DOI:
10.1039/C5QI00277J
(Research Article)
Inorg. Chem. Front., 2016,
3, 808-813
Isostructural compartmentalized spin-crossover coordination polymers for gas confinement†
Received
4th December 2015
, Accepted 22nd February 2016
First published on 14th March 2016
Abstract
Here we present two FeII coordination polymers that possess discrete compartments suitable for CO2 physisorption despite the lack of permanent channels. The two crystalline materials, of general formula [Fe(btzbp)3](X)2 (X = ClO4 or BF4), present voids of ca. 250 Å3, which each can accommodate up to two CO2 molecules. The abrupt spin transition can be modified upon CO2 sorption, and different magnetic behaviour is observed depending on the number of molecules sorbed.
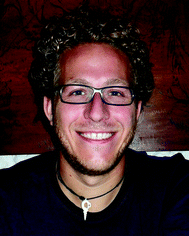 Guillermo Mínguez Espallargas | Guillermo Mínguez Espallargas graduated from the University of Seville (Spain) in 2004, and received his PhD in 2007 at the University of Sheffield (UK) under the supervision of Prof. Lee Brammer. In 2008 he moved to the Instituto de Ciencia Molecular (ICMol) of the University of Valencia (Spain), where he currently holds a “Ramón y Cajal” fellowship (selected in 1st position in the national ranking of the 2013 call in the Chemistry area). He is co-author of 52 papers, with an h-index of 21. He was the gold medal winner in the European Young Chemist Award in 2008, received the RSC Dalton Young Chemist Award in 2009, and was selected to attend the 63rd Lindau Nobel Laureate Meeting in 2013. His research interests cover MOFs, molecular magnetism, non-covalent interactions, and solid–gas reactions, among other topics. |
Introduction
Coordination polymers represent an important area of coordination chemistry with foremost applications in catalysis, magnetism, electronics and optics. In the last two decades the study of coordination polymers,1 which are formed by metal centres linked by organic ligands, has experienced a substantial progress and enormous research effort has been focused on the synthesis and study of novel structures with singular chemical and physical properties.2 Among these, particular interest has been drawn to those that present permanent channels and large voids, thus allowing the accommodation of guest molecules such as solvents or gases. These porous coordination polymers, also known as metal–organic frameworks (MOFs),3,4 are crystalline solids that allow the combination of porosity and other physical properties typical of dense coordination polymers, with applications as sensors,5 in magnetism,6 in the separation7 and storage8 of gases, or drug delivery,9 among others.
Another area of much interest within the field of coordination chemistry is the study of coordination cages.10 These discrete charged units, of many varied stoichiometries, present the same structural basis as coordination polymers, that is, the coordination of organic linkers and metal centers, albeit with the formation of soluble materials with an ample range of host–guest chemistry.
These two flourishing areas of coordination chemistry have been combined for the first time by Fujita and co-workers,11 who have transferred the host–guest chemistry of coordination cages into the crystalline state by synthesising a porous MOF composed of M6L4 octahedral molecular cages which has allowed the determination of molecular structures otherwise unachievable.
Very recently, we have exploited this concept of networked cages in the presence of latent porosity to develop unconventional crystalline solids capable of gas physisorption despite the absence of channels.12 The formation of compartmentalized coordination polymers (hereafter CCP) has resulted in high sorption selectivities towards different mixtures of gases.13 Furthermore, we have used as metallic centers Fe2+ ions able to undergo a thermal spin-crossover (SCO) transition. This feature has made these coordination polymers of interest in molecular magnetism since the confinement of the gas molecules in a restricted space has allowed switching the magnetic properties through gas sorption. These magnetic materials (CCP-1 and CCP-2) present infinite chains in which adjacent FeII centers are linked by three organic ligand forming discrete voids of 135 Å3 due to the syn-conformation of the ligands. Up to one molecule of CO2 can be located in each void at 1 bar and 298 K. Thus, CCP-1 and CCP-2 are coordination polymers that present features typical of coordination cages.
In this work we have prepared isostructural analogues to these coordination polymers by extending the length of the flexible organic ligand with the purpose of augmenting the gas sorption capacity. Thus, we have synthesized two FeII compartmentalized coordination polymers of formulae [Fe(btzbp)3](ClO4)2 (CCP-3) and [Fe(btzbp)](BF4)2 (CCP-4) (btzbp = 4,4′-bis((1H-tetrazol-1-yl)methyl)-1,1′-biphenyl) which present discrete voids of 257 Å3. Gas sorption measurements show that two molecules of CO2 can be loaded in each void at 1 bar and 298 K. In addition, both compounds present a spin transition centred at 195 and 199 K, respectively, similar to that observed in CCP-1 and CCP-2, although different magnetic behaviour has been observed depending on the number of CO2 molecules that are physisorbed.
