Biofilms in shower hoses – choice of pipe material influences bacterial growth and communities†
Received
15th January 2016
, Accepted 18th February 2016
First published on 19th February 2016
Abstract
Flexible polymeric pipe materials are commonly used as shower hoses or connections to faucets in the last meters of building plumbing, but these tend to leach high concentrations of carbon that encourage bacterial growth. Here we compared the microbiological impact of six such materials, with both a short-term material comparison test and a daily shower simulator operating for eight months. The materials ranked differently in the migration potential and biomass formation potential assays of the comparison test, but overall these results correlated (R2 > 0.77) with long-term biofilm development in the shower simulator. The biofilm concentration after eight months ranged from 2 × 106 cells per cm2 on the control material (PE-Xc) to 2 × 108 cells per cm2 on a typical shower hose (PVC-P). However, the differences in four of the six materials were much less pronounced after eight months than in the early months. The communities were characterized with 16S rRNA amplicon sequencing clustered with both material and time (R2 = 0.31; R2 = 0.25), and correlated strongly with the biofilm concentration (R2 > 0.74). A universal core consisting of 7 genera accounted for 44% of all sequences, and accounted for more of young and high-biomass biofilms. Genera containing opportunistic pathogens were more common in low-biomass pipes. We conclude that choice of materials is not only critical for determining biofilm concentration, but also community composition. Our results show that a seemingly small choice in plumbing material in the final meters of distribution can make a considerable difference in the building plumbing ‘exposome’.
Water impact
Directly before consumers are exposed to shower water, it passes through a final meter of flexible pipe (i.e., the shower hose). We evaluated several hoses and found dramatic differences in biofilm quantity and composition. Since shower water often stagnates in these hoses for more than 24 hours, this small choice in building plumbing drastically shapes microbial exposure in homes.
|
1. Introduction
Flexible materials are commonly used in building plumbing due to their versatility. The final connections to various faucets and appliances (e.g., showerhead or extendable kitchen faucet) are often flexible hoses made from synthetic polymers, rubber, or silicone. Many of these materials leach biodegradable organic carbon in concentrations that essentially nullify carbon reduction efforts in drinking water treatment,1–3 resulting in biologically unstable water with increased planktonic and biofilm concentrations.4 Flexible materials are not properly considered under water quality regulations, despite the fact that there is clear evidence that flexible materials result in dramatically more biofilm formation than hard plastics, stainless steel, or copper pipes.1,5 Moreover, these final connections are coming under increased scrutiny for biofilm development due to concerns over the establishment and proliferation of opportunistic pathogens,6 and the potential exposure of consumers.7 Thus, it is essential that the quality of these final connection materials in the last meter of drinking water distribution be critically assessed for their contribution to biological growth.
The importance of material choice with respect to microbiology in drinking water applications is typically considered only on broad scales, e.g., where metals are compared with plastics and/or cement.5,8–11 These studies demonstrated large-scale differences in nutrient leaching behaviors that contribute to considerable differences in biofilm concentrations and composition. However, within the finer scales of one category such as flexible plastics, there can still be considerable differences in material quality that need to be explored and understood thoroughly.12 As government regulations slowly begin to consider the biological impact of pipe materials,13 industry together with researchers has developed standardized short-term tests that are useful in developing new materials.1,12 While these tests are useful for material evaluation and comparison, it is unknown how well they represent the materials' behavior in practical use. Materials tend to change their quality with age as leaching diminishes and biofilm is established.14,15 In addition, realistic use of materials in buildings involves temperature and stagnation selection factors that are not necessarily reproduced in short-term tests or in reactors designed to model distribution systems. Many of these selective factors are favorable for microbial growth and likely dictate development of a specific community that could even encourage opportunistic pathogen proliferation.6
In the present study, biofilm development was investigated in six plastic pipe materials, commercially advertised for use as potable water or shower hoses. We used a slew of methods to quantify and characterize the microbial communities that developed during (1) material comparison tests and (2) an 8-month daily shower simulation. The novelty of this work is the specific focus on flexible material and the comparison between data from material tests and actual biofilm growth during use. Our results show that a seemingly small choice in plumbing material in the final meters of distribution can make a considerable difference in the building plumbing ‘exposome’.
2. Materials and methods
2.1. Materials tested
Six commercially available pipe materials with widely varying qualities were chosen for comparison (Table 1). Material A was a hard plastic pipe specifically approved for drinking water use in Europe, and was used as a control against the five flexible pipes. Material B was a flexible pipe, also approved for drinking water, typically used to connect taps (e.g., kitchen faucets) to the main water supply of a house. Material C was a silicone shower hose that meets German drinking water material standards, and Material D was a shower hose with a silver-ion antibacterial coating. Materials E and F were typical shower hoses with a 4-fold price difference. Where possible, information on the material type and legislative certifications is provided in Table 1.
Table 1 Overview of the six materials chosen for comparison
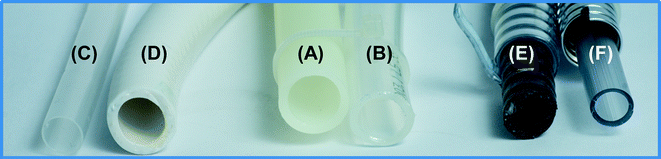
|
|
Type |
Category |
Material |
Additional details |
Approval by DVGW (German Technical and Scientific Association for Gas and Water) and SVGW (Swiss Technical Association for Gas and Water) are identical and require passing the KTW guideline for migration16 and the W270 guideline for microbial enhancement.13 Due to purchase in Switzerland or Germany, only these certifications are listed. The material may concurrently pass guidelines and standards from other countries (e.g. BPP[NL], BS 6920 [UK]).
Cross-linking method unknown.
|
A |
Hard |
Drinking water pipe |
PE-Xc |
Approved by DVGW & SVGWa |
B |
Flexible |
Drinking water hose |
PE-Xb |
Approved by DVGW & SVGWa |
C |
Flexible |
Shower hose |
Silicone |
Approved by DVGWa |
D |
Flexible |
Shower hose |
Unknown |
Coated with patented inorganic silver-ion coating |
E |
Flexible |
Shower hose |
PVC-P |
With ash addition; expensive hose |
F |
Flexible |
Shower hose |
PVC-P |
Cheap hose compared to E |
2.2. BioMig material test package
The BioMig test package,12 which was designed to quantify the organic carbon migration potential and biological impact of materials in contact with drinking water, was used on all six materials. Briefly, the test package consists of two assays, namely a migration potential assay and a biomass formation potential (BFP) assay. The migration potential assay involves seven consecutive 24 hour incubation cycles at 60 °C and measures the total organic carbon (TOC) and the growth potential of bacteria on the TOC that leached from the materials on days 1, 3, and 7. Growth potential of the leachate was measured in an assay with an inoculated drinking water microbial community and incubation for 6 days at 30 °C with shaking at 120 rpm.17 The BFP assay quantifies both biofilm and planktonic growth with flow cytometry after 14 days of incubation of the test material together with a drinking water microbial community at 30 °C with shaking. Both assays use a surface-to-volume ratio of 1 cm−1, and bottled mineral water (Evian, France) serves as test water and inoculum. In the growth potential assays, a minimal medium mix with phosphate (10 mg L−1), nitrogen (12 mg L−1), and iron (0.3 mg L−1) is added to ensure that carbon is the only limiting nutrient.
