DOI:
10.1039/D2TA09338C
(Paper)
J. Mater. Chem. A, 2023,
11, 12902-12909
Engineering pore nanospaces by introducing aromatic effects in UiO-66 for efficient separation of light hydrocarbons†
Received
30th November 2022
, Accepted 2nd February 2023
First published on 14th February 2023
Abstract
Natural gas, as one of the most widely used clean energies, has attracted significant attention due to its abundance, safe nature, and low cost but requires further separation and purification to produce downstream commodities more efficiently. In this case, the simultaneous removal of ethane and propane from natural gas is very important but challenging due to their similar physical and chemical properties. Herein, a pore-nanospace-engineering strategy was developed to construct a series of UiO-66-type zirconium MOFs by introducing different aromatic linkers, i.e., UiO-66, UiO-66-Naph, DUT-52 and UiO-66-Anth, for natural gas separation and purification, revealing that the appropriate aromatic effect plays a vital role in the separation of the ternary C3H8/C2H6/CH4 gas mixture. Specifically, UiO-66-Naph exhibited the best C2/C3 (C2H6 and C3H8) light hydrocarbon separation potential from CH4, indicating that the introduction of naphthalene moieties not only effectively reduced the pore size but also provided a suitable aromatic pore-environment for C2H6/C3H8-framework interactions through dispersion and induction forces, which was corroborated by theoretical calculations and transient breakthrough experiments.
10th anniversary statement
On the occasion of the 10th anniversary of Journal of Materials Chemistry A, it is my great honour and pleasure to dedicate this work. Since 2010, I have been an Advisory Board member of Journal of Materials Chemistry. I have been involved in this journal and later Journal of Materials Chemistry A and enjoyed the service and knowledge acquisition in this community and contributed 50 publications. As one of my most favourite journal series, the Journal of Materials Chemistry family always attracts me because of its high-quality works, covering wide interdisciplinary fields in materials chemistry, especially the materials relevant to energy, catalysis, sensors and gas adsorption applications, which I am particularly interested in.
|
1. Introduction
Nowadays, the increasing severity of energy crisis and environmental pollution seriously threaten human survival and development together with the growth of global economy, owing to the burning of large amounts of fossil fuels.1,2 Therefore, it is essential to explore new clean energy sources as an alternative to the traditional fossil fuels, such as coal and oil. In this respect, natural gas, as a type of abundant energy, has presented great potential in the chemical industry and daily life, mainly because of its abundance, high calorific value, low air pollution (NOx, SO2, and smoke/particle), and low carbon dioxide emissions.3–5
It is well known that natural gas is mainly composed of CH4 (75–90%), C2H6 (0–20%), C3H8 (0.01–5%), and small amount of impurities (H2S, CO2, N2, water vapor, etc.).6–8 Notably, CH4 is not only a promising energy alternative but also an important chemical feedstock for the production of various downstream commodities.3,4,9 Additionally, C2H6 and C3H8 are important raw materials in the chemical industry.5,10,11 However, a small amount of C2H6 and C3H8 impurities can have serious influence on the conversion rate of CH4, cyclic steady state, and safety in CH4 storage, transportation, and utilization.12–14 Hence, it is highly indispensable to realize the efficient separation of C2H6 and C3H8 over CH4 from an industrial viewpoint.
The industrial separation of low-concentration C2–C3 light hydrocarbons from CH4 usually relies on cryogenic distillation technology at high pressure and low temperature, resulting in high cost and energy footprint penalty.15–17 Therefore, the development of cost- and energy-effective separation technology is of great importance.18,19 Recently, nonthermal-driven adsorption separation technology based on porous solid materials has attracted significant attention owing to its low cost and energy efficiency.20–25
Due to their high surface areas, designable structures and tunable functionalities, metal–organic frameworks (MOFs), as a class of porous solid materials constructed with metal ions/clusters and organic ligands through coordination bonds, have shown great potential in gas adsorption and separation.26–31 Among them, zirconium-based MOFs (Zr-MOFs) constructed with strong Zr–O bonds have been intensively exploited on account of their high thermal and chemical stability, which bestow them with great practical application potential in gas adsorption and separation. In particular, the UiO-66 family, constructed from saturatedly coordinated Zr-clusters and derivatives of terephthalic acid, has become a type of representative Zr-MOFs. A prerequisite for the effective separation of C2–C3 light hydrocarbons from CH4 based on MOFs is elaborate manipulation of their pore system because of the similar molecular size and chemical property of CH4/C2H6/C3H8, especially C2H6/CH4.32 To date, tremendous efforts have been devoted to developing highly effective MOF adsorbents through various strategies for purifying CH4 from a binary C2H6/CH4 or C3H8/CH4 gas mixture. However, the simultaneous removal of both C2H6 and C3H8 from ternary gases mixture in a one-step process and recovery of C3H8 are still industrially required but challenging.33–36
Herein, we developed a pore-nanospace-engineering strategy to optimize the pore-nanospaces for the separation of low-concentration C2–C3 from CH4 by constructing a series of UiO-66-type Zr-MOFs, UiO-66/66-Naph/66-Anth and DUT-52, in which the pore size, volume, surface and environment could be finely tuned by introducing different aromatic moieties (Fig. 1).37–39 Noteworthily, UiO-66-Naph was demonstrated to be the best candidate, exhibiting the highest C3H8/CH4 selectivity, CH4 productivity and C2H6/C3H8 capture capacity compared to the other three Zr-MOFs, owing to its appropriately reduced pore size and rich aromaticity (extensive π-electron system), which provided multiple interaction sites for C2H6 and C3H8. Furthermore, theoretical calculations confirmed that the optimized pore nanospace and surface facilitated C–H⋯π interactions in the van der Waals (vdW) region, which play a pivotal role for the challenging separation of C2–C3 light hydrocarbons from CH4.
