DOI:
10.1039/D3VA00134B
(Critical Review)
Environ. Sci.: Adv., 2023,
2, 1340-1350
Application of a differential technique in laser-induced fluorimetry/pulsed LED-fluorimetry: simple and reliable analysis of uranium raw materials in the nuclear fuel cycle – a mini-review
Received
16th May 2023
, Accepted 22nd August 2023
First published on 22nd August 2023
Abstract
Application of a differential technique in laser-induced fluorimetry/pulsed LED-fluorimetry is recommended for routine accurate determination of uranium over a wide range of concentrations, based on the comparison of the response of the standard with a sample of similar but unknown concentration on the same sample weight basis. In this approach, the procedure of elimination of interference by simple dilution of the sample has the distinct advantage of being quick and very simple to perform because it does not require any chemical preparation or solvent extraction. It is a self-standardized methodology of measurement and guarantees the quality of an analytical result (accuracy, high precision, reliability, comparability, and traceability). Application of a differential technique in laser-induced fluorimetry/pulsed LED-fluorimetry will be useful for the analysis of uranium in ores, certification of reference materials, borehole core assay, beneficiation product analysis, and other diverse applications in the entire nuclear fuel cycle worldwide.
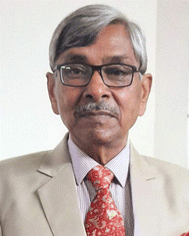 D. P. S. Rathore | Dr D. P. S. Rathore, FICS, FRSC, M-ACS, IUPAC-Affiliate Member, PhD Chemistry (1981), A.M.U., Aligarh, India, is Former Scientific Officer-G at the Atomic Minerals Directorate for Exploration and Research, Department of Atomic Energy, India. Dr Rathore has served in different capacities for 34 years, as Incharge, in different regional chemical laboratories, as Coordinator, mobile geochemical laboratories in various parts of the country and was involved in providing the quick feedback of analytical data support for the various ongoing uranium exploration projects of his directorate. His field of research interest/expertise is metal complexation, spectrophotometric determination and metallochromic indicator synthesis, chelating polymeric resins, optical fluorimetry, laser-induced/LED fluorimetry, reference measurement procedures and geostandard certifications. He is actively involved as a reviewer for more than 30 international journals. |
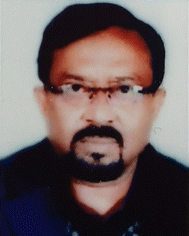 P. K. Tarafder | Dr Pranab Kumar Tarafder is an Indian Nuclear Scientist who served in the Atomic Minerals Directorate for Exploration & Research of the Department of Atomic Energy, Government of India for more than 33 years in different capacities. He has vast experience in the field of Analytical Chemistry and research. He has published more than 100 research papers in various international journals of repute, and authored a technical book. Besides, he has supervised about a dozen MSc dissertation studies, and three PhD thesis studies. He is the recipient of a few national awards and a few international accolades. He had reviewed more than 50 research papers so far. He is an Editorial Board Member of a few international journals. |
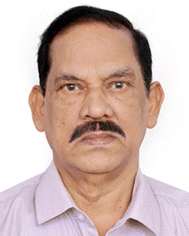 V. Balaram | Dr V. Balaram, Former Emeritus Scientist at the Geochemistry Division, CSIR – National Geophysical Research Institute, Hyderabad-500 007, India carried out studies in geochemistry, mineral exploration, spectroscopy, environmental chemistry, and climate change. He has over 330 publications in international peer-reviewed journals, with 7355 citations (h-index 44 & i 10-index 139) and guided 5 PhD students. He is the recipient of several prestigious national and international awards such as the “National Geoscience Award” from the Government of India, New Delhi (2000). His name is included in Stanford University's new list of the top 2% of Scientists in the World in 2020, 2021 & 2022. |
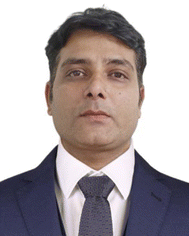 M. Mishra | Mr Manoj Mishra has completed his engineering with electronics and communication in the year 2007 and joined Quantalase in the year 2008 as an Electronics Engineer. He is currently working as a Technical Manager in Quantalase Enterprises Private Limited, Indore, India. He has been deeply involved in various planning and development activities of the company. He is taking care of the production of existing products as well as new development. His expertise and area of interest is in Embedded Systems and Instrumentation. |
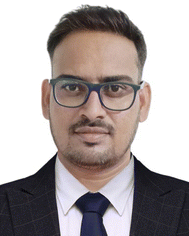 J. Pari | Mr Jaiprakash Pari has completed his Bachelor of Engineering (B.E.) Degree in Electronics & Communication from Rajiv Gandhi Proudyogiki Vshwavidyalaya, Bhopal, India (https://www.rgtu.net) in June 2009. He is presently working in Quantalase Enterprises Private Limited as a Manager and is actively involved in the production of existing instruments as well as in research and development activities. His expertise and area of interest is in analog circuits, instrumentation and optics. |
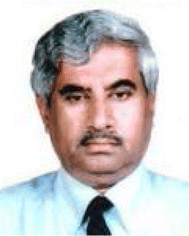 A. G. Bhujle | Mr Ashok G. Bhujle is a Former Senior Scientific Officer SO/H+ from 12th batch of training school (1968–69) of BARC, Mumbai, Head of Laser Instrumentation and Electronics Division of Raja Ramanna Centre for Advanced Technology, Department of Atomic Energy, India, and presently, Director of Quantalase Enterprises Pvt. Ltd., Indore, India. |
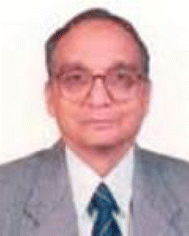 D. D. Bhawalkar | Dr Dilip D. Bhawalkar is Former Distinguished Scientist, and founder Director of Raja Ramanna Centre for Advanced Technology, Department of Atomic Energy, Indore, India. Dr Bhawalkar received the S.S. Bhatnagar Award (1984), UGC National Lecturer (1984), Goyal Award for Physics (1997), and Firodia Award (2000). He was awarded Padam Shree by the Government of India (2000). He was elected a Fellow of the Indian Academy of Sciences, Bangalore; National Academy of Sciences, India; Optical Society of USA, and Foreign Fellow of the Russian Academy of Natural Sciences. He is presently Director of Quantalase Enterprises Pvt. Ltd., India. |
Environmental significance
A simple, rapid, cost-effective, eco-friendly, comparable and traceable method for uranium determination is critically evaluated and presented. It is a self-standardized methodology of measurement and guarantees the quality of an analytical result. In this approach, the procedure of elimination of interference by simple dilution of the sample solution using a push-button pipette has the distinct advantage of being quick and very simple to perform because it does not require any chemical preparation or solvent extraction.
|
1. Introduction
Uranium, thorium and plutonium are the basic elements for utilization of “nuclear fission” energy.1 On average, the earth's crust contains nearly 4 mg kg−1 uranium, 12 mg kg−1 thorium and practically no plutonium. Natural uranium has two main isotopes, 238U (99.3%) and 235U (0.7%). 235U is the only naturally occurring ‘fissile’ material. The extra available neutrons after maintaining the chain reaction are utilized in transmuting the naturally occurring 238U and 232Th isotopes to produce man-made fissile isotopes 239Pu and 233U, respectively. The fission energy is utilized in a nuclear power reactor for generation of electricity. The fissile and fertile materials are used only for generating fission energy and additional fissile materials respectively, while fossil fuels like coal or oil have several applications other than generating energy. Uranium is an element of strategic importance for producing nuclear energy for sustainable growth worldwide.