2.3. Realistic building plumbing simulator (BPS)
A realistic building plumbing simulator (BPS) was constructed to simulate conditions of discontinuous warm water flow that drinking water and shower hoses might experience in a typical system (e.g. daily shower use) (Fig. 1). Each pipe material (length 1.5 m, diameter 7–11 mm) was connected in triplicate to a building's hot water system where the maximum temperature was 42 °C, and which was flushed once daily for 15 minutes at a flow rate of 1 L min−1 with the use of a magnetic valve on a timer. While this flow rate was considerably lower than in a real shower, it allowed use of the water pressure directly from the same tap. Between flushing cycles, the BPS was exposed to ambient room temperature (18–23 °C). All connections between the tap and the BPS were made with a similar length of a polyurethane flexible pipe suited for use in the food industry and associated X-splitters (Festo, Esslingen am Neckar, Germany). Ball valves (Festo, Esslingen am Neckar, Germany) placed at the beginning of the test lines were used to control flow and water pressure to be approximately equal between all 18 lines. Water used for flushing was non-chlorinated drinking water (Dübendorf, Switzerland). The water is characterized in detail in Table S1.†
 |
| Fig. 1 Schematic of the building plumbing simulator (BPS). The system was connected to an ordinary hot water tap in a laboratory building. A magnetic valve controlled flow, which was split between test materials using X-splitters and 8 mm food-industry quality hoses. The 18 test material hoses were connected in triplicate, with ball valves to control flow in individual hoses. | |
2.4. Biofilm sampling and detachment
After one, two and eight months of operation, biofilm samples were collected by cutting 30 cm from the distal end of each pipe. The 30 cm length was chosen in order to achieve enough total biofilm suspension volume for analysis and sufficiently capture the heterogeneity of the biofilms, while considering the limitation of total length of pipe. Of the 30 cm cut at each sampling event, 28 cm was used for biofilm removal and quantification via repeated sonication. Each end of each pipe was plugged using a stopper specific to the pipe material. The pipe segment was filled with sterile glass beads (3 mm diameter) and 0.2 μm filtered bottled mineral water (Evian, France). After this stabilization step, samples were stored at 4 °C for a maximum of 3 days before detachment and analysis. For biofilm detachment, the pipe segment with stoppers and glass beads was sonicated by submersion in a sonication bath (Bandelin Sonorex, Rangendingen, Germany) for 5 minutes, and the biofilm suspension (without the glass beads) was removed and collected. This sonication procedure was repeated 5 times with freshly filtered bottled mineral water added each time. After the fifth sonication, the glass beads were discarded and the pipes were filled with filtered bottled mineral water and inverted 30 times. This final rinse water was added to the biofilm suspension. The entire biofilm suspension (32–150 mL) was sonicated with a Bandelin Sonorex device (Sonopuls HD 2200, Bandelin Sonorex, Rangendingen, Germany) with a needle at 40% intensity with 50% power for 30 seconds. In the eighth month, a biofilm extraction blank consisting of the filtered water with needle sonication was processed alongside the biofilm samples. The biofilm suspension was then subjected to quantification by the means described below. Blanks were not included for each material, and thus initial colonization on the materials may contribute to inter- and intra-hose variation.
2.5. Fluorescence staining and flow cytometry of water samples and biofilm suspensions
Staining and flow cytometric analysis were done as is described previously.18–20 In short, for a working solution, SYBR® Green I (SG) (Invitrogen AG, Basel, Switzerland) was diluted 100× in anhydrous dimethylsulfoxide (DMSO). For measurement of intact cells, propidium iodide (PI; 30 mM) was mixed with the SYBR® Green I working solution to a final PI concentration of 0.3 mM. These working solutions were stored at −20 °C until use. From every water sample, 500 μL was stained with SG or SGPI at 10 μL mL−1. From every biofilm suspension, 200 μL was stained with SG or SGPI at 10 μL mL−1. Samples were preheated to 35 °C (3 min), then incubated with stain in the dark for 10 min at 35 °C before measurement. Flow cytometric measurements were performed, as is described previously,21 using a BD Accuri C6® flow cytometer (BD Accuri cytometers, Belgium) equipped with a 50 mW laser emitting at a fixed wavelength of 488 nm. The flow cytometer is equipped with volumetric counting hardware, calibrated to measure the number of particles in 50 μL of a 500 μL sample; or 100 μL of a 200 μL sample. Measurements were performed at a pre-set flow rate of 66 μL min−1. A threshold value of 800 a.u. was applied on the green fluorescence channel (FL1). Data analysis was performed using the BD Accuri CFlow® software, following the procedure described previously.20 Briefly, bacterial signal was selected using electronic gating on the density plots of green fluorescence (FL1; 533 nm) and red fluorescence (FL3; > 670 nm). The total cell concentration (TCC) was calculated from the results from the SG stain, and the intact cell concentration (ICC) was calculated from the results from the SGPI stain.
2.6. Adenosine tri-phosphate (ATP) analysis for biofilm suspensions
Total ATP was determined using the BacTiter-Glo™ reagent (Promega Corporation, Madison, WI, USA) and a luminometer (Glomax, Turner Biosystems, Sunnyvale, CA, USA) as is described elsewhere.22 A biofilm suspension sample (100 μL) and the ATP reagent (100 μL) were warmed to 38 °C simultaneously in separate sterile Eppendorf tubes. The sample and the reagent were combined and then luminescence was measured after a 20 second reaction time at 38 °C. The data were collected as relative light units (RLU) and converted to ATP (nM) by means of a calibration curve made with a known ATP standard (Promega). ATP was measured in triplicate for all samples, and the relative standard deviation among technical replicates was always below 4%.
2.7. Total organic carbon (TOC)
TOC concentration was determined by thermal oxidation to CO2 and infrared detection with the non-purgeable organic carbon method according to EN 1484 (TOC-VCPH, Shimadzu, Kyoto, Japan). All samples were diluted tenfold with a purified water with TOC < 5 μg mL−1 organic carbon.
2.8. DNA extraction
After analysis, the remaining volume of biofilm suspension or water sample was filtered using sterile techniques on a 0.22 μm polycarbonate Nucleopore® membrane filter (47 mm diameter, Whatman, Kent, UK). The filter was inserted into a 5 mL tube and stored at −20 °C before DNA extraction with the Power Water DNA Isolation Kit (MoBio Laboratories, Inc., Carlsbad, CA) according to manufacturers' instructions. The sample processing strategy of filtering the biofilm suspension, which was treated as a liquid sample throughout, leant itself to use of the Power Water DNA Isolation Kit.
