DOI:
10.1039/D2MH01178F
(Review Article)
Mater. Horiz., 2023,
10, 292-312
Microbial electrosynthesis: carbonaceous electrode materials for CO2 conversion†
Received
23rd September 2022
, Accepted 2nd December 2022
First published on 2nd December 2022
Abstract
Microbial electrosynthesis (MES) is a sustainable approach to address greenhouse gas (GHG) emissions using anthropogenic carbon dioxide (CO2) as a building block to create clean fuels and highly valuable chemicals. The efficiency of MES-based CO2 conversion is closely related to the performance of electrode material and, in particular, the cathode for which carbonaceous materials are frequently used. Compared to expensive metal electrodes, carbonaceous materials are biocompatible with a high specific surface area, wide range of possible morphologies, and excellent chemical stability, and their use can maximize the growth of bacteria and enhance electron transfer rates. Examples include MES cathodes based on carbon nanotubes, graphene, graphene oxide, graphite, graphite felt, graphitic carbon nitride (g-C3N4), activated carbon, carbon felt, carbon dots, carbon fibers, carbon brushes, carbon cloth, reticulated vitreous carbon foam, MXenes, and biochar. Herein, we review the state-of-the-art MES, including thermodynamic and kinetic processes that underpin MES-based CO2 conversion, as well as the impact of reactor type and configuration, selection of biocompatible electrolytes, product selectivity, and the use of novel methods for stimulating biomass accumulation. Specific emphasis is placed on carbonaceous electrode materials, their 3D bioprinting and surface features, and the use of waste-derived carbon or biochar as an outstanding material for further improving the environmental conditions of CO2 conversion using carbon-hungry microbes and as a step toward the circular economy. MES would be an outstanding technique to develop rocket fuels and bioderived products using CO2 in the atmosphere for the Mars mission.
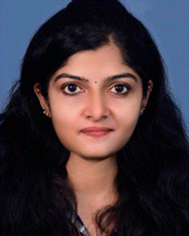
G. S. Lekshmi
| Lekshmi Gopakumari Satheesh Chandran has received a PhD in 2021 from the Centre for Nanoscience and Technology, Anna University, India for research work entitled “Facile Wick and Oil Flame Derived r-GO Decorated with Silver Oxide Nanostructures for Environmental Remediation & Biological Applications”. Currently, she is working as a post-doctoral fellow at the International Centre for Research on Innovative Bio-based Materials (ICRI-BioM), Lodz University of Technology, Poland. She is currently working on the engineering of biofilm-coated electrode materials for CO2 conversion. Her main research interests are graphene-based materials for energy/environment application, 2D materials, electrochemical biosensors, and microbial electrochemical systems. |
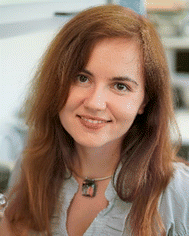
Kateryna Bazaka
| Prof. Kateryna Bazaka is an ARC Future Fellow and ANU Futures Fellow at the Australian National University, Australia, where she leads Aerospace Systems Engineering. Katia's interests broadly include the development of novel nanotechnologies that offer maximum efficiency at a minimal environmental cost to facilitate sustainable societal and economic development. Where this issue is presently addressed by sustainable materials syntheses, designing bio-degradable products, and exploring sustainable energy sources, Katia's goal is to apply green chemistry approaches across the entire life cycle of nanotech products, from design and nanomaterial synthesis to utilization and disposal. Currently, Katia's research focuses on the development of efficient methods that minimize the process steps and dramatically reduce the use of expensive and/or hazardous reagents to be applied to low-cost natural and waste sources to produce sophisticated, high-value nanomaterials with a wide range of applications. Her Google Scholar shows over 200 articles with about 7400 citations, 46 h-index, and 121 i10-index. |
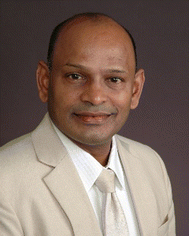
Seeram Ramakrishna
| Professor Seeram Ramakrishna, FREng, Everest Chair, is a world-renowned poly-disciplinary scholar at the National University of Singapore (NUS). He is named among the World's Most Influential Minds and World's Highly Cited Researchers. His Google Scholar shows over 2000 articles with about 150 900 citations, 176 h-index, and 1317 i10-index. He is the Chairman of the Plastics Recycling Center of Excellence at PRAS, Singapore. He is a member of the Extended Producer Responsibility Advisory Committee of the Ministry of Sustainability and the Environment (MSE), Singapore. His book, An Introduction to Circular Economy, received the 2021 Springer Nature: China New Development Award. He is honored with the Excellent Presentation Award by the ICWMT (International Conference on Waste Management and Technology) 2021 initiated by the Basel Convention Regional Centre for Asia and the Pacific and UNEP. He is a member of the ISO Committees on ISO59020/TC323 Circular Economy and WG3 on Circularity. He is a member of UNESCO's Global Independent Expert Group on Universities and the 2030 Agenda. |
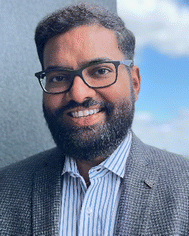
Vignesh Kumaravel
| Dr. Vignesh Kumaravel is the Senior Principal Investigator and Assistant Professor at the International Centre for Research on Innovative Bio-based Materials (ICRI-BioM), Lodz University of Technology, Poland. He is named among the 2022 ranking of the world’s Top 2% of scientists. He is leading the Polymers and Biomaterials (PolyBioMat) group with several post-doctoral researchers, and PhD students. The main research interests of PolyBioMat are: (a) Antimicrobial food packaging polymers; (b) Dental tissue engineering; (c) Antimicrobial orthopedic implants; and (d) Bio-electrochemical conversion of CO2 into value-added products. He has completed several research projects through national/international collaborations. He has published more than 50 research articles with about 3000 citations, 30 h-index, and 37 i10 index. Vignesh is an expert in business relations tailored to commercialize the outcomes of research with various industries. He has developed several novel materials and technologies in collaboration with eminent scientists from academia (Spain, Italy, Malaysia, Republic of Korea, United Kingdom, Qatar, and Ireland) and industry. |
1. Introduction
Climate change is one of the toughest challenges facing humanity today, accelerated significantly by greenhouse gas (GHG) emissions.1 In recent years, sweltering heat waves have broken the highest temperature records all around the world, damaging agricultural lands, cutting out power, sparking wildfires, collapsing airport runways, and severely injuring thousands in Europe alone.2 Heatwaves can influence the density of airborne microbes, as well as change the microbial dispersal in the troposphere, thereby bringing people closer to pathogens, increasing the infection rate of life-threatening diseases, and triggering the emergence of multidrug-resistant microbes.3 The link between human health and climate change, and the need for collaborative actions were highlighted at the 21st United Nations Climate Change Conference in Glasgow (Conference of the Parties (COP26)).4 However, the realization of the ambitious United Nations Sustainable Development Goals to eradicate poverty, provide peace and security, and safeguard the environment by 2030 are greatly challenged by such global issues as GHG emissions, organic waste generation, and the energy crisis that have been exacerbated further by rapid urbanization, industrialization, and population growth.5 Given the significant role that carbon dioxide (CO2) plays in GHG-related climate change, there are strong financial and political incentives to develop state-of-the-art CO2 capture, utilization, and storage (CCUS) techniques.
A growing trend amongst the CCUS techniques is the utilization of CO2 as a building block to create clean fuels and highly valuable chemicals. The techniques that may be employed for CO2 conversion vary widely and include those based on photoelectrochemical,6 photocatalytic,7 electrocatalytic,8 thermocatalytic,9 radiolytic,9 and biochemical10 processes. The key features of important CO2 conversion methods have been compared (Table S1 in the ESI†) emphasizing the differences in reaction pathways, end products, benefits, and drawbacks of each approach. As a method of artificial photosynthesis, photoelectrochemical CO2 conversion generates a variety of products, including formate, formaldehyde, formic acid, methane, methanol, and ethanol. Financial feasibility and product selectivity are the advantages of photoelectrochemical CO2 conversion along with the utilization of the inexhaustible solar energy. The poor light-absorption capability and stability of electrodes are the major bottlenecks in photoelectrochemical CO2 conversion.
Substantial temperatures and pressures are usually required for the chemical conversion of CO2 into value-added chemicals since CO2 is a thermodynamically stable molecule. The performance and scalability of thermocatalysis are powered by heat energy to produce hydrocarbons and olefins. The thermocatalysis of CO2 involves the adsorption of CO2 molecules on catalyst surfaces, followed by the need for crucial activation energy (Ea) to bend the chemical bonds between C and O. The poor performance of catalysts and the challenges in thermal energy management are the main barriers of this technology.
The primary difficulties with photocatalytic conversion are low product selectivity, poor stability, and low energy efficiency. The main obstacles with electrocatalytic conversion are the demand for high overpotentials, more intricate reaction pathways, and a liquid electrolyte with a low solubility of CO2 that lowers the current density. In contrast to these methods, microbial electrosynthesis (MES) is one of the most affordable technologies that can convert renewable electricity and anthropogenic CO2 to high-value-added products at room temperature and pressure.11 In MES, electroactive bacteria (EAB) are used as a biocatalyst on appropriate electrode materials to recycle the anthropogenic CO2.12
The choice of the electrode material plays a significant role in defining the efficiency and product selectivity of the CO2 conversion process. Carbonaceous materials are commonly preferred as electrodes in MES-based CO2 conversion because of their chemical stability, electrochemically active surface area, biocompatibility, and electrical conductivity.13 The absorption and adsorption capabilities are among the most significant features of carbonaceous electrodes. The simplest, cheapest, and least denaturing way to immobilize bacteria is physical adsorption, which is accomplished through physical forces such as van der Waals, ionic, and π–π interactions. The process typically involves the bacteria being simply deposited onto carbonaceous electrode materials.
Carbonaceous materials have also been combined with other materials to increase the specific area of the electrode and hence increase the current density.
Carbonaceous materials have a significantly greater level of chemical stability because of the dispersion of their carbon atoms, which are extremely stable. These characteristics include high specific surface area and porous morphology, both increasing the number of points available for microorganism attachment.14 Another significant advantage of using carbonaceous materials is their process flexibility. It enables the engineering of biocompatible electrodes with complex architectures, high surface area, and stability to maximize the desired level of growth of bacteria and enhance electron transfer rates. This is because the efficiency of product formation is highly correlated with the microbe-electrode interface and extracellular electron transfer. Hence, surface-decorated carbonaceous electrodes have been demonstrated to foster these interactions and promote electron transfer mechanisms.15
Most of the research in MES has taken place over the last ten years, and numerous biocathodes have been developed for CO2 conversion. The historical milestones in MES-based CO2 conversion using the carbonaceous electrode materials over this period are schematically shown in Fig. 1.16–34
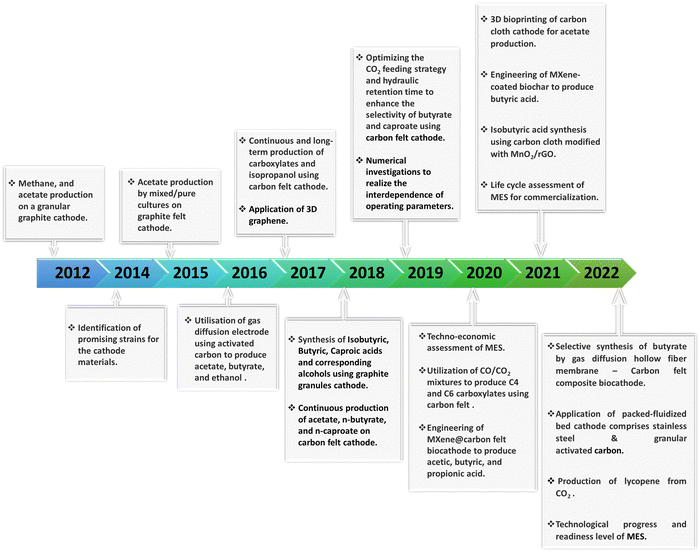 |
| Fig. 1 Schematic of the historical milestones in MES-based CO2 conversion using carbonaceous electrodes during the last 10 years. | |
Although there is an evident lack of a techno-economically driven roadmap for validating and demonstrating the technology on a large scale, MES has received great attention in laboratory-scale research.26 Recently, much research has focused on engineering cost-effective electrode materials and the biotechnological significance of microbial CO2 fixation, along with the conversion of precursors to commercial chemicals. Nevertheless, understanding the interdisciplinary features of the MES system remains in its early stages. The significance of CO2 electro-biorefinery and the commercialization challenges of MES technology have been brought to light in a recent study.35 The work focused on examining the essential features of MES such as microbial CO2 fixation, electron transport mechanism, and the key findings. In a similar study, the fabrication strategies and characterization of graphene biocathodes for MES have been explored.14 However, there are no comprehensive investigations on the significance of various carbonaceous materials as biocathodes for MES-enabled conversion of CO2.
Herein, we present a critical review of the most promising state-of-the-art carbonaceous biocathodes, including the techniques used for their fabrication and assembly (e.g., 3D bioprinting), their surface features, efficiency, and product selectivity. We also briefly discuss the important role played by biocompatible electrolytes, and the choice of reactor configurations, as well as provide a brief outline of the thermodynamics and kinetics of the system. The challenges, prospects, and industrial development of MES-driven CO2 conversion are outlined, making a note of emerging strategies such as cold plasma. Techniques based on cold plasma may be used to help control or enhance microbial performance and biomass accumulation, which may have not been touched upon in this context in the previously published reviews.