Uranium is nearly ubiquitous,2–4 due to its polyvalence (+4, +6), large atomic radius (0.97 Å), high chemical reactivity, relative solubility of U(VI) compounds in aqueous solution, and relative insolubility of U(IV) compounds. Uranium forms many compounds, enters into the structure of many minerals and disperses readily. Under oxidizing conditions, hexavalent uranium appears as the uranyl ion, UO22+(VI), which is linear and polar in nature. The uranyl ion is very stable, maintains its identity through many chemical transformations, as is found in many secondary uranium minerals.
There are many methods for the determination of uranium in geological materials from uranium producing industries:5 gravimetric, volumetric, spectrophotometric, fluorimetric, radiometric and XRF analysis. Choice of a particular method depends primarily on the nature of the sample being analysed and on the amount of uranium present in it. In a conventional fluorimetric method for uranium determination,6,7 after solvent extractive separation of uranium from the accompanying matrix, the liquid sample containing the uranium compound is first evaporated carefully to dryness and the residue is fused at a high temperature in a muffle furnace or muffle oven with a carbonate-fluoride flux to produce a solid disc. The disc is then placed in an optical fluorimeter where it is illuminated by ultraviolet light to cause the uranium present to fluoresce. This conventional fluorimetric method for the analysis for uranium in rock sample solutions suffers from lack of sensitivity, limited precision, and complicated time consuming preparative chemical procedures using solvent extractive separation of uranium. All other methods require isolation/separation of uranium from the accompanying matrix under the conditions by using selective reagents, adjusting pH conditions, using masking agents, separations (if any) using solvent extraction, etc. So far, all reported methods for uranium determination have an adverse effect on the environment and need immediate replacement.
Laser-induced fluorimetry/pulsed LED-fluorimetry is a well-documented, highly sensitive, and versatile technique for the determination of uranium in water samples at μg L−1 levels,4,8 and is at the same time more challenging due to a wide variety of water samples differing in total dissolved salts including saline water and fluoride content as well as due to the practical impossibility of preserving natural water samples.9–18 In contrast, mineralised rock sample solutions are easier to analyse through the application of a differential technique in laser-induced fluorimetry/pulsed LED-fluorimetry for their uranium content due to their easy preservation over a period of time/absence of instability problems.
There is great pressure to change or replace the age old conventional time-consuming solvent extractive methods for uranium determination in diverse matrices, owing to the skilled manpower necessity, non-eco-friendly, generated analytical wastes, non-green analytical methodology and rising costs, even in developed countries.19–21
There are three methods of measurement in an instrumental technique,22 namely (1) calibration method, (2) standard additions, and (3) differential technique. All these methods have a significant bearing on the reliability, cost-effectiveness and traceability of measurement data. The applications of all these methods hold good when a linear relationship between signal response and concentration of analyte is established. Truly, if proper care is not taken, the results obtained will depend on the vagaries of the analyst.
In the calibration method,23 to establish the calibration graph using pure diluted working standard solutions it is assumed that there is no ‘matrix effect’ i.e., reduction or enhancement of the signal by other matrix components accompanying the analyte of interest in the sample taken for analysis. The calibration method is generally applied in spectrophotometric/fluorimetric determinations after the isolation of the analyte of interest from the accompanying matrix under the conditions by using selective reagents, adjusting pH conditions, using masking agents, separations (if any) using solvent extraction, etc. The system should be free from matrix effects. Moreover, the calibration method is based on two assumptions: (1) the errors in the calibration experiment occur only in the instrumental response plotted on the vertical Y-axis. (2) The magnitude of errors in vertical Y-axis values is independent of the analyte concentration. Both these assumptions have their limitations. In case of the first assumption, the stock solution of standards of the analyte of interest can be made up with an error of ca. 0.1% or better, while errors associated with the working standard solutions used for plotting the calibration graph may vary appreciably. The errors in the working standard solutions (diluted solutions) will depend on the type of glass wares (pipettes, volumetric flasks) used for dilutions, acidity, concentration levels and on storing/aging etc. The second assumption is often unlikely to be true because the relative errors in measurement are constant while the absolute errors will increase as the analyte concentration increases. The total errors in the determination of analyte mass thus depend on the precision of the method as well as on the number of steps involved. Direct methods having high precision and involving minimum steps are more desirable to obtain the reliable analytical data.
The method of ‘standard additions’ is a fundamental method for analyte determination because it minimizes the errors produced by composition and physical properties of the sample solution (without any separation of the analyte of interest from the accompanying matrix).23–25 The method of standard additions has been recommended for the determination of uranium in water samples using laser-induced fluorimetry.26 Truly, it is a simple means of verifying analytical values obtained by analytical working curves without the need for using another analytical instrumental method as a reference method. In fact, it is a self-calibration or auto-standardization method and is well documented in the literature for the determination of uranium in water samples.