2.9. 16S qPCR
qPCR reactions were performed on a LightCycler 480-II (Roche) and analyzed using the LightCycler 480 ver. 1.5.1 software (Roche). Quantification of bacterial 16S rRNA genes was carried out using the primers Bact349F/Bact806R and probe Bac516F23 (ESI,† Table S2). Reaction conditions are detailed in Table S3,† and the reactions were performed using a LightCycler 480 Probes Master hot start reaction mix (Roche), each primer at a concentration of 0.9 μmol L−1, and the probe at 0.3 μmol L−1. The results were evaluated using the point-fit method for absolute quantification.
The total reaction volumes were 10 μl with a sample volume of 2 μL. DNA extracts with DNA concentrations ranging from 0.1 to 106 μg mL−1 were diluted 10- and 100-fold in AE buffer (Qiagen) and measured in triplicate. 100-fold dilution results were used, except where results were below the limit of quantification and 10-fold dilution results were used.
Quantification standards were obtained from a suitable PCR product cloned into a pGEM T-Easy vector (Promega), purified with a Qiaprep Spin Miniprep Kit (Qiagen), quantified using the Qubit System (Thermo Fisher Scientific) and diluted in 10-fold serial dilutions in AE buffer to concentrations from 500 million to 50 copies per reaction. Standards were measured in quadruplicate. High linearity (R2 = 0.998) in standards was observed. There were no signs of inhibition when a standard was spiked into a sample (data not shown).
Limit of detection was determined by the quantification of the biofilm extraction blank that consisted of filtered water processed alongside biofilm suspensions. DNA was extracted from this blank and it was diluted the same as the other samples. Limit of quantification was defined as the most dilute standard detected with standard deviation of Cp (crossing point) <0.5.
2.10. Amplicon sequencing with Illumina MiSeq
Approximately 1 ng of DNA extract from each sample was subjected to PCR amplification using modified universal bacterial primers Bakt_341F and Bakt_805R,24 which target the V3–V5 region of the 16S rRNA gene, and were adapted with a tail to facilitate binding of Nextera adapters (ESI,† Table S2). Index PCR was performed to add the Nextera XT v2 Index Kit adapters (Illumina) to the amplicon. PCR reaction conditions are detailed in Table S3.† After each PCR reaction, products were purified using the Agencort® AMPure® XP system (Beckman Coulter, Inc., Bera, CA).
Each product was quantified using the Kapa Library Quantification Kit performed on a 7500 Fast Real-Time PCR System (Applied Biosystem). Using these concentrations, the samples were normalized before subjected to running on the MiSeq platform using a MiSeq Reagent Kit v2 (300-cycles, #MS-102-2002) according to manufacturer's protocol. Due to low quality in the first run, a second run, with 50% PhiX instead of 5%, was run to improve quality. All sequencing was done at the Genetic Diversity Centre (GDC) of ETH, Zurich.
2.11. Statistics
To compare quantification of biofilm from the realistic BPS, all measurements of detached biofilm suspensions were normalized to the pipe surface area from which the biofilm was removed. These biofilm surface-normalized concentrations are referred to as biofilm concentrations throughout the text. In the case of TCC, ATP and 16S gene copies, concentrations were then log transformed. All subsequent statistical tests were performed in R (v 3.2.3)25 with an alpha of 0.05. A Shapiro–Wilk test was performed and all measures except log (TCC) were found to be not normally distributed, and thus non-parametric methods were used to compare the means of these measures. T-tests and Tukey's HSD were used for TCC, and non-parametric Kruskal–Wallis26 tests were used for all other measures. Spearman's rank correlation coefficients were used to compare biofilm concentration metrics.
2.12. Sequencing statistics
The libraries from the two runs were subject to stringent quality control, and these steps, trimming methods, and merging steps are detailed in Table S4.† The two runs were not significantly different (p = 1.0, Adonis) and were thus merged for subsequent analysis in order to increase the total number of reads. The UPARSE workflow was used to cluster the reads into OTUs and create count tables (Table S4†). A total of 5.6 million (95%) amplicons (e.g. merged and quality filtered reads) from 57 samples were mapped to 828 OTUs. Usearch-UTAX classifier was used to assign taxonomy for the OTUs based on the green-genes database version 13/5.27 All samples were subsequently scaled to the minimum depth of 14
880 sequences per sample.
In R, Phyloseq28 (v 1.14.0) was used for community analysis. Non-metric multi-dimensional scaling (NMDS) using Bray–Curtis dissimilarity was performed for visualization of results. From the vegan package, Adonis (non-parametric permutation, MANOVA) tests were used to fit experimental factors and the Envfit function was used to fit vectors for numerical variables to the NMDS ordination.29
2.13. Measuring realistic stagnation scenarios
In order to determine what stagnation scenario is “realistic” for the building plumbing elements tested in this study, 7 households recorded their water usage for one week. Focus was placed on the kitchen faucet and the shower hose, two locations where flexible hosing is almost always used in Swiss households. Households varied from 2–5 persons per household. Participants were asked to mark the half-hour period in which the tap was opened. For maximum stagnation time, it was also assumed that any marked use could be at the beginning or the end of the half hour period. To calculate total stagnation time, 2 minutes of use per kitchen faucet opening, and 15 minutes of use per shower were used.
3. Results and discussion
Six different water pipe materials (Table 1) were compared in short-term material tests and a long-term building plumbing simulator (Fig. 1). Migration and growth potential assays showed clear material differences (Fig. 2 and 3) and largely predicted growth in the BPS operated over 8 months (Fig. 4 and 6). Bacterial communities were influenced by both material and time (Fig. 7). Core communities (Fig. 8) and community membership reflected the differences between materials and the dynamic changes in biofilms over time.