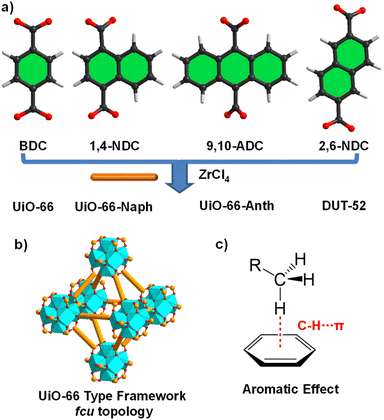 |
| Fig. 1 (a) Ligands of the four MOFs. The green color highlights the size and direction of the aromatic systems. (b) Structure of UiO-66 type frameworks with the ligands simplified as yellow sticks. (c) Schematic representation of aromatic effect toward RCH3via C–H⋯π interaction. | |
2. Results and discussion
Synthesis, phase purity and porosity
Four Zr-MOFs, UiO-66, UiO-66-Naph, UiO-66-Anth and DUT-52, were synthesized through the solvothermal reaction of ZrCl4 with the corresponding ligands H2BDC (terephthalic acid), H21,4-NDC (1,4-naphthalenedicarboxylic acid), H29,10-ADC (9,10-anthracenedicarboxylic acid) and H22,6-NDC (2,6-naphthalenedicarboxylic acid), respectively, in N,N-dimethylformamide (DMF) with the addition of a modulator (formic acid, trifluoroacetic acid or HCl) (Fig. 1a),32,40 forming isostructures with the fcu-net topology. The phase purity of the as-prepared bulk samples was confirmed by PXRD patterns (Fig. S1–S4 in the ESI,† respectively), matching well with the simulated patterns from reported structures.32,39 The thermal stability of the four MOFs was assessed by thermogravimetric analysis (TGA) and variable-temperature PXRD (VT-PXRD) patterns, demonstrating that they are stable up to 400 °C except for UiO-66-Anth, which is stable up to 300 °C (Fig. S9–S13,† respectively). Moreover, the chemical stability of the four MOFs was evaluated by treating the samples in hot water (60 °C for UiO-66 and 80 °C for the other MOFs) and aqueous solutions with different pH values (6 M HCl for UiO-66/66-Naph, 0.5 M HCl for DUT-52, 1 M HCl for UiO-66-Anth, pH = 12 for UiO-66/66-Naph/66-Anth, and pH = 10 for DUT-52), and then checked by PXRD and N2 (77 K) adsorption experiments, revealing that all the MOFs retained their structural integrity, which indicates their excellent robustness (Fig. S5–S8, S15–S18 and Table S1,† respectively).
N2 (77 K) adsorption measurements were performed to evaluate the porosity of the four MOFs, which all presented type I microporous adsorption isotherms (Fig. 2a and S19–S22,† respectively). The Brunauer–Emmett–Teller (BET) surface areas (SBET) and pore volumes follow the order of DUT-52 (1641 m2 g−1 and 0.70 cm3 g−1) > UiO-66 (1305 m2 g−1 and 0.47 cm3 g−1) > UiO-66-Naph (881 m2 g−1 and 0.40 cm3 g−1) > UiO-66-Anth (676 m2 g−1 and 0.32 cm3 g−1) (Table S4†), which is consistent with the order of the pore size distribution (PSD) calculated based on the DFT method, namely, DUT-52 (1.03 and 1.73 nm) > UiO-66 (0.86 and 1.11 nm) > UiO-66-Naph (0.55 and 1.14 nm) > UiO-66-Anth (0.57 and 1.01 nm), respectively. This demonstrates the effective pore-nanospace manipulation by introducing an aromatic system with different sizes and arrangements (Fig. 2b and Table S4†). It is worth noting that the introduction of more extensive π-electron systems not only endowed the UiO-66 family with more aromatic-rich pore-environment, thereby enhancing the C2H6/C3H8-framework interactions versus CH4, but also finely tuned the pore size, pore volume and pore surface, which may impact the separation of C2–C3 light hydrocarbons from CH4.
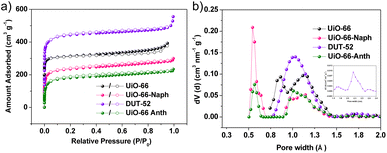 |
| Fig. 2 (a) N2 adsorption isotherms of UiO-66, UiO-66-Naph, DUT-52 and UiO-66-Anth at 77 K. Solid symbols: adsorption and open symbols: desorption. (b) Pore size distribution of the four MOFs calculated in the QSDFT mode. | |
C1–C3 light hydrocarbon adsorption
To evaluate the gas separation performance, the CH4, C2H6 and C3H8 adsorption isotherms were measured at 273, 283 and 298 K (Fig. 3a–d and S23–S26†), respectively. All the MOFs presented much higher C2H6 and C3H8 uptake capacity than CH4, indicating the preferential C2H6 and C3H8 adsorption. This can be attributed to the higher polarizability and larger kinetic size of the C2H6 and C3H8 guests, imposing stronger gas-framework interactions in the confined pore-nanospace.41 Notably, the introduction of bulk naphthalene and anthracene in UiO-66 resulted in a reduction in amount of CH4, C2H6 and C3H8 adsorption, stemming from the reduced pore volume. However, the uptake ratios of C2H6/CH4 and C3H8/CH4 for UiO-66, UiO-66-Naph and UiO-66-Anth were different, especially in the low-pressure region (0.05 bar), where the values for C2H6/CH4 and C3H8/CH4 are 12/50 for UiO-66, 16/54 for UiO-66-Naph, and 26/76 for UiO-66-Anth. These results suggest that the aromatic-functionalized MOFs may present better low-concentration C2–C3 light hydrocarbons separation potential from CH4 after enlarging the aromatic rings, which was further confirmed by transient breakthrough experiments (vide infra).