The classical example of the differential technique is the accurate and precise spectrophotometric determination of major oxides,27–29 like SiO2 and Al2O3. In the differential technique, the precision of results is materially improved, if a relative rather than absolute concentration is determined. The differential technique is based on the comparison of the fluorescence of the standard with a sample of similar but unknown concentration on the same sample weight basis, by the use of H3PO4–NH4H2PO4 as a fluorescence enhancing reagent. In the differential technique, the error in measurements is confined to the difference in two signal response measurements. Under the conditions for the use of the differential technique, in which the fluorescence of the unknown concentration in the cuvette is matched with that of the standard of accurately known concentration using H3PO4–NH4H2PO4 as a fluorescence-enhancing reagent, all samples including at least three independent standard samples/certified reference materials are subjected to exactly the same procedures and measurement steps such that the whole methodology is checked,30 which amounts to a fluorimetric titration. In this way, the accuracy and precision of the ‘differential technique’ were found to be comparable with those of the differential spectrophotometric technique as well as with those of classical titrimetric and gravimetric methods. The differential technique with titrimetry thus gives rise to an absolute methodology30 based on the use of analytical chemical standards or certified reference materials. The use of certified reference materials as standards ensures calibration, control, and optimization of the quality of analytical data.5,31–35 The application of the differential technique holds good when a linear relationship between the analyte response and concentration is established. The system must be free from interference effects.19,20 The use of at least three independent standards of accurately known concentration in differential technique further minimises the total errors associated with dilution steps, measurement of signal, and overall errors associated with the method of measurement.19,20 The differential technique is like applying indirectly the ‘method of least squares’ while obtaining the more reliable common factor of slope.35 In this way, the differential technique using reference standards guarantees the quality of an analytical result (accuracy, high precision, reliability, comparability & traceability). It is a self-standardized and an absolute methodology of measurement.
In this review article, which is partly based on our e-poster presentation in the URAM-2023 International Conference at IAEA Vienna, Austria,36 an overview of the recent advances in laser-induced fluorimeter/pulsed LED-fluorimeter technologies for the determination of uranium in ores, certification of reference materials, borehole core assay, beneficiation product analysis, and other diverse applications in the entire nuclear fuel cycle worldwide for the sustainable growth of the nuclear industry is presented and critically evaluated.
2. Experimental
Reagents
Fluorescence enhancing reagent.
An acidic buffer mixture of H3PO4 (1 M) and NH4H2PO4 (2.17 M) has been used as a fluorescence-enhancing reagent.19,20 The reagent was prepared by dissolving 250 g of ammonium dihydrogen phosphate (AR, Merck) in distilled water and adding 68 ml phosphoric acid (AR, Merck) 85% wt in H2O (99.99% trace metal basis) and making up to 1 litre with distilled water.
Aqueous standard U3O8 (1 mg ml−1) stock solution.
An aqueous standard stock solution of uranyl ions of 1 mg per ml was prepared from U3O8 or uranyl nitrate of analytical-reagent grade having 10% HNO3.20 The concentration of uranium in this stock solution was verified using the method of Davies & Gray.37
The recommended primary standard is a 1 mg ml−1 (1000 ppm U) uranyl nitrate solution made up with 10% HNO3 (2.11 g UO2(NO3)2·6H2O per litre, AnalaR Grade (BDH)) or U3O8 after ignition of UO2(NO3)2·6H2O at 850–900 °C till constant weight (1 g U3O8 per 1000 ml in 10% HNO3).
Reference standards.
IAEA low grade uranium ores, Torbernite (Australia) – S1 (0.471% U3O8), Torbernite (Spain) – S2 (0.313% U3O8) and Carnotite (USA) – S3 (0.418% U3O8), were used as standard reference samples. The solutions of standard reference samples were prepared as per the prescribed procedure. The concentrations of uranium in S1, S2 and S3 are 0.00942, 0.00626 and 0.00836 mg ml−1 U3O8 in 10% HNO3, respectively.
Alternatively, 0.01 mg ml−1 U3O8 was prepared by diluting 1 ml of the 1 mg ml−1 U3O8 to 100 ml maintaining 10% HNO3. Similarly, 0.005 mg ml−1 U3O8 was prepared by diluting 1 ml of the 1 mg ml−1 U3O8 to 200 ml maintaining 10% HNO3. These solutions serve as reference standards.20
All other chemicals were of analytical-reagent grade. Distilled water was used throughout.
Rock sample solution preparations.
A sample solution of powdered rock sample/standard uranium samples/certified reference materials (0.5 g, 150–200 mesh) is obtained by repeated evaporation with HF–HNO3 and then evaporation with HNO3 (to remove F−). The sample is digested with HNO3 and boiled for 15–20 min to get a clear solution. If the sample solution is not clear, a few drops of H2O2 are added and then boiled vigorously for a further 20–30 min to decompose the excess H2O2. If a little unattacked residue remains, it is filtered off, washed and brought into solution by sintering and fusing with a minimum amount of a mixture of sodium fluoride and potassium pyrosulphate. The melt, after cooling, is dissolved in nitric acid, and made up to 100 ml in a calibrated flask. The final solution is in 10% HNO3
3. Results and discussion
Available technologies
Laser based instrumental techniques.
Laser based instrumental techniques, employing fluorimeters, spectrofluorimeters and phosphorimeters, have evolved during the past three decades, utilising the half-life of phosphorescent uranyl compounds and their decay as a diagnostic method for the low cost, simple and direct determination of uranium at μg L−1 levels in natural water samples for hydro-geochemical reconnaissance surveys for uranium. These are critically evaluated and discussed in depth by Rathore.21
Laser-induced fluorimetry.
In this technique,4Fig. 1, the heart of the system is the light source – a compact sealed nitrogen laser, which emits ultraviolet (337 nm) short lived pulses (3–4 × 10−9 s) at a repetition rate of 15 pulses per second. A laser is used in preference to other sources of ultraviolet light because the resultant pulse is intense yet self-terminating, monochromatic, and highly directional; the full output power is thus easily directed and focused on the sample cell. The phosphorescence in the cell is detected by a photomultiplier tube (PMT) isolated by a green-transmitting filter (480–540 nm). This technique comprises the steps of exciting the uranyl ion in the sample by projecting electromagnetic energy of suitable wavelengths and measuring the decay with time of the uranium phosphorescence after the termination of the incident electromagnetic radiation.
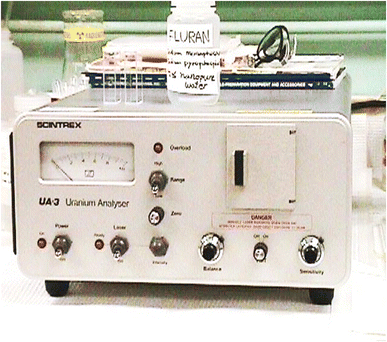 |
| Fig. 1 UA-3 uranium analyzer (SCINTREX, Canada). | |
Rathore et al.19 studied acidic buffer mixtures containing different dihydrogen phosphates plus phosphoric acid as uranyl fluorescence-enhancing reagents in laser fluorimetry. In laser-induced fluorimetry, the choice of the fluorescence-enhancing reagent is of great importance and depends mainly on the matrix composition (presence of quenching and/or absorbing species) and uranium concentration level. The choice of an appropriate fluorescence enhancing reagent for different types of sample matrices is essential. A fluorescence enhancing reagent of pH ∼ 7 is most suitable for water samples. For rock samples, an acidic buffer has distinct advantages over others. The authors utilized the technique used in differential spectrophotometry for selecting the appropriate reference standard concentration to obtain the maximum precision possible in any given analysis and introduced the differential technique in laser-induced fluorimetry. A simple one or two-step dilution of sample solution with distilled water removes accompanying matrix effects (a linear decrease in uranium content but an exponential decrease in quenching effects). The application of the differential technique in laser-induced fluorimetry/pulsed LED-fluorimetry is free from high concentration of nitric acid/pH effects and accompanying prefilter/post filter matrix effects because of microlitre sample volumes as per the prescribed procedure.19,20 It was interesting to note that in practice, the accuracy and precision of the differential technique were found to be comparable with those of the differential spectrophotometric technique as well as with those of classical titrimetry and gravimetric methods.