 |
| Fig. 2 Migration potential tests for the six tested materials showing (A) the total organic carbon (TOC) and (B) growth potential of the migrated carbon. Data are stacked values from three of seven sequential migrations (Mig1, Mig3, and Mig7), with each migration conducted at 60 °C and lasting 24 hours. Error bars are adjusted standard deviations from duplicate migration tests, with triplicate growth potential tests from each duplicate. | |
 |
| Fig. 3 Biomass formation potential test (30 °C, 14 days) for the 6 tested hose materials. Stacked bars show total cell concentrations measured in the planktonic phase and biofilm phase after 14 days of growth relative to the surface area of the material. Error bars represent standard deviation from biological triplicates. | |
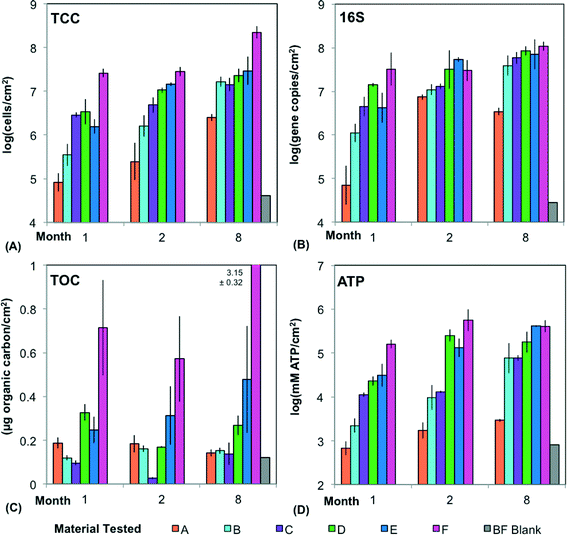 |
| Fig. 4 Biofilm formation on the six tested hose materials quantified using four methods after 1, 2, and 8 months of operation in a realistic building plumbing simulator (BPS). (A) Total cell concentration (TCC), (B) 16S rRNA gene copies (16S) measured by qPCR, (C) total organic carbon (TOC), and (D) concentration of adenosine tri-phosphate (ATP) were measured on biofilm suspensions and normalized to the available surface area. Error bars are from biological triplicates of each material type. Technical triplicates for each measurement (for ATP and 16S) are not included in standard deviation calculation. BF Blank refers to a biofilm extraction blank processed with other samples during the 8th month analysis. | |
3.1. Short-term material tests show differences in migration and growth behavior between materials
The six materials reacted differently in a comprehensive test package (BioMig12) that measures migration potential at 60 °C and biomass formation potential (BFP) at 30 °C. Fig. 2 shows the results from the migration potential assay, with migrated TOC (Fig. 2a) and growth potential of the leachate (Fig. 2b) from 3 of the 7 migrations (days 1, 3, and 7) stacked to visualize the total migration potential. Materials A–C, approved for use in drinking water (Table 1), leached less TOC (0.4–4.2 μg cm−2) than Materials D–F (Fig. 2a), and had relatively less biological growth supported by the leachate (4.5–5.1 ×106 cells per cm2) (Fig. 2b). In contrast, Materials E and F leached higher amounts of carbon (9.7–10.4 μg cm−2) and the leachate supported substantially more growth (3.8–4.8 × 107 cells per cm2). An interesting outlier to this trend was Material D, which leached by far the highest total amount of TOC (54.9 μg cm−2), and supported the least growth (2.7 × 106 cells per cm2). This contrasting behavior is directly attributed to the inorganic silver-ion coating with antimicrobial properties, which seems to inhibit growth during the first migration (with 38.47 μg cm−2 organic carbon leaching, but no detectable growth on this leachate). This effect diminished in later migrations, indicating that much of the coating was removed in the first migration. The efficiency of conversion from leached carbon to cell growth also varied considerably. This proportion of assimilable organic carbon (AOC) can be calculated using the expectation that 1 μg of carbon produces 107 cells.12 Material C has notably efficient conversion, leaching nearly 100% AOC compared to Materials A, B, E, and F ranging from 10% to 65%, and Material D, with antimicrobial inhibition, at 0.5% assimilable carbon (Fig. S1†). With this wide range, it is evident that using TOC leaching from pipes as a sole proxy for biological growth potential is completely erroneous. Many existing materials tests quantify TOC leaching from pipes, but their focus is rather on sensory aspects or potential disinfectant by-product formation,30–33 and thus they strive to include this non-utilizable portion, as the complex structures that bacteria cannot break down may contribute to taste and odor compounds.
The results from the BFP assay are shown in Fig. 3, with growth after 14 days in the planktonic and biofilm phases stacked. Similar to the results of the migration potential assay, there is a clear division between Materials A–C and Materials D–F, with Materials A–C supporting less growth (total BFP of 1.7–6.7 × 107 cells per cm2) than Materials D–F (total BFP = 2.9–8.3 × 108 cells per cm2). The ranking amongst Materials A–C varies between the BFP and migration potential assays, but the differences between these materials are not extreme in either assay (12% difference in growth supported by leachate and 10% difference in total BFP). The high growth supported by Material D further suggests that any inhibitory effect of the patented inorganic silver-ion coating was short-lived. It also may be localized, as 74% of the growth occurred in the planktonic phase, away from the inhibitory surface. Differences between the migration test and the BFP test can also be driven by the difference in temperatures (60 °C in migration compared to 30 °C in BFP), which could influence both the rate of leaching and the specific compounds released. Results from similarly classified materials ranged from quite different to extremely similar to previous results with the same test-package,12 indicating that variation in production quality and further production details (e.g. percent flexibilizer added) can be critical to performance of the materials.
3.2. Realistic use conditions differ from material tests
A survey of 7 households revealed that the maximum stagnation time for an individual flexible pipe typically occurred overnight in shower hoses. The maximum stagnation time observed in one week in a shower hose ranged between 13.5 hours (5-person household) and 49.5 hours (2-person household). The average maximum stagnation time was 28.1 hours for showers, and only 17 hours for kitchen faucets. Interestingly, while the maximum time differed considerably by location in the household, the estimated total stagnation time was similar (23.8 hours per day for kitchen faucets and 23.5 hours per day for showers). Material tests (section 3.1 above) use fixed conditions (time/temperature) over a short duration. For practical reasons, these conditions are not consistent with the actual long-term use of the products, but are rather designed to capture the maximum potential impact of the material in a reasonable amount of time. To measure realistic use, the BPS was run with shower-temperature water (42 °C) for a short time (15 minutes), with stagnation during 23.75 hours at ambient room temperature, and a total duration of 8 months. According to this survey, a stagnation time of 23.75 hours reflects shower usage in a household of 1–2 persons.
3.3. Biofilm development in the realistic BPS is influenced by material and time
Over the course of 8 months, biofilm growth observed with a variety of methods (TCC, qPCR for 16S, TOC, and ATP) in a BPS using discontinuous water flow similar to that of a typical daily shower, was highly variable and dependent upon both material and time (Fig. 4).
The materials with approval for drinking water applications (A–C) had a significantly lower biofilm concentration than Materials D–F across all four measures of biofilm (Fig. 4) (p < 0.001, t-test; p < 0.001, Kruskal–Wallis Test). This is driven by Materials A and F, the extremes in each category. When comparing individual materials pair-wise, the only significant differences involved A (monthly averages ranging from 7.9 × 104 to 6.3 × 106 cells per cm2) or F (monthly averages 2.5 × 107–2.0 × 108 cells per cm2). A was significantly lower than all materials except B, and Material F was significantly higher than all materials except D (p < 0.05, Tukey's HSD). The similarity between Materials B–E becomes especially obvious in month 8, when average biofilm concentrations ranged only from 1.6–3.2 × 107 cells per cm2. This diminished effect of material with time may be attributed to diminished nutrient leaching.
While an increase in biofilm concentration is clear (Fig. 4), it is difficult to draw conclusions about when or if biofilms entered steady state using only three time points. Biofilm concentrations in month 8 were significantly higher than the other months using TCC (material averages ranging from 6.3 × 106 to 2.0 × 108 cells per cm2 compared to months 1 and 2 ranging from 7.9 × 104 to 3.2 × 107 cells per cm2) (p < 0.03, Tukey's HSD). However, using the other measurements of biofilm concentration, month 8 was not always significantly different than the earlier months (p > 0.05, Kruskal multiple comparisons). While other studies suggest that steady state for drinking water biofilms is reached in two to three months or less,34,35 it is likely that more time was required in this scenario, with discontinuous flow and inconsistent temperatures. With the high amounts of carbon leaching from the pipes, growth is limited based on the additional influx of nitrogen, phosphorous and other nutrients from the water during flushes. The biofilm concentrations in this study were also higher than those in distribution systems with continuous flow36,37 and systems with chlorine,38 and had the most similarity (via qPCR) to biofilms on PEX within a building.39 Due to destructive sampling, a higher temporal resolution could not be achieved in the current study.