2. MES reactor designs
MES is a cutting-edge hybrid technology to produce sustainable and renewable energy from an inexpensive microbial medium. Various reactor configurations (H-type-Fig. 2(a), single chamber-Fig. 2(b), dual chamber-Fig. 2(c), and stirred tank-Fig. 2(d)) for MES-based CO2 conversion, and the schematic of electron transfer mechanism (Fig. 2(e)) are shown in Fig. 2. Single- and dual-chamber reactors are commonly used in MES. An effective bioreactor design should consider increased productivity and the validation of desired parameters to deliver consistency and higher quality products while remaining cost-effective. A bioreactor design and its method of operation are determined by the type of microorganisms, ideal conditions needed for the formation of the intended product, value of the products, and the production volume.
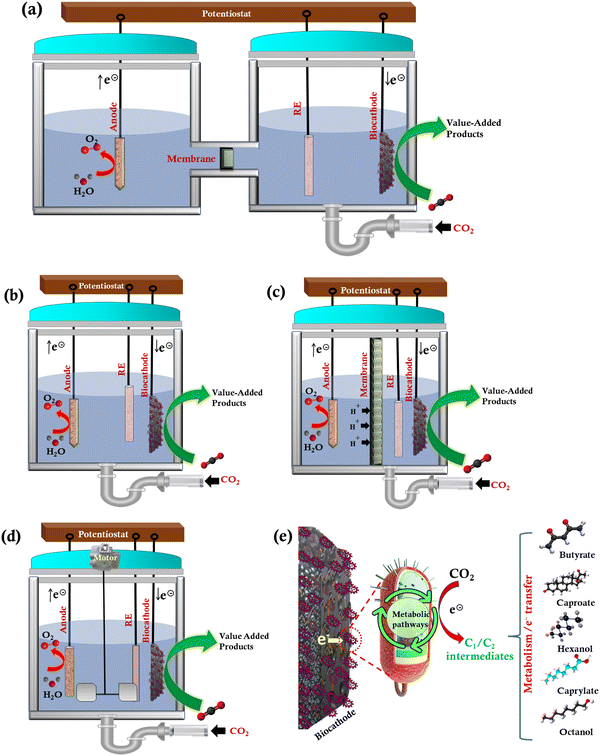 |
| Fig. 2 Reactor configurations for MES-based CO2 conversion: (a) H-type, (b) single chamber, (c) dual chamber, (d) continuous stirred tank, and (e) schematic of electron transfer mechanism. | |
In a single-chamber reactor, the anode and cathode are either placed in separate chambers or in a single chamber that shares the electrolyte (Fig. 2(b)). In the case of a dual chamber reactor, protons are typically conducted from the anode to the cathode using a cation exchange membrane (CEM, Fig. 2(c)). Protons and electrons are produced at the anode and transferred to the cathode by an externally applied electrical potential. Membranes enable the movement of ions from one chamber to the other to complete the electrical circuit. The potentially reactive components of the medium cannot, however, diffuse between the two chambers. The membrane, however, lowers the electrical current and performance in a two-chamber system because it can increase the reactor's electrical resistance. But a single chamber system does not have to deal with the membrane's additional resistance. It is frequently employed to generate CH4, but the anodic reaction does not involve water electrolysis, which would have produced oxygen, but rather the mixed bacterial cultures digest organic matter. H-Type MES reactors have typically been employed for lab-scale studies because of their flexibility36 (Fig. 2(a)).
There are different modes of operation in the MES reactors such as batch, continuous, semi-continuous, and fed-batch based on the microbial culture and medium feeding strategy. Microorganisms are introduced into the sterile culture medium in the batch mode after it has been sterilized. Cells, substrates, nutrients (salts and vitamins), and product concentrations change throughout this reaction period. The product is harvested once the fermentation has run for a certain period. The use of acetogenic bacteria in the storage of H2 and the capture of CO2 to produce formic acid were studied.37 The demonstration of the MES in the batch-mode stirred-tank bioreactors (Fig. 2(d)) showed that scaling up the whole existing system is technically feasible. The process has a CO2 conversion efficiency of 100%, and it produced formic acid at a specific rate of 48.3 mmol g−1 h−1.37 Batch bioreactors are simple to use and consist of a single tank that can perform a series of processes. The stirred tank reactor, which mixes the reactants in the tank, has an integrated heating and cooling system (Fig. 2(d)). Because of the relatively short growth period and lesser capital investment compared to continuous mode for the same bioreactor volume, batch reactors have advantages such as more flexibility with different production systems and reduced risk of contamination or cell mutation.
Using a tubular-type reactor, the associated scaling-up issue, such as maximizing the electrode and membrane surface area concerning the reactor volume, may be easily handled.38 The inner tube, which sits inside the larger outer tube, has radial holes on one side. A plastic inner rod is repeatedly revolved around an anode electrode assembly that is located inside the inner tube. The outer surface of the inner tube is surrounded by a concealer to cover up the perforations, creating a barrier between the internal volumes of the two tubes. There has been a lot of interest in using electroactive microorganisms in MES to produce valuable compounds from CO2 and electricity as a sustainable way to convert extra energy from renewable energy sources into reliable commodities.
To advance the current densities and efficiencies, the field must discuss the interaction between microbial catalysts and the cathode, which is the core element of MES. It is critical to consider how the electrode and biocatalyst designs can be altered to overcome cellular and geometric electron transfer rate constraints. A two-chamber reactor configuration could avoid contact between sensitive microorganisms at the cathode and oxygen produced at the anode. This kind of reactor is beneficial for separating the product stream from the reaction and requires less culture medium because the anode chamber can be loaded with water or buffer solutions. Consequently, the product purity can be enhanced. As the membrane allows the products produced cathodically to pass through, the product yield will be reduced.
H-Cells have proven to be excellent platforms for preliminary research or comparing the performance of different MES components, such as membrane or cathode materials (Fig. 2(a)). Because the continuous mode promotes cell growth, this may be one of the reasons by which dense biofilms have been obtained primarily with this type of reactor. Planktonic populations are easily wiped out of the reactor during the continuous mode because it is related to selection pressure. Nevertheless, the operational mode is just one of the several elements influencing biofilm formation. Recently, solid-state electrolytes have been engineered to enhance the product selectivity and purity for the electrochemical conversion of CO2 in a four-chamber reactor with a bipolar membrane.39 In another recent study, a three-compartment reactor (anode compartment, centre flow compartment, and cathode compartment) was designed for the electrochemical conversion of CO2.40 An alkaline stable anion and cation exchange membranes were employed. A cation exchange media was used in the central compartment to provide the required solution conductivity for the produced formic acid in the cathode compartment. Similar kinds of reactor configurations and electrolytes should be developed in MES to enhance the product yield, selectivity, and purity.
Different reactors have distinct benefits and drawbacks. Continuous stirred tank reactors are found to be adaptable for a variety of bioprocesses. The energy requirements of this type of reactor may increase as they scale up.38 High biomass concentration is present in biofilm-forming reactors. This type of a reactor has more compact reactor volumes and low energy needs; however, it has mass transfer limitations as the biomass concentrations rise.41 The rate-limiting step in rotating packed bed biofilm reactors is diffusion across the gas-liquid interface, which requires careful management to maintain adequate rotation despite the effective mass transfer from the bulk gas to the electrode surface.42 Monolithic Biofilm Reactor includes electrode materials with large pore size, large specific surface area, and significant mechanical strength to avoid biomass washout at higher dilution rates. The drawbacks include reliance of channel shape and a low gas flow rate.43 Since mechanical mixing and operation in various modes are not necessary, bubble column reactors have low maintenance and operating expenses. Its drawbacks include the need to optimize bubble size for successful mass transfer.44
Biofilm-coated anodes have been commonly utilized in microbial fuel cells (MFC) to convert chemical energy into electricity.45 Recently, a microbial carbon capture (MCC) technology has been established through the utilization of biofilm-coated anode and cathode.45 MCC is an advanced technology that integrates the microbial fuel cell (utilizes biofilm coated anode) and MES (biofilm coated cathode). In MCC, the CO2 derived from the wastewater treatment in the anodic chamber could be transferred into the cathodic chamber using an external pipe, and then the produced CO2 could be converted into high-value-added products using biofilm-coated cathodes.
3. Significance and fabrication of carbonaceous biocathodes
Biofilm formation on the electrode is crucial to ensure high levels of electron transfer during the bioconversion process since electrochemically active microorganisms are the essential factors affecting the MES performance.46 The electron transfer mechanism serves as the prime factor in CO2 conversion, and catalytic sites must easily bind to the CO2 molecules.47 Extracellular electron transfer (EET) is a critical step in MES, and several investigations have been conducted to uncover the molecular pathways involved in EET. The actual mechanism, on the other hand, remains a mystery. The choice and development of kinetically efficient electroactive bacteria is frequently complicated. The total advancement of the anode or cathode biofilms can take extended periods due to their slow growth kinetics, which increases the overall reactor initiation time.48 Using carbonaceous materials with a large surface area and porosity that promote microbial attachment and biofilm formation can therefore improve the efficiency of MES for CO2 conversion.
Electrode material synthesis and assembly
There are several established and emerging processes that could be used to engineer carbonaceous electrodes with the desired physicochemical properties (Fig. 3). Of particular significance is the engineering of the electrode surface since the properties of the interface define both cell-surface interactions and the efficiency of the electron transfer.49 For example, versatile biocathodes can be fabricated using a convenient process of spray coating conductive inks containing carbon nanotubes (CNTs), where the use of surfactants ensures uniform nanoparticle dispersion in the ink.50 In spray coating, the biocatalyst can be directly sprayed on the pre-heated electrodes with an airbrush at high pressure to produce a uniform porous biofilm. Dip-coating is another cost-effective method for depositing materials onto virtually any electrode surface. The advantages of dip coating include low cost and simple adjustment of layer thickness. According to recent findings, novel MXene-coated electrodes were produced through dip-coating to improve the electrical conductivity, charge transfer efficiency, and selective microbial enrichment characteristics, which led to an increase in the output of cathodic current.30 The extent of accumulation of microorganisms (Macellibacteroides, Peptostreptococcaceae, Firmicutes, Bacteroidetes (Bacteroidia), and Firmicutes (Clostridia)) in the biofilm on the MXene-coated electrodes was related to both the level of volatile fatty acids (VFAs) production and current density.
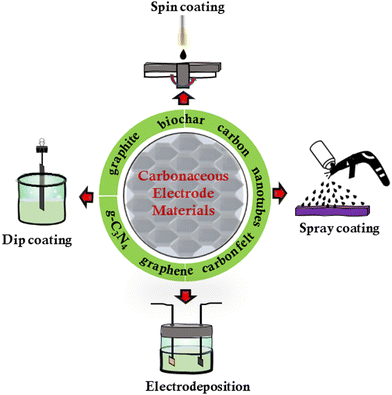 |
| Fig. 3 Schematic of the fabrication of carbonaceous electrode materials. | |
3D Bioprinting
Three-dimensional (3D) printing is a family of additive manufacturing techniques that have received great attention in recent years.51 A layer-by-layer deposition procedure guided by computer-aided design (CAD) software enables the design of intricate 3D structures with efficiency and speed. It is a versatile technique to engineer materials with a myriad of unique chemical, mechanical, and electrical properties.52 The engineering of electrodes for assorted electrochemistry applications is becoming increasingly dependent on 3D printing technologies. Nevertheless, the direct metal laser melting method in 3D printing is very expensive.53
Macroporous 3D electrodes have been identified to increase the surface area for the growth of biofilms and enhance the efficiency of MES. However, the challenge is to utilize a conventional technique to precisely engineer electrodes with geometrical complexity. 3D bioprinting has emerged as a promising method for directly depositing microorganisms on the carbon electrode surface.54,55 The schematic on the designing of carbonaceous biocathode using 3D bioprinting is shown in Fig. 4. The catalytically active live cells could be printed into various self-supporting 3D geometries using the bio-ink. 3D bioprinted material has an enormous scale (up to 225 cm2), varied cell densities, fine filament thicknesses (down to 100 m), and great catalytic efficiency. Printed cells proliferated locally to disperse themselves and were biologically active for up to 4 months. This technique can be used to tailor the cell density and intercellular spacing to the single-cell level employing a supplementary shear-thinning filler made of nitrocellulose.