Experimental section
Materials
All reagents and solvents were commercially available and used without further purification.
Synthesis of the ligand (btzbp).
The ligand 4,4′-bis((1H-tetrazol-1-yl)methyl)-1,1′-biphenyl (btzbp) was synthesized following a three-step synthesis:
4,4′-Bis(azidomethyl)-1,1′-biphenyl.
A mixture of sodium azide (1.0 g, 15 mmol) and 4,4′-bis(bromomethyl)-1,1′-biphenyl (3.4 g, 10 mmol) was dissolved in 15 mL of dry DMF and stirred at 60 °C for 10 hours. Then, 100 mL of water was added and the product was extracted with ether (3 × 10 mL). The organic layer was washed three times with water (3 × 10 mL). Yield 80%. IR (KBr disk) ν 2109.5 cm−1 (N3); 1H-NMR (300 MHz, DMSO): δ 4.5 (4 H, CH2–N3), 7.5 (4 H, CH aromatic), 7.8 (4 H, CH aromatic).
(1,1′-Biphenyl)-4,4′-diyldimethanamine.
4,4′-Bis(azidomethyl)-1,1′-biphenyl (1.5 g, 5.7 mmol) was dissolved in 25 mL of dry THF and added dropwise to a stirring solution of LiAlH4 (0.75 g) in 25 mL of dry THF cooled to 0 °C under an Ar atmosphere. When the addition was complete, the solution was heated to reflux with stirring for 4 h. Then, the excess of LiAlH4 was quenched by slowly adding 0.75 mL of water, followed by 0.75 mL of a 15% aqueous NaOH solution and finally 2.25 mL of water with the suspension maintained at 0 °C under constant stirring. The crude product was filtered and washed with ethyl acetate and dried under reduced pressure to yield the pure product. Yield 82%. IR (KBr disk) N3 band was not observed.
4,4′-Bis((1H-tetrazol-1-yl)methyl)-1,1′-biphenyl.
A mixture of (1,1′-biphenyl)-4,4′-diyldimethanamine (1.5 g, 7.1 mmol), sodium azide (0.92 g, 14.1 mmol) and triethyl orthoformate (12 mL, 69.3 mmol) was dissolved in 20 mL of acetic acid under an Ar atmosphere and heated at 90 °C for 4 days. After cooling to room temperature, the solvent was evaporated and the solid was washed with methanol to yield the pure product. Yield 74%. 1H-NMR (300 MHz, DMSO): δ 5.8 (4 H, CH2), 7.4 (4 H, CH aromatic), 7.7 (4 H, CH aromatic), 9.6 (2 H, CH tetrazole).
Synthesis of [Fe(btzbp)3](ClO4)2 (CCP-3).
A solution of Fe(ClO4)2·xH2O (28.0 mg) in 2 mL of MeCN was added into a solution of btzbp (63.6 mg, 0.2 mmol) in 24 mL of MeCN. A white crystalline precipitate appeared after a few days. The white powder was filtered and washed with MeCN. Phase purity was established by X-ray powder diffraction (vide infra). Yield 61%. Anal. calc. C48H42FeN24Cl2O8 (1209.75): C, 47.66; H, 3.50; N, 27.79%. Found: C, 47.50; H, 3.48; N, 26.94%.
Synthesis of [Fe(btzbp)3](BF4)2 (CCP-4).
A solution of Fe(BF4)2·xH2O (33.8 mg) in 2 mL of MeCN was added into a solution of btzbp (63.6 mg, 0.2 mmol) in 24 mL of MeCN. A white crystalline precipitate appeared after a few days. The white powder was filtered and washed with MeCN. Phase purity was established by X-ray powder diffraction (vide infra). Yield 70%. Anal. calc. C48H42FeN24B2F8 (1184.53): C, 48.67; H, 3.57; N, 28.38%. Found: C, 47.86; H, 3.54; N, 27.47%.