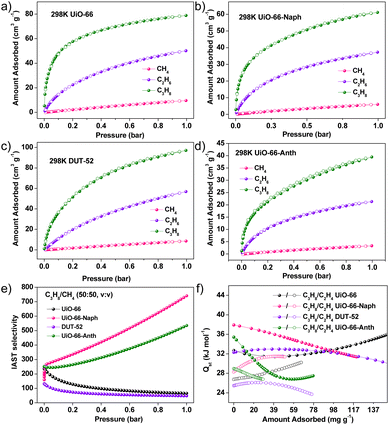 |
| Fig. 3 Gas adsorption and separation performance of UiO-66, UiO-66-Naph, DUT-52 and UiO-66-Anth. (a–d) CH4, C2H6 and C3H8 adsorption isotherms of the MOFs at 298 K. (e) IAST selectivity of the MOFs for C3H8/CH4 (50 : 50, v/v) at 298 K. (f) Qst of C2H6 and C3H8 adsorption of the MOFs as a function of the surface coverage. | |
The ideal adsorption solution theory (IAST) model with dual-site Langmuir–Freundlich fitting was implemented to calculate the C2H6/CH4 and C3H8/CH4 selectivity in different mixing ratios (Fig. 3e, S35–S37 and Table S4†).42 For C2H6/CH4 (10
:
90 or 50
:
50, v/v), the IAST selectivity showed a decreasing trend in the order of UiO-66-Anth ≥ UiO-66-Naph > UiO-66 > DUT-52 at 298 K and 1 bar. In the case of C3H8/CH4 (10
:
90 or 50
:
50, v/v), the IAST selectivity presented a similar descending trend of UiO-66-Naph > UiO-66-Anth > UiO-66 > DUT-52. The above-mentioned results manifest that MOFs with a small pore size, which can be achieved by introducing condensed aromatic rings, are beneficial for improving the C2H6/CH4 and C3H8/CH4 adsorption selectivity, while MOFs with a larger pore size result in higher C2H6/C3H8 uptake. Noteworthily, the C2H6/CH4 and C3H8/CH4 (50
:
50, v/v) selectivity for UiO-66-Naph are higher than that of many reported MOF materials under ambient conditions, demonstrating that the introduction of an aromatic system with an appropriate size can effectively enhance the C2–C3 light hydrocarbon adsorption (Table S3†). For comparison with the many reported strategies applied to optimize the C1–C3 light hydrocarbon separation ability of porous materials (Table S2†), we calculated the increasing ratio of the C3/C1 IAST selectivity (C3/C1, 50
:
50) to evaluate the efficiency of these strategies. It is noteworthy that the aromatic effect is among the most effective strategies reported thus far.
The isosteric heat of adsorption (Qst) for C2H6 and C3H8 was calculated based on the gas adsorption isotherms at 273, 283 and 298 K using the Clausius–Clapeyron equation (Fig. S23–S34†).43 As depicted in Fig. 3f, the Qst values at near zero coverage for C2H6/C3H8 show a clear decreasing trend from UiO-66-Naph (28.3/37.9 kJ mol−1) ≈ UiO-66-Anth (28.9/35.1 kJ mol−1) to UiO-66 (26.8/32.7 kJ mol−1) and DUT-52 (24.6/28.9 kJ mol−1), suggesting decreased gas-framework interactions. For the three MOFs with the same ligand length but different fused aromatic rings, UiO-66 has the lowest Qst value, demonstrating the effectiveness of the aromatic systems, which result in an enhanced confined effect. The comparable C2–C3 hydrocarbon Qst values of UiO-66-Anth and UiO-66-Naph imply that the introduction of an oversized aromatic system does not necessarily lead to stronger gas-framework interactions. Compared with UiO-66/66-Naph/66-Anth, DUT-52 with the largest pore size showed the lowest Qst values, although it also has a larger aromatic system than UiO-66, indicating that a delicate balance between the pore size and aromatic ring size is important for the gas-framework interactions.