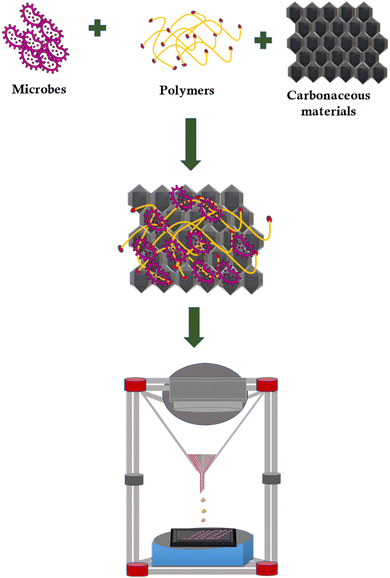 |
| Fig. 4 Schematic for the design of carbonaceous biocathode using 3D bioprinting. | |
According to a most recent study, Shewanella oneidensis MR-1 was employed as a living ink to build an anode using 3D bioprinting technology.48S. oneidensis cells successfully survived the bioprinting process and generated a stable current for nearly 93 h. To create a living material system containing catalytically active microorganisms for 3D bioprinting, scientists have also reported the application of freeze-dried live Saccharomyces cerevisiae yeast cells.56 3D bioprinted materials displayed superior performance with long-term viability. The engineered porous structures could enhance the mass transfer rate and produce a greater quantity of high-value-added products. Specifically, 3D bioprinted electrodes might feasibly obtain high biofilm density because of the substantial adhesion of microorganisms during the process. Thus, 3D bioprinting provides a possible solution before the controlled production of custom-developed materials, including that with channels at the micro and macroscales, with the added benefit of rapid prototyping, testing, and optimization.
Morphology and topology
Electrode materials can be broadly classified as composites containing either single or multi-dimensional nanoparticles. Generally, composites with one-dimensional (1D) particles have constituent elements that exhibit the morphology of nanotubes, nanowires, nanofibers, and nanorods. Owing to their distinctive tube-like nanostructure, selectively chosen crystal aspects, and oriented electron and mass transfer, 1D materials have received a great deal of attention.57 Major intervening products in CO2 conversion can be formed and adsorbed on the active sites owing to the high specific surface area of 1D materials. These materials can also easily combine with other catalysts to create high-yield composite electrode materials for CO2 conversion. For instance, CNTs were easily combined with bacterial catalysts (S. oneidensis MR-1) and improved the charge transport while increasing the number of bacterial cells available on the electrode surface.58
Two-dimensional (2D) electrocatalysts are atomically thin and appear to be attractive materials for CO2 conversion. 2D materials could indeed reveal their catalytic active sites more uniformly because of their planar structure. They also have a great density of surface atoms and edge defects, which can lead to exceptional efficiency in CO2 conversion.59 In addition, the high surface area and small diffusion route for charge transfer can facilitate CO2 electroreduction. Researchers commenced a thorough assessment of microbial growth factors linked to various feedstock and assimilation processes, including acetogens employing H2/CO2 on a 2D electrode.60 According to the findings, formate and methanol appear to be the most effective electron mediators for feedstock bioconversion under anaerobic and aerobic conditions. It was also demonstrated that direct microbial attachment to the cathode was severely hindered since there is a fundamental difference in the kinetics of electrochemical and biological processes.60
Without any surface modifications, porous 3D materials with z large specific surface area can improve microbial-electrode interaction and substrate mobility within the electrode. In addition, 3D electrodes are adaptable for additional spatial customization, and their active sites provide superior contacts for electron transfer via direct or mediated mechanisms.61 For instance, the porous 3D carbon felt (CF) layer can offer a greater surface area that probably supported gas bubble mass movement through the production and attachment of microns to nanosized bubbles, which eventually reduced the gas-diffusion limits, and enhanced electrochemical performance and biofilm growth.
4. Advances of carbonaceous biocathodes for CO2 conversion
Carbonaceous electrodes could naturally offer substantially improved specific surface area and adsorption properties for immobilizing biofilms compared to metal electrodes. Furthermore, they present a wide range of topological features and possibilities for surface chemistry functionalization of suitable materials. Carbonaceous electrodes can also provide a steady immobilization matrix for biofilms, effectively transfer electrons from their active centers, and are economically advantageous. Since the overall MES performance mainly relies on the interactions between biofilms and the electrode surface, including microbial adhesion, electron transport, and electrode resistance, carbonaceous materials can act as promising electrodes. The key findings and the significance of state-of-the-art carbonaceous electrodes for CO2 conversion via MES have been highlighted in this section.
Carbon nanotubes (CNTs)
Due to their unique size, electronic conductivity, mechanical, and chemical characteristics, CNTs are recognized as ground-breaking materials in electronics.62 CNTs are a potential material in MES because they are on the same length scale as enzymes, making adsorption and crosslinking more easily achieved. Various investigations have been performed on the immobilization of enzymes on CNTs to produce a hybrid nanobiocatalytic platform with high stability and reusability.63,64 The enzymes can be modified employing different surface functionalization procedures on the CNTs to achieve the desired properties. In a recent study, Ni-modified CNTs cathodes were designed for the direct delivery of CO2 to the electroactive bacteria (in this instance, cells of Sporomusa ovata)and they showed a 76.3% lower electron transfer resistance.65 For controllable electrochemical syngas production, a highly permeable silver gas diffusion electrode on a CNT-supported hydrophobic membrane was developed.66 The performance of this membrane was tested with various chamber thicknesses, microbial electrolyte compositions, and the direct gas delivery mode. In contrast to the conventional flow-by mode, CO2 was purged into the electrolyte by directly flowing it through the gas diffusion electrode, where it was electrochemically converted to syngas, and larger tunable CO/H2 ratios with higher CO faradaic efficiencies (>90%) were recorded. Since the target syngas oxidizing bacteria such as Clostridium strains are anaerobic, the cathode chamber was monitored under anaerobic conditions with continuous CO2 purging.
Graphene
Graphene can promote bacterial proliferation, cell adhesion, as well as cellular metabolism. It possesses great electrical conductivity, high carrier mobility, exceptional intrinsic mechanical strength, chemical stability, and a large specific surface area. Graphene has been intensively investigated in MES throughout the previous decade. According to recent findings, the formation of an effective electroactive biofilm on graphene electrodes with microorganisms such as Burkholderiales sp., Sulfurospirillum sp., Acetoanaerobium sp., and Lysinibacillus sp. can greatly improve the performance of MES.67 By encouraging the expression of signaling molecules in microorganisms, graphene can both speed up cell growth and function as a mediator to increase the rate of the electron transfer process.68 The effect of pyrochar (a persistent, refractory organic carbon molecule generated when the biomass is burned at 300–700 °C in the presence of low oxygen) and graphene on the bio-methanation process with hydrogen (H2) supplies were compared.69 After repeated intermittent supply, the incorporation of graphene could increase theoretical methane (CH4) production by 78%; this improvement was ascribed to the high electrical conductivity and substantial specific surface area of graphene. According to microbial analysis, the OTUs (Operational Taxonomic Unit) associated with the order SHA-98 of bacteria and the genus Methanothermobacter of archaea may lead to the establishment of a new syntrophic relationship that increases the robustness of the biomethanation process.
Graphene oxide (GO)
A conducting scaffold of rGO/microorganisms can be formed through microbial reduction, which can enable electron transport from the cathode to microbial cells.70 This technique is expected to significantly intensify the attachment of planktonic microbial cells to electrodes as compared to natural biofilm formation. The aggregates with mixed microbial communities are expected to expedite the syntrophic relationship between methanogens and exoelectrogens. By electropolymerizing conductive poly(3,4-ethylenedioxythiophene) on carbon cloth modified with GO film, researchers claimed that they were able to create a new cathode.71 Using an actinobacteria (members of Coriobacteriia), CO2 reduction to CH4 was examined on the GO/poly(3,4-ethylenedioxythiophene) electrode. The CH4 production rate of 315.3 ± 13.2 mmol m−2 day−1 was achieved with a faradaic efficiency greater than 92% at −0.9 V. In another study, it was observed that GO-coated biocompatible Cu electrodes promoted the development of a thick, electroactive biofilm, whereas the untreated copper electrodes were merely covered with dispersed and broken cells.72 These findings demonstrate that GO could improve the MES efficiency for CO2 conversion while also acting as an exceptional conductor with electrochemical properties.
Reduced graphene oxide (rGO)
Compared to other carbonaceous materials, rGO has frequently been investigated to improve the MES performance, especially in producing VFAs. With phyla Proteobacteria (Betaproteobacteria), Bacteroidetes, and Firmicutes as the predominant microbial communities on the cathode, copper ferrite/r-GO nanocomposites were recently used to produce VFAs in a single chamber MES.73 The yield of VFAs was superior owing to the better conductivity and increased surface area of rGO. In a similar study, VFAs production of ∼50.07 g m−2 day−1 was attained using the MnO2/rGO nanohybrid, which was ∼2.16 folds higher as compared to that produced using the bare rGO cathode (23.09 g m−2 day−1) in a single chamber MES.29 Due to the high reduction current provided by rGO in MnO2 and the low charge transfer resistance, the rapid electron transfer from microbes to VFAs was made possible. Microbial communities were easily grown on the MnO2/rGO electrodes owing to the large surface area and biocompatibility of the latter. The MnO2/rGO/carbon cloth cathode delivered a current of 7.8 mA with a Coulombic efficiency of 66.4% for VFAs production. Due to the high biomass inclusion and improved EET, this biofilm enabled highly efficient bidirectional electron transfers among both bacteria and electrodes. In comparison to naturally sourced biofilms, this 3D electroactive biofilm produced a 25-fold increase in the outward current and a 74-fold rise in the inward current.
Graphite
A viable and affordable approach for enhancing cathode performance of MES is the use of a graphite biocathode. By placing a porous conductive material, such as graphite granules, in contact with a current collector in the cathode chamber of MES, the surface area for biofilm adherence can be effectively increased. In a recent study, a 3D graphite cathode treated with nitric acid was employed for the reduction of CO2 into acetate.74 The experiments were carried out using the Clostridium strains. Copper (Cu)-modified graphite blocks electrode demonstrated CH4 production at a rate of 201 nmol cm−3 day−1 and the greatest current density of 0.6 A m−2, and its performance remained constant over time.75 Another study showed that electricigens bacteria such as B. subtilis can be cultivated on graphite electrodes and generate significant current densities at strong oxidizing potentials through outer membrane cytochromes.76 During the initial stages of biofilm formation and expansion, the terminal group chemistry on graphite electrodes affects the constitution and comparative density of the microbial population.77 When introducing acetate as an electron donor during the first stage of biofilm growth, the graphite electrodes functionalized with –NH2, –COOH, and –OH groups showed a greater current density compared to unmodified graphite electrodes under the same operating conditions. This is attributed to the surface charge on the electrodes affecting their ability to support cell development and produce current. Thus, the capability of the electrode surface to interact electrostatically with the bacteria promotes the occurrence of preferential electroactive bacterium attachment during the early stages of colonization. Consequently, the positively charged bacteria can easily adhere to the graphite cathode surface and can easily form biofilms.78
Graphite felt (GF)
Porous GF has a surface area of ∼0.995 m2 g−1, which improves electrochemical efficiency and biofilm growth in the MES system.79 The bacteria can be fed directly with electrons using GF as the substrate. Even though GF has a neutral surface charge, increasing surface hydrophilicity and the addition of functional groups to GF can improve biofilm adherence and proliferation on the electrode surface. The functional groups on the GF surface are intended to encourage direct electron transfer (DET). Studies revealed that GF can be used to change the cathode surface properties and contribute to MES becoming an increasingly feasible, adaptable, and cost-effective strategy for CO2 conversion and renewable energy recovery.80 The idea of converting CO2 to a C6 medium-chain carboxylate and combining it with acetate and n-butyrate using fibrous GF electrodes has recently been realized.78 It was accomplished by an improved forced-flow-through flat-plate reactor that was run with continuous nutrient feeding, allowing the pores of a fibrous GF electrode to be fully colonized by a thick biofilm, then accomplishing the objective of bacterial growth across the entire electrode volume.
Graphitic carbon nitride (g-C3N4)
g-C3N4 is a classic polymer semiconductor that is broadly used in photocatalysis because of advantages such as superior chemical stability, relative affordability, and sustainable visible light response.79 Recently, Serratia marcescens Q1 was used as the biocatalyst to build photo-assisted biocathodes such as WO3/MoO3/g-C3N4 and Ag3PO4/g-C3N4 with Z-scheme heterojunctions.81,82 The light-harvesting capability and acetate production rates were enhanced by WO3/MoO3/g-C3N4. The photocorrosion of Ag3PO4 was prevented well by g-C3N4, enabling effective acetate synthesis from HCO3− in a photo-assisted MES. CuO/g-C3N4-modified CF was utilized as a photocathode for the MES chemical production from CO2.83 MES performance was greatly improved by the CuO/g-C3N4 heterojunction. The electron transfer rate was increased, and the cathodic charge transfer resistance was decreased by photogenerated electrons from CuO/g-C3N4. Instead of indirect electron transfer (IET) via H2, CuO/g-C3N4 primarily increased the electron availability of electroautotrophic bacteria. A recent study reveals the first evidence of effective formate production from CO2 in a photo-assisted MES system using a g-C3N4-NiCoWO4 photoanode and a g-C3N4-activated carbon (AC) fiber biocathode with Escherichia coli. The Z-scheme heterojunction created by the NiCoWO4-g-C3N4/AC fiber enhanced the electron-hole separation process and ∼12.8 mM day −1 of formate was generated with a conversion efficiency of 1.48%.84
Activated carbon (AC)
AC is one of the most frequently used carbonaceous electrode materials in MES. When applied, AC can take the form of granules, cloths, and other fibrous materials. In addition to promoting electroautotrophic microbe adhesion on the cathode and the growth of planktonic cells in the solution, AC offers a significant surface area for microbial colonization.85 A fluidized 3D electrode with granular AC particles has recently been developed using planktonic cells. In comparison to the control without granular AC, the volumetric acetate production rate was increased by 2.8 times with granular AC, and 3.92 g L−1 acetate production was attained within 24 days. A new double-chamber MES integrated system with a slurry electrode and mixed culture was recently designed, and the impact of powdered AC concentration on chemical production was also assessed.86 Compared to the control group without AC (4.8 g L−1), the integrated system modified with 5 g L−1 AC produced approximately 13.4 g L−1 acetate. Nevertheless, the yield of acetate was reduced when the AC concentration was raised to 10 and 20 g L−1. This was ascribed to the inhibition of microbial growth on the electrode at higher concentrations of AC.
