseni, Synthesis, characterization, and biological activities of organosoluble and thermally stable xanthone-based polyamides, J. Mater. Sci., 2013, 48, 2520–2529 CrossRef.
- V. Hasantabar, M. M. Lakouraj, E. N. Zare and M. Mohseni, Synthesis, Characterization, and Biological Properties of Novel Bioactive Poly(xanthoneamide-triazole-ethersulfone) and Its Multifunctional Nanocomposite with Polyaniline, Adv. Polym. Technol., 2017, 36, 309–319 CrossRef CAS.
- R. Shen, Y. Chen, Z. Li, H. Qi and Y. Wang, Synthesis and biological evaluation of disubstituted amidoxanthones as potential telomeric G-quadruplex DNA-binding and apoptosis-inducing agents, Bioorg. Med. Chem., 2016, 24, 619–626 CrossRef CAS PubMed.
- D. I. S. P. Resende, P. Pereira-Terra, J. Moreira, J. Freitas-Silva, A. Lemos, L. Gales, E. Pinto, E. Sousa, P. M. d. Costa and M. M. M. Pinto, Synthesis of a Small Library of Nature-Inspired Xanthones and Study of their Antimicrobial Activity, Molecules, 2020, 25, 2405 CrossRef CAS PubMed.
- A. M. Waszkielewicz, A. Gunia, N. Szkaradek, K. Pytka, A. Siwek, G. Satala, A. J. Bojarski, E. Szneler and H. Marona, Synthesis and evaluation of pharmacological properties of some new xanthone derivatives with piperazine moiety, Bioorg. Med. Chem. Lett., 2013, 23, 4419–4423 CrossRef CAS PubMed.
Footnote |
† These authors contributed equally to this work. |
|
This journal is © the Partner Organisations 2020 |
Click here to see how this site uses Cookies. View our privacy policy here.