Single crystal diffraction
A single crystal of CCP-4 was mounted on a glass fibre using a viscous hydrocarbon oil to coat the crystal and then transferred directly to the cold nitrogen stream for data collection.‡ X-ray data were collected on the same crystal at 240 K (CCP-4-HS) and then at 120 K (CCP-4-LS) on a Supernova diffractometer equipped with a graphite-monochromated Enhance (Mo) X-ray source (λ = 0.71073 Å). The program CrysAlisPro, Oxford Diffraction Ltd, was used for unit cell determination and data reduction. Empirical absorption correction was performed using spherical harmonics, implemented in the SCALE3 ABSPACK scaling algorithm. Crystal structures were solved and refined against all F2 values using the SHELXTL suite of programs.14 Due to the tiny size of the crystal (and thus weak diffraction data), only the Fe atoms were refined anisotropically. The disordered anions were modelled over two orientations with fixed thermal parameters, with occupancies 50
:
50. Hydrogen atoms were placed in calculated positions that were refined using idealized geometries (riding model) and assigned fixed isotropic displacement parameters. CCDC-1440481 (CCP-4-HS) and -1440482 (CCP-4-LS) contain the supplementary crystallographic data for this paper. A summary of selected bond distances and angles is provided in Table S1,† and a summary of the data collection and structure refinements is provided in Table S3.†
X-ray powder diffraction
Polycrystalline samples of CCP-3 and CCP-4 were lightly ground in an agate mortar and pestle and filled into 0.5 mm borosilicate capillaries prior to being mounted and aligned on an Empyrean PANalytical powder diffractometer, using Cu Kα radiation (λ = 1.54056 Å). For each sample, two repeated measurements were collected at room temperature (2θ = 2–60°) and merged in a single diffractogram. Pawley refinements15 were performed using the TOPAS computer program16 and revealed an excellent fit to a one-phase model for compounds CCP-3 (Rwp = 0.0218; GOF = 1.502) and CCP-4 (Rwp = 0.0239; GOF = 1.225), indicating the absence of any other detectable crystalline phases.
Magnetic measurements
Magnetic susceptibility measurements were carried out on single-phased polycrystalline samples with a Quantum Design MPMS-XL-5 SQUID susceptometer. The susceptibility data were all collected at 1 K min−1, with an applied field of 0.1 T. Magnetic susceptibility measurements of the gas loaded systems were performed by sealing a glass tube with 10 mg of CCP-4 and a known amount of CO2, or after heating a loaded sample to remove the CO2 molecules. Prior to the gas loading, the samples were activated by heating in situ at 150 °C for 3 h under vacuum. In order to discard leak effects, the experiment was repeated twice obtaining the same results.
Gas sorption isotherms
High-pressure adsorption isotherms of CO2, CH4 and N2 were measured at different temperatures ranging from 283 to 333 K in an IGA-3 gravimetric analyser (Hiden Isochema) using approximately 50 mg of sample placed in the balance. Before each adsorption experiment, the sample was outgassed at 423 K under a final pressure of 10−5 Pa for four hours. The sample was then cooled down under high vacuum up to the target temperature that was controlled using a recirculating thermostatic bath. Adsorption measurements were performed by introducing the gas to build up the desired pressures of the isotherms. We have applied a virial equation for fitting experimental data points and it was found that a fourth grade polynomial is able to properly describe the CO2 isotherms. The heat of adsorption was calculated according to the Clausius–Clapeyron equation from the isotherms measured at different temperatures.
Surface deposition studies
Two different substrates were studied for this purpose: silicon and quartz substrates. The same procedure was carried out in the two cases. The substrates were washed by immersion in an acid piranha solution for three minutes and subsequent washing with water. Then, the clean substrates were introduced in a fresh solution containing both the ligand and the metal. After a few days the substrate was removed from the solution, washed with acetonitrile and dried over a nitrogen stream.
SEM studies were performed on a Hitachi S-4800 microscope operating at an accelerating voltage of 10 kV after metallization of the samples (Au–Pd).
Results and discussion
Structural characterization
We have previously reported12,13 the formation of two compartmentalized coordination polymers (CCP) which present thermal spin transition with the use of 1,4-bis(tetrazol-1-ylmethyl)benzene (hereafter btzx) as a ligand. With the aim of increasing the CO2 sorption capacity of these CCPs, we have designed an organic ligand that extends the intermetallic distance by adding an extra benzene ring in its skeleton. Thus, the ligand 4,4′-bis((1H-tetrazol-1-yl)methyl)-1,1′-biphenyl (hereafter btzbp, see Scheme 1) is 4.5 Å larger than the previously used btzx.