Carbon felt (CF)
The distinctive features of 3D CF can provide sufficient contact area and volume for microbial colonization, transportation of microbes, and increased electroactivity.87 The conversion of CO2 to n-butyrate (nC4) and n-caproate (nC6) using CF biocathode and a densely packed mixed bacterial species biocatalyst (i.e., cable bacteria) was recently reported.23 Maximum production rates such as 3.2 ± 0.1 g L−1 day−1 for nC4 and 0.95 ± 0.05 g L−1 day−1 for nC6 were obtained. This study also presented a forced flow-through mode of operation to build a substantial biofilm on the CF cathode that was poised at about 0.85 V vs. SHE. Even though it also generated other chemicals, the system could produce acetate at a rate of up to 9.85 ± 0.65 g L−1 day−1 with a 46.2% electron-to-acetate recovery. The biofilm was formed across the whole thickness of the CF and their interspace. Microorganisms attached to the CF are were to absorb electrons from the cathode surface directly or indirectly to reduce CO2.23 By controlling the composition of the cathodic microbial community, the modified CF electrode could increase the biocatalytic activity.88 A modified CF improved the biocatalytic activity by changing the microbial population composition by increasing acetogens such as Acetobacterium. A high rate of acetate production was ascribed to the improved biocompatibility for biofilm growth on the cathode surface and internal fibers.
Carbon dots (CDs)
Due to their distinctive characteristics, CDs have received a lot of attention, and this has led to the development of promising nanobiointerfaces in conjugation with biomolecules such as enzymes, proteins, antibodies, and genetic materials. In a three-electrode MES system, the CDs fed S. oneidensis MR-1 significantly increased bioelectricity production.89 The zeta potential of the CDs was observed to be substantially connected with the effectiveness of bioelectricity generation. Recently, an inorganic–biologic hybrid was investigated to improve energy conversion in MES by merging a typical electroactive bacterium, Geobacter sulfurreducens, and a highly conductive N-doped Fe3O4 with a CD shell.90 This cathode demonstrated more efficacy, and the superior functionality was accompanied by increased bioactivity, cellular adherence, and moderate biofilm resistance.90 CDs promote the improved metabolism of S. oneidensis MR-1 due to the increase in intracellular electron generation, ATP level, active extracellular secretions, transmembrane, and EET.89
Carbon fibers
Due to their unique properties, including their fibrous structure, high porosity, quick adsorption kinetics, and porous storage capacity, carbon fibers are comparatively innovative but by far one of the most significant electrode materials. The inclusion of carbon fiber in the cathode can enhance the bacterial activity toward the oxygen reduction reaction and increase the specific surface area of the electrode material, maintaining the good performance of the biocathodes. In a recent investigation, a hybrid carbon fiber cathode and a two-chamber MES cell were designed, and the effectiveness of the electroactive biofilm and the EET mechanisms were systematically investigated. The activity and charge transfer resistance of the electroactive biofilms that were formed on the surfaces of the biocathode were excellent. At a cathodic potential of 1.0 V vs. Ag/AgCl and Coulombic efficiency of 75.8 ± 9.9%, CH4 was produced at a rate of 298.0 46.7 mL L−1 day−1.91 In a recent investigation, polypropylene used in face masks was converted into carbon fibers.92 Unquestionably, it may meet the global dilemma of face mask recycling and/or upcycling for the creation of a sustainable environment and society and the development of more value-added products utilizing recycled carbon fibers for CO2 conversion.
Carbon brush
Carbon brush cathodes are ideal for MES because of their wide porous surface area, low resistance, and good electrical conductivity. The dense and organized bristle dispersion could prevent the biofouling of electrode materials.93 CO2 conversion efficiency was enhanced using Methanothrix species-coated carbon brush cathodes. DET was observed between Methanothrix species and the carbon brush, indicating that the carbon brush is an outstanding biocathode for MES.94 In addition, transcriptional analyses revealed that the Methanothrix species colonizing the carbon brush surface were actively expressing genes that code for the electromethanogenesis-specific enzymes instead of acetoclastic methanogenesis-specific enzymes.
Reticulated vitreous carbon (RVC) foam
RVC foam is an open porous network made of the electrically and thermally conductive vitreous carbon. 3D hierarchical porous morphology with excellent conductivity makes RVC materials suitable as candidates for MES. An improvement in the efficiency of MES for VFAs production was observed with 3D hierarchical RVC cathodes with large surface areas.69 The consistent 3D structure and abundance of active sites in the RVC foam coating promote mass dispersion and microbial development, thus increasing butyrate production. The results of the elevated sequencing demonstrated the selective enrichment of Firmicutes, a phylum that produces butyrate and is electrogenic. These findings imply that the large-pore network structure, conductivity, and selective microbial enrichment of RVC could improve the MES performance.78 The high surface-to-volume ratio of the electrode maximizes the surface area available for the development of biofilms, allowing for higher catalyst loading. In addition, the nanostructure enables the formation of a continuous electroactive biofilm with current densities up to −200 A m−2 and acetate production rates up to 1330 g m−2 day−1.78
MXenes
Due to their advantageous properties, including exceptional conductivity, ion intercalation behavior, and hydrophilicity, an emerging family of 2D materials called MXenes are viable candidates for cathode materials.95 After their discovery, MXenes have attracted a great deal of attention in both the scientific community and industry because of their outstanding material properties and environmental credentials. The cytotoxicity and biocompatibility of MXenes have been influenced by its concentration and surface coating thickness.96 For instance, exposure to high concentrations of TiC and MAX phase, up to 400 g mL−1, showed considerable cytotoxicity effects through mechanical and oxidative stress in human fibroblasts and HeLa cells.97 The CO2 conversion efficiency and microbial adherence can be improved by controlling the Tx group of MXenes.98 Owing to the high reactivity of the protons in –OH Tx termination, the adsorbed intermediates and biological agents could be connected to the catalyst surface via MO–H coordination by momentarily removing protons from the Tx group.
Nanosheets of 2D stacked MXenes have a large surfaces area and various functional groups that render them easy to modify to control their interactions with biomolecules or to change their conductivity, allowing them to be optimized for specific electrode applications.99 Both VFAs production and current density were increased by the MXene. As a result of the high surface roughness of electrode materials with MXene coatings, the electrical conductivity, charge transfer efficiency, and specific microbial enrichment properties were improved. The cathodic current production of MXene-coated cathodes was increased by 2.3 times compared to that of uncoated electrodes.28 The average Coulombic efficiency (%CE) and the cathodic current density of MXene-coated cathodes were 41% and 174.2 mA m2, respectively.30 The high electrode conductivity was attributed to the availability of more active sites. The biologically catalyzed redox reactions may be responsible for the increased MES performance. The active sites of MXene were the hollow surface gaps that developed between its stacked nanosheet architectures.30 Microbial growth was supported by the active sites of the cathode.
Biochar
The application of biochar, a robust material-based pathway for waste minimization and boosting the effectiveness of the circular economy, has increasingly gained attraction in the environment sector. It can be produced using a variety of strategies, e.g., through pyrolysis when carbonaceous materials are burned with little to no oxygen, or as a by-product of plasma catalytic liquefaction.100 The high porosity of biochar, combined with the presence of minerals, inorganic ions, and other physicochemical factors, promotes microbial breakdown, decomposition, and fermentation.101 Biochar is a good biocathode that has the potential to reduce the energy and carbon footprint associated with the fabrication of electrodes.102 By immobilizing different Clostridium species, biochar electrodes could protect them and lessen their inhibitory effects while enhancing the synthesis of caproic acid from CO2.102 Biochar can also absorb long-chain products such as caproic acid due to its porous structure and high specific surface area (89 m2 g−1), and thereby, it can reduce the toxicity of bacteria such as Clostridium kluyveri. Reduced lag phase and a rise in the caproic acid production of up to 0.50 g L−1 were observed in MES experiments that used biochar.
A recent study suggested using 1.0 g g−1 dry matter sludge-derived biochar to increase and stabilize the MES performance, where the bacterial species Thermincola were grown on the anode and cells of Methanothermobacter on the cathode.103 The findings revealed that in comparison with the control group, biochar significantly increased VFAs production by 17.9% and CH4 production by 24.7%. It should be noted that biochar is an important electrode material for other processes that aim to capture and convert greenhouse gases. For example, recent findings have shed light on how plasma and biochar could positively improve CO2 conversion.104 At a flow rate of 7 L min−1, a maximum CO2 conversion of 10.0% was achieved in the plasma CO2 breakdown process, while the energy efficiency reached ∼28.4%. CO2 conversion and energy efficiency were enhanced as biochar was added to CO2 plasma, even at modest flow rates of about 6 L min−1.104
The key findings of studies involving recent carbonaceous materials in the production of high-value-added compounds from CO2 using MES are summarized in Table S2 (ESI†). The high specific surface area, electrical conductivity, electrochemical stability, and biocompatibility are the major advantages of carbonaceous electrode materials. The common advantages and disadvantages of the carbonaceous materials are given in Table S3 (ESI†). Microbial adherence is influenced by a wide range of electrode geometries and material properties, which has an impact on the biofilm thickness and electron transfer rate. Engineering of porous 3D electrode and 3D bioprinting are the most significant state-of-the-art technologies to enrich the product yield of CO2 conversion in MES.
The utilization of appropriate surface modifiers and additives could facilitate the microbial adherence by altering the surface functionality of carbonaceous materials. Another important factor affecting CO2 conversion in MES is biofilm thickness, which was not considered in most of the recent findings. A significant improvement in the CO2 conversion efficiency could be achieved by a small increase of the inner biofilm thickness for porous 3D carbon electrodes.105 The thickness of biofilm formation is directly influenced by the total effective surface area per volume of the reactor. The high performance of a carbonaceous electrode material relies on the surface area, porosity, biofilm thickness, and efficient electron utilization by the microbes. Nevertheless, the structure-activity relationship and the crucial physicochemical features of the state-of-the-art carbonaceous materials have not been investigated in detail.
The product yield and faradaic/Coulombic efficiency of an MES-based CO2 conversion process are ruled by various factors such as the applied potential, reactor configuration, nature of microbe, characteristic of electrode-microbe interface, influence of redox mediators, CO2 flow rate, reactor operation mode, volume of reaction mixture, starting substrate concentration, and operation cycle time. Consequently, assessing the benefits and drawbacks of carbonaceous electrode materials is highly complicated because the performance of each material is unique in terms of current density, product yield/quality, type of microorganisms, biofilm growth, etc. Consequently, the microbe-electrode interfacial reactions and electron transfer mechanisms should be studied in detail using the advanced spectroscopic techniques (e.g., electrochemical surface plasmon resonance, electrostatic microscopy, and other in-situ spectroscopy techniques).
Compared to electrocatalytic CO2 conversion, the poor voltage efficacy of MES is one of the primary unsatisfactory elements. This can be avoided by adopting a full analytical framework to improve the MES reactor designs for achieving high current densities at low applied voltages. The significant overpotentials of an electrochemical system are cathode activation, Ohmic, and concentration overpotentials. The activation threshold for a specific electrochemical process and the characteristics of the catalysts in exceeding the activation barrier are connected to the activation overpotential. Ohmic overpotential relies on the conductivity of the electrolyte and is related to the movement of ions between the anode and the cathode. To overcome the cathode overpotential, mass transfer between the anode and the cathode should be improved, either employing innovative reactor designs or local surface hydrodynamics.
Despite having the greatest of all overpotentials in classical electrolysis cells, cathode overpotential is substantially lower than that in MES. Ohmic overpotential in the MES can be reduced using carbonaceous electrodes with a large surface area and reactor configurations without providing a significant space between the anode and the cathode. Density functional theory (DFT), COSMOL multiphysics, and microkinetic models can be used to minimize the overpotential in MES by investigating the reaction kinetics, surface features of electrode materials, bonding of key intermediates, the influence of redox mediators, and reactor configurations.6
5. Biocompatible carbonaceous electrolytes
Although the effectiveness and conversion rate of MES is yet to be optimized to the level that would make it feasible for commercialization and scale up, it still offers a promising method for CO2 conversion. One of the ways in which the current limitation could be overcome is through the engineering of different types of natural deep eutectic solvents with the needed level of biocompatibility, which can serve as the co-electrolyte in the MES for CO2 conversion. In addition to conventional H-shaped reactors or dual-chamber flow reactors, solid-state electrolytes, such as proton-conducting perfluorinated sulfonic acid polymer, provide good electrical conductivity and prevent the usage of salts that can contaminate the liquid product stream, which can be used in MES.106 With adjustable CO/H2 ratios, the integrated electrocatalytic membrane electrode might well produce flexible syngas feedstock from CO2, greatly increasing the versatility of downstream syngas bioconversion to valuable chemicals.