Theoretical calculations
Considering that the IAST selectivity calculation based on the pure-component adsorption isotherms may not be able to reflect the practical separation capacity of the C1–C3 gas mixture, we further investigated the gas adsorption mechanism through theoretical calculations using the grand canonical Monte Carlo (GCMC) method.44–47 The structural models of all the MOFs were constructed based on the single-crystal structures of UiO-66 and DUT-52, respectively. It is worth noting that the benzene rings repulse each other in the simulated UiO-66-Anth structure owing to the bulky volume of anthracene, thereby resulting in a very twisted and unreasonable conformation. Thus, it is expected that UiO-66-Anth contains framework defects to some extent to reduce the density of the anthracene rings to stay in a reasonable low-energy conformation, as evidenced by the PSD (2.19 nm), solid 13C NMR, and TGA (stable only up to 300 °C, lower than other Zr-MOFs) analyses (Fig. S9, S13, S14 and S44†). Consequently, the ideal structure model for UiO-66-Anth was not constructed. The density distribution results for CH4/C2H6/C3H8 at 1 bar in the other three Zr-MOFs reveal that all three gases are primarily located in the tetrahedral cage through vdW force interactions (Fig. S46†). It can be seen that DUT-52 has more pore nanospace for gas capture than the other two Zr-MOFs. Furthermore, the gas density in the tetrahedral cage increased gradually together with an increase in the gas molecule size, indicating gradually enhanced gas-framework interactions. The calculated binding energies of CH4, C2H6 and C3H8 for UiO-66-Naph (23.5, 33.2, and 44.6 kJ mol−1) are higher than that of UiO-66 (18.9, 23.5, and 43 kJ mol−1), indicating stronger gas-framework interactions, which can be attributed to the aromatic effect in the present of similar small pores (Table S5†), respectively. However, for DUT-52, its calculated CH4 (16.8 kJ mol−1) and C3H8 (32.8 kJ mol−1) binding energies are lower than that of UiO-66, while the C2H6 binding energy (26.7 kJ mol−1) is slightly higher than that of UiO-66. These results are consistent with the Qst results, implying that large pores have a negative impact on the gas-framework interactions. Concurrently, the binding energies of C2H6 and C3H8 are much higher than that of CH4 for all three MOFs, consistent with the experimental results, further highlighting the potential C2–C3 light hydrocarbon separation performance from CH4. For all three MOFs, CH4, C2H6 and C3H8 are grasped in their tetrahedral cage mainly through C–H⋯π interactions. Notably, the C–H⋯π interacting distances in UiO-66-Naph for all three gases are shorter than that in UiO-66, and consequently stronger gas-framework interactions existed in the former structure (Fig. 4). Specifically, the C–H⋯π distances in UiO-66 follow the order of CH4 (3.84–3.87, average 3.84 Å) > C2H6 (3.54–3.61, average 3.57 Å) > C3H8 (3.11–3.42, average 3.28 Å), that in UiO-66-Naph is CH4 (3.18–3.71, average 3.39 Å) > C2H6 (3.07–3.28, average 3.17 Å) > C3H8 (2.95–3.70, average 3.27 Å), and that in DUT-52 is CH4 (3.82–3.94 Å, average 3.87 Å) > C2H6 (3.09–3.41 Å, average 3.25 Å) > C3H8 (2.95–3.70 Å, average 3.39 Å).48,49 In addition, the unconventional C–H⋯O hydrogen bonds appeared in UiO-66, with the values of 3.45 Å (C2H6) and 3.94 Å (C3H8). The average C–H⋯π distances are consistent with the calculated binding energy results. For example, all the average distances of UiO-66-Naph are shorter than the corresponding distances of UiO-66. The average distances between CH4, C3H8 and DUT-52 are longer than that of UiO-66, while the distance for C2H6 is shorter. Overall, the theoretical calculations demonstrate that properly aligned large aromatic systems with a suitable size in the pore nanospaces not only result in a smaller pore size, but also provide more potential donors for C–H⋯π interactions, thus leading to enhanced C2–C3 light hydrocarbon separation performance from CH4.
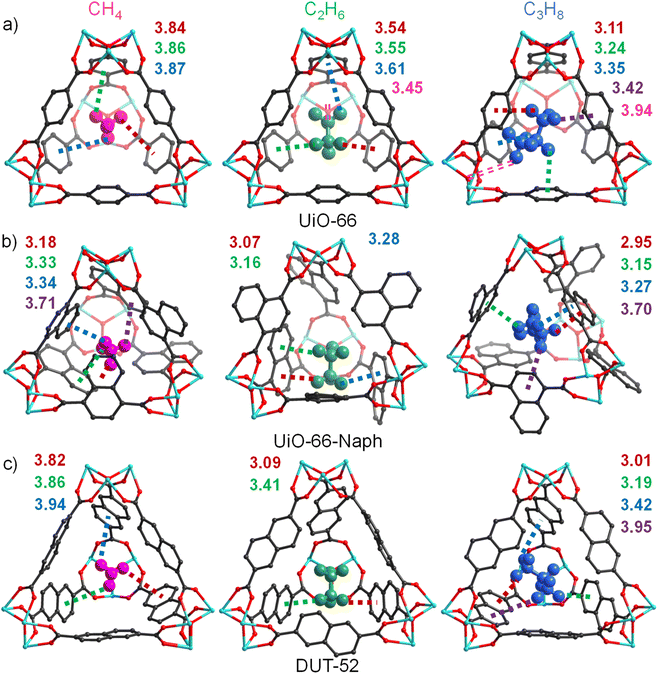 |
| Fig. 4 GCMC-simulated primary absorption sites of CH4/C2H6/C3H8 in (a) UiO-66, (b) UiO-66-Naph and (c) DUT-52. Dotted line, C–H⋯π interaction; double dotted line, C–H⋯O interaction. The hydrogen atoms of the MOFs have been omitted for clarity. | |
Breakthrough experiment
To evaluate the practical separation performance, transient breakthrough experiments were carried out for the four Zr-MOFs under ambient conditions, in which the CH4/C2H6/C3H8 (85
:
10
:
