DOI:
10.1039/C4SC03854A
(Edge Article)
Chem. Sci., 2015,
6, 2877-2884
γ-Al2O3 supported Pd@CeO2 core@shell nanospheres: salting-out assisted growth and self-assembly, and their catalytic performance in CO oxidation†
Received
12th December 2014
, Accepted 27th February 2015
First published on 27th February 2015
Abstract
In this paper, we have successfully demonstrated the clean synthesis of high-quality Pd@CeO2 core@shell nanospheres with tunable Pd core sizes in water, and furthermore loaded the as-obtained Pd@CeO2 products on commercial γ-Al2O3via electrostatic interaction. KBr here plays two key roles in inducing the growth and self-assembly of Pd@CeO2 core@shell nanospheres. First, Br− ions can retard the reduction of Pd2+ ions via the formation of the more stable complex of [PdBr4]2− so as to tune the size of Pd cores. Second, it greatly decreases the colloidal stability, and hence the surface polarity-weakened Pd and CeO2 NPs have to spontaneously self-assemble into more stable and ordered structures. Among different-sized Pd samples, the as-obtained 8 nm-Pd@CeO2/Al2O3 one exhibits the best performance in catalytic CO oxidation, which can catalyze 100% CO conversion into CO2 at 95 °C, which is much lower than the previously reported CeO2-encapsulated Pd samples.
1. Introduction
Pd based catalysts have received continuous attention for decades in a wide range of applications.1–5 For example, in organic chemistry, Pd(0) or its compounds have become indispensable to a mass of carbon–carbon bond forming reactions like Suzuki, Heck, and Stille coupling.6–10 Besides, supported PdOx has been recognized as one of the best catalysts for catalytic CH4 oxidation.11 Due to the harsh conditions they often work in, especially under high temperatures, the Pd catalysts find it hard to resist aggregation or growth, resulting in the heavy loss of catalytic active centers and hence severe catalytic degradation and even inactivation. That means it is very important for Pd NPs to well keep their original sizes and shapes and thus their long-lasting optimal catalytic performance. Thus, how to improve the thermal stability of Pd nanoparticles (NPs) becomes a serious and urgent global problem that needs to be solved. Among different solutions, to form core@shell nanostructures has been identified as the most efficient way.12–18 By densely coating with shell components, the Pd core can be physically protected, the mass transformation of which is able to be prevented during both the synthesis and the long-term catalytic cycling.
A variety of Pd@metal oxide core@shell nanostructures have been successfully fabricated, including Pd@SiO2,19,20 Pd@ZnO,21 Pd@TiO2,22 and Pd@CeO2.23 Among these oxides, CeO2 seems more distinctive as the shell because of its excellent chemical and physical properties such as extra-low-temperature oxygen storage capacity24 and the ability to generate strong synergistic effects associated with noble metals.25–28 Recently, Pd@CeO2 core@shell nanostructures have been successfully obtained via self-assembly that occurs between functionalized Pd NPs and Ce(IV) alkoxides in organic solutions.29–32 However, there still exist two problems in the Pd@CeO2 system. Firstly, such multi-step assembly requires precise control and complex surface modification, and moreover the unavoidable usage of expensive and toxic organic surfactants and solvents that are harmful for our health and environment. Secondly, the use of supports gives important benefits for enhancing the thermal stability of CeO2. However, the hydrophobic alkyl capping of such Pd@CeO2 core@shell nanostructures is repelled by the hydrophilic surface of metal oxide supports, especially the most used high-surface-area γ-Al2O3 powders. Before loading Pd@CeO2, it is inevitable to change the polarity of the γ-Al2O3 supports via complex and expensive surface modification.35 It is not conducive to large-scale synthesis and has seriously limited applications of catalysts. So in this study, the following two points have been focused upon in order to address the above mentioned issues. One is the aqueous clean synthesis of high-quality size-tunable Pd@CeO2 core@shell nanostructures without usage of organics, and the other is the direct assembly of them on γ-Al2O3 supports uniformly without any surface pretreatment.
Recently, an auto-redox relationship that happens between Ce3+ and noble metal ions has aroused scientists' interests. Following this strategy, Ag@CeO2 core@shell33–35 and Pt@CeO2 multi-core@shell nanospheres36 have been facilely obtained, and moreover the whole preparation processes are totally clean without using any expensive and toxic organopalladium compounds, long-chain alkyl surfactants and high boiling solvents, as well as complex synthetic steps and laborious post-treatment. More importantly, the naked surface of the Ag@CeO2 and Pt@CeO2 nanospheres favor them exhibiting better catalytic performance compared with those modified by surfactants. However unfortunately, such an advanced strategy does still not work in the very important Pd@CeO2 system (see Fig. S1 in ESI†) due to lack of enough driving force. Despite thus obtained bare Pd NPs are only supported on CeO2 with no ordered hybrid structure, it is still highly expected to find an appropriate thermodynamic condition to realize the desirable Pd@CeO2 core@shell ones.