Biofilm concentration data from qPCR, TCC, and ATP correlated strongly with each other (Fig. 5) (ρ > 0.85, Spearman rank correlation). ATP had the strongest correlation with the intact cells (ICC) (ρ = 0.92, Spearman rank correlation), showing that these two measures of viability and activity are complementary for biofilms, as was previously demonstrated for drinking water samples.40 TOC had weaker correlations (ρ ranging from 0.52 to 0.65, Spearman rank correlation) with all other measures of biofilm concentration. This is not surprising, as TOC includes organic matter other than cells, such as extracellular polymeric substance (EPS).
 |
| Fig. 5 Correlations between biofilm concentration measures. For each scatter plot, n = 54, with measurements from 3 time-points on 3 triplicates of 6 material types. Spearman rank correlation values are superimposed on plots with potential values ranging from 0 (no correlation) to 1.00 (perfect correlation). Values less than 0.7 are in blue, >0.7 and <0.9 in green, >0.9 in red. Values for the Spearman rank correlation are identical whether or not the values are log-transformed. | |
3.4. BioMig data predicts material behavior in realistic BPS
The BioMig test package largely predicted the behavior of the six test materials in the realistic BPS for an extended time period. This test package produces several metrics of material performance, but the most comparable metric to biofilm formation in the BPS (Fig. 4A) is the biofilm biomass formed in the BFP test (Fig. 3). A log–log correlation between the two TCC measurements by month (Fig. 6) showed that the BioMig metric predicted the behavior of the month 2 measurements best, (R2 = 0.83, linear regression), compared to month 1 (R2 = 0.78), and month 8 (R2 = 0.77). A 1
:
1 ratio is not achieved in any of the tests, with biomass quantification typically about 1 log lower in the realistic BPS than the BFP test. While the absolute numbers are not the same, both the rankings and degree of difference are reasonably well predicted with the BioMig test package, even when both hard and flexible pipes are considered together.
 |
| Fig. 6 Correlation between the biofilm concentrations in the realistic building plumbing simulator (in Fig. 4A) with the biofilm biomass formation potential measured in a BioMig assay (in Fig. 3). Biofilm was sampled from the building plumbing simulator in biological triplicates on three occasions, in months 1, 2, and 8 of operation. The biofilm biomass formation potential is the average from a 2 week material test conducted in triplicate. | |
Monthly variations in correlation patterns are likely related to differences in the growth conditions of the BioMig test and the BPS. In the BioMig test, inorganic nutrients and oxygen are supplied in excess of the 14 day test period, whereas in the BPS, inorganics are supplied only intermittently (water replaced at each shower event) over 8 months. On top of this, growth potential tests of the water used in the BPS showed that it was primarily carbon-limited, but that inorganic nutrient limitations (nitrogen and phosphorous) quickly hindered growth, even with AOC concentrations as low as 1 mg L−1 (ESI,† Table S5). This theory of multiple nutrient limitations is supported with the data from materials A–C in early months, which has the highest linearity. Here, the inorganics supplied by intermittent water flow were likely sufficient to match the low amount of carbon leaching from materials. There is a clear need to investigate the growth of complex bacterial communities under conditions of continuously diminishing organic carbon supply and intermittent supply of inorganic nutrients in more detail.
3.5. Bacterial community structure driven by both material and time
The bacterial communities of all hoses consisted of 19 bacterial phyla from which individual phyla were present above 1% of any given sample. This is comparable to the phyla-level diversity found in other studies (e.g. 9 phyla41 and 14 phyla8). Five dominant phyla (Proteobacteria, Bacteroidetes, Planctomycetes, Actinobacteria, and Acidobacteria) accounted for 94% of all sequences (range 67–99.9% per sample). The most dominant phylum was Proteobacteria (78.8% of all reads; range 23–99.9% per sample), most of which were Alpha-Proteobacteria (range 3–25% per sample) which often dominates drinking water biofilms.14,41–43
Bacterial communities clustered according to both material and month, with material forming tight clusters within the month that they were measured (Fig. 7). Both month and material were significant factors for explaining the differences in community structure (p < 0.001, Adonis) with the material explaining slightly more variation than the month (R2 = 0.31 and 0.25, respectively, Adonis). The different materials leach different amounts (Fig. 2) and different types1 of organic carbon, which can in turn select and promote growth of different bacteria from the same source (water phase). Such differences have been previously demonstrated with obvious differences in pipe material (e.g. metal, cement, and plastic8), but not for such a narrow functional group of pipe materials. Neither bacterial concentrations (Fig. 4) nor bacterial community structure (Fig. 7) stabilized within the experimental time frame. With continued growth and diminishing carbon leaching from pipe materials, nutrient and oxygen gradients develop and change dynamically, and thus the bacterial communities also shift. Seasonality in the drinking water44 may also be reflected in the biofilm phase due to daily seeding. Further studies will investigate these dynamics with higher temporal resolution than was possible here due to destructive sampling methods.
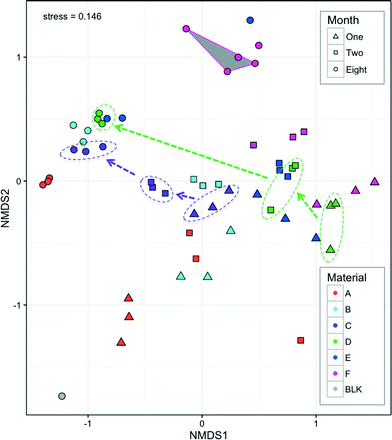 |
| Fig. 7 NMDS calculated with Bray–Curtis similarity between samples from six different hoses during eight months in a building plumbing simulator. Color is by material and symbol shape is by month. Dotted lines and arrows link biological triplicate samples for two materials to highlight the clustering through time. Three technical replicates were applied to one Material F (month 8) DNA extract, and these are linked with a gray triangle. BLK is a blank of the biofilm extraction procedure. | |
All four measures of biofilm concentration correlated with the NMDS analysis (p < 0.001, EnvFit), with a range of correlations in a similar direction (R2 range 0.37–0.88) (ESI,† Fig. S2). Interestingly, when biofilm concentrations measured by TCC were similar in month 8 for Materials B–E (1.6–3.2 × 107), communities also clustered closely (Fig. 7). This co-variance further indicates that the nutritional additions of the pipe material which influenced biofilm concentrations (Fig. 3) also drove the community structure. It was recently proposed that such knowledge could be used to manage bacteria under the framework of drinking water ‘probiotics’.45,46 This concept suggests that engineers should encourage the establishment of benign bacterial communities instead of aiming to kill or otherwise minimize all bacteria (e.g. disinfection, anti-bacterial pipes). If materials can be designed to leach carbon that selects a desirable community structure in the long-term, then shower hoses may offer a very practical avenue for application of these concepts. This is further discussed below with respect to genera that include opportunistic pathogens.