5, v/v/v) mixture flowed over a fixed-bed column at a flow rate of 5 mL min−1 (Fig. 5a–d and Table S4†), respectively. For all four MOFs, high-grade CH4 (UiO-66 > 98.7%, UiO-66-Naph > 99.6%, DUT-52 > 95.6%, UiO-66-Anth > 91.4%) was first eluted, whereas C2H6 and C3H8 were retained in the fixed-bed column for a certain time, and then eluted one after the other. The retention time of C2H6 is similar for UiO-66, UiO-66-Naph and DUT-52, while that for UiO-66-Anth is significantly short. Alternatively, the retention time of C3H8 showed a decreasing trend of UiO-66-Naph > UiO-66 > DUT-52 ≫ UiO-66-Anth. The high-grade CH4 productivity for UiO-66-Naph is 2.25 mmol g−1, which is obviously higher than that for UiO-66 (1.65 mmol g−1), DUT-52 (2.08 mmol g−1) and UiO-66-Anth (0.17 mmol g−1), suggesting the better low-concentration C2–C3 hydrocarbon separation potential of UiO-66-Naph. Surprisingly, the C2H6 (0.18 mmol g−1) and C3H8 (0.77 mmol g−1) capture capacity by UiO-66-Naph is slightly higher than that by UiO-66 (C2H6: 0.17 mmol g−1 and C3H8: 0.74 mmol g−1), and even comparable with that of DUT-52 (C2H6: 0.28 mmol g−1 and C3H8: 0.63 mmol g−1), although the surface area and pore volume of UiO-66-Naph are significantly smaller than that of UiO-66 and DUT-52, which also contradict the uptake capacity measured from the single-gas adsorption isotherms (Fig. 3a–c). This indicates that the aromatic effect plays a significant role in the practical separation of the ternary CH4/C2H6/C3H8 (85
:
10
:
5, v/v/v) gas mixture with a low concentration of C2H6 and C3H8, endowing UiO-66-Naph containing a larger aromatic system with much more efficient utilization of its pore nanospaces to take up low-concentrated C2H6 and C3H8 gases from the gas mixture. In contrast, UiO-66-Anth with the largest aromatic system exhibited the shortest C2/C3 retention time, the lowest CH4 production (0.17 mmol g−1) and the lowest C2/C3 capture amount (0.15/0.29 mmol g−1). The reason for this may be that the too small pore size and low pore volume of UiO-66-Anth limit the gas diffusion in the dynamic separation processes, resulting in low gas capture and quick breakthrough. Moreover, the inevitable formation of framework defects due to the steric repulsion between the bulky anthracene rings is detrimental to the validity of the aromatic effect. Alternatively, DUT-52 with the largest pore size and volume showed the worst C2/C1 and C3/C1 selectivity, adsorption Qst and C3 capture capacity. Although its CH4 productivity was better than UiO-66 and C2 capture capacity better than UiO-66-Naph, the overall C2/C3 retention times were not improved compared with UiO-66-Naph. Therefore, it can be concluded that pore engineering with an appropriate aromatic system is an important factor in the dynamic gas separation process of the ternary C3H8/C2H6/CH4 mixture, depending on the trade-off of the pore size and aromatic ring size. To better assess the C3H8/CH4 separation performance from the ternary CH4/C2H6/C3H8 gas mixture, the separation potential (Δq) was calculated based on the transient breakthrough experiments, in which UiO-66-Naph presented the highest Δq value of 0.75 mmol g−1 compared with UiO-66 (0.71 mmol g−1), DUT-52 (0.61 mmol g−1) and UiO-66-Anth (0.2 mmol g−1), further suggesting the aromatic effect (for the calculation details, see Section S7 in the ESI†). Multiple transient breakthrough experiments on the four MOFs were conducted, which revealed almost the same gas retention time as the first breakthrough curve, suggesting their excellent durability and recyclability (Fig. S38–S41,† respectively).
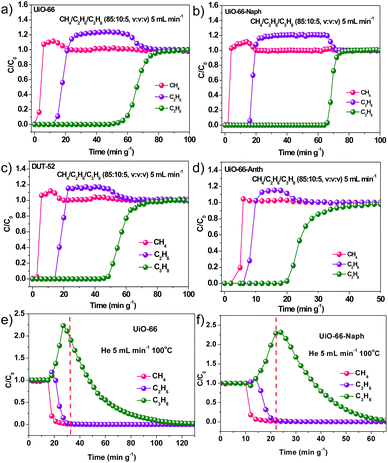 |
| Fig. 5 Transient breakthrough curves (a–d) of CH4/C2H6/C3H8 (85 : 10 : 5, v/v/v) mixture for UiO-66, UiO-66-Naph, DUT-52 and UiO-66-Anth, and desorption curves (e and f) for UiO-66 and UiO-66-Naph, respectively. | |
To evaluate their regeneration potential, an He stream with a rate 5 mL min−1 was flowed over the packing column at 100 °C, while the composition of the eluted gas was monitored by a gas chromatograph. As shown in Fig. 5e, f, S42 and S43,† UiO-66-Naph can be fully activated after 70 min, even shorter than UiO-66 (120 minutes) and comparable with DUT-52, indicating a low regeneration energy requirement, which is beneficial for practical industrial application. This implies that the enhanced aromatic effect in UiO-66-Naph has little influence on the desorption of CH4/C2H6/C3H8 gases, which may be related with the decrease in the Qst values when more C2H6 and C3H8 gas molecules are adsorbed in UiO-66-Naph (Fig. 3f). It should be noted that high-grade C3H8 (UiO-66 > 99.5%, UiO-66-Naph > 99.1%, DUT-52 > 96.2%, UiO-66-Anth > 96.0%) can be afforded after the CH4 and C2H6 off-gases are eluted completely.