The multiple dilution errors introduced by using class-A glass pipettes19–22,38–40 are additive and are not very suitable for multiple or large dilutions because of their inherent inaccuracies. The single push-button microlitre pipettes40 combined with superior accuracy and precision (inaccuracies of ±0.6% and imprecision < 0.2% over the volume range from 100 to 1000 microlitres) with ease of use for applications, where we cannot afford to make mistakes, are most suitable for single step large dilutions, thus minimizing the multiple dilution errors. Samples containing higher concentrations of uranium are diluted using the rule of 10-fold dilution.19
Basically, application of the differential technique in laser/LED fluorimetry is based on three main aspects: (1) interference removal of associated ions: by simple dilution using a push-button pipette,40 (2) fluorescence enhancing reagent: an acidic buffer mixture19 of H3PO4 (1 M) and NH4H2PO4 (2.17 M) has been used as a fluorescence-enhancing reagent (pH ∼ 2.5), and (3) differential technique as a method of measurement.19–22
Application of the differential technique in laser-induced fluorimetry/pulsed LED-fluorimetry for the direct determination of uranium is recommended for routine accurate determination of uranium over a wide range of concentrations in borehole core samples, ores, concentrates and other diverse matrices in the nuclear fuel cycle. By taking advantage of the high sensitivity of laser-induced fluorimetry/pulsed LED-fluorimetry, the interferences from the associated and accompanying elements are eliminated by simple one or two-step dilution of the sample solution using Eppendorf/push-button microlitre pipettes, thereby bringing the concentration of uranium within the operational range of the instrument, followed by measurement with the differential technique in laser-induced fluorimetry/pulsed LED fluorimetry using a more suitable acidic buffer mixture of H3PO4–NH4H2PO4 (pH ∼ 2.5, H3PO4, 1 M and NH4H2PO4, 2.17 M) as a fluorescence-enhancing reagent. The experiments are designed in such a way that for very diluted sample solutions, prefilter (species absorbing at the laser wavelength of 337 nm, LED wavelength of excitation at 400 nm) and post filter (species absorbing at the maximum fluorescence wavelengths, 480–560 nm) effects are negligible (verified by spectrophotometry). Beyond doubt, the differential technique is a self-standardized methodology traceable to international standards.
Reproducibility of the method.
The differential laser-induced/LED fluorimetry method has been evaluated using standards, SY-2, SY-3, reference uranium ore, BL-2a, low-grade uranium ore-IAEA reference samples, 1 mg ml−1 U3O8 standard solution and core samples of diverse matrices. The relative standard deviation of the method was 0.3–0.5% in nine replicates for 0.04–3.4% U3O8 in mineralized silicate rock samples19 and 0.5–0.9% at 18.1, 36.2, 61.2, and 99.6% U3O8 in concentrates and mineralized grab samples.20 It was found that the precision of the differential technique is within the acceptable ‘pure geochemistry’ type of analysis (RSD ∼ 1.0%) in samples containing more than 0.01% uranium and the measurement remains unaffected by the choice of the laser-induced/LED fluorimeter instrument. The results are in good agreement with the published data and those obtained by conventional fluorimetry and other methods, and of comparable precision to those obtained by titrimetric assay.19–22 A comparative analytical performance of the application of the differential technique in laser-induced fluorimetry with other methods of uranium determination is summarized in depth20 and further modified in Table 1. The estimated relative expanded uncertainty values obtained for uranium content in standard IAEA samples are S1, 0.04 g kg−1, S2, 0.06 g kg−1, S3, 0.04 g kg−1, and for S4, 0.10 g kg−1, respectively.41 These low uncertainty values obtained for uranium show high metrological quality of the application of the differential technique in laser/LED fluorimetry (Table 2).
Table 1 Comparison of the analytical performance of the application of the differential technique in laser/LED fluorimetry with others for the determination of uranium
Conventional fluorimetry1 |
Solvent-extractive spectrophotometry1 |
Classical titrimetric and gravimetric methods1 |
Application of the differential technique |
(1) Time consuming. Multiple steps |
(1) Time consuming. Multiple steps |
(1) Time consuming. Multiple steps |
(1) Analysis time is 1–2 minutes per sample |
(2) Separation step is mandatory |
(2) Separation step is mandatory |
(2) Separation step is required except in Davies & Gray's method |
(2) No separation step is needed. Simple 1 to 2 step dilution of sample solution using a push-button pipette |
(3) Less precision |
(3) Less precision |
(3) High precision |
(3) High precision |
(4) Moderate accuracy |
(4) Moderate accuracy |
(4) High accuracy |
(4) High accuracy |
(5) Applicable up to 500 ppm U3O8 |
(5) Applicable to more than 500 ppm to 1% U3O8 |
(5) Applicable to more than 5% U3O8 |
(5) Applicable to a dynamic range of concentrations (>0.01 U3O8; RSD < 1%) |
(6) Quantity of sample needed is moderate but analytical waste generated is maximum |
(6) Quantity of sample needed is moderate but analytical waste generated is maximum |
(6) Quantity of sample needed as well as analytical waste generated are maximum |
(6) Quantity of sample: microvolumes, analytical waste generated is minimum |
(7) Cost of chemicals for analysis per sample after solution preparation is 6 times that of the DT-LIF method |
(7) Cost of chemicals for analysis per sample after solution preparation is 5–10 times that of the differential technique |
(7) Cost of chemicals for analysis per sample after solution preparation is 20 times that of the DT-LIF method |
(7) Cost of chemicals for analysis per sample after solution preparation is lowest |
(8) Uses the calibration method which is based on assumptions |
(8) Uses the calibration method which is based on assumptions |
(8) Uses weighing or volume by difference method. Hence, more reliable |
(8) Differential technique using reference standards guarantees the quality of an analytical result. It is a self-standardized method. Results are traceable |
Table 2 Measurement of uncertainty in standard samples through the application of the differential technique in laser/LED fluorimetry
Sample |
% U3O8 |
% U3O8 |
Us(U3O8) |
Relative expanded uncertainty, coverage factor k = 2 (95% confidence interval), % |
Founda |
Recommended valueb |
Relative combined standard uncertainty |
The results are an average of eight determinations ± S.D.