Investigations on the effects of diverse microbial growth media on electrocatalysis revealed that the systems consistently produced syngas at >90% faradaic efficiencies using Clostridium strains.66 Serine glycerol electrolyte increased the yield of methanol production in the electro-enzymatic conversion of CO2 by two times compared to the Tris–HCl buffer (0.22 mM) and 16 times compared to the control reaction (i.e., the enzymatic reaction in the absence of NADH regeneration).107 Polymeric electrolytes such as amphiphilic polycarbonates have low Tg values. A significant percentage of polar carbonyl groups in aliphatic polycarbonates are conducive to ion transport and provide strong solubility for a variety of salts.108
6. Product selectivity
One of the key advantages of MES lies in its potential to produce a wide range of commercial products via anthropogenic CO2 conversion. As already discussed, the electron transfer process is to a large degree governed by the properties of the cathode, which offers areas for microbial film development and attachment. In addition to cathode development, improvements in the chain length of end products and the current density may be realized using genetically engineered microorganisms as these allow for the fine-tuning of bioelectrode performance. For example, genetically-modified Shewanella oneidensis bacteria were used to modify biocathodes for ethanol production.109 The schematic of the production of various small-chain and long-chain chemicals from CO2 conversion using MES and carbon biocathodes is shown in Fig. 5.
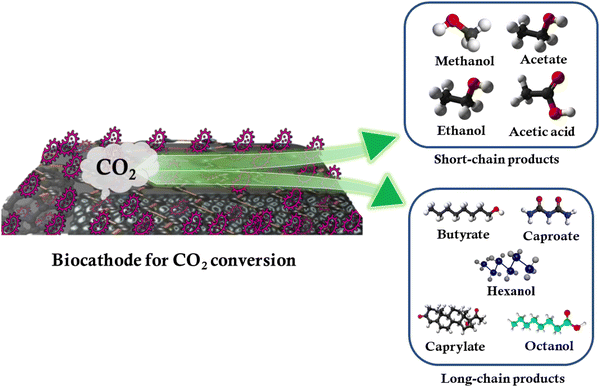 |
| Fig. 5 Schematic of the production of various small-chain (methanol, ethanol, acetic acid, and acetate) and long-chain chemicals (butyrate, caproate, caprylate, and octanol) from CO2 conversion using MES and carbon biocathodes. | |
Small-chain products
Cathode modification enhances electrode-microorganism electron transport, resulting in increased MES productivity and selectivity. Limited studies have been carried out on the influence of electrode materials on microbial proliferation to synthesize specific compounds. Cathodes modified with cobalt phosphide, MoS2, and Ni-Mo alloy have recently been employed for the selective synthesis of C2 and C4 compounds.110 To minimize the agglomeration and dissolution of metal oxide nanoparticles, they must be deposited on appropriate conducting surfaces such as carbonaceous electrodes due to their excellent flexibility and porosity. A great majority of C1 gas fermentation research has been carried out in stirred tank reactors,111 where a gas-liquid mass transfer can be boosted through increasing impeller speed, which separates the gas stream into smaller bubbles with a higher specific surface area.112 Acetate is among the most widely prepared small chain value-added products from CO2 conversion in MES. Products such as butyrate, isobutyrate, ethanol, and isopropanol have also been produced by MES. Studies reveal that mesophilic conditions delivered the highest quantity (525.84 ± 1.55 mg L−1) and the fastest rate of acetate synthesis (49.21 ± 0.49 mg L−1 day−1). Compared to psychrophilic and thermophilic conditions, the relative abundance of the Bacteroidetes phylum in the biofilm significantly increased under a mesophilic environment. It was observed that Clostridium, Treponema, Acidithiobacillus, Acetobacterium, and Acetoanaerobium dominated the genera in the biofilm under mesophilic conditions; however, the genera diversity reduced under psychrophilic and thermophilic conditions.113
Long-chain products
Owing to the significance of multi-carbon products, the potential for MES to produce long/medium-chain organic molecules has been actively researched in recent years. Medium-chain fatty acids are superior to short-chain fatty acids because they are the building blocks for various fuels and chemicals.114 Chain elongation can be used to synthesize up to C4 products such as caproate, valerate, and caprylate, as well as isoamyl alcohol, which has significantly increased the practical feasibility of MES in CO2 conversion. It was carried out by modifying a particular metabolic process. Microbial chain elongation and the design of biochemical reactors play an important role in the long-chain product formation. Long-chain hydrocarbons, such as gasoline, have a high energy density and octane rating. It is well known that the complex reaction pathways and severe thermodynamic restrictions associated with MES CO2 conversion make it difficult to achieve multiple-carbon fuels. In the presence of organic electron donors, such as ethanol, lactate, and carbohydrates, chain elongation has been considered an interesting bioprocess for converting short-chain carboxylates, such as acetate and n-butyrate, into medium-chain carboxylates, such as n-caprylate and n-caproate, with H2 as the by-product.115 Using CO2 and ethanol as the binary electron donors, a maximum caproic acid production rate of 2.41 g L−1 day−1 and a concentration of 7.66 g L−1 were attained.116 Nevertheless, further studies must be carried out on EET processes and microbial roles in complex microbiomes in the generation of alcohol and longer-chain carboxylates. The production of isobutyric, n-butyric, and n-caproic acids as well as their corresponding alcohols, utilizing CO2 as the only carbon source, was reported with the help of a specific type of mixed reactor microbiota.12 Genomic sequencing research supports the concept that isobutyric acid is created via the isomerization of n-butyric acid and that this process includes acetogenesis, solventogenesis, and reverse oxidation.
7. Thermodynamics and kinetics
Microorganism that are in syntrophic relationships with one another all over various phyla or orders of the same phylum are motivated by energetic boundaries. The thermodynamics of the intermediate reactions are altered by these energetic boundaries.
stands for the usual applied external voltage because CO2 bio-reduction is non-spontaneous in nature. According to the equation, it is the electromotive force between two half-cells, namely, the cathode half-cell and the anode half-cell. |  | (1) |
As indicated by eqn (1), the bio-electrochemical reactions are typically reversible in nature, particularly for CO2 bioreduction to high-value-added products (VAP).where ED stands for electron donor, and a, b, and c are stoichiometric constants. The equilibrium constant K for such a process is defined as follows. | 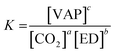 | (3) |
The equation allows for a relationship between the equilibrium constant “K” and the standard Gibbs energy as follows. | ΔG° = −RT ln K | (4) |
Here, T is the absolute standard temperature and R is the ideal gas constant (8.314 J mol−1 K−1).
Based on whether the transformation in the path of the formation of value-added products is exothermic or endothermic, CO2 bio-reduction processes can be classified as either spontaneous or non-spontaneous from a thermodynamic perspective. Spontaneous reactions are known as favorable reactions when they have a negative ΔG° value and unfavorable reactions when they do the opposite.
The thermodynamic favorability is highly influenced by the type of electron donor and is not always present. Particularly in comparison to this, electron donors have a wide range of intensive and extensive properties that can influence how spontaneous a biochemical reaction is. The anode potential plays a crucial role in CO2 bioreduction by pushing the electrons in that direction. The quantity of metal oxides in the electrodes has a significant impact on the anode potential. According to a recent study, the next oxidation reaction causes the Fe3O4 layer to form on the anode in the iron-water system.117
| 3Fe2+ + 4H2O →Fe3O4 + 8H+ + 2e− | (5) |
The following equation describes the anode potential of this system.
| E = 0.98 − 0.236 pH − 0.0886log[Fe2+] | (6) |
This demonstrates that the
E° anode is not solely reliant on the concentration of Fe(
II) ions. The
E° anode for a 1 M Fe(
II) solution at pH 7 would be 0.672 V. The anode overpotential issue, nevertheless, would cause the actual potential to be higher than this. The loss in reaction activation is a representation of this overpotential. Comparable to how it influences microbial function in biochemical reactions, Fe(
II) concentration has an impact on both.
The favorable scenario for the upstream generation process and the downstream usage method for CO2 bio-reduction was indicated by the low partial pressure of intermediate electron donors.118 Furthermore, a significant factor affecting the solubility in water is the partial pressure in the gas phase. In this case, increased partial pressure is necessary to ensure more gas in the liquid phase. Thermodynamic analysis showed that the most spontaneous reaction was the formation of acetic acid, which was followed by n-caproic and n-butyric acids, while the conditions chosen limited the production of alcohols.119 This study clarifies the discrepancies between thermodynamic predictions and experimental findings, enabling the best working conditions and the highest hydrocarbon concentration from a single substrate. There is currently a well-established thermodynamic technique for understanding and predicting biomass yields for microbial growth processes that are based on dissipated Gibbs energy. It also indicates that a thermodynamic technique can be utilized to assess the maintenance needs. Thermodynamic concerns in methodology are largely concerned with the ability to forecast stoichiometric co-effluents. Furthermore, it would be beneficial to evaluate any potential impacts of temperature or substrate and media component concentration on the growth processes.
As CO2 is absorbed by water, its density rises, it leaves the interphase, and is replaced by water with low CO2 concentration. As the water becomes saturated with CO2, the rate of CO2 absorption slows down from its initial high rate. For practical purposes, it can be assumed that a saturated gas-liquid interface will remain so. The absorption of CO2 is strongly influenced by temperature and rises as the temperature falls. Five different cathode types were tested in recent research, and in each cathode, CO2 was sparged every 24 h. More CH4 formation was observed because of this step, which was discovered as there was a peak in the electron flow in response. The lag phase was also extremely short because CO2 was the sole substrate available, and periodic CO2 sparging ensured that there was enough substrate for the initial growth phase. Otherwise, CO2 bioreduction to CH4 typically exhibits a distinct latent period.120
There has been limited research on the kinetics of microbial CO2 conversion. Due to the thermodynamic and kinetic constraints of CO2 conversion, the interfacial reactions emerging at the electrode are the rate-determining steps. At the interface between the two reaction phases, the composition of the substrate following the microbial hydrolysis reaction can be quantitatively measured. The hydrolysis products, primarily short-chain fatty acids, serve as a substrate for biogas generation by acetogenic and methanogenic bacteria in the second reactor stage. The fundamental CO2 conversion methods are biochemical transformations with and without voltage application.
As described in the thermodynamics section, an electron donor's choice can influence the reaction's overall preference. The condition of the electron donor is critical in this scenario. Several kinetic processes, such as mass transfer, charge transfer, and microbial growth, occur concurrently in CO2 reduction, resulting in a complex and difficult-to-determine rate-controlling step. As a result, while determining which of the kinetic phases in CO2 reduction is constraining the creation of value-added products, the system and its situation must first be specified.121 The rate-limiting steps in both the operations are different. The main rate-controlling step in the scenario of CO2 reduction without applied voltage is the formation of a reducing equivalent. Nevertheless, in the case of CO2 reduction with applied voltage, the microorganisms still need time to adjust to the circumstances, and a lag phase arises that can last from hours to days. However, the electron transport mechanism is commonly thought to be rate-limiting. Another rate-controlling factor is that H2 is practically insoluble in water.36 The risk increases when the solution contains various salts, and the temperature is typically not optimal for microbe operation. To assess the rate-limiting step, both the reacting species and their circumstances must be considered.
To determine the level of detail required to depict kinetics from differential to total conversion, various kinetic models are available. These models include power laws, power laws with inhibition, and Langmuir–Hinshelwood–Hougen–Watson (LHHW) approaches. The approach of Weatherbee and Bartholomew, which was recently used for the methanation of carbon monoxide (CO), is analogous to how LHHW-type rate equations are calculated. The first proposed mechanism, (CO2 + 2˙ → CO˙ + O˙), considers the first cleavage of carbon-oxygen bonds, followed by the hydrogenation of carbon and carbenes to CH4 and the hydrogenation of adsorbed oxygen to water. It is believed that CH4, CO2, and H2 will adsorb dissociatively. The mechanism used here is similar to the prediction by Weatherbee and Bartholomew. The differences among trials and all hypotheses are checked on equality in Bartlett's X2 test, which is used to distinguish amongst LHHW models. If it is revealed that this hypothesis is wrong, the model with the highest difference between trial and prediction is eliminated.
8. Challenges and prospects
There are significant challenges in developing a commercially sustainable CO2 electro-biorefinery process. According to a recent techno-economic analysis of MES with CO2 conversion, power consumption accounts for up to 69% of the primary operational costs.26 In the environmental and bioenergy industries, MES-based CO2 conversion is a very interesting and promising technology as it not only reduces GHG emissions but also converts CO2 into value-added bioproducts to ease the energy crisis. Nevertheless, several drawbacks prevent MES from being used in real-world situations.
In most recent studies, chemical processes have been mainly employed to produce carbonaceous electrode materials for MES. The environment could suffer significant damage from these chemical methods. It would be an excellent strategy as the electrode materials could be engineered from waste products under the context of the circular economy. For instance, multiwalled CNT electrode was fabricated from plastic packaging waste and successfully applied in supercapacitors.122 The capacitive performance of these CNTs was superior compared to that of chemically-derived commercial CNTs. This finding is highly advantageous regarding the potential use of waste-derived CNTs for MES CO2 conversion, which could be a positive development for circular economy.