3. Conclusion
In summary, we demonstrated that a properly functionalized UiO-66 topological MOF, UiO-66-Naph, featuring optimized pore nanospaces by virtue of the introduction of a naphthalene moiety in the linear carboxylic acid, exhibited efficient low-concentration C2–C3 light hydrocarbon separation from a ternary CH4/C2H6/C3H8 gas mixture. The experimental and simulation results from four isostructural Zr-MOFs with systematic modification of the pore environment by aromatic rings of different sizes revealed that the introduction of a bulky aromatic system with suitable size in the UiO-66 framework not only effectively reduced the pore size, but also provided a more aromatic-rich pore environment, thereby leading to a significantly enhanced separation performance for the low-concentrated C2–C3 light hydrocarbons from CH4. In addition, the excellent physical and chemical stability of UiO-66-Naph guarantee adequate ability for practical separation application. Compared with its UiO-66, DUT-52 and UiO-66-Anth analogues, UiO-66-Naph presented better C2/C1 and C3/C1 selectivity, CH4 productivity, C3H8 capture capacity, and the longest C2/C3 retention time, facilitated by the stronger C–H⋯π interactions and pore confinement. Moreover, its easy regeneration ability comparable with DUT-52 with larger pores and better than UiO-66 suggests a lower regeneration energy requirement, further highlighting that the bulky aromatic-functionalized UiO-66-Naph is a promising adsorbent for the separation of the ternary CH4/C2H6/C3H8 gas mixture. This work can provide guidelines for the design of new porous solid adsorbents in the challenging gas adsorption and separation field.
4. Experimental
General procedure
The standard synthesis procedure was performed by dissolving zirconium tetrachloride and carboxylate ligands in N,N-dimethylformamide (DMF) at room temperature in a 20 mL glass vial, followed by the addition of a modulator.28,29,39 The as-obtained mixture was sealed and placed in a pre-heated oven at 120 °C for a few days. Crystallization was carried out under static conditions. After cooling in air to room temperature, the resulting crystals were filtered and repeatedly washed with DMF.
Synthesis of UiO-66
A mixture of p-phthalic acid (0.083 g, 0.5 mmol) and zirconium tetrachloride (0.12 g, 0.5 mmol) was dissolved in formic acid (3.5 mL) and DMF (16.5 mL). After stirring for 10 min, this solution was transferred to a 40 mL glass vial and kept at 120 °C for 5 days. After cooling to room temperature, the resulting crystals were obtained and washed repeatedly with acetone.
Synthesis of UiO-66-Naph
A mixture of 1,4-naphthalenedicarboxylic acid (H21,4-NDC, 0.11 g, 0.5 mmol) and zirconium tetrachloride (0.12 g, 0.5 mmol) was dissolved in trifluoroacetic acid (1.5 mL) and DMF (18.5 mL). After stirring for 10 min, this solution was transferred to a 40 mL glass vial and kept at 120 °C for 5 days. After cooling to room temperature, the resulting crystals were obtained and washed repeatedly with acetone.
Synthesis of DUT-52
A mixture of 2,6-naphthalenedicarboxylic acid (H22,6-NDC, 0.11 g, 0.5 mmol) and zirconium tetrachloride (0.12 g, 0.5 mmol) was dissolved in concentrated HCl (0.5 mL) and DMF (19.5 mL). After stirring for 10 min, the solution was transferred to a 40 mL glass vial and kept at 120 °C for 5 days. After cooling to room temperature, the resulting crystals were obtained and washed repeatedly with acetone.
Synthesis of UiO-66-Anth
A mixture of 9,10-anthracenedicarboxylic acid (9,10-ADC, 0.15 g, 0.5 mmol) and zirconium tetrachloride (0.12 g, 0.5 mmol) was dissolved in trifluoroacetic acid (1.5 mL) and DMF (18.5 mL). After stirring for 10 min, the solution was transferred to a 40 mL glass vial and kept at 120 °C for 5 days. After cooling to room temperature, the resulting crystals were obtained and washed repeatedly with acetone.
Author contributions
Zhang-Wen Wei and Cheng-Yong Su directed the research projects and supervised the work, as well as contributed to funding acquisition; Xiao-Hong Xiong designed and planned the study; Liang Zhang conducted the experiments and finished formal analysis; the original draw was written by Liang Zhang and Cheng-Xia Chen; Liu-Li Meng and Lu-Zhu Qin revised the manuscript and provided valuable suggestions. All authors discussed the results and contributed to the paper.
Conflicts of interest
There are no conflicts to declare.
Acknowledgements
We acknowledge financial support from the National Natural Science Foundation of China (Grants 21821003, 22001271, 21890380, 22090061, and 22003079), Local Innovative and Research Teams Project of Guangdong Pearl River Talents Program (Grant 2017BT01C161), Natural Science Foundation of Guangdong Province (Grant 2021A1515010298), Guang Dong Basic and Applied Basic Research Foundation (Grant 2020A1515110365), and Fundamental Research Funds for the Central Universities.