Herein, the classic “salting-out effect” was first proposed in the clean synthesis of core@shell nanostructures. The salting-out effect can be generally explained as introducing a large number of inorganic salts into the mother solution so as to make the original solute supersaturated and thus being separated by crystallization, mostly applied in the biological areas for extraction. Until now, from a view point of synthesis, the most successful strategy of noble metal-based core@shell nanostructures is the seeded growth process. Tang and co-workers have reported a series of works on such an area.37–39 However, after carefully checking out the previous reports, there is still no report on such synthesis of NPs. In this work, we have tried to utilize the salting-out strategy to disclose the evolution of how high-quality Pd@CeO2 core@shell nanospheres were formed, and how inorganic metal salts (KCl, KBr or KI) worked during the growth and the self-assembly process of Pd and CeO2 components. Furthermore, we have systematically studied the size and the support (γ-Al2O3) influences on the catalytic performance of the Pd@CeO2 core@shell nanospheres towards CO oxidation. The whole synthesis is shown in Scheme 1.
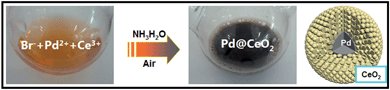 |
| Scheme 1 The synthetic process of Pd@CeO2 core@shell nanospheres. | |
2. Experimental section
2.1 Synthesis of 13 nm Pd@CeO2 core@shell nanospheres
The whole synthetic process is described in Scheme 1. 300 mg of KBr was dissolved in 20 mL of H2O first. The solution was heated to 60 °C for 10 min. Then 0.8 mL of Na2PdCl4 aqueous solution (1 mmol of PdCl2 and 2 mmol of NaCl were dissolved in 16 mL of H2O) and 2 mL of Ce(NO3)3 aqueous solution (0.1 M) were added followed by dropping 5 mL of ammonia (50 μL 25% ammonia dissolved in 20 mL of H2O). The mixture was heated at 60 °C for 1 h. After cooling down to room temperature, the product was purified by centrifugation and washed with water three times.
Pd@CeO2 core@shell nanospheres with other Pd core sizes were synthesized under similar reaction conditions, just by changing the amount of KBr and ammonia. For example, 300 mg KBr and 200 μL 25% ammonia, 150 mg KBr and 50 μL 25% ammonia, and 500 mg KBr and 50 μL 25% ammonia were used to synthesize 8 nm, 11 nm, and 17 nm sized Pd@CeO2 core@shell nanospheres, respectively. The final samples are named as 8 nm-, 11 nm-, 13 nm-, and 17 nm-Pd@CeO2, according to the size of Pd NPs.
2.2 Synthesis of Pt@CeO2 multi-core@shell nanospheres
The synthesis was similar to the above process. A certain amount of KI was dissolved in 20 mL of H2O first. The solution was heated to 60 °C for 10 min. Then 0.8 mL of K2PtCl4 aqueous solution (1 mmol of K2PtCl4 dissolved in 16 mL of H2O) and 2 mL of Ce(NO3)3 aqueous solution (0.1 M) were added, followed by dropping 5 mL of diluted ammonia (50 μL 25% ammonia dissolved in 5 mL of H2O). The mixture was heated at 60 °C for 1 h. After cooling down to room temperature, the product was purified by centrifugation.
2.3 Synthesis of Au@CeO2 core@shell nanospheres
The synthesis was similar to the above process, except using HAuCl4 and KCl instead of Na2PdCl4 and KBr, respectively.
2.4 Synthesis of CeO2 NPs
The synthesis was the same as the synthesis of Pd@CeO2 except for adding Na2PdCl4.
2.5 Pd@CeO2 supported on γ-Al2O3
100 mg commercial γ-Al2O3 powder was mixed with 100 mL water and 100 μL 25% ammonia. The mixture was heated at 95 °C for 2 h. Meanwhile, 100 mg of the Pd@CeO2 sample was dispersed in 50 mL water by ultrasound treatment. Finally, the as-obtained Pd@CeO2 colloids were dropped into the γ-Al2O3 dispersion very slowly. After heated at 95 °C for another 2 h, the products could be collected by centrifugation.
2.6 Heat treatment
All the samples have been calcined before catalytic tests. The calcination processes were performed in air at a heating rate of 5 °C min−1 to 600 °C and kept for three hours, and then cooled down to room temperature naturally.
2.7 Characterization
The X-ray diffraction patterns of the products were collected on a Rigaku-D/max 2500 V X-ray diffractometer with Cu-Kα radiation (λ = 1.5418 Å), with an operating voltage and current maintained at 40 kV and 40 mA. Transmission electron microscopic (TEM) images were obtained with a TECNAI G2 high-resolution transmission electron microscope operating at 200 kV. XPS measurements were performed on an ESCALAB-MKII 250 photoelectron spectrometer (VG Co.) with Al Kα X-ray radiation as the X-ray source for excitation.