3.6. Core communities reflect dynamic changes in biofilms
In order to see which taxa were the most important for community clustering, core communities were calculated by looking at genera that were present in 100% of samples. Fig. 8 shows the Universal Core, Transient Core and Material Cores for each set of biological replicates. The Universal Core consisted of genera common to all biofilms (54 samples). The Material Cores consisted of genera common to a particular material throughout time (9 samples). The Transient Cores were common to all samples within a sampling period (18 samples). Genera could belong to both the Material Core and the Transient Core.
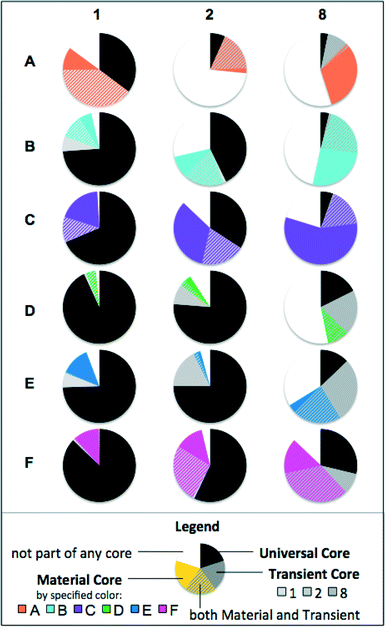 |
| Fig. 8 Core communities by combined relative abundance for the six materials at three time points (months 1, 2, and 8) in a building plumbing simulator. The Universal Core (black) represents genera common to all samples, while the Transient Core (grey) was common to all samples during a given month. Material Cores (material-specific color) were present in all samples of a material, and genera shared by the Transient Core and the Material Core are hatched with gray and material-specific color. | |
Seven genera were common to all biofilm samples (Universal Core), and these genera accounted for 44% of all sequences from normalized samples (range 2–98% per sample). In a similar analysis of drinking water samples from 5 networks in various locations across the US, 78% of all sequences belonged to a universal or network-specific core, even with much more varied pipe materials.11 Despite diversity generally being higher in drinking water communities than biofilms,41,42 material-specific niches develop within biofilms41 which result in more localized biofilm community variation compared to the bulk water phase. In all materials, the core community decreased over time, concurrent with diversity increasing in most, but not all materials (ESI,† Fig. S3).
The trends for these core communities could be separated by whether materials encouraged low-biomass (A–C) or high-biomass (D–F) development (Fig. 4). Materials D–F generally had larger Universal Cores than Materials A–C, and had a higher proportion of Transient Core than the Material Core. Materials A–C had smaller Universal Cores, and a higher proportion of the Material Core than the Transient Core. The high amounts of material-specific organic carbon leaching Materials D–F likely favored the growth of a few fast-growing bacteria. These few fast-growers, once established, are not likely to be completely displaced (high Universal Core). However, together with rapid biofilm development the available niches change drastically as carbon leaching diminishes and nutrient and oxygen gradients develop, and thus, new bacteria are able to outcompete (higher Transient Core, lower Material Core). With limited organic carbon from the material (A–C), there is little growth to select universally suited bacteria (low Universal Core). While the bacteria that first attach randomly are not necessarily displaced by competition, these do not necessarily match the bacteria suited for growth in all biofilms (higher Material Core). These biofilms are less subject to changing niches (lower Transient Core).
3.7. Membership in communities is dependent on materials
The seven genera common to all samples were Caulobacter, Bradyrhizobium, Sphingomonas, Methyloversatilis, Legionella, and Phenylobacterium, and unclassified genera within Comamonadaceae. Bradyrhizobium and Comamonadaceae have previously been associated with regrowth in pure water,47 and might be expected as background. Comamonadaceae contributed up to 24% of a single biofilm sample, and thus should be considered an important family for biofilm formation in drinking water. The family Comamonadaceae has also been associated with biological instability in the same region,48 and may have been so prevalent in that study due to detachment from biofilms.
The most prevalent of the core genera, Caulobacter, was most abundant during month 1 and generally decreased with time. Members of Caulobacter are frequently studied for their swarm cell growth phase and advantages with both attachment and continuous dispersal within biofilms.49–51 However, to our knowledge, Caulobacter is not frequently reported as a key member of drinking water biofilms. Other taxa were more prevalent with increasing time. Syntrophobacterales, a strictly anaerobic and sometimes sulfate reducing order, previously associated with EPS in anaerobic membrane bioreactors,52 is most abundant in month 8. As biofilms thicken, oxygen and nutrient gradients are created,41 and foster these more diverse metabolisms.
Other prevalent taxa had behavior specific to materials, further emphasizing the importance of material choice. The order Sphingomonadales was prevalent across all samples, but was lowest in Material F. Sphingomonas have previously been identified in distribution system biofilms41,43 and in shower curtain biofilms.53 Other orders, Actinomycetales and Solirubrobacterales, were mostly prevalent in low-biomass supporting materials (A–C).
In contrast, some orders tended to appear in high-biomass supporting materials. Saprospirales was most prevalent with Materials D–F in later months. Sediminibacterium, an iron-oxidizing genus within this order, accounted for most of this, and has been identified in several other drinking water systems.54,55 An orange color, perhaps related to iron deposition from earlier plumbing in the system, was most notable on Material D, due to the white colored material that was easily stained (Table 1). On Material F, Bdellovibrio was particularly prevalent. As this genus of bacteria is a parasite on other bacteria,56 it likely thrived in the high-biomass environment.
While sequencing resolution did not allow identification of specific opportunistic pathogens, several genera that include opportunistic pathogens were observed. One of the 7 Universal Core genera was Legionella, with a maximum of 9% in a sample (month 2 on Material D). The maximum warm water temperature was 42 °C both in this BPS and throughout the building, and this temperature range has been associated with Legionella colonization.57 It is likely that Legionella was present in the building's plumbing and inoculated the test system, enabling establishment there. Pseudomonas was most common in Materials A and B during month 2, making up 72% and 24% of these samples, respectively. Mycobacterium and Nocardia were mostly associated with Materials C and A, with 16% of the month 2 samples on Material C identified as Mycobacterium. Interestingly, Materials A–C, which generally supported a lower total biomass, had higher relative abundance attributed to these four genera (Legionella, Pseudomonas, Nocardia, and Mycobacterium) than Materials D–F (ESI,† Fig. S4A). Even when considering absolute abundance, Materials A–C consistently had higher values for Mycobacterium and Nocardia than Materials D–F (ESI,† Fig. S4B). Opportunistic pathogens have been reported in shower plumbing previously,6 yet the patterns of their prevalence seem to be complex. This study suggests that plastics supporting low-biomass preferentially select certain opportunistic pathogen containing genera. While this study was not specifically a pathogen study, the data warrants further investigation into this phenomenon. It may also support the probiotics concepts proposed by Wang et al.,45 in that a material with strong leaching behavior that supports a higher biomass can better protect against certain opportunistic pathogens.