The oxygen evolution reaction dominates the anode reaction in MES systems, which splits water. This poses a problem for MES design and development. To reduce resistance, prevent toxicity, and efficiency losses, oxygen penetration to the cathode must be prevented. To resolve these challenges, there is a strong need to identify novel reactor designs:
(a) The use of mixed microbial cultures to excavate away oxygen.123
(b) Replacing the anode reaction––the most effective strategy to avoid any harm from oxygen to the cathodic reaction is certainly its avoidance.105,124 An anode reaction that fails to produce oxygen or hydrogen peroxide does not affect cathodic bacteria. The reaction of oxygen evolution demands a significant overpotential on several anode materials. As a result, the oxidation of chemical compounds at lower overpotentials in these materials appears to be highly appealing.
(c) Purging inert gas such as nitrogen into the cathode chamber, as is done for other obligatory anaerobic bioprocesses, can be an alternative for small-scale laboratory research to ensure anoxic conditions.125
(d) Employing customized membranes, such as polymer materials designed for use in abiotic chemical reactors. Adopting bipolar membranes has the additional benefit of decreasing the voltage required for the cathode reaction via a pH gradient across the membrane.126,127 There is no magic solution to fix these problems, and because there is no “yet another” solution in MES concepts, goal levels, and financial constraints will all play important roles in determining which path to take to minimize the problem.
During the CO2 conversion process, product crossover is very difficult to tackle even with ion-selective membranes as the products are rarely charged.128,129 The applied voltage, flow rate of the catholyte, and type of anion exchange membrane govern the product crossover. Therefore, reliable computational models should be developed to prevent product crossover and to increase the CO2 conversion efficiency.
Fabrication of novel materials
Many biocathodes cannot be synthesized using the current methods in a way that is practical for industrial use. The required cathode should have the following features for usage as a biocathode: higher yield, great chemical resilience, biocompatibility, good mechanical strength, surface area, and low cost. The features of cathode materials are critical in electrohydrogenesis and electromethanogenesis processes. The technical barriers to commercialization should be overcome by creating highly scalable synthesis methods for carbonaceous bioelectrodes. Among the simplest ways to tackle this problem is through 3D bioprinting technology and cold plasma to control the microbial phenotype/genotype, and biomass accumulation. Even then, more research will be needed in the future to build flexible, durable, and more effective carbon materials for MES.
Kinetics and theoretical models
Computational modelling of MES has been underutilized to date, but it is required to reach a breakthrough knowledge of the process-limiting phases. Accurate thermodynamic and kinetic models must be established to increase the productivity and the characteristics of MES at the molecule/system levels. CO2 is the primary feedstock and the sole carbon source in most MES reactors; its concentration has a significant impact on cell dynamics. Because of its quite high half-saturation constant and limited solubility, microbes are extremely sensitive to minor variations in soluble CO2 concentration. A model suggests that using pure CO2 as a feeding gas can attenuate this impact, as demonstrated experimentally.121 It is crucial to highlight, however, that eliminating a kinetic barrier may not be sufficient to significantly enhance output because CO2 diffusion could be the primary limitation at a certain time. The findings also showed that the CO2 delivery strategy has a significant impact on reactor performance. Because mass transfer coefficients are rarely provided in MES studies, it is impossible to determine whether inefficient CO2 supply systems are one of the reasons that attained output rates remain low across the field.
Novel micro-organisms
Some novel microorganisms found in salty environments (halophiles) such as mangroves and salt marshes provide good habitats for CO2 fixation species and play a significant role in the carbon cycle. Saline conditions may serve as an appropriate venue for the development of innovative and efficient CO2-fixing organisms capable of producing value-added products with diverse metabolism. Presently, Newlight Technologies, California, USA, is converting air and GHG into a natural biomaterial that can be converted into value-added products utilizing microorganisms found in the ocean. Prospective MES-based CO2 conversion includes the use of hybrid systems composed of autotrophs for CO2 reduction and heterotrophs for further output conversion after CO2 reduction. Parallel to this, hybrids of autotrophs at the biocathode and heterotrophs at the anode could be employed to simultaneously treat organic waste streams while saving energy. If thermophilic bacterial populations could be adapted to function at higher temperatures, the device performance may be raised only through a modification of the system parameters.
Novel strategies to modify microbial performance and biomass accumulation
It has already been mentioned that genetic modification has been used to control the behavior of microbial populations, and in doing so improve the efficacy of the MES conversion process and selectivity of the products that result from this conversation. In addition to the direct modification of the genome, desirable changes in the phenotype and genotype of the microorganisms, as well as their cell-surface interactions and biofilm-forming ability, may be improved through other means, e.g., plasma treatment. Here, the plasma is typically a mildly ionized gas that is generated when electricity is applied to a gas or a mixture of gases. The applied electric field provides energy to the light electrons, which then activate the gas molecules via excitation, ionization, and dissociation, producing the reactive species and enabling chemical reactions to take place under ambient conditions while maintaining the bulk temperature of the gas low, in some cases at room temperature (with the sub-type of plasma typically referred to as ‘cold plasma’).
Plasma-based technologies have already demonstrated their potential for CO2 conversion as electrons may activate CO2 molecules, resulting in new compounds without the need to heat the entire gas.130 This allows for reactions such as CO2 splitting and dry reforming of CH4, which would be thermodynamically expensive otherwise. Nevertheless, there are numerous constraints in the energy efficiency, conversion, and product selectivity of CO2 conversion employing plasma.
In the field of biotechnology, however, plasma treatment offers possibilities for improving the efficiency of a variety of bioprocesses, from the bioremediation of waste materials to the synthesis of therapeutic molecules. Depending on the treatment dose and regime, a combination of oxidative stress from plasma-generated reactive oxygen and nitrogen species, UV irradiation, mild heating, and drying from gas flow effects may induce a number of desirable responses in cells. These may include increased solute mass transfer across the cell membrane. In addition to this, the changes in the metabolic activity can increase the catalytic activity and promote biofilm formation.
Plasma treatment can induce random mutations, with a selective pressure then used to select mutants with the desired set of properties, such as tolerance to specific physical or chemical parameters of the process. According to recent reports, the investigation used Geobacillus sp. strain WSUCF1, a thermophile able to produce cellulolytic enzymes with increased activity. In comparison to the control, a 4 min treatment with cold plasma increased the rates of glucose conversion by 74% and biomass yield by 60%. Plasma-treated WSUCF1 also showed improved microbial growth.131 Varied bacterial species and their knockout mutants can be affected by atmospheric cold plasma, with such parameters as treatment duration influencing the treatment outcome.132
One of the key advantages of cold plasma treatment lies in its flexibility. The optimization of reactor architecture and operation parameters (carrier gas, applied voltage, and frequency) can be used to control the nature of physical/chemical effects, and hence the biochemical reactions that take place. Such amenability to customization makes the integration of plasma into other processes easier, especially when compared to other methods that could be used to stimulate cellular activity. However, it is often difficult to deconvolute the specific mechanisms that underpin treatment-response relationships, including the impact of plasma exposure on gene expression, bacterial adaptive signaling pathways, and resistance over the short/long term. Therefore, a deeper comprehension of bacterial reaction to various stressors is required, as doing so may help disclose comprehensive pathways of plasma-induced effects. Additional research could assess the degree to which virulence or other biological characteristics are altered in response to stress and resistance-related gene expression. This would advance knowledge on managing and lessening these occurrences.
In the context of MES, the use of plasma-mutated or plasma-stimulated microbial populations may improve the effectiveness and product selectivity of MES. Where plasma is used for stimulation of bioactivity, by facilitating thermodynamically upstream processes with non-thermal plasma, one can boost the efficiency and selectivity while preventing catalysts sintered at room temperature and pressure. Even though the interaction between the plasma and the catalyst surface is not always well understood, it allows for rapid start-up and shutdown cycles in addition to functioning at low temperatures.
Commercialization
Prior to introducing the appropriate carbonaceous cathode architecture into large-scale industrial applications, several operation conditions, especially gas diffusion at the cathode-microbe-media interface, should be optimized.
PyroCO2 is a European Green Deal project, which is a revolutionary platform that will convert industrial CO2 to chemical building blocks utilizing a novel biochemical technique, in which CO2 is subsequently transformed into a variety of value-added products. From 9,100 tonnes of industrial CO2 and green H2, the PyroCO2 prototype plant is expected to generate at least 4000 tonnes of acetone each year.
Kiverdi industry, California, USA is using NASA (National Aeronautics and Space Administration)-inspired carbon-transforming technology to address global warming problems. It provides a solution for reverse plastic, CO2 aquafeed, air protein, revives soil, and unique cycle. The business turns CO2 into goods made of biological materials. The innovations of this company would be beneficial for the circular economy. In particular, the air protein would be exceptionally advantageous to address the challenge of feeding the astronauts for long-term space missions.
Utilizing microbes and renewable energy, Evonik and Siemens intend to transform CO2 into specialty compounds. The two businesses are engaged in a collaborative research effort named Rheticus that focuses on electrolysis and fermentation techniques. The initiative, which will last for two years, was just inaugurated. The first test plant at the Evonik facility in Marl, Germany has planned to manufacture chemicals such as butanol and hexanol, both of which serve as feedstocks for products such as special plastics and nutritional supplements. The following phase could involve the construction of a factory with a 20
000 tonne annual production capacity. Other specialized chemicals or fuels could also be potentially produced.
Cemvita industry, USA is working on in-space biomanufacturing for deep space exploration, low carbon bioprocessing technologies for the mining industry, and carbon capture, use, and storage for the oil, gas, and petrochemical industries. By eliminating 250 million tonnes of CO2 from the environment annually by 2050, Cemvita and its partners will help reverse climate change.
Integrating microbial CO2 conversion with other industrial processes such as wastewater treatment, flue gas treatment, biogas upgrading, and direct CO2 conversion to value-added products will be more efficient. This might help to address the significant environmental concerns posed by global warming while also reducing typical barriers related to the cost and effectiveness of massive microbial CO2 fixation and conversion technologies in the future.
Energy efficiency
The price of electricity for MES processing may be another barrier for scaling up this technology. Several techniques for reducing energy input could be implemented, including the integration of renewable energy sources with MES, the decrease of anodic oxygen evolution reaction overpotential, and the chain elongation of short-chain fatty acids to medium chain fatty acids for easy extraction. More investigations on electricity-driven microbial chain elongation are needed to establish a medium chain fatty acids production process that is efficient, reliable, and controllable. To detect energy consumption from the various components in MES, a full energy analysis should be performed.
9. Summary and outlook
MES is one of the most exciting approaches for resolving environmental crises such as the depletion of fossil fuels and global warming. It is a potential technique to produce carbohydrates, proteins, clean fuels, and various value-added chemicals from anthropogenic CO2. An overview of the significance of the state-of-the-art carbonaceous electrodes for MES CO2 conversion has been presented in this study. The application of MES to CO2 conversion will advance in both completeness and flexibility with the introduction of new technologies and concepts.
The development of low-cost electrode materials with superior performance is essential to overcome the challenges in MES-based CO2 conversion. Consequently, carbonaceous electrode materials have been employed to enhance the CO2 conversion rate since they are biocompatible, highly conductive, and chemically stable with exceptional electrocatalytic activities. Carbonaceous materials such as CNTs, graphene, GO, grow, graphite, GF, g-C3N4, AC, CF, CDs, carbon fibers, carbon brush, RVC, MXenes, and biochar have been investigated for MES-based CO2 conversion. The development of 3D hierarchical porous morphology may improve the adsorption of intermediates on the bioelectrode surface. The capacity to form biofilms and electron transfer rate could be enhanced by adjusting the electrical characteristics of carbonaceous materials.
The upward potential of MES is greatly influenced by the diversity of microbial cultures, electrode materials, and bioproducts. Regarding the kinetics and thermodynamics of MES-based CO2 conversion using carbonaceous electrodes, the investigations are extremely constrained. The most suitable materials to expand the MES technology to the industrial level are 2D and 3D carbonaceous materials. Among them, porous 3D materials with a large specific surface area could improve microbe-electrode interaction, electron transfer rate, gas diffusion, substrate mobility within the electrode, and electrochemical performance.
Carbonaceous electrode materials should be engineered from waste products under the context of the circular economy. Highly scalable and ecofriendly fabrication strategies should be developed to conquer the technical barriers in commercialization. The flexibility, durability, effectiveness, biocompatibility, and product selectivity of the state-of-the-art carbonaceous electrodes should be further improved for validating and demonstrating the MES-based CO2 conversion technology at a large scale. Compared to conventional techniques (dip/spray/spin coatings, and electrodeposition), 3D bioprinting is an innovative approach to engineering the biofilm-coated electrodes with unique features to achieve high product selectivity. Reliable theoretical models should be developed at the molecule/system level to realize the significant rate-limiting steps, reactions at the electrode-microbe interface, extracellular electron transfer mechanism, formation/bonding of intermediates, product crossover, and product selectivity in detail.
Cutting-edge plasma technology can be utilized to improve CO2 conversion. The outcomes of CO2 dissociation by cold plasma and plasma-induced synergistic effects have been described. Continuous stirred tanks are giving way to attached growth bioreactors such as membrane and trickling bed (Fig. 2(d)). It appears that attachment growth can maintain a high level of microbial biomass within the system, hence increasing the efficiency of the system. Product selectivity in MES is primarily determined by the genetically engineered bacteria involved, the type of reactors, and electrode materials used. Utilizing the appropriate microbes (mixed or pure culture) and reactor designs could improve the CO2 conversion efficiency to produce long-chain products.