2.8 Catalytic test
20 mg of catalysts were mixed with 20 mg of SiO2 powders. The mixture was put in a stainless steel reaction tube. The experiment was carried out under a flow of reactant gas mixture (1% CO, 20% O2, balance N2) at a rate of 30 mL min−1 (SV = 90
000 mL (g−1 h−1)). The composition of the gas was monitored on-line by gas chromatography (GC 9800).
3. Results and discussion
3.1 Pd@CeO2 core@shell nanospheres
The transmission electron microscope (TEM) images in Fig. 1A show that the as-obtained Pd@CeO2 hybrids are uniform and monodisperse sub-45 nm nanospheres. From Fig. 1B–D, it is found that these nanospheres have a core@shell superstructure. In any hybrid nanosphere, the shell is built up by hundreds of tiny CeO2 NPs assembling together. The Pd NPs beneath the shell can be distinguished by their deeper contrast from CeO2. Every sub-13 nm Pd particle is firmly embedded in the center of 5 nm CeO2 nanoparticle aggregation as a single core. It can be seen from the HRTEM images in Fig. 1D that the lattice spacing (0.31 nm) corresponds well with the characteristic (111) planes of fluorite phase CeO2. However, the dense CeO2 coating makes it hard to observe any clear crystal planes of Pd in the core position, so high-angle annular dark-field scanning transmission electron microscopy (HAADF-STEM) image (Fig. 1E–G) has been used to further analyze the distribution of Pd and Ce components in the nanospheres. It indicates that Ce element spreads everywhere and Pd only exists in the center of the nanospheres, well confirming the Pd@CeO2 core@shell nanostructure.
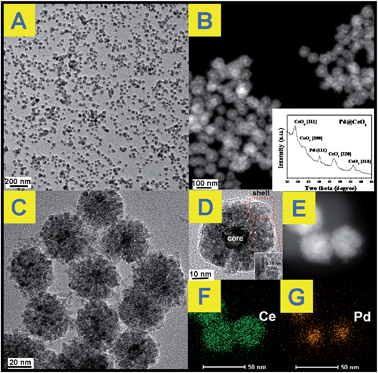 |
| Fig. 1 (A), (C) and (D): TEM images; (B) inside; (B) and (E) STEM image; (F) and (G) mapping analysis of the as-obtained Pd@CeO2 core@shell nanospheres. | |
The powder X-ray diffraction (XRD) pattern of the as-obtained sample (Fig. 1B, inset) matches well with those of the standard Pd (JCPDS no. 46-1043) and CeO2 (JCPDS no. 34-0394). The X-ray photoelectron spectroscopy (XPS) curves (Fig. S2†) identify that the two peaks at 349.9 eV and 340.4 eV correspond well to the Pd 3d5/2 and 3d3/2 spin orbit peaks of Pd, respectively, while the peaks at 881.9 eV and 900.2 eV can be assigned to Ce 3d5/2 and 3d3/2 spin orbit peaks, respectively. The Pd content in the as-obtained Pd@CeO2 core@shell nanospheres is 11 wt% determined by elemental analysis using inductively coupled plasma atomic emission spectrometry (ICP-AES). The specific surface area of the as-obtained Pd@CeO2 sample calculated from the BET curve (Fig. S3†) reaches about 72.25 m2 g−1, which is much bigger than that (19.46 m2 g−1) of our previously reported Pt@CeO2 multi-core@shell nanospheres.40 This is possibly caused by the smaller size of Pd@CeO2 hybrids.
To understand the effects of the feeding amount of KBr and NH3·H2O on the formation of Pd@CeO2 core@shell nanospheres, a series of control experiments have been designed to get a deeper insight into how the core@shell nanostructure evolved. We first studied the usage amount of KBr on the structural evolution by TEM characterization. As shown in Fig. S4,† no uniform hybrid nanostructure could be found in the absence of Br−. The corresponding EDX analysis (Fig. S5†) can identify that these irregular NPs are disorderly mixed Pd and CeO2 components. After adding 50 mg of KBr, each Pd nanoparticle began to be surrounded by hundreds of small CeO2 NPs to form a core@shell-like structures, but they aggregated seriously (Fig. S6†), and many un-coated Pd NPs could be easily found from the TEM images. With increasing the KBr amount to 150 mg, the core@shell spherical nanostructure became more clear, uniform and monodisperse (Fig. 2A and B). The Pd NPs grow from 11 nm to 13 nm to 17 nm on average while increasing the KBr amount from 150 mg to 300 mg to 500 mg as shown in Fig. 2C–F. If the NH3·H2O amount was increased to 200 μL in the presence of 300 mg of KBr, we could still obtain core@shell structured nanospheres as shown in Fig. 2P and Q, however both of the core@shell nanospheres and the Pd cores became much smaller. It means the increased alkalinity of the solution accelerated the aggregation of CeO2 NPs to give the Pd cores a timely stronger protection, resulting in the smaller size of Pd@CeO2 core@shell nanospheres as well. However, if we use NaOH to replace NH3·H2O, the product is an aggregation composed by numerous small NPs mixed together, forming the disordered Pd–CeO2 hybrids as shown in Fig. S7.†