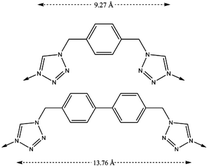 |
| Scheme 1 Chemical structures of the ligands btzx (top) and btzbp (bottom). The dashed arrows represent the distances between the two coordinating nitrogens. | |
The reaction of btzbp with two different FeII salts in refluxing MeCN for 4 h affords two coordination polymers, CCP-3 and CCP-4, with the general formula [Fe(btzbp)3](X)2 (X = ClO4 or BF4). By the use of microwave irradiation17 (82 °C), the synthesis time could be significantly reduced to 5 min. Nevertheless, a higher quality material was obtained from the reflux reaction. Structural analysis of CCP-4 in the high-spin (HS) and the low-spin (LS) states were determined using the same single crystal at 240 and 120 K, respectively. In both states, CCP-4 crystallizes in the space group P63, and is composed of [Fe(btzbp)3]2+ units that form one-dimensional chains that run parallel to the crystallographic c-axis with Fe⋯Fe distances of 16.1445 Å at 240 K (Fig. 1). The FeII centers are triply bridged by the btzbp organic ligands, in which the two phenyl rings are not coplanar, causing a reduction in symmetry from P63/m to P63 when compared with CCP-1 and CCP-2 that contain the shorter btzx ligand. The BF4− counterions are coplanar to the FeII atoms and separate the different chains, which are closely packed affording a framework with no permanent channels but with internal discrete voids of 257 Å3 at 240 K (235 Å3 at 120 K), which are practically double in size to those found in CCP-1 and CCP-2 (135 Å3). Each FeII center, which lies on the 3-fold axis, is octahedrally coordinated by six tetrazole nitrogen atoms from btzbp ligands. The Fe–N distances are 2.16(3) Å at 240 K while these are 2.01(3) Å at 120 K. The distances are in good agreement with Fe–N distances at HS and LS respectively in Fe-tetrazolate systems.18 The change in the length corresponds to the change in the spin of the FeII (lower Fe–N distances at LS), which is also accompanied by a colour change of the crystal from colorless (240 K) to pink (120 K).
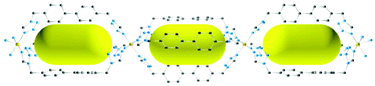 |
| Fig. 1 Crystal structure of CCP-4 viewed along the b-axis. The BF4− anions and hydrogen atoms have been removed for clarity. Key: Fe, orange; C, gray; N, blue. The yellow ellipsoids are placed in the structure to represent the empty space of the internal voids. | |
Sorption properties
Although the crystal structures of CCP-3 and CCP-4 present no permanent channels, we have recently reported13 the mechanism by which the analogues with shorter ligands, CCP-1 and CCP-2, can load different gases in their internal voids. Due to the structural similarity, we have explored the sorption capabilities of CCP-4.
Single-component isotherms have been applied to determine the loading capacities towards CO2, N2 and CH4 at 298 K. The isosteric heats of adsorption on CCP-2 and CCP-4 for CO2, shown in Fig. S4,† were calculated from the Clausius–Clapeyron equation using adsorption data collected at 283, 298, 313 and 333 K to quantify the adsorption affinity for CO2. The isosteric heat of adsorption for CO2 at zero coverage on CCP-4 was estimated to be 16.3 kJ mol−1, which is slightly lower than that found in CCP-2 (22.0 kJ mol−1).