Overall, the industrial application of MES-based CO2 conversion technology holds a promising sustainable future with a green economy and environment. In the years ahead, these carbonaceous materials will be crucial for various technologies including energy, healthcare, bioenergy, and the ecosystem. To achieve our vision of sustainable infrastructure and launch additional enterprises generating fuels from CO2, more economic advancements in this area are necessary. MES-based CO2 conversion would be a critical technology for establishing a colony on Mars, which could be beneficial for developing rocket fuels and bioderived products using the CO2 in the Martian atmosphere.
Author contributions
The main idea of this review was from VK. The literature search and the original draft preparation were performed by GSL. The critical revision and review were conducted by VK. VK, KB, and SR were involved in the editing and discussion of the key findings.
Conflicts of interest
There are no conflicts of interest to declare.
Acknowledgements
This research was conducted as a part of the International Research Agendas PLUS programme of the Foundation for Polish Science, co-financed by the European Union under the European Regional Development Fund (MAB PLUS/2019/11). K. B. acknowledges the funding form the Australian Research Council (FT190100819 and DP180101254), and the Australian National University Futures Scheme.
References
- M. Strack, S. J. Davidson, T. Hirano and C. Dunn, Curr. Climate Change Rep., 2022, 8, 71–82 CrossRef.
- E. Rousi, K. Kornhuber, G. Beobide-Arsuaga, F. Luo and D. Coumou, Nat. Commun., 2022, 13, 3851 CrossRef CAS PubMed.
- D. I. Drautz-Moses, I. Luhung, E. S. Gusareva, C. Kee, N. E. Gaultier, B. N. Premkrishnan, C. F. Lee, S. T. Leong, C. Park and Z. H. Yap, Proc. Natl. Acad. Sci. U. S. A., 2022, 119, e2117293119 CrossRef CAS PubMed.
- M. Alexander, M. Eissa, R. McDermott-Levy, R. Osborne, E. Pleuss, P. Prabhakaran and C. Sorensen, J. Climate Change Health, 2022, 5, 100117 CrossRef PubMed.
- P. T. Anastas and J. B. Zimmerman, Curr. Opin. Green Sustainable Chem., 2018, 13, 150–153 CrossRef.
- V. Kumaravel, J. Bartlett and S. C. Pillai, ACS Energy Lett., 2020, 5, 486–519 CrossRef CAS.
- A. Behera, A. K. Kar and R. Srivastava, Mater. Horiz., 2022, 9, 607–639 RSC.
- M. M. Ayyub and C. Rao, Mater. Horiz., 2021, 8, 2420–2443 RSC.
- W. Zhang, D. Ma, J. Pérez-Ramírez and Z. Chen, Adv. Energy Sustainability Res., 2022, 3, 2100169 CrossRef.
- R. Gupta, A. Mishra, Y. Thirupathaiah and A. K. Chandel, Biomass Convers. Biorefin., 2022 DOI:10.1007/s13399-022-02552-8.
- M. C. M. Yau, M. Hayes and S. Kalathil, RSC Adv., 2022, 12, 16396–16411 RSC.
- D. A. Jadhav, S.-G. Park, S. Pandit, E. Yang, M. A. Abdelkareem, J.-K. Jang and K.-J. Chae, Bioresour. Technol., 2022, 345, 126498 CrossRef CAS PubMed.
- J. Lai, A. Nsabimana, R. Luque and G. Xu, Joule, 2018, 2, 76–93 CrossRef CAS.
- L. Chen, H. Yu, J. Zhang and H. Qin, RSC Adv., 2022, 12, 22770–22782 RSC.
- S. Bajracharya, A. Krige, L. Matsakas, U. Rova and P. Christakopoulos, Chemosphere, 2022, 287, 132188 CrossRef CAS PubMed.
- C. W. Marshall, D. E. Ross, E. B. Fichot, R. S. Norman and H. D. May, Appl. Environ. Microbiol., 2012, 78, 8412–8420 CrossRef CAS PubMed.
- T. de Campos Rodrigues and M. A. Rosenbaum, ChemElectroChem, 2014, 1, 1916–1922 CrossRef CAS.
- S. Bajracharya, A. ter Heijne, X. D. Benetton, K. Vanbroekhoven, C. J. Buisman, D. P. Strik and D. Pant, Bioresour. Technol., 2015, 195, 14–24 CrossRef CAS PubMed.
- S. Bajracharya, K. Vanbroekhoven, C. J. Buisman, D. Pant and D. P. Strik, Environ. Sci. Pollut. Res., 2016, 23, 22292–22308 CrossRef CAS PubMed.
- N. Aryal, A. Halder, P.-L. Tremblay, Q. Chi and T. Zhang, Electrochim. Acta, 2016, 217, 117–122 CrossRef CAS.
- J. B. Arends, S. A. Patil, H. Roume and K. Rabaey, J. CO2 Util., 2017, 20, 141–149 CrossRef CAS.
- I. Vassilev, P. A. Hernandez, P. Batlle-Vilanova, S. Freguia, J. O. Krömer, J. R. Keller, P. Ledezma and B. Virdis, ACS Sustainable Chem. Eng., 2018, 6, 8485–8493 CrossRef CAS.
- L. Jourdin, S. M. Raes, C. J. Buisman and D. P. Strik, Front. Energy Res., 2018, 6, 7 CrossRef.
- S. Gadkari, M. Shemfe, J. A. Modestra, S. V. Mohan and J. Sadhukhan, Phys. Chem. Chem. Phys., 2019, 21, 10761–10772 RSC.
- L. Jourdin, M. Winkelhorst, B. Rawls, C. J. Buisman and D. P. Strik, Bioresour. Technol. Rep., 2019, 7, 100284 CrossRef.
- L. Jourdin, J. Sousa, N. van Stralen and D. P. Strik, Appl. Energy, 2020, 279, 115775 CrossRef CAS.
- A. Krige, U. Rova and P. Christakopoulos, J. Environ. Chem. Eng., 2021, 9, 106189 CrossRef CAS.
- K. Tahir, W. Miran, J. Jang, N. Maile, A. Shahzad, M. Moztahida, A. A. Ghani, B. Kim, H. Jeon and D. S. Lee, Sci. Total Environ., 2021, 773, 145677 CrossRef CAS PubMed.
- D. Thatikayala, D. Pant and B. Min, Sustainable Energy Technol. Assessments, 2021, 45, 101114 CrossRef.
- K. Tahir, W. Miran, J. Jang, A. Shahzad, M. Moztahida, B. Kim and D. S. Lee, Chem. Eng. J., 2020, 381, 122687 CrossRef CAS.
- T. Okoroafor, S. Haile and S. Velasquez-Orta, J. Hazardous, Toxic Radioactive Waste, 2021, 25 DOI:10.1061/(ASCE)HZ.2153-5515.0000537.
- Y. Wu, W. Li, L. Wang, Y. Wu, Y. Wang, Y. Wang and H. Meng, Chemosphere, 2022, 308, 136088 CrossRef CAS PubMed.
- M. Roy, N. Aryal, Y. Zhang, S. A. Patil and D. Pant, Curr. Opin. Green Sustainable Chem., 2022, 35, 100605 CrossRef CAS.
- H. Wu, H. Pan, Z. Li, T. Liu, F. Liu, S. Xiu, J. Wang, H. Wang, Y. Hou, B. Yang, L. Lei and J. Lian, Chem. Eng. J., 2022, 430, 132943 CrossRef CAS.
- S. Y. Lee, Y.-K. Oh, S. Lee, H. N. Fitriana, M. Moon, M.-S. Kim, J. Lee, K. Min, G. W. Park and J.-P. Lee, Bioresour. Technol., 2021, 320, 124350 CrossRef CAS PubMed.
- G. Mohanakrishna, I. M. A. Reesh, K. Vanbroekhoven and D. Pant, Sci. Total Environ., 2020, 715, 137003 CrossRef CAS PubMed.
- K. Hernández-García, B. Cercado, E. Rivero and F. Rivera, Fuel, 2020, 279, 118463 CrossRef.
- P. He, W. Han, L. Shao and F. Lü, Biotechnol. Biofuels, 2018, 11, 1–13 CrossRef PubMed.
- C. Xia, P. Zhu, Q. Jiang, Y. Pan, W. Liang, E. Stavitski, H. N. Alshareef and H. Wang, Nat. Energy, 2019, 4, 776–785 CrossRef CAS.
- J. J. Kaczur, H. Yang, Z. Liu, S. D. Sajjad and R. I. Masel, Front. Chem., 2018, 6, 263 CrossRef PubMed.
- V. Sivalingam and C. Dinamarca, Chem. Eng. Trans., 2021, 86, 1483–1488 Search PubMed.
- G. Swain, K. L. Maurya, R. K. Sonwani, R. S. Singh, R. P. Jaiswal and B. Rai, Bioresour. Technol., 2022, 351, 126921 CrossRef CAS PubMed.
- M. U. Monir, A. Abd Aziz, F. Khatun and A. Yousuf, Renewable Energy, 2020, 157, 1116–1123 CrossRef CAS.
- S. Sauerschell, S. Bajohr and T. Kolb, Energy Fuels, 2022, 36, 7166–7176 CrossRef CAS.
- S. Zhang, J. Jiang, H. Wang, F. Li, T. Hua and W. Wang, J. CO2 Util., 2021, 51, 101640 CrossRef CAS.
- S. Tian, Y.-J. Jiang, Y. Cao, J.-R. Zhang, Y. Zhou and Y. Wang, Chem. – Eur. J., 2022, e202202317, DOI:10.1002/chem.202202317.
- Q. Fu, Y. He, Z. Li, J. Li, L. Zhang, X. Zhu and Q. Liao, Energy Convers. Manage., 2022, 268, 116018 CrossRef CAS.
- B. S. Zakaria and B. R. Dhar, Bioresour. Technol., 2019, 289, 121738 CrossRef CAS PubMed.
- B. Mohapatra and P. S. Phale, Curr. Pollution Rep., 2022 DOI:10.1007/s40726-022-00231-w.
- A. B. Tahar, A. Romdhane, N. Lalaoui, N. Reverdy-Bruas, A. Le Goff, M. Holzinger, S. Cosnier, D. Chaussy and N. Belgacem, J. Power Sources, 2018, 408, 1–6 CrossRef.
- J. R. C. Dizon, A. H. Espera Jr, Q. Chen and R. C. Advincula, Addit. Manuf., 2018, 20, 44–67 CAS.
- A. Ambrosi and M. Pumera, Chem. Soc. Rev., 2018, 47, 7213–7224 RSC.
- M. C. Freyman, T. Kou, S. Wang and Y. Li, Nano Res., 2020, 13, 1318–1323 CrossRef CAS.
- D. Wangpraseurt, S. You, Y. Sun and S. Chen, Trends Biotechnol., 2022, 40, 843–857 CrossRef CAS PubMed.
- J. S. Sravan, A. Tharak, J. A. Modestra, I. S. Chang and S. V. Mohan, Bioresour. Technol., 2021, 326, 124676 CrossRef PubMed.
- F. Qian, C. Zhu, J. M. Knipe, S. Ruelas, J. K. Stolaroff, J. R. DeOtte, E. B. Duoss, C. M. Spadaccini, C. A. Henard and M. T. Guarnieri, Nano Lett., 2019, 19, 5829–5835 CrossRef CAS PubMed.
- N. Baig, I. Kammakakam and W. Falath, Mater. Adv., 2021, 2, 1821–1871 RSC.
- T. Kou, Y. Yang, B. Yao and Y. Li, Small Methods, 2018, 2, 1800152 CrossRef.
- X. Li, S. Wang, L. Li, X. Zu, Y. Sun and Y. Xie, Acc. Chem. Res., 2020, 53, 2964–2974 CrossRef CAS PubMed.
- N. J. Claassens, C. A. Cotton, D. Kopljar and A. Bar-Even, Nat. Catal., 2019, 2, 437–447 CrossRef CAS.
- X. Zhu, J. Jack, A. Leininger, M. Yang, Y. Bian, J. Lo, W. Xiong, N. Tsesmetzis and Z. J. Ren, Resour., Conserv. Recycl., 2022, 184, 106395 CrossRef CAS.
- R. D. Rodriguez, A. Khalelov, P. S. Postnikov, A. Lipovka, E. Dorozhko, I. Amin, G. V. Murastov, J.-J. Chen, W. Sheng and M. E. Trusova, Mater. Horiz., 2020, 7, 1030–1041 RSC.
- S. Dutta, R. Patil and T. Dey, Nano Energy, 2022, 96, 107074 CrossRef CAS.
- A. Kumari, R. Rajeev, L. Benny, Y. Sudhakar, A. Varghese and G. Hegde, Adv. Colloid Interface Sci., 2021, 297, 102542 CrossRef CAS PubMed.
- B. Bian, M. F. Alqahtani, K. P. Katuri, D. Liu, S. Bajracharya, Z. Lai, K. Rabaey and P. E. Saikaly, J. Mater. Chem. A, 2018, 6, 17201–17211 RSC.