3.8. Implications for regulations
Standard commercially available shower hoses (Materials E and F) were made from the same material (PVC-P). The differences between these materials observed in all tests are attributed to unknown production details (e.g. percentage and type of flexibilizer added). However, in all tests except the first month of the BPS, these two materials were ranked in the top three biomass producing materials (Fig. 2–4). Interestingly, these hoses are not specifically approved for use in drinking water applications,12 yet can be used in shower hoses, as a shower is not legally considered as a drinking water source. This regulation makes little sense, as the most frequently cited biological health concern for growth in drinking water is opportunistic pathogens, which infect via inhalation, as in showers.58 At the same time, the microbial communities that materials select are not considered in material certification processes at all. Certain opportunistic pathogens may have actually preferred the certified materials (ESI,† Fig. S4). This study is a clear indication that the testing of the microbiological impact of materials in contact with drinking water should be expanded considerably and should be extended towards all pipe and plumbing materials to which the consumer is ultimately exposed.
4. Conclusions
This study demonstrates the importance of attention to detail when planning a building plumbing system. In shower hoses, stagnation times can easily reach 28 hours in real households, and temperatures vary only between 20 °C and 42 °C – conditions which are optimal for bacterial growth. Functionally similar materials intended for use as shower hoses or final connections, all plastic and most flexible, had distinct microbiological reactions along several threads:
• In short term material comparison tests, materials with approval for use in drinking water applications released less organic carbon and supported less growth than those that were not regulated.
• Growth in a realistic BPS with discontinuous warm water flow reflected that of a comprehensive material-testing package (BioMig) designed to assess migration behavior and biological impact of materials in water.
• Bacterial community development was dependent on both material and month in a realistic BPS, with tight clusters by material shifting in a similar direction over time.
• Even with the same influent water, only 44% of sequences were from genera shared across all samples, with a higher proportion shared by young and high-biomass biofilms, demonstrating that that the materials and the biofilms developing on them offer significant space for diversification.
• The community structure was related to biofilm concentration. After 8 months, four of the six materials supported similar biofilm concentrations and had similar communities, drawing into question the long-term efficacy of some advanced material designs.
• The occurrence of certain potential opportunistic pathogens may be negatively correlated with the biofilm concentration.
Acknowledgements
The authors acknowledge financial support from MERMAID, a Marie Sklodowska-Curie Initial Training Network, under grant no. 607492. We also acknowledge financial support from “The Commission for Technology and Innovation CTI” (KTI-Nr. 15857.1 PFIW-IW) and industrial partners Geberit International AG and Georg Fischer JRG AG. Sequencing data analyzed in this paper were generated in collaboration with the Genetic Diversity Centre (GDC), ETH Zurich, with support and protocols from Aria Minder and Silvia Kobel. Karin Beck and Helmut Bürgmann are acknowledged for assistance with qPCR assays. We acknowledge Marcel Lüscher, GF JRG AG, for the donation of some plumbing supplies.
References
- M. Bucheli-Witschel, S. Kötzsch, S. Darr, R. Widler and T. Egli, Water Res., 2012, 46, 4246–4260 CrossRef CAS PubMed.
- M. LeChevallier, N. Welch and D. Smith, Appl. Environ. Microbiol., 1996, 62, 2201–2211 CAS.
- D. van der Kooij, J. - Am. Water Works Assoc., 1992, 84, 57–65 CAS.
- B. E. Rittmann and V. L. Snoeyink, J. - Am. Water Works Assoc., 1984, 76, 106–114 CAS.
- Z. Zhu, C. Wu, D. Zhong, Y. Yuan, L. Shan and J. Zhang, Appl. Biochem. Biotechnol., 2014, 173, 1564–1578 CrossRef CAS PubMed.
- L. M. Feazel, L. K. Baumgartner, K. L. Peterson, D. N. Frank, J. K. Harris and N. R. Pace, Proc. Natl. Acad. Sci. U. S. A., 2009, 106, 16393–16399 CrossRef CAS PubMed.
- N. J. Ashbolt, M. E. Schoen, J. A. Soller and D. J. Roser, Water Res., 2010, 44, 4692–4703 CrossRef CAS PubMed.
- H. Wang, S. Masters, M. A. Edwards, J. O. Falkinham 3rd and A. Pruden, Environ. Sci. Technol., 2014, 48, 1426–1435 CrossRef CAS PubMed.
- W. Lin, Z. Yu, X. Chen, R. Liu and H. Zhang, Appl. Microbiol. Biotechnol., 2013, 97, 8393–8401 CrossRef CAS PubMed.
- J. Lu, H. Y. Buse, V. Gomez-Alvarez, I. Struewing, J. Santo Domingo and N. J. Ashbolt, J. Appl. Microbiol., 2014, 117, 905–918 CrossRef CAS PubMed.
- P. Ji, J. Parks, M. A. Edwards and A. Pruden, PLoS One, 2015, 10, e0141087 Search PubMed.
- G. Wen, S. Kötzsch, M. Vital, T. Egli and J. Ma, Environ. Sci. Technol., 2015, 49, 11659–11669 CrossRef CAS PubMed.
- DVGW Technical rule - Code of practice W 270-2007 Microbial enhancement on materials to come into contact with drinking water – testing and assessment, Wirtschafts -und Verlagsgesellschaft Gas und Wasser mbH, 2007.
- J. Inkinen, T. Kaunisto, A. Pursiainen, I. T. Miettinen, J. Kusnetsov, K. Riihinen and M. M. Keinanen-Toivola, Water Res., 2014, 49, 83–91 CrossRef CAS PubMed.
- P. Quevauviller, O. F. X. Donard and A. Bruchet, Appl. Organomet. Chem., 1991, 5, 125–129 CrossRef CAS.
-
Guideline for Hygienic Assessment of Organic Materials in Contact with Drinking Water (KTW Guideline), Umwelt Bundesamt (Federal Environmental Agency of Germany), Dessau-Roβlau, Germany, 2008 Search PubMed.
- E. I. Prest, F. Hammes, S. Kötzsch, M. C. M. van Loosdrecht and J. S. Vrouwenvelder, Water Sci. Technol.: Water Supply, 2016 DOI:10.2166/ws.2016.001.
- F. Hammes, M. Berney, Y. Wang, M. Vital, O. Köster and T. Egli, Water Res., 2008, 42, 269–277 CrossRef CAS PubMed.
- M. Berney, M. Vital, I. Hülshoff, H.-U. Weilenmann, T. Egli and F. Hammes, Water Res., 2008, 42, 4010–4018 CrossRef CAS PubMed.
- E. I. Prest, F. Hammes, S. Kötzsch, M. C. M. van Loosdrecht and J. S. Vrouwenvelder, Water Res., 2013, 47, 7131–7142 CrossRef CAS PubMed.
- E. I. Prest, J. El-Chakhtoura, F. Hammes, P. E. Saikaly, M. C. M. van Loosdrecht and J. S. Vrouwenvelder, Water Res., 2014, 63, 179–189 CrossRef CAS PubMed.