Notes and references
- G. George, N. Bhoria, S. AlHallaq, A. Abdala and V. Mittal, Sep. Purif. Technol., 2016, 158, 333–356 CrossRef CAS
.
- T. Tian, Z. Zeng, D. Vulpe, M. E. Casco, G. Divitini, P. A. Midgley, J. Silvestre-Albero, J.-C. Tan, P. Z. Moghadam and D. Fairen-Jimenez, Nat. Mater., 2018, 17, 174–179 CrossRef CAS PubMed
.
- Y. He, W. Zhou, G. Qian and B. Chen, Chem. Soc. Rev., 2014, 43, 5657–5678 RSC
.
- T. A. Makal, J.-R. Li, W. Lu and H.-C. Zhou, Chem. Soc. Rev., 2012, 41, 7761–7779 RSC
.
- Y. He, R. Krishna and B. Chen, Energy Environ. Sci., 2012, 5, 9107–9120 RSC
.
- J. A. Mason, M. Veenstra and J. R. Long, Chem. Sci., 2014, 5, 32–51 RSC
.
- X. Zhang, L. Li, J.-X. Wang, H.-M. Wen, R. Krishna, H. Wu, W. Zhou, Z.-N. Chen, B. Li, G. Qian and B. Chen, J. Am. Chem. Soc., 2020, 142, 633–640 CrossRef CAS PubMed
.
- T. Kundu, M. Wahiduzzaman, B. B. Shah, G. Maurin and D. Zhao, Angew. Chem., Int. Ed., 2019, 58, 8073–8077 CrossRef CAS PubMed
.
- Z. Niu, X. Cui, T. Pham, P. C. Lan, H. Xing, K. A. Forrest, L. Wojtas, B. Space and S. Ma, Angew. Chem., Int. Ed., 2019, 58, 10375 CrossRef CAS
.
- P. Guo, M. Chang, T. Yan, Y. Li and D. Liu, Chin. J. Chem. Eng., 2022, 42, 10–16 CrossRef
.
- K. Jiang, L. Zhang, T. Xia, Y. Yang, B. Li, Y. Cui and G. Qian, Sci. China Mater., 2019, 62, 1315–1322 CrossRef CAS
.
- J. Li, X. Luo, N. Zhao, L. Zhang, Q. Huo and Y. Liu, Inorg. Chem., 2017, 56, 4141–4147 CrossRef CAS PubMed
.
- J. Jia, L. Wang, F. Sun, X. Jing, Z. Bian, L. Gao, R. Krishna and G. Zhu, Chem.–Eur. J., 2014, 20, 9073–9080 CAS
.
- P. Deria, D. A. Gómez-Gualdrón, W. Bury, H. T. Schaef, T. C. Wang, P. K. Thallapally, A. A. Sarjeant, R. Q. Snurr, J. T. Hupp and O. K. Farha, J. Am. Chem. Soc., 2015, 137, 13183–13190 CrossRef CAS PubMed
.
- S. J. Geier, J. A. Mason, E. D. Bloch, W. L. Queen, M. R. Hudson, C. M. Brown and J. R. Long, Chem. Sci., 2013, 4, 2054–2061 RSC
.
- Z. Chen, P. Li, R. Anderson, X. Wang, X. Zhang, L. Robison, L. R. Redfern, S. Moribe, T. Islamoglu, D. A. Gómez-Gualdrón, T. Yildirim, J. F. Stoddart and O. K. Farha, Science, 2020, 368, 297–303 CrossRef CAS PubMed
.
- L. Wang, W. Zhang, J. Ding, L. Gong, R. Krishna, Y. Ran, L. Chen and F. Luo, Nano Res., 2022 DOI:10.1007/s12274-022-4915-0
.
- R.-B. Lin, S. Xiang, W. Zhou and B. Chen, Chem, 2020, 6, 337–363 CAS
.
- W. G. Cui, T. L. Hu and X. H. Bu, Adv. Mater., 2020, 32, e1806445 CrossRef PubMed
.
- J. Pang, F. Jiang, M. Wu, C. Liu, K. Su, W. Lu, D. Yuan and M. Hong, Nat. Commun., 2015, 6, 7575 CrossRef PubMed
.
- R. Augelletti, M. Conti and M. C. Annesini, J. Cleaner Prod., 2017, 140, 1390–1398 CrossRef CAS
.
- M. J. Ahmed and S. K. Theydan, J. Nat. Gas Sci. Eng., 2014, 18, 1–6 CrossRef CAS
.
- G. S. Cheripally, A. Mannava, G. Kumar, R. Gupta, P. Saha, B. Mandal, R. Uppaluri, S. Gumma and A. K. Ghoshal, J. Chem. Eng. Data, 2013, 58, 1606–1612 CrossRef CAS
.
- Y. Zhang, L. Yang, L. Wang, S. Duttwyler and H. Xing, Angew. Chem., Int. Ed., 2019, 58, 8145–8150 CrossRef CAS PubMed
.
- H.-F. Zhang, M. Li, X.-Z. Wang, D. Luo, Y.-F. Zhao, X.-P. Zhou and D. Li, J. Mater. Chem. A, 2018, 6, 4260–4265 RSC
.