Results by the following methods were used to evaluate the uranium content: S 1 av. photo: thio, 0.473; dibenzoyl methane, 0.470; vol. cerium sulphate titration, 0.469. S2 av. photo: thio, 0.314; arsenazo, 0.313; vol. cerium(IV) sulphate quant., 0.313. S3 av. photo: thio, 0.420; dibenzoyl methane, 0.419; arsenazo, 0.0.415; vol. cerium sulphate titration, 0.417. S4 av. photo.: thio, 0.377; DBM, 0.376; arsenazo, 0.372; vol. cerium(IV) sulphate quant.%: 0.377.
|
S1 IAEA sample |
0.470 ± 0.002 |
0.471 |
0.002 |
0.004 |
0.4 |
S2 IAEA sample |
0.312 ± 0.001 |
0.313 |
0.003 |
0.006 |
0.6 |
S3 IAEA sample |
0.416 ± 0.002 |
0.418 |
0.002 |
0.004 |
0.8 |
S4 IAEA sample |
0.372 ± 0.003 |
0.375 |
0.005 |
0.010 |
1.0 |
Other elements present |
Elements: % |
Al2O3 |
CaO |
CuO |
Fe2O3 |
MgO |
Na2O |
SiO2 |
B2O3 |
BaO |
BeO |
P2O5 |
K2O |
S1 |
6.4 |
0.06 |
0.04 |
3.6 |
0.39 |
<0.1 |
85.0 |
0.04 |
0.03 |
— |
<0.7 |
1.1 |
S2 |
4.5 |
0.078 |
0.24 |
10.0 |
0.15 |
0.07 |
76.0 |
0.02 |
0.03 |
<0.003 |
— |
— |
S3 |
6.0 |
0.55 |
<0.02 |
0.5 |
1.2 |
1.05 |
>80 |
<0.003 |
0.04 |
— |
<0.1 |
2.5 |
S4 |
16.5 |
0.52 |
0.02 |
12.8 |
10.6 |
0.18 |
58.0 |
0.05 |
0.03 |
— |
0.54 |
1.4 |
% |
Cr2O3 |
Ga2O3 |
Li2O |
MnO |
NiO |
PbO |
SnO |
TiO2 |
V2O5 |
CoO |
ThO2 |
S1 |
0.01 |
0.002 |
0.006 |
0.03 |
0.002 |
0.02 |
— |
0.5 |
0.03 |
— |
10 ppm |
S2 |
<0.001 |
0.002 |
0.06 |
0.02 |
<0.001 |
0.008 |
0.003 |
0.04 |
0.008 |
— |
10 ppm |
S3 |
<0.004 |
— |
— |
<0.09 |
— |
— |
— |
0.33 |
0.58 |
— |
4 ppm |
S4 |
0.006 |
0.005 |
0.02 |
0.35 |
0.004 |
0.1 |
— |
0.81 |
0.08 |
0.004 |
5 ppm |
Linearity of response and fitting a straight line by the least squares method.
In order to check the linearity between percentage U3O8 and fluorescence reading, a graph is plotted from the analysis data of samples. It is a straight line graph. The method of least squares35 was used to calculate the fitted values of fluorescence readings. These fitted values are very close to the observed values, indicating negligible errors.19 The use of at least three independent standards/certified reference materials in the differential technique further minimises the total errors associated with dilution steps, measurement of signals, and overall errors associated with the method of measurement.19–22 The signal response of these independent standards should be in agreement with each other within the reported uncertainty limits/relative standard deviation of the differential technique.
Percentage composition range of mineralised rock samples of diverse matrices
Mineralised rock samples of diverse matrices from different uranium exploration projects having the percentage composition in the range were studied: SiO2, 49.95–53.21, TiO2, 1.43–2.8, Al2O3, 9.75–13.43, Fe2O3, 0.92–4.45, FeO, 0.43–10.73, MnO, 0.01–0.24, MgO, 0.07–3.57, CaO, 0.10–6.17, Na2O, 0.13–2.91, K2O, 0.96–1.76, P2O5, 0.05–0.35%, and in μg g−1 Zr, 125–143, Sr, 62–141, Rb, 41–102, Ba, 245–1001, Y, 20–25, Ni, 41–60, Co, 37–56, Cr, 21–48, V, 205–285, Cu, 53–118, Pb, 102–160, ThO2, <100. The samples containing 0.01–0.55% U3O8 can be analysed directly in a 0.5 g sample after 100 ml dilution basis and then taking a 100 μl aliquot as per the prescribed procedure for measurement of fluorescence intensity. The sample solution is suitably simply diluted in one or two steps with distilled water using Eppendorf single push-button microlitre pipettes to bring the concentration of uranium within the low operational range of the instrument, which also brings the concentration of quencher impurities well below the tolerance limits of the method in the final solution for subsequent fluorescence measurements. The application of the differential technique is a unique application to the real samples, tested, evaluated and applied to a large number of samples of diverse matrices from different ongoing uranium projects over a period of more than 20 years in different regional chemical laboratories.
Pulsed LED-fluorimetry/fluorescence spectrophotometry.
Recent advances in narrow and broad-band LED light sources from the far UV to the NIR wavelength regions have also opened up the flexibility for additional designs, electronics, and instrumentation. With the addition of new fluorescence reagents42 or probes/sensing materials,43–45 advancements in the technologies have continued in high performance qualification and portable instrumentation, and have a promising future.46 Recently, Balaram and Sawant47 reviewed available portable analytical instruments in mineral exploration studies. With the advent of bright and more stabilized LED lights, a pulsed LED-fluorimeter based on this excitation source has become available from Quantalase Enterprises Pvt. Ltd., Indore, India (http://quantalase.in/services).48
The excitation source was a bank of pulsed LEDs emitting at 400 nm (model LF-2,48Table 3). Several innovations were added to the instrument to make it more reliable, more versatile and easier to operate than the nitrogen laser fluorimeter. With LEDs in the visible range (400 nm) replacing lasers (337 nm) in the UV range, the tolerance levels of many associated interfering elements improved by almost 10 times in this new LED-based technique. Tolerance to pre-filters like Fe(III) has been found to be high (10 fold increase as compared to laser-induced fluorimetry). Same is the case with post filters like Mn, Cu, Cr etc. It was found that the tolerance to many ions has been enhanced by at least 10 times in LED fluorimetry in comparison to laser-induced fluorimetry. A pulsed LED-fluorimeter is an excellent substitute to the UA-3 laser-induced fluorimeter available in the market for uranium determination in diverse matrices in the nuclear fuel cycle including hydrogeochemical reconnaissance surveys for field and base laboratory investigations (Fig. 2).