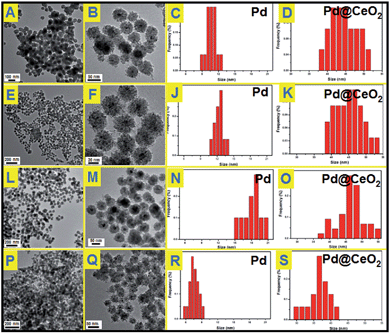 |
| Fig. 2 TEM images and particle size distributions of Pd@CeO2 core@shell nanospheres obtained by addition of different amounts of KBr in the reaction solution: (A), (B), (C) and (D) 150 mg KBr, 50 μL NH3·H2O; (E), (F), (J) and (K) 300 mg KBr, 50 μL NH3·H2O; (L), (M), (N) and (O) 500 mg KBr, 50 μL NH3·H2O; (P), (Q), (R) and (S) 300 mg KBr, 200 μL NH3·H2O. | |
The used amounts of KBr and NH3·H2O as well as the final sizes of the Pd core and the nanosphere have been summarized in Table 1. The results show that the sizes of Pd NPs are strongly dependent on the feeding amount of KBr as well as NH3·H2O. As reported, Br− ions can retard the reduction of Pd2+ ions via the formation of [PdBr4]2−, because the overall stability constant of [PdBr4]2− is nearly 104 times higher than that of [PdCl4]2− while [Br−]/[Cl−] = 7.5.40–42 According to the Nernst equation, the potential of Pd2+ is greatly reduced due to the formation of a more stable complex [PdBr4]2−.43 Obviously stable coordination of Pd2+ ions does not favor the independent nucleation of a Pd nanoparticle but its growth. This could give a good explanation for the phenomenon that the Pd NPs grew bigger and bigger while continuously increasing the used amount of KBr from 0 to 500 mg. However the self-assembly behavior of Pd and CeO2 remains still unclear, which is most likely driven by thermodynamics. Here we have tried to propose the salting-out effect to clarify this process.
Table 1 The usage amounts of KBr, NH3·H2O and the size of products
KBr (mg) |
NH3·H2O (μL) |
Nanosphere (nm) |
Pd (nm) |
CeO2 (nm) |
0 |
50 |
— |
<9 |
5 |
150 |
50 |
43 |
11 |
5 |
300 |
50 |
44 |
13 |
5 |
500 |
50 |
46 |
17 |
5 |
300 |
200 |
36 |
8 |
5 |
Generally, introducing a mass of inorganic salts to the mother aqueous solution will make the original solute supersaturated and thus being separated by crystallization. These salts can somehow weaken the polarity of the solute, resulting in reduced solubility in water. Comparatively the difference in our case is that the solutes were Pd and CeO2 NPs. So once KBr was introduced into the solution, these surface polarity-weakened Pd and CeO2 NPs in water have to spontaneously self-assemble into more stable and ordered structures due to highly decreased colloidal stability. Consequently, a large amount of Pd@CeO2 core@shell nanosphere deposits was observed and easily collected from the reaction solution after standing for about 30 min. As shown in Fig. S8,† after purification, these black deposits could be well re-distributed in water to form a very stable colloid until KBr was added again. The deposition–purification process can be successfully repeated for cycles by addition of KBr into the colloids.
3.2 Au@CeO2 and Pt@CeO2 core@shell nanostructures
Other kinds of halogen ions have been also studied instead of Br−. As shown in Fig. S9 and S10,† it is found that neither Cl− nor I− works to induce the self-assembly behavior between Pd and CeO2, demonstrating that the inducing effect of Br− is specific for the Pd–CeO2 system. The growth mechanism of the core@shell nanostructures could be also understood as the salting-out effect as mentioned above (as shown in Scheme 1). The precipitation of two components is accompanied by a self-assembly process to form the more stable core@shell nanoparticles. Following this principle, we successfully use KCl and KI to induce the formation of Au@CeO2 and Pt@CeO2 nanostructures, respectively. As shown in Fig. S11 and S12,† under the similar reaction conditions (300 mg of KBr), Br− couldn't induce the formation of either Au@CeO2 or Pt@CeO2 nanospheres. Unexpectedly, KCl specifically works towards Au–CeO2, while KI towards Pt–CeO2. For the KCl–Au–CeO2 system, until 300 mg KCl were added in the reaction solution, a core@shell nanostructure similar to Pd@CeO2 could be also observed. (Fig. S13†). While for the KI–Pt–CeO2 system as shown in Fig. 3, just a small amount of KI could induce the formation of uniform small Pt@CeO2 multi-core@shell nanospheres. The higher the concentration of KI in the reaction solution, the bigger the hybrid nanospheres. 30, 100 and 500 mg KI could assist the fabrication of 27, 52 and 86 nm Pt@CeO2 nanospheres, respectively.