- X. Zhu, J. Jack, Y. Bian, X. Chen, N. Tsesmetzis and Z. J. Ren, ACS Sustainable Chem. Eng., 2021, 9, 6012–6022 CrossRef CAS.
- F. Ameen, W. A. Alshehri and S. A. Nadhari, ACS Sustainable Chem. Eng., 2019, 8, 311–318 CrossRef.
- R. Wang, H. Li, J. Sun, L. Zhang, J. Jiao, Q. Wang and S. Liu, Adv. Mater., 2021, 33, 2004051 CrossRef CAS PubMed.
- B. Wu, R. Lin, X. Kang, C. Deng, A. D. Dobson and J. D. Murphy, Renewable Sustainable Energy Rev., 2021, 152, 111690 CrossRef CAS.
- Y. He, Q. Li, J. Li, L. Zhang, Q. Fu, X. Zhu and Q. Liao, Renewable Energy, 2022, 185, 862–870 CrossRef CAS.
- Q. Li, Q. Fu, H. Kobayashi, Y. He, Z. Li, J. Li, Q. Liao and X. Zhu, Sustainable Energy Fuels, 2020, 4, 2987–2997 RSC.
- N. Aryal, L. Wan, M. H. Overgaard, A. C. Stoot, Y. Chen, P.-L. Tremblay and T. Zhang, Bioelectrochemistry, 2019, 128, 83–93 CrossRef CAS PubMed.
- D. Thatikayala and B. Min, Sci. Total Environ., 2021, 768, 144477 CrossRef CAS PubMed.
- S. Shakeel, A. H. Anwer and M. Z. Khan, J. Cleaner Prod., 2020, 269, 122391 CrossRef CAS.
- G. Baek, L. Shi, R. Rossi and B. E. Logan, Chem. Eng. J., 2022, 435, 135076 CrossRef CAS.
- N. Eghtesadi, K. Olaifa, F. M. Perna, V. Capriati, M. Trotta, O. Ajunwa and E. Marsili, Bioelectrochemistry, 2022, 147, 108207 CrossRef CAS PubMed.
- K. P. Katuri, S. Kamireddy, P. Kavanagh, A. Muhammad, P. Ó. Conghaile, A. Kumar, P. E. Saikaly and D. Leech, Water Res., 2020, 185, 116284 CrossRef CAS PubMed.
- V. Flexer and L. Jourdin, Acc. Chem. Res., 2020, 53, 311–321 CrossRef CAS PubMed.
- T. O. Ajiboye, A. T. Kuvarega and D. C. Onwudiwe, Nano-Struct. Nano-Objects, 2020, 24, 100577 CrossRef CAS.
- G. Zhen, X. Lu, T. Kobayashi, G. Kumar and K. Xu, Chem. Eng. J., 2016, 284, 1146–1155 CrossRef CAS.
- Z. Cai, L. Huang, X. Quan, Z. Zhao, Y. Shi and G. L. Puma, Appl. Catal., B, 2020, 267, 118611 CrossRef CAS.
- W. Kong, L. Huang, X. Quan, Z. Zhao and G. L. Puma, Appl. Catal., B, 2021, 284, 119696 CrossRef CAS.
- T.-s Song, T. Li, R. Tao, H. F. Huang and J. Xie, Sci. Total Environ., 2022, 818, 151820 CrossRef CAS PubMed.
- P. Gupta and N. Verma, Chem. Eng. J., 2022, 446, 137029 CrossRef CAS.
- Z. Dong, H. Wang, S. Tian, Y. Yang, H. Yuan, Q. Huang, T.-S. Song and J. Xie, Bioresour. Technol., 2018, 269, 203–209 CrossRef CAS PubMed.
- Y. Jiang, Q. Liang, N. Chu, W. Hao, L. Zhang, G. Zhan, D. Li and R. J. Zeng, Sci. Total Environ., 2020, 741, 140198 CrossRef CAS PubMed.
- L. Yang, Y. Chen, Q. Wen, H. Xu, X. Pan and X. Li, Electrochim. Acta, 2022, 428, 140959 CrossRef CAS.
- S. Tian, X. Yao, T.-S. Song, Z. Chu, J. Xie and W. Jin, ACS Sustainable Chem. Eng., 2020, 8, 6777–6785 CrossRef CAS.
- C. Yang, H. Aslan, P. Zhang, S. Zhu, Y. Xiao, L. Chen, N. Khan, T. Boesen, Y. Wang and Y. Liu, Nat. Commun., 2020, 11, 1379 CrossRef CAS PubMed.
- J. Cheng, R. Xia, H. Li, Z. Chen, X. Zhou, X. Ren, H. Dong, R. Lin and J. Zhou, ACS Sustainable Chem. Eng., 2022, 10, 3935–3950 CrossRef CAS.
- Z. Zhang, X. Lu, C. Niu, T. Cai, N. Wang, Y. Han, R. Zhang, Y. Song and G. Zhen, Fuel, 2022, 310, 122450 CrossRef CAS.
- M. Robertson, A. Güillen Obando, J. Emery and Z. Qiang, ACS omega, 2022, 7, 12278–12287 CrossRef CAS PubMed.
- A. Schievano, D. Pant and S. Puig, Front. Energy Res., 2019, 7, 110 CrossRef.
- C. Liu, X. Yuan, Y. Gu, H. Chen, D. Sun, P. Li, M. Li, Y. Dang, J. A. Smith and D. E. Holmes, ACS Sustainable Chem. Eng., 2020, 8, 11368–11375 CrossRef CAS.
- J. L. Hart, K. Hantanasirisakul, A. C. Lang, B. Anasori, D. Pinto, Y. Pivak, J. T. van Omme, S. J. May, Y. Gogotsi and M. L. Taheri, Nat. Commun., 2019, 10, 522 CrossRef CAS PubMed.
- G. P. Lim, C. F. Soon, N. L. Ma, M. Morsin, N. Nayan, M. K. Ahmad and K. S. Tee, Environ. Res., 2021, 201, 111592 CrossRef CAS PubMed.
- B. Scheibe, J. K. Wychowaniec, M. Scheibe, B. Peplinska, M. Jarek, G. Nowaczyk and Ł. Przysiecka, ACS Biomater. Sci. Eng., 2019, 5, 6557–6569 CrossRef CAS PubMed.
- C. Y. J. Lim, A. D. Handoko and Z. W. Seh, Diamond Relat. Mater., 2022, 130, 109461 CrossRef CAS.
- Y. Cui, Z. Cao, Y. Zhang, H. Chen, J. Gu, Z. Du, Y. Shi, B. Li and S. Yang, Small Sci., 2021, 1, 2100017 CrossRef CAS.
- R. Zhou, R. Zhou, X. Zhang, Z. Fang, X. Wang, R. Speight, H. Wang, W. Doherty, P. J. Cullen and K. Ostrikov, ChemSusChem, 2019, 12, 4976–4985 CrossRef CAS PubMed.
- X. Sun, H. K. Atiyeh, M. Li and Y. Chen, Bioresour. Technol., 2020, 295, 122252 CrossRef CAS PubMed.
- B. Wu, R. Lin, X. Ning, X. Kang, C. Deng, A. D. Dobson and J. D. Murphy, Bioresour. Technol., 2022, 358, 127294 CrossRef CAS PubMed.
- C. Yin, Y. Shen, R. Yuan, N. Zhu, H. Yuan and Z. Lou, Bioresour. Technol., 2019, 284, 315–324 CrossRef CAS PubMed.
- H. Zhang, Q. Tan, Q. Huang, K. Wang, X. Tu, X. Zhao, C. Wu, J. Yan and X. Li, ACS Sustainable Chem. Eng., 2022, 10, 7712–7725 CrossRef CAS.
- L. Jourdin and T. Burdyny, Trends Biotechnol., 2021, 39, 359–369 CrossRef CAS PubMed.
- J. Jack, H. Fu, A. Leininger, T. K. Hyster and Z. J. Ren, ACS Sustainable Chem. Eng., 2022, 10, 4114–4121 CrossRef CAS.
- Z. Zhang, H. Wang, Y. Nie, X. Zhang and X. Ji, Front. Chem., 2022, 10, 894106 CrossRef CAS PubMed.
- B. Song, A. Qin and B. Z. Tang, Cell Rep. Phys. Sci., 2022, 3, 100719 CrossRef CAS.
- S. Ikeda, Y. Takamatsu, M. Tsuchiya, K. Suga, Y. Tanaka, A. Kouzuma and K. Watanabe, Essays Biochem., 2021, 65, 355–364 CrossRef CAS PubMed.
- F. Kracke, A. B. Wong, K. Maegaard, J. S. Deutzmann, M. A. Hubert, C. Hahn, T. F. Jaramillo and A. M. Spormann, Commun. Chem., 2019, 2, 45 CrossRef.
- F. M. Schwarz, F. Oswald and V. Müller, ACS Sustainable Chem. Eng., 2021, 9, 6810–6820 CrossRef CAS.
- A. Rückel, J. Hannemann, C. Maierhofer, A. Fuchs and D. Weuster-Botz, Front. Microbiol., 2021, 12, 655390 CrossRef PubMed.
- H.-Y. Yang, N.-N. Hou, Y.-X. Wang, J. Liu, C.-S. He, Y.-R. Wang, W.-H. Li and Y. Mu, Sci. Total Environ., 2021, 790, 148128 CrossRef CAS PubMed.
- K. Wang, T.-Q. Shi, L. Lin, P. Wei, R. Ledesma-Amaro, X.-J. Ji and H. Huang, Biotechnol. Adv., 2022, 59, 107984 CrossRef CAS PubMed.
- M. V. Reddy, A. ElMekawy and D. Pant, Biofuels, Bioprod. Biorefin., 2018, 12, 966–977 CrossRef CAS.
- Y. Jiang, N. Chu, D.-K. Qian and R. J. Zeng, Bioresour. Technol., 2020, 295, 122266 CrossRef CAS PubMed.
- C. M. Martinez and L. H. Alvarez, Biotechnol. Adv., 2018, 36, 1412–1423 CrossRef CAS PubMed.
- F. Di Capua, F. Pirozzi, P. N. Lens and G. Esposito, Chem. Eng. J., 2019, 362, 922–937 CrossRef CAS.
- L. Rovira-Alsina, M. Romans-Casas, M. D. Balaguer and S. Puig, Bioresour. Technol., 2022, 354, 127181 CrossRef CAS PubMed.
- G. Zhen, S. Zheng, X. Lu, X. Zhu, J. Mei, T. Kobayashi, K. Xu, Y.-Y. Li and Y. Zhao, Bioresour. Technol., 2018, 266, 382–388 CrossRef CAS PubMed.
- M. Kazmi, M. Irfan, L. Zhou, S. Yuan, H. Fatima, L.-Y. Tian, Y.-L. Ye, Q.-S. Lu, X.-Y. Lu and S.-Z. Yang, Renewable Sustainable Energy Rev., 2022, 156, 111997 CrossRef CAS.
- A. Abbas, Y. M. Yi, F. Saleem, Z. Jin, A. Veksha, Q. Yan, G. Lisak and T. M. Lim, Int. J. Energy Res., 2021, 45, 19611–19622 CrossRef CAS.
- L. Laguillaumie, Y. Rafrafi, E. Moya-Leclair, D. Delagnes, S. Dubos, M. Spérandio, E. Paul and C. Dumas, Bioresour. Technol., 2022, 354, 127180 CrossRef CAS PubMed.
- M. Abdollahi, S. Al Sbei, M. A. Rosenbaum and F. Harnisch, Front. Microbiol., 2022, 13, 947550 CrossRef PubMed.
- M. D. P. A. Rojas, M. Zaiat, E. R. González, H. De Wever and D. Pant, Process Biochem., 2021, 101, 50–58 CrossRef CAS.
- R. Pärnamäe, S. Mareev, V. Nikonenko, S. Melnikov, N. Sheldeshov, V. Zabolotskii, H. Hamelers and M. Tedesco, J. Membr. Sci., 2021, 617, 118538 CrossRef.
- W. Lai, Y. Qiao, J. Zhang, Z. Lin and H. Huang, Energy Environ. Sci., 2022, 15, 3603–3629 RSC.
- C. McCallum, C. M. Gabardo, C. P. O’Brien, J. P. Edwards, J. Wicks, Y. Xu, E. H. Sargent and D. Sinton, Cell Rep. Phys. Sci., 2021, 2, 100522 CrossRef CAS.
- J. Zhang, W. Luo and A. Züttel, J. Catal., 2020, 385, 140–145 CrossRef CAS.
- C. G. Okoye-Chine, K. Otun, N. Shiba, C. Rashama, S. N. Ugwu, H. Onyeaka and C. T. Okeke, J. CO2 Util., 2022, 62, 102099 CrossRef CAS.
- N. K. Rathinam, M. Bibra, M. Rajan, D. Salem and R. K. Sani, Bioresour. Technol., 2019, 278, 477–480 CrossRef CAS PubMed.
- A. Patange, C. O’Byrne, D. Boehm, P. Cullen, K. Keener and P. Bourke, Front. Microbiol., 2019, 10, 2841 CrossRef PubMed.
|
This journal is © The Royal Society of Chemistry 2023 |
Click here to see how this site uses Cookies. View our privacy policy here.