As shown in Fig. 2, CCP-4 is able to adsorb two molecules of CO2 in each discrete compartment at 12 bar and 298 K, whereas a much lower content of N2 and CH4 is observed. By contrast, CCP-1 and CCP-2 have a CO2 maximum capacity of one molecule per void. Therefore, we have successfully increased the content in each void by chemical design. This has a direct impact on the working capacity,19 here defined as the difference of the uptake at 800 kPa minus the uptake at 50 kPa. As shown in Fig. 2b, the CO2 working capacity of CCP-4 is more than twice than that of CCP-2, due to the larger adsorption capacity as well as the lower isosteric heat of adsorption of CCP-4 compared to CCP-2. In addition, the use of a larger ligand does not penalize the high volumetric sorption observed in CCP-1 and CCP-2, as the dense nature of the material is maintained. At 1 bar and 298 K the system is able to store 29 mL of CO2 per mL of material, which is similar in value to the storage capacity of MIL-100 and greater than that presented by MOF-5, although lower than the storage-record of MOF-74-Mg (see Table 1). This is a consequence of the high density of compartmentalized coordination polymers, which contrasts with the situation encountered in the vast majority of MOFs, which have a low density value due to the presence of large channels in the structure.20
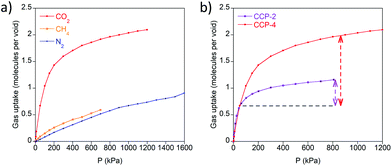 |
| Fig. 2 (a) Gas sorption isotherms at 298 K for CO2, CH4 and N2 for CCP-4 and (b) comparison between the capability of CCP-2 and CCP-4 in CO2 uptake. The double arrowed lines indicate the working capacities of each system. | |
Table 1 Volumetric CO2 capacity for different systems
Compound |
CO2 sorption (mL CO2 per mL MOF) |
Ref. |
CCP-4
|
29 |
This work |
CCP-1
|
29 |
13
|
CCP-2
|
27 |
13
|
MIL-100 |
28 |
21
|
MOF-5 |
11 |
22
|
MOF-74-Mg |
128 |
23
|
Magnetic properties
Magnetic susceptibility measurements performed on the polycrystalline samples of CCP-3 and CCP-4 show that both compounds present a spin transition centred at 195 and 199 K, respectively, similar to that observed in CCP-1 and CCP-2 (200 K), albeit no hysteresis is found. This behaviour can be ascribed to some loss in cooperativity due to the longer distance between each iron centre in the crystal. However, it is surprising to observe that the abruptness in the transition is maintained with metal centres separated by 16 Å. This is probably related to the polymeric structure of the materials, with low dimensionality, and the existence of a triple bridge between the iron centres. As can be observed in Fig. 3, the use of the larger ligand also causes an increase in the residual high-spin fraction, which is ca. 20%.
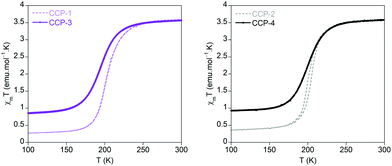 |
| Fig. 3 Temperature dependence of the product of the magnetic susceptibility and temperature (χmT) for CCP-3 (purple) and CCP-4 (black), compared with those of CCP-1 and CCP-2 (dashed lines). | |
We have previously studied two different approaches of modulating the spin transition of CCP-1 with chemical stimuli: (i) the inclusion of CO2 molecules shifts the transition temperature to a higher temperature;12 (ii) the use of ligand mixtures subtly modifies the transition temperature and also new transitions at lower temperatures are generated.24
In the present case the perturbation exerted by the physisorption of CO2 has been studied for CCP-4. Upon inclusion of one molecule of CO2 in each internal void, a shift of T1/2 from 199 K to 206 K has been observed (Fig. 4). Thus, physisorption of CO2 stabilizes the LS state, as was also observed in CCP-1 and CCP-2, due to the interaction between CO2 and the cationic framework. Interestingly, upon additional CO2 loading (two molecules in each void), no further increase of T1/2 is observed. On the contrary, a reduction of T1/2 occurs, reaching 202 K. This behaviour could be caused by gas–gas interaction inside the void of the compartmentalized coordination polymer. The two CO2 molecules interact with each other, thus decreasing the interaction between the gas and the framework, and therefore reducing the effects in T1/2.
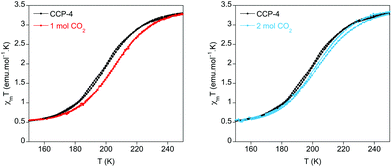 |
| Fig. 4 Temperature dependence product of the magnetic susceptibility and temperature (χmT) for CCP-4 loaded with one (red line) and two (blue line) molecules of CO2 in each void. | |
Surface deposition
Surface deposition studies have been carried out as preliminary tests in order to examine the possible preparation of membranes for CO2 capture or selective separation of gases.25 For this purpose, we have analysed the deposition of CCP-4 on two different substrates, quartz and silicon, by drop casting and we concluded that the choice of substrate plays an important role in the result. The deposition is not complete on a quartz substrate, with only some areas covered. However, on these regions we were able to find some hexagonal microcrystals of the system (Fig. 5a). On the other hand, when using a silicon substrate, a complete coverage is achieved, with each silicon substrate covered by a layer of 400 μm of micrometric particles with irregular shapes (Fig. 5b). Confirmation of the formation of CCP-4 on top of the substrate was achieved with grazing incidence X-ray diffraction (GIXRD). As shown in Fig. 6, the same diffractogram is obtained for the film and the polycrystalline powder, thus demonstrating the formation and deposition of the compartmentalized coordination polymer. Additionally, the magnetic behaviour of the deposited material shows that the spin transition is maintained (Fig. S8†).