Table 3 Technical specifications of LED fluorimeters, model LF-2
Pulsed UV LEDs (Light Emitting Diodes) emitting at 400 nm wavelength and with a suitable filter. 20 μJ or higher less than 1%, 5 years |
Detector – photomultiplier tube with a precision multilayer optical filter |
Analyte volume – 6 ml cuvette, made from ultra-low fluorescence fused silica |
Dynamic range: 0.1–1000 ppb (0.1–1000 micrograms per litre)
|
Accuracy: better than 10%. Can be significantly better if the instrument is used in a temperature-controlled environment |
Reproducibility: better than 5% |
Averaging: the average of 1280 measurements is used for calculation of uranium concentration
|
LED fluorimeter: Quantalase Enterprises Private Limited, Indore, India (http://quantalase.in/services) |
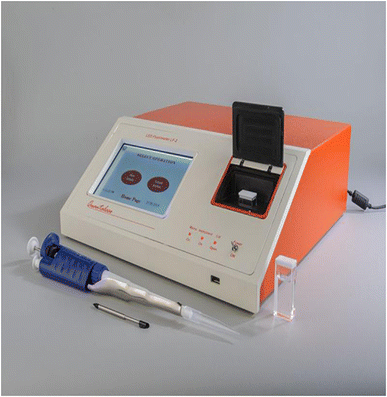 |
| Fig. 2 LED fluorimeter (model LF-2) (QUANTALASE, India). | |
Limitations and potential challenges associated with the differential technique.
The instrumental response was found to be stable and reproducible. The time needed for a uranium analysis is only 1–2 min. However, it was observed that at low laser intensity, the instrumental response fluctuates. If fluctuations persist, then the instrument should not be used for analysis. It requires replacement of the laser tube as recommended in the instruction manual of the instrument. In titanium rich grab sample matrices containing up to 32% TiO2 in 10% nitric acid, the data between methods differ by less than 0.5% relative to Davies and Gray's titration method. However, higher amounts of TiO2 in 10% nitric acid cause hydrolysis of sample solution. Even on hydrolysis due to higher titanium content, the data between methods differ by less than 2%, which may be attributed to variations in the uranium content and is discussed in depth.20 The application of the differential technique in laser/LED fluorimetry is recommended for routine accurate determination of uranium in mineralised rock samples over a wide range of concentrations >0.01% uranium. Merck make phosphoric acid was found to be most suitable with negligible process blank. There are other methods reported in the literature for trace uranium determination after separation from the accompanying matrix.49–52
Analytical eco-scale of the differential technique in the laser-induced fluorimetry technique.
An ideal approach to green analytical methodology would be to reduce the number of stages in a given analytical procedure. The differential technique in laser-induced fluorimetry is a technique with excellent green analysis grade in comparison to the conventional pellet fluorimetry with a lower eco-scale score.53
The inter-laboratory comparison.
The inter-laboratory comparison experiment (ILCE-5-2017) programme on the determination of uranium in various matrices by the differential technique in laser-induced fluorimetry/pulsed LED-fluorimetry was organized by the Chemistry Group, Atomic Minerals Directorate for Exploration & Research, Hyderabad. Most laboratories have reported highly precise results and the Z-scores are in the acceptable limits (Z-score −3 ≤ z ≤ 3).53 The Chemistry Group has applied this technique to various geological matrices like silicate rocks and mineralised rocks, core and high grade grab samples (up to 40% U3O8); various Nb–Ta LREE and HREE refractory minerals (columbite–tantalite (0.026 to 0.36% U3O8), monazite and xenotime (0.5% U3O8), zircon, garnet); and beneficiation products (like feed/leach liquors/residue and yellow cake (up to 75% U3O8)) and in high purity materials (100% U3O8) including validating certified reference materials (SY-2, SY-3, IAEA S-1 to S-4, IGS-33, 34 & 36, BL-2a, BL-5 ranging 0.028 to 7% U).53–55
Application of the differential technique in inductively coupled plasma emission spectrometry.
A comparative study for the determination of total concentration of uranium in borehole core samples using the differential technique in laser induced fluorimetry and ICP-OES has been carried out. This shows that the results of the measurements carried out without any chemical preparation or extraction by both the ICP-OES and ‘differential technique in laser-induced fluorimetry’ are not significantly different.56 The ICP-OES results obtained for the measurement of uranium in these diverse geological samples compare favourably by using the differential technique in laser-induced fluorimetry, as a ‘reference measurement procedure’. A relative reference measurement procedure for the determination of total mass fraction of uranium in mineralized rocks, ores and similar matrices through the application of the differential technique in inductively coupled plasma emission spectrometry at 409.014 nm wavelength is presented, based on the comparison of net reading or indication of reference ore solutions with a sample of similar but unknown mass fraction of uranium on the same sample weight or dilution basis. Under standard operating conditions of the instrument, ICP-OES measurements were performed at 409.014 nm wavelength after checking the instrument stability by a C–Ar test, robustness of plasma and linearity response using aqueous standard U3O8 solutions. The estimated relative measurement uncertainty values obtained using the results of replicate analyses (“top-down” approach) in the ICP-OES procedure for the mass fraction of uranium in low grade uranium ore IAEA samples and CANMET-reference uranium ore are S1, 0.0015; S2, 0.002; S3, 0.002; S4, 0.0015; and BL-2a, 0.001, respectively.57 Both approaches show low measurement uncertainty in the determination of the total mass fraction of uranium without any chemical separation or extraction steps using a differential technique in the ICP-OES measurement procedure and are comparable with the differential technique in laser-induced fluorimetry. The differential technique in the ICP-OES measurement procedure will be useful for the analysis of uranium in ores, certification of reference materials, borehole core assay, and other diverse applications in the nuclear fuel cycle57 and further highlights its unique potentiality.58
Differential technique in the laser-induced fluorimetry/pulsed LED-fluorimetry procedure.
Take 100 microlitre aliquots with an Eppendorf micropipette/push-button pipette of test samples (containing U3O8 concentration in the range from 0.01 to 0.55%) and three reference standard solutions (take at least one higher reference standard containing 0.07–0.08% U3O8), having 0.5 g sample to 100 ml dilution, separately into 25 ml calibrated flasks (i.e., final concentration of U3O8 when diluted to 25 ml volume is in the range from 2 to 110 ng ml−1), add 10 ml of an acidic phosphoric acid–hydrogen phosphate buffer of pH ∼ 2.5 as a fluorescence enhancing reagent and dilute to the mark with distilled water. Measure the fluorescence of the reagent blank prepared in a similar manner but containing no uranium. Then, measure the fluorescence of the solutions prepared as above.