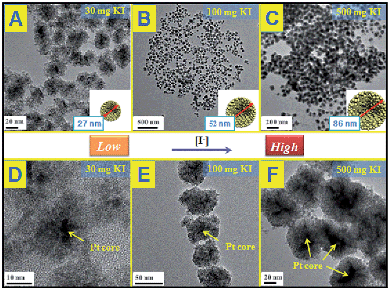 |
| Fig. 3 TEM images of Pt@CeO2 multi-core@shell nanospheres prepared by KI assistance; (A) and (D): 30 mg; (B) and (E): 100 mg; (C) and (F): 500 mg. | |
3.3 Pd@CeO2 supported on γ-Al2O3
Electrostatic attraction is a universal force that exists between oppositely charged components. It is noticed the as-obtained Pd@CeO2 core@shell nanospheres are positively charged (zeta potential: +25.3). So in this consideration, negatively charged γ-Al2O3 was supposed to be able to support the Pd@CeO2 core@shell nanospheres. The commercial γ-Al2O3 is neutral, however, their surface can carry negative charges (zeta potential: −15.5) if refluxed in aqueous NH3·H2O. As expected once the Pd@CeO2 colloids were added into the solution of the negative γ-Al2O3, the black precipitation appeared and could be easily collected at the bottom of the flask. The TEM images (Fig. 4) show that the as-prepared Pd@CeO2 core@shell samples with different Pd particle sizes have been successfully loaded onto the needle-like commercial γ-Al2O3 supports uniformly and kept their original monodispersity. No scattered or agglomerated ones are found. This could be attributed to the strong electrostatic attraction between CeO2 and γ-Al2O3 and the electrostatic repulsion between Pd@CeO2 nanospheres themselves. Compared with the previous report by Gorte's group, such pure inorganic loading strategy greatly simplifies the synthetic steps.11,33–36
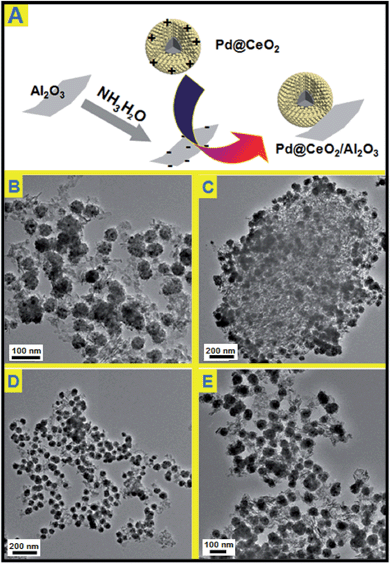 |
| Fig. 4 (A) Schematic representation of the self-assembly of Pd@CeO2 and commercial γ-Al2O3; (B) TEM images of 11 nm-Pd@CeO2/Al2O3; (C) 13 nm-Pd@CeO2/Al2O3; (D) 17 nm-Pd@CeO2/Al2O3; (E) 8 nm-Pd@CeO2/Al2O3. | |
3.4 Thermal stability
Before catalytic tests, the thermal stability of Pd@CeO2/Al2O3 was first examined by calcination at 600 °C for three hours in air. By comparing the TEM images (Fig. 5), it is found that there is no change in size, shape and structure, and no agglomeration happened in the cases of all the four Pd@CeO2 samples. Their excellent thermal ability could be attributed to the strong protection of the CeO2 shell and the support of γ-Al2O3.
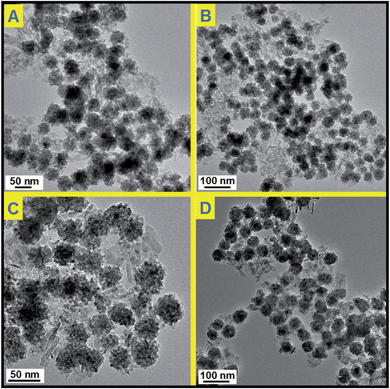 |
| Fig. 5 TEM images of Pd@CeO2/Al2O3 products after heating at 600 °C for 3 hours; (A) 11 nm; (B) 13 nm; (C) 17 nm; (D) 8 nm. | |
3.5 Catalytic CO oxidation
All the samples have been thermally treated in advance at 600 °C for three hours before every catalytic test. Then catalytic CO oxidation into CO2 has been chosen as the model reaction to study the catalytic activity and stability of the as-obtained Pd@CeO2 and Pd@CeO2/Al2O3 samples. The evaluation of catalytic activity was performed in a fixed-bed reactor coupled online with a gas chromatograph (see the catalytic activity measurement for CO oxidation in the Experimental section). Fig. 6A shows the typical conversion ratio of CO as a function of reaction temperature over five catalysts under the conditions that the feed gas containing 1 vol% CO and 99 vol% air was allowed to pass through the reactor at a total flow rate of 50 mL min−1, corresponding to a gas hourly space velocity (GHSV) of 15
000 mL h−1 g−1 cat. CO conversion curves in Fig. 6 show that the Pd@CeO2/Al2O3 samples exhibited a size-dependent catalytic performance. The T100 (100% conversion temperature) of the four samples follows such a sequence: 8 nm-Pd@CeO2/Al2O3 (95 °C) < 11 nm-Pd@CeO2/Al2O3 (145 °C) < 13 nm-Pd@CeO2/Al2O3 (250 °C) < 17 nm-Pd@CeO2/Al2O3 (280 °C). The 8 nm-Pd@CeO2/Al3O4 has a lower T100 of about 95 °C compared with the previous report by Gorte's group (about 110 °C),29 which is even much better than the currently reported Pt@CeO2 (135 °C),36 Au@CeO2 (155 °C)13 and Pt–CeO2 (140 °C)44 systems.