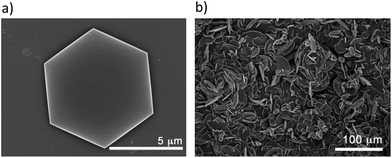 |
| Fig. 5 (a) Hexagonal microcrystals of CCP-4 on a quartz substrate; (b) irregular micrometric particles of CCP-4 covering all the silicon substrate. | |
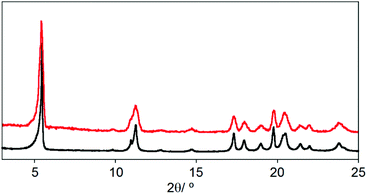 |
| Fig. 6 XRPD of polycrystalline powder of CCP-4 (black line) and GIXRD of the film for the same material (red line). | |
Conclusions
In this work we have prepared two compartmentalized coordination polymers with the general formula [Fe(btzbp)3](X)2 (X = ClO4 or BF4), which can be of interest in two different areas: MOFs and molecular magnetism. In the former area we have shown that these crystalline solids possess internal cavities that have been proved to be suitable for accommodating up to two molecules of CO2 each. In molecular magnetism we have shown that these polymers are examples of multifunctional magnetic materials responding to a chemical stimulus. In fact, they present spin-crossover phenomena which can be tuned by sorption of CO2. Thus, an increase in the transition temperature at low loadings from T1/2 = 199 K to T1/2 = 206 K has been observed as a result of the gas–framework interaction. Upon further loading, the presence of gas–gas interactions reduces the effects on the framework causing a reduction in the shift of the transition temperature, being T1/2 = 202 K. Finally, the formation of thin films opens the possibility to easily process these materials in the form of membranes to be used in gas separation processes.
Acknowledgements
Financial support from the Spanish MINECO (CTQ2014-59209-P, MAT2014-56143-R and MAT2012-38567-C02-01), the Generalitat Valenciana (Prometeo and ISIC-Nano programs) and the VLC/Campus Program is gratefully acknowledged. We thank the Spanish government for the provision of a Severo Ochoa project (SEV-2012-0267) and a María de Maeztu project (MDM-2015-0538). G.M.E. acknowledges the Blaise Pascal International Chair for financial support. M.G.-M. thanks MICINN for a predoctoral FPU grant and the EU for a Marie Sklodowska-Curie postdoctoral fellowship (H2020-MSCA-IF-EF-658224). N.C.G. thanks the Generalitat Valenciana for a Val-i+d predoctoral fellowship. J. M. Martínez-Agudo and G. Agustí from the University of Valencia are gratefully acknowledged for magnetic measurements.
Notes and references
-
(a) B. F. Hoskins and R. J. Robson, J. Am. Chem. Soc., 1989, 111, 5962 CrossRef CAS;
(b) B. F. Hoskins and R. J. Robson, J. Am. Chem. Soc., 1990, 112, 1546 CrossRef CAS.
- E. Coronado, M. Giménez-Marqués, G. Mínguez Espallargas and L. Brammer, Nat. Commun., 2012, 3, 828 CrossRef PubMed.
- See special issues on MOFs: Chem. Rev., 2012, 112, 673; Chem. Soc. Rev., 2014, 43, 5415.
-
(a) H. Furukawa, K. E. Cordova, M. O'Keeffe and O. M. Yaghi, Science, 2013, 341, 6149 CrossRef PubMed;
(b) A. G. Slater and A. I. Cooper, Science, 2015, 348, 6238 CrossRef PubMed.
- Z. Hu, B. J. Deibert and J. Li, Chem. Soc. Rev., 2014, 43, 5815 RSC.
- E. Coronado and G. Mínguez Espallargas, Chem. Soc. Rev., 2013, 42, 1525 RSC.
- J.-R. Li, R. J. Kuppler and H.-C. Zhou, Chem. Soc. Rev., 2009, 38, 1477 RSC.
- L. J. Murray, M. Dincă and J. R. Long, Chem. Soc. Rev., 2009, 38, 1294 RSC.