Compute the results using the differential technique as follows19 (Fig. 3):
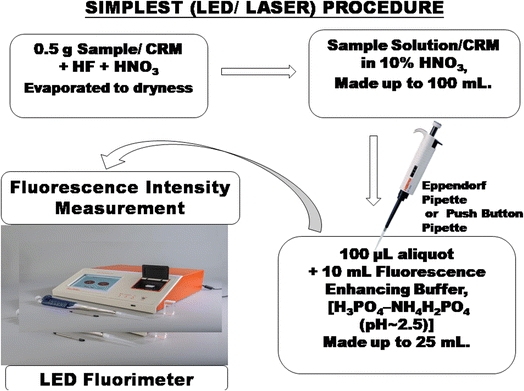 |
| Fig. 3 Differential technique in the laser-induced fluorimetry/pulsed LED-fluorimetry procedure. | |
(1) Compute a factor for each of the three comparison standards:
(2) Calculate an average from the three factors,
(3) Compute the percent U3O8 in the sample:
Average factor × net fluorescence of sample solution (on the same sample weight basis) = percent U3O8 |
Alternately, take suitable aliquots of uranium standard solution with full scale deflection/reading of the instrument for obtaining high precision. Measure the fluorescence of the reagent blank prepared in a similar manner but containing no uranium. Compute the results as described previously.20
4. Conclusions
Application of the differential technique in laser-induced fluorimetry/pulsed LED-fluorimetry has inherent high metrological quality. Application of the differential technique in laser/LED fluorimetry fulfills the basic essential requirements: accuracy and high precision, applicability to diverse sample matrices for wide applications in the entire nuclear fuel cycle, and simple, rapid, direct, and easy equipment calibration, method standardization and operation, eco-friendly, cost-effective, high sample throughput, comparability, and traceability. It is a self-standardized methodology of measurement and guarantees the quality of an analytical result.
Over the years, the differential technique in laser-induced fluorimetry/pulsed LED-fluorimetry will continue to grow for the reliable measurement of uranium to fulfill the mission and mandate of uranium processing industries in the entire nuclear fuel cycle for their sustainable growth worldwide.
This methodology may give direction in the area of microchemistry in developing new reliable methods for substance amount measurement by measuring at micro/nanogram masses using microvolumes (and lower) with the use of more sophisticated and compact advanced instrumentation more compatible with environmental friendly objectives in future.
Author contributions
All authors have contributed their best.
Conflicts of interest
There are no conflicts of interest to declare.
References
- R. Chidambaram and C. Ganguly, Curr. Sci., 1996, 70, 21–35 CAS.
-
Editorial Staff, Significance of Mineralogy in the Development of Flowsheets for Processing Uranium Ores, Technical Report Series No. 196, IAEA, 1980 Search PubMed.
-
B. Mason and C. B. Moore, Principles of Geochemistry, Wiley Eastern Limited, New Delhi, India, 4th edn, 1985, pp. 46–47 Search PubMed.
- J. C. Robbins, CIM Bull., 1978, 71, 61–67 CAS.
-
Analytical Techniques in Uranium Exploration and Ore Processing, Technical Reports Series no. 341, IAEA, Vienna, 1992, Chapters 3–4 and references cited there in Search PubMed.
-
G. R. Waterbury, Fluorimetric Analysis for Uranium in Natural Waters: HSSR Symposium, Grand Junction, Colorado, 1977 Search PubMed.
- P. K. Tarafder, P. Murugan, L. Kunkal and D. P. S. Rathore, J. Radioanal. Nucl. Chem., 2002, 253, 135–142, DOI:10.1023/A:1015880904919.
- V. Balaram, A. Rani and D. P. S. Rathore, Geosyst. Geoenviron., 2022, 1, 100043, DOI:10.1016/j.geogeo.2022.100043.
- D. P. S. Rathore, Explor. Res. At. Miner., 2013, 23, 207–215 CAS.
- D. P. S. Rathore, J. Radioanal. Nucl. Chem., 2013, 298, 717–719, DOI:10.1007/s10967-013-2432-z.
- D. P. S. Rathore, Hum. Ecol. Risk Assess., 2013, 19, 1147–1149, DOI:10.1080/10807039.2013.791148.
- D. P. S. Rathore, J. Geol. Min. Res., 2013, 5, 108–113 Search PubMed.
- D. P. S. Rathore, J. Radioanal. Nucl. Chem., 2013, 298, 721–723, DOI:10.1007/s10967-013-2445-7.
- D. P. S Rathore and V. K Garg, Int. J. Occup. Environ. Med., 2014, 5, 169–171 Search PubMed.
- D. P. S. Rathore, Curr. Sci., 2014, 106, 792 Search PubMed.
- D. P. S. Rathore, J. Radioanal. Nucl. Chem., 2014, 302, 745–746, DOI:10.1007/s10967-014-3392-7.
- D. P. S. Rathore, Chem. Sci. J., 2017, 8, 154, DOI:10.4172/2150-3494.1000154.
- D. P. S. Rathore, Adv Recycling Waste Manag, 2017, 2, 146, DOI:10.4172/2475-7675.1000146.
- D. P. S. Rathore, P. K. Tarafder, M. Kayal and M. Kumar, Anal. Chim. Acta, 2001, 434, 201–208, DOI:10.1016/S0003-2670(01)00850-9.
- D. P. S. Rathore and M. Kumar, Talanta, 2004, 62, 343–349, DOI:10.1016/j.talanta.2003.08.002.
- D. P. S. Rathore, Talanta, 2008, 77, 9–20, DOI:10.1016/j.talanta.2008.06.019.
- D. P. S. Rathore, Res. At. Miner., 2007, 17, 145–149 Search PubMed.
-
J. C. Miller and J. N. Miller, Statistics for Analytical Chemistry, Ellis Horwood PTR Prentice Hall, 1993, p. 117 Search PubMed.
-
Analytical Methods Committee, AMC Technical Briefs, No. 1, Royal Society of Chemistry, London, 2000, https://www.rsc.org/lab/rsccorn/amc Search PubMed.
-
B. Welz and M. Sperling, Atomic Absorption Spectrometry, Wiley-VCH, NY, 3rd edn, 1999, p. 501 Search PubMed.
-
J. C. Robbins, C. Castledine and W. Kostiak, Analytical Procedures for UA-3 Uranium Analysis -Applications Manual, Scintrex Ltd., Ontario, Canada, 1985 Search PubMed.
- L. Shapiro and W. W. Brannock, US Geol. Survey Bull., 1962, 1144A, 1–56 Search PubMed.