- F. Hammes, F. Goldschmidt, M. Vital, Y. Wang and T. Egli, Water Res., 2010, 44, 3915–3923 CrossRef CAS PubMed.
- K. Takai and K. Horikoshi, Appl. Environ. Microbiol., 2000, 66, 5066–5072 CrossRef CAS PubMed.
- A. Klindworth, E. Pruesse, T. Schweer, J. Peplies, C. Quast, M. Horn and F. O. Glöckner, Nucleic Acids Res., 2013, 41, e1 CrossRef CAS PubMed.
-
R Development Core Team, R Foundation for Statistical Computing, 2015 Search PubMed.
-
F. de Mendiburu, R Packag. version 1.2-3, https://CRAN.R–project.org/package=agricolae.
- D. McDonald, M. N. Price, J. Goodrich, E. P. Nawrocki, T. Z. DeSantis, A. Probst, G. L. Andersen, R. Knight and P. Hugenholtz, ISME J., 2012, 6, 610–618 CrossRef CAS PubMed.
- P. J. McMurdie and S. Holmes, PLoS One, 2013, 8, e61217 CAS.
-
J. Oksanen, F. G. Blanchet, R. Kindt, P. P. Legendre, P. R. Minchin, R. B. O'Hara, G. L. Simpson, P. Solymos, H. Henry, H. Stevens and H. Wagner, R Packag. http://cran.r-project.org/web/packages/vegan/index.html, 2013 Search PubMed.
- T. H. Heim and A. M. Dietrich, Water Res., 2007, 41, 757–764 CrossRef CAS PubMed.
- D. Brocca, E. Arvin and H. Mosbæk, Water Res., 2002, 36, 3675–3680 CrossRef CAS PubMed.
- I. Skjevrak, A. Due, K. O. Gjerstad and H. Herikstad, Water Res., 2003, 37, 1912–1920 CrossRef CAS PubMed.
- S. T. Ryssel, E. Arvin, H.-C. H. Lützhøft, M. E. Olsson, Z. Procházková and H.-J. Albrechtsen, Water Res., 2015, 81, 269–278 CrossRef CAS PubMed.
- M. J. Lehtola, T. Juhna, I. T. Miettinen, T. Vartiainen and P. J. Martikainen, J. Ind. Microbiol. Biotechnol., 2004, 31, 489–494 CrossRef CAS PubMed.
- M. J. Lehtola, I. T. Miettinen and P. J. Martikainen, Can. J. Microbiol., 2002, 48, 494–499 CrossRef CAS PubMed.
- G. Liu, J. Q. Verberk and J. C. Van Dijk, Appl. Microbiol. Biotechnol., 2013, 97, 9265–9276 CrossRef CAS PubMed.
- M. J. Lehtola, M. Laxander, I. T. Miettinen, A. Hirvonen, T. Vartiainen and P. J. Martikainen, Water Res., 2006, 40, 2151–2160 CrossRef CAS PubMed.
- H. Wang, S. Masters, Y. Hong, J. Stallings, J. O. Falkinham, M. A. Edwards and A. Pruden, Environ. Sci. Technol., 2012, 46, 11566–11574 CrossRef CAS PubMed.
- J. Inkinen, T. Kaunisto, A. Pursiainen, I. T. Miettinen, J. Kusnetsov, K. Riihinen and M. M. Keinänen-Toivola, Water Res., 2014, 49, 83–91 CrossRef CAS PubMed.
- E. Siebel, Y. Wang, T. Egli and F. Hammes, Drinking Water Eng. Sci., 2008, 1, 1–6 CrossRef.
- G. Liu, G. L. Bakker, S. Li, J. H. Vreeburg, J. Q. Verberk, G. J. Medema, W. T. Liu and J. C. Van Dijk, Environ. Sci. Technol., 2014, 48, 5467–5476 CrossRef CAS PubMed.
- K. Henne, L. Kahlisch, I. Brettar and M. G. Höfle, Appl. Environ. Microbiol., 2012, 78, 3530–3538 CrossRef CAS PubMed.
- I. Douterelo, M. Jackson, C. Solomon and J. Boxall, Appl. Microbiol. Biotechnol., 2015, 1–11 Search PubMed.
- A. J. Pinto, J. Schroeder, M. Lunn, W. Sloan and L. Raskin, mBio, 2014, 5(3), e01135-14 CrossRef PubMed.
- H. Wang, M. A. Edwards, J. O. Falkinham 3rd and A. Pruden, Environ. Sci. Technol., 2013, 47, 10117–10128 CrossRef CAS PubMed.
- C. R. Proctor and F. Hammes, Curr. Opin. Biotechnol., 2015, 33, 87–94 CrossRef CAS PubMed.
- C. R. Proctor, M. A. Edwards and A. Pruden, Environ. Sci.: Water Res. Technol., 2015, 1, 882–892 Search PubMed.
- K. Lautenschlager, N. Boon, Y. Wang, T. Egli and F. Hammes, Water Res., 2010, 44, 4868–4877 CrossRef CAS PubMed.
- V. Hughes, C. Jiang and Y. Brun, Curr. Biol., 2012, 22, R507–R509 CrossRef CAS PubMed.
- D. Bodenmiller, E. Toh and Y. V. Brun, J. Bacteriol., 2004, 186, 1438–1447 CrossRef CAS PubMed.
- C. Berne, D. T. Kysela and Y. V. Brun, Mol. Microbiol., 2010, 77, 815–829 CAS.
- Z. Yu, X. Wen, M. Xu and X. Huang, Bioresour. Technol., 2012, 117, 333–340 CrossRef CAS PubMed.
- S. T. Kelley, U. Theisen, L. T. Angenent, A. St Amand and N. R. Pace, Appl. Environ. Microbiol., 2004, 70, 4187–4192 CrossRef CAS PubMed.
- H. Wang, C. Hu, X. Hu, M. Yang and J. Qu, Water Res., 2012, 46, 1070–1078 CrossRef CAS PubMed.
- L. Kahlisch, K. Henne, L. Gröbe, I. Brettar and M. G. Höfle, Microb. Ecol., 2012, 63, 383–397 CrossRef PubMed.
- R. M. Welsch, J. R. Zaneveld, S. M. Rosales, J. P. Payet, D. E. Burkepile and R. V. Thurber, ISME J., 2015, 219, 1–5 Search PubMed.
- E. Bédard, S. Fey, D. Charron, C. Lalancette, P. Cantin, P. Dolcé, C. Laferrière, E. Déziel and M. Prévost, Water Res., 2015, 71, 244–256 CrossRef PubMed.
- J. O. Falkinham, E. D. Hilborn, M. J. Arduino, A. Pruden and M. A. Edwards, Environ. Health Perspect., 2015, 123, 749–758 Search PubMed.
Footnote |
† Electronic supplementary information (ESI) available. See DOI: 10.1039/c6ew00016a |
|
This journal is © The Royal Society of Chemistry 2016 |
Click here to see how this site uses Cookies. View our privacy policy here.