- M. Giménez-Marqués, T. Hidalgo, C. Serre and P. Horcajada, Coord. Chem. Rev., 2016, 307, 342 CrossRef.
-
(a) M. J. Smulders, I. A. Riddell, C. Browne and J. R. Nitschke, Chem. Soc. Rev., 2013, 42, 1728 RSC;
(b) T. R. Cook and P. J. Stang, Chem. Rev., 2015, 115, 7001 CrossRef CAS PubMed.
-
(a) Y. Inokuma, S. Yoshioka, J. Ariyoshi, T. Arai, Y. Hitora, K. Takada, S. Matsunaga, K. Rissanen and M. Fujita, Nature, 2013, 495, 461 CrossRef CAS PubMed;
(b) S. Yoshioka, Y. Inokuma, M. Hoshino, T. Sato and M. Fujita, Chem. Sci., 2015, 6, 3765 RSC.
- E. Coronado, M. Giménez-Marqués, G. Mínguez Espallargas, F. Rey and I. J. Vitórica-Yrezábal, J. Am. Chem. Soc., 2013, 135, 15986 CrossRef CAS PubMed.
-
M. Giménez-Marqués, N. Calvo Galve, M. Palomino, S. Valencia, F. Rey, G. Sastre, I. J. Vitórica-Yrezábal, M. Jiménez-Ruiz, J. A. Rodríguez-Velamazán, M. A. González, E. Coronado and G. Mínguez Espallargas, submitted.
- G. M. Sheldrick, Acta Crystallogr., Sect. A: Found. Crystallogr., 2008, 64, 112 CrossRef CAS PubMed.
- G. S. Pawley, J. Appl. Crystallogr., 1981, 14, 357 CrossRef CAS.
-
A. A. Coelho, TOPAS-Academic, Version 4.1, 2007, see http://www.topas-academic.net Search PubMed.
- J. Klinowski, F. A. Almeida Paz, P. Silva and J. Rocha, Dalton Trans., 2011, 40, 321 RSC.
- G. Aromí, L. A. Barrios, O. Roubeau and P. Gamez, Coord. Chem. Rev., 2011, 255, 485 CrossRef.
- M. Palomino, A. Corma, F. Rey and S. Valencia, Langmuir, 2010, 26, 1910 CrossRef CAS PubMed.
- R. E. Morris and P. S. Wheatley, Angew. Chem., Int. Ed., 2008, 47, 4966 CrossRef CAS PubMed.
- J. A. Mason, T. M. McDonald, T. Bae, J. E. Bachman, K. Sumida, J. J. Dutton, S. S. Kaye and J. R. Long, J. Am. Chem. Soc., 2015, 137, 4787 CrossRef CAS PubMed.
- A. Ö. Yazaydin, R. Q. Snurr, T.-H. Park, K. Koh, J. Liu, M. D. LeVan, A. I. Benin, P. Jakubczak, M. Lanuza, D. B. Galloway, J. L. Low and R. R. Willis, J. Am. Chem. Soc., 2009, 131, 18198 CrossRef CAS PubMed.
- S. R. Caskey, A. G. Wong-Foy and A. J. Matzger, J. Am. Chem. Soc., 2008, 130, 10870 CrossRef CAS PubMed.
- N. Calvo Galve, E. Coronado, M. Giménez-Marqués and G. Mínguez Espallargas, Inorg. Chem., 2014, 53, 4482 CrossRef CAS PubMed.
- Z. Xiang and D. Cao, J. Mater. Chem. A, 2013, 1, 2691 CAS.
Footnotes |
† Electronic supplementary information (ESI) available: Additional figures and crystallographic data. CCDC 1440481 and 1440482. For ESI and crystallographic data in CIF or other electronic format see DOI: 10.1039/c5qi00277j |
‡ Crystal data for CCP-4. [Fe(C16H14N8)3](BF4)2. Space group P63, Z = 2, M = 1184.53. HS: T = 240(2) K, a = b = 10.3650(10) Å, c = 32.289(5) Å, V = 3004.2(6) Å3. R1 = 0.2271 and wR2 = 0.5406. LS: T = 120(2) K, a = b = 10.2235(14) Å, c = 31.903(8) Å, V = 2887.8(11) Å3. R1 = 0.2157 and wR2 = 0.5271. |
|
This journal is © the Partner Organisations 2016 |
Click here to see how this site uses Cookies. View our privacy policy here.