-
J. A. Maxwell, Rocks and Minerals Analysis, Interscience, New York, 1968, pp. 337–357 Search PubMed.
-
Z. Marczenko, Separation and Spectrophotometric Determination of Element, Ellis Horwood Ltd., New York, 1986, p. 66 Search PubMed.
-
M. Valcarcel, Principles of Analytical Chemistry-A Textbook, Springer, Berlin, Heidelberg, 2000, vol. 6, p. 263 Search PubMed.
- Absolute methods in analytical chemistry (Technical Report) (1995), prepared for publication by A. Hulanicki, International Union of Pure and Applied Chemistry, Analytical Chemistry Division,
Commission on General Aspects of Analytical Chemistry, Pure Appl. Chem., 1995, 67, 1905–1911 CrossRef CAS and references cited therein..
- Analytical Methods Committee, Analyst, 1995, 120, 29–34 RSC.
-
Uses of Certified Reference Materials, ISO Guide 33, International Organization for Standardization, Geneva, 1989 Search PubMed.
-
Use of NIST Standard Reference Materials for Decisions on Performance of Analytical Chemical Methods and Laboratories, NIST Special Publication 829, National Institute of Standards and Technology, Gaithersburg, MD, 1992 Search PubMed.
-
J. H. Pollard, A Handbook of Numerical and Statistical Techniques, Cambridge University Press, Cambridge, 1977, ch. 15 Search PubMed.
-
D. P. S. Rathore, P. K. Tarafder, V. Balaram, M. Mishra, J. Pari, A. G. Bhujle and D. D. Bhawalkar, Simple and Reliable analysis of Uranium in Mineralised Rocks, Ores, Beneficiation Products and other Diverse matrices: application of Differential Technique in Laser-induced Fluorimetry/Pulsed LED-Fluorimetry-A Green Methodology, E-poster presented, Track-3, International Symposium on Uranium Raw Material for the Nuclear Fuel Cycle: Innovation for Sustaining Future Resources and Production (URAM- 2023), IAEA Headquarters, Vienna, Austria, 8–12 May 2023 (Abstract ID#040) Search PubMed.
- W. Davies and W. Gray, Talanta, 1964, 11, 1203–1211, DOI:10.1016/0039-9140(64)80171-5.
-
Borosil Catalogue, Glass Works Ltd, Mumbai, 1999–2000, pp. 37–38, https://www.borosil.com.
-
Riviera Catalogue-cum-price list, Riviera Glass Pvt. Ltd., Mumbai, 1999–2000, pp. 30–34, https://www.rivieraglass.co.in.
-
Eppendorf Pipettes, https://www.pipettes.com.
- D. P. S. Rathore, M. Kumar and P. K. Tarafder, Accredit. Qual. Assur., 2012, 17, 75–84, DOI:10.1007/s00769-011-0838-2.
- M. Krishnakumar, G. Johnson and A. A. Patwardhan, J. Radioanal. Nucl. Chem., 2023, 332, 1795–1804, DOI:10.1007/s10967-023-08897-2.
- W. Liu, D. Xing, Z. Bai, Y. Wang, Z. Yang, L. Zhang, L. Xu, L. Zhang, L. Chen, Y. Li, D. Gui, J. Diwu, J. Wang, R. Zhou, Z. Chai and S. Wang, Environ. Sci. Technol., 2017, 51, 3911–3921, DOI:10.1021/acs.est.6b06305.
- W. Liu, Y. Wang, L. Song, M. A. Silver, J. Xie, L. Zhang, L. Chen, J. Diwu, Z. Chai and S. Wang, Talanta, 2019, 196, 515–522, DOI:10.1016/j.talanta.2018.12.088.
- Z. Zhang, D. Zhang, C. Shi, W. Liu, L. Chen, M. Yu, Juan Diwu, J. Li and S. Wang, Environ. Sci.: Nano, 2019, 6, 1457–1465, 10.1039/C9EN00148D.
-
Fluorescence Spectrophotometry, https://www.thermoscientific.com/MaterialsScience Search PubMed.
- V. Balaram and S. S. Sawant, Minerals, 2022, 12, 394, DOI:10.3390/min12040394.
-
Quantalase Enterprises Pvt. Ltd., Indore, India, Quantalase, https://quantalase.in Search PubMed.
- S. K. Pradhan and B. Ambade, J. Radioanal. Nucl. Chem., 2021, 330, 1621, DOI:10.1007/s10967-021-08009-y.
- S. K. Pradhan and B. Ambade, Anal. Sci., 2020, 36, 207–212, DOI:10.2116/analsci.19P216.
- S. K. Pradhan, B. Ambade and P. K. Tarafder, Appl. Radiat. Isot., 2020, 160, 109126, DOI:10.1016/j.apradiso.2020.109126.
- P. K. Tarafder, P. K. Ghosh and S. K. Pradhan, J. Radioanal. Nucl. Chem., 2017, 313, 353–360, DOI:10.1007/s10967-017-5334-7.
-
M. Kumar, and M. Krishnakumar, A Manual on Fluorimetric Determination of Uranium in Geomaterials, Released on 13th September, 2017, Diamond Jubilee (1957-2017)
of the Chemistry Group, Atomic Minerals directorate for Exploration and Research, Department of Atomic Energy, Hyderabad-500016, India, 2017 Search PubMed.
-
A. K Jain, A. Pandey, K. Bahuguna and M. Kumar, Decomposition of columbite-tantalite minerals by various fluxes for simple and direct determination of uranium using differential technique in LED induced fluorimeter, Published in, Proceedings of International Symposium on ‘Emerging Trends in Analytical Chemistry, 2016, ETAC-2016, pp. 25−27 Search PubMed.
-
A. Pandey, A. K. Jain, S. Prakash and M. Kumar, A simple, rapid and accurate determination of uranium in refractory minerals like zircon, garnet, monazite using LED based fluorimetry, Published in, Proceedings of International Symposium on ‘Emerging Trends in Analytical Chemistry, 2016, (ETAC-2016), pp. 39−41 Search PubMed.
- P. Murugesan, S. K. Jain, M. Kumar, P. K. Tarafder and D. P. S. Rathore, Explor. Res. At. Miner., 2013, 23, 137–144 CAS.
- P. Murugesan, C. R. Khorge, A. A. Patwardhan, M. Kumar, P. K. Tarafder and D. P. S. Rathore, J. Anal. At. Spectrom., 2014, 29, 1912–1917, 10.1039/C4JA00224E.
- D. P. S. Rathore, J. Anal. Bioanal. Tech., 2017, 8, 6, DOI:10.4172/2155-9872.1000389.
|
This journal is © The Royal Society of Chemistry 2023 |
Click here to see how this site uses Cookies. View our privacy policy here.