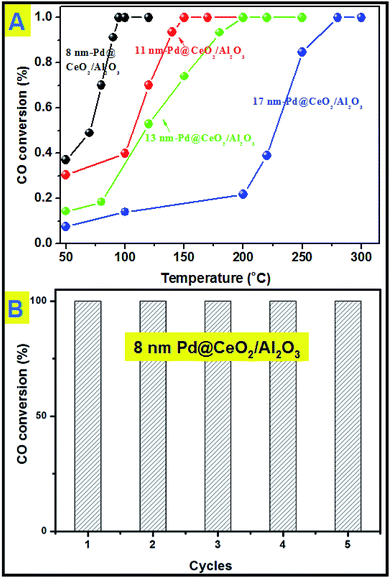 |
| Fig. 6 (A) catalytic CO conversion of Pd@CeO2/Al2O3 with different Pd core sizes; (B) cycling test of 8 nm-Pd@CeO2/Al2O3. | |
Generally, smaller Pd NPs are more active in catalytic CO oxidation. However, compared with the 2 nm-Pd@CeO2 core@shell nanospheres,29 the as-obtained 8 nm-Pd@CeO2/Al2O3 show better performance. This should be attributed to our clean synthesis route that favors the formation of a more strongly coupled interface between the Pd and CeO2 components, resulting in its excellent catalytic performance. Besides, if we compare the catalytic performance of the Pd–CeO2 sample obtained without addition of KBr with that of 8 nm-Pd@CeO2 (Fig. S14†) and with that of 8 nm-Pd@CeO2/Al2O3, it is clearly found that an ordered self-assembled core@shell structure and Al2O3 support are both beneficial for improving the activity of Pd catalysts.
Next, a cycling test was done to study the stability of 8 nm-Pd@CeO2/Al2O3, and before every cycle it was calcined in advance at 600 °C in air. As shown in Fig. 6B, after five successful cycles 8 nm-Pd@CeO2/Al2O3 still maintained 100% conversion rate of CO into CO2 at the testing temperature of 100 °C. In the TEM image taken after the cycling test (Fig. S15†) it is seen that 8 nm-Pd@CeO2/Al2O3 kept their original core@shell nanostructure well, indicating its excellent catalytic performance under long-term high-temperature catalytic conditions.
Research has been focused on the reactivity occurring at the interface of noble metal/ceria hybrids.45 In order to investigate the importance of maximizing the metal–support interaction by the core@shell approach, H2-TPR (H2-temperature programmed reduction) test has been done and is shown in Fig. 7. Two broad peaks observed at 390 and 760 °C for bare CeO2 can be attributed to the reduction of surface capping oxygen and bulk oxygen of CeO2, respectively.46 While, in the curve of Pd–CeO2 (prepared without addition of KBr), the two peaks have been reduced to 351 and 733 °C from 390 and 760 °C, respectively. However for 8 nm-Pd@CeO2, an intense and sharp peak could be clearly observed at 128 °C. The greatly reduced temperature indicates its higher oxygen delivery capacity which is strongly related to the ultra-high catalytic performance of the core@shell nanostructure.
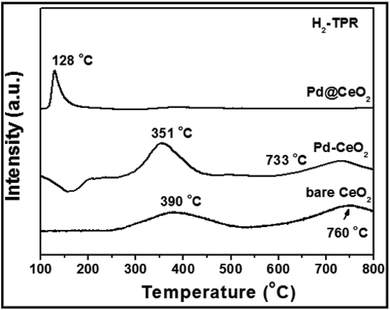 |
| Fig. 7 H2-TPR spectra of 8 nm-Pd@CeO2, Pd–CeO2 and bare CeO2 samples. | |
4. Conclusion
In conclusion, we have successfully developed a novel and low-cost auto-redox strategy to construct the Pd@CeO2 core@shell nanostructures with tunable Pd core sizes. The reaction is triggered between Ce(OH)3 and Na2PdCl4 in air without addition of any reducing agents or surfactants except for using KBr. The as-obtained Pd@CeO2 nanospheres are uniform and completely built up by spontaneously self-assembled naked Pd and CeO2 NPs. KBr here is reasonably supposed to play two key roles in determining the growth of Pd NPs and the final self-assembled hybrid spherical structures. First, Br− ions retarded the reduction of Pd2+ ions via the formation of the more stable complex of [PdBr4]2− so as to be able to tune the size of Pd cores. Second, due to the “salting-out effect” in water, it greatly decreases the colloidal stability, and hence the surface polarity-weakened Pd and CeO2 NPs have to spontaneously self-assemble into more stable and ordered structures. Besides interestingly, the similar “salting-out effect” strategy works well in the formation of other noble metal@CeO2 hybrids. It shows specific inducing effects that Cl− is specific towards the Au@CeO2 system, Br− towards Pd@CeO2, and I− towards Pt@CeO2.