- C. X. Chen, Z. W. Wei, J. J. Jiang, S. P. Zheng, H. P. Wang, Q. F. Qiu, C. C. Cao, D. Fenske and C. Y. Su, J. Am. Chem. Soc., 2017, 139, 6034–6037 CrossRef CAS PubMed
.
- Y. Chen, D. Lv, J. Wu, J. Xiao, H. Xi, Q. Xia and Z. Li, Chem. Eng. J., 2017, 308, 1065–1072 CrossRef CAS
.
- S. Xian, J. Peng, Z. Zhang, Q. Xia, H. Wang and Z. Li, Chem. Eng. J., 2015, 270, 385–392 CrossRef CAS
.
- H. F. Drake, Z. Xiao, G. S. Day, S. W. Vali, L. L. Daemen, Y. Cheng, P. Cai, J. E. Kuszynski, H. Lin, H.-C. Zhou and M. R. Ryder, ACS Appl. Mater. Interfaces, 2022, 14, 11192–11199 CrossRef CAS PubMed
.
- C. Jiang, X. Wang, Y. Ouyang, K. Lu, W. Jiang, H. Xu, X. Wei, Z. Wang, F. Dai and D. Sun, Nanoscale Adv., 2022, 4, 2077–2089 RSC
.
- J. Pang, C. T. Lollar, S. Che, J.-S. Qin, J. Li, P. Cai, M. Wu, D. Yuan, M. Hong and H.-C. Zhou, CCS Chem., 2021, 3, 1701–1709 CrossRef CAS
.
- J. H. Cavka, S. Jakobsen, U. Olsbye, N. Guillou, C. Lamberti, S. Bordiga and K. P. Lillerud, J. Am. Chem. Soc., 2008, 130, 13850–13851 CrossRef PubMed
.
- Y. Xiong, T. Yang, S. Chen, C.-H. Zhang, C.-X. Chen, Z.-W. Wei, D. Wang, J.-J. Jiang and C.-Y. Su, Cryst. Growth Des., 2019, 19, 300–304 CrossRef CAS
.
- D. Wang, T. Zhao, Y. Cao, S. Yao, G. Li, Q. Huo and Y. Liu, Chem. Commun., 2014, 50, 8648–8650 RSC
.
- Y. Chen, Z. Qiao, D. Lv, H. Wu, R. Shi, Q. Xia, H. Wang, J. Zhou and Z. Li, Ind. Eng. Chem. Res., 2017, 56, 4488–4495 CrossRef CAS
.
- S. Gao, C. G. Morris, Z. Lu, Y. Yan, H. G. W. Godfrey, C. Murray, C. C. Tang, K. M. Thomas, S. Yang and M. Schröder, Chem. Mater., 2016, 28, 2331–2340 CrossRef CAS
.
- X. Wang, L. Li, Y. Wang, J.-R. Li and J. Li, CrystEngComm, 2017, 19, 1729–1737 RSC
.
- G. Han, K. Wang, Y. Peng, Y. Zhang, H. Huang and C. Zhong, Ind. Eng. Chem. Res., 2017, 56, 14633–14641 CrossRef CAS
.
- V. Bon, I. Senkovska, M. S. Weiss and S. Kaskel, CrystEngComm, 2013, 15, 9572–9577 RSC
.
- P. M. Schoenecker, C. G. Carson, H. Jasuja, C. J. J. Flemming and K. S. Walton, Ind. Eng. Chem. Res., 2012, 51, 6513–6519 CrossRef CAS
.
- J.-R. Li, R. J. Kuppler and H.-C. Zhou, Chem. Soc. Rev., 2009, 38, 1477–1504 RSC
.
- K. S. Walton and D. S. Sholl, AIChE J., 2015, 61, 2757–2762 CrossRef CAS
.
- J. L. C. Rowsell and O. M. Yaghi, J. Am. Chem. Soc., 2006, 128, 1304–1315 CrossRef CAS PubMed
.
-
Accelrys Materials Studio Release Motes, Release 5.5.1, Accelrys Software, Inc., San Diego, 2010 Search PubMed
.
- L. Valenzano, B. Civalleri, S. M. Chavan, S. Bordiga, M. Nilsen, S. Jakobsen, K. Lillerud and C. Lamberti, Chem. Mater., 2011, 23, 1700–1718 CrossRef CAS
.
- L. Gong, Y. Ye, Y. Liu, Y. Li, Z. Bao, S. Xiang, Z. Zhang and B. Chen, ACS Appl. Mater. Interfaces, 2022, 14, 19623–19628 CrossRef CAS PubMed
.
- M. Fischer, F. Hoffmann and M. Fröba, ChemPhysChem, 2010, 11, 2220–2229 CrossRef CAS PubMed
.
- D. Lv, Z. Liu, F. Xu, H. Wu, W. Yuan, J. Yan, H. Xi, X. Chen and Q. Xia, Sep. Purif. Technol., 2021, 266, 118198 CrossRef CAS
.
- Y. Zhang, L. Yang, L. Wang, X. Cui and H. Xing, J. Mater. Chem. A, 2019, 7, 27560–27566 RSC
.
Footnote |
† Electronic supplementary information (ESI) available: Materials and instrumentation, PXRD, TGA, gas adsorption, C3/C1 IAST selectivity comparison, breakthrough experiment details, theoretical calculations. See DOI: https://doi.org/10.1039/d2ta09338c |
|
This journal is © The Royal Society of Chemistry 2023 |
Click here to see how this site uses Cookies. View our privacy policy here.