With the help of electrostatic interactions, these positively charged Pt@CeO2 samples can be uniformly and strongly supported on the negatively charged Al2O3. Thanks to their ordered self-assembled core@shell structure and Al2O3 support, these Pd catalysts exhibited excellent activity and stability in catalytic CO oxidation. Among different-sized Pd samples, the as-obtained 8 nm-Pd@CeO2/Al2O3 shows the best performance, which can catalyze 100% CO conversion into CO2 at a relatively low temperature of 95 °C compared with those of the previously reported CeO2-encapsulated Pd samples. It is believed that such a “salting-out effect” strategy is of great significance in the synthesis of self-assembled inorganic NPs and design of highly active and stable catalysts in real-world applications.
Acknowledgements
This work was supported by the financial aid from the National Natural Science Foundation of China (Grant nos 91122030, 51272249, 21210001, 21221061 and 21401186), and the MOST of China (Grant no. 2014CB643802).
Notes and references
- X. M. Chen, G. H. Wu, J. M. Chen, X. Chen, Z. X. Xie and X. R. Wang, J. Am. Chem. Soc., 2011, 133, 3693 CrossRef CAS PubMed.
- M. Crespo-Quesada, A. Yarulin, M. Jin, Y. Xia and L. Kiwi-Minsker, J. Am. Chem. Soc., 2011, 133, 12787 CrossRef CAS PubMed.
- H. C. Peng, S. F. Xie, J. H. Park, X. H. Xia and Y. N. Xia, J. Am. Chem. Soc., 2013, 135, 3780 CrossRef CAS PubMed.
- M. Jin, H. Zhang, Z. Xie and Y. Xia, Energy Environ. Sci., 2012, 5, 6352 CAS.
- M. García-Melchor, A. A. C. Braga, A. Lledós, G. Ujaque and F. Maseras, Acc. Chem. Res., 2013, 46, 2626 CrossRef PubMed.
- N. Miyaura and A. Suzuki, Chem. Rev., 1995, 95, 2457 CrossRef CAS.
- M. Jin, H. Zhang, Z. Xie and Y. Xia, Angew. Chem., Int. Ed., 2011, 50, 7850 CrossRef CAS PubMed.
- J. K. Stille, Angew. Chem., Int. Ed. Engl., 1986, 25, 508 CrossRef.
- S. L. Buchwald, Acc. Chem. Res., 2008, 41, 1439 CrossRef CAS PubMed.
- L. Xue and Z. Lin, Chem. Soc. Rev., 2010, 39, 1692 RSC.
- M. Cargnello, J. Jaén, J. Garrido, K. Bakhmutsky, T. Montini, J. Gámez, R. Gorte and P. Fornasiero, Science, 2012, 337, 713 CrossRef CAS PubMed.
- P. M. Arnal, M. Comotti and F. Schuth, Angew. Chem., Int. Ed., 2006, 45, 8224 CrossRef CAS PubMed.
- J. Qi, J. Chen, G. D. Li, S. X. Li, Y. Gao and Z. Y. Tang, Energy Environ. Sci., 2012, 5, 8937 CAS.
- R. Güttel, M. Paul and F. Schüth, Catal. Sci. Technol., 2011, 1, 65 Search PubMed.
- A. D. Pandey, R. Guttel, M. Leoni, F. Schuth and C. Weidenthaler, J. Phys. Chem. C, 2010, 114, 19386 CAS.
- C. Galeano, R. Guttel, M. Paul, P. Arnal, A. H. Lu and F. Schuth, Chem.–Eur. J, 2011, 17, 8434 CrossRef CAS PubMed.
- C. Kuo, T. Hua and M. Huang, J. Am. Chem. Soc., 2009, 131, 17871 CrossRef CAS PubMed.
- I. Lee, J. B. Joo, Y. D. Yin and F. Zaera, Angew. Chem., Int. Ed., 2011, 50, 10208 CrossRef CAS PubMed.
- Y. Hu, K. Tao, C. Wu, C. Zhou, H. Yin and S. Zhou, J. Phys. Chem. C, 2013, 117, 8974 CAS.
- Z. Chen, Z. Cui, P. Li, C. Cao, Y. Hong, Z. Wu and W. Song, J. Phys. Chem. C, 2012, 116, 14986 CAS.
- H. Sun, J. He, J. Wang, S. Zhang, C. Liu, T. Sritharan, S. Mhaisalkar, M. Han, D. Wang and H. Chen, J. Am. Chem. Soc., 2013, 135, 9099 CrossRef CAS PubMed.
- B. Liu, Q. Wang, S. Yu, P. Jing, L. Liu, G. Xu and J. Zhang, Nanoscale, 2014, 6, 11887 RSC.
- N. Zhang, S. Liu, X. Fu and Y. Xu, J. Phys. Chem. C, 2011, 115, 22901 CAS.
- J. Zhang, H. Kumagai, K. Yamamura, S. Ohara, S. Takami, A. Morikawa, H. Shinjoh, K. Kaneko, T. Adschiri and A. Suda, Nano Lett., 2011, 11, 361 CrossRef CAS PubMed.
- Y. H. Zhang, N. Zhang, Z. Tang and Y. J. Xu, ACS Sustainable Chem. Eng., 2013, 1, 1258 CrossRef CAS.
- N. Zhang and Y. J. Xu, Chem. Mater., 2013, 25, 1979 CrossRef CAS.
- X. Wang, X. Y. Li, D. P. Liu, S. Y. Song and H. J. Zhang, Chem. Commun., 2012, 48, 2885 RSC.
- Y. Zhu, S. R. Zhang, J. J. Shan, L. Nhuyen, S. H. Zhan, X. L. Gu and F. Tao, ACS Catal., 2013, 3, 2627 CrossRef CAS.
- M. Cargnello, W. T. Montini, R. J. Gorte and P. Fornasiero, J. Am. Chem. Soc., 2010, 132, 1402 CrossRef CAS PubMed.
- N. L. Wieder, M. Cargnello, K. Bakhmutsky, T. Montini, P. Fornasiero and R. J. Gorte, J. Phys. Chem. C, 2011, 115, 915 CAS.
- L. Adijanto, D. A. Bennett, C. Chen, A. S. Yu, M. Cargnello, P. Fornasiero, R. J. Gorte and J. M. Vohs, Nano Lett., 2013, 13, 2252 CrossRef CAS PubMed.
- L. Adijanto, A. Sampath, A. S. Yu, M. Cargnello, P. Fornasiero, R. J. Gorte and J. M. Vohs, ACS Catal., 2013, 3, 1801 CrossRef CAS.
- T. Kayama, K. Yamazaki and H. Shinjoh, J. Am. Chem. Soc., 2010, 132, 13154 CrossRef CAS PubMed.
- T. Mitsudome, Y. Mikami, M. Matoba, T. Mizugaki, K. Jitsukawa and K. Kaneda, Angew. Chem., Int. Ed., 2011, 50, 136 Search PubMed.
- J. Zhang, L. P. Li, X. S. Huang and G. S. Li, J. Mater. Chem., 2012, 22, 10480 RSC.
- X. Wang, D. P. Liu, S. Y. Song and H. J. Zhang, J. Am. Chem. Soc., 2013, 135, 15864 CrossRef CAS PubMed.
- J. Du, J. Qi, D. Wang and Z. Tang, Energy Environ. Sci., 2012, 5, 6914 CAS.
- G. Li and Z. Tang, Nanoscale, 2014, 6, 3995 RSC.
- J. Chen, D. Wang, J. Qi, G. Li, F. Zheng, S. Li, H. Zhao and Z. Tang, Small, 2015, 11, 420 CrossRef CAS PubMed.
- H. Zhang, M. Jin, Y. Xiong, B. Lim and Y. X. Xia, Acc. Chem. Res., 2013, 46, 1783 CrossRef CAS PubMed.
- Y. Xiong, H. Cai, Y. Yin and Y. Xia, Chem. Phys. Lett., 2007, 440, 273 CrossRef CAS PubMed.
- H. Peng, S. Xie, J. Park, X. Xia and Y. Xia, J. Am. Chem. Soc., 2013, 135, 3780 CrossRef CAS PubMed.
- M. Jin, H. Liu, H. Zhang, Z. Xie, J. Liu and Y. Xia, Nano Res., 2011, 4, 83 CrossRef CAS PubMed.
- H. Zhou, H. Wu, J. Shen, A. Yin, L. Sun and C. Yan, J. Am. Chem. Soc., 2010, 132, 4998 CrossRef CAS PubMed.
- M. Cargnello, V. V. T. Doan-Nguyen, T. R. Gordon, R. E. Diaz, E. A. Stach, R. J. Gorte, P. Fornasiero and C. B. Murray, Science, 2013, 341, 771 CrossRef CAS PubMed.
- Y. Zuo, X. Huang, L. Li and G. Li, J. Mater. Chem. A, 2013, 1, 374 CAS.
Footnote |
† Electronic supplementary information (ESI) available: XPS, BET analyses of the as-obtained Pd@CeO2 core@shell nanospheres; TEM images of other control experiments, including using KCl and KI instead of KBr to synthesize Pd–CeO2 system; using KCl and KI to induce the forming of Au@CeO2 and Pt@CeO2, respectively. See DOI: 10.1039/c4sc03854a |
|
This journal is © The Royal Society of Chemistry 2015 |
Click here to see how this site uses Cookies. View our privacy policy here.