DOI:
10.1039/D1TB02757C
(Review Article)
J. Mater. Chem. B, 2022,
10, 3587-3600
Recent advances in the design of antimicrobial peptide conjugates
Received
15th December 2021
, Accepted 22nd February 2022
First published on 22nd February 2022
Abstract
Antimicrobial peptides (AMPs) are ubiquitous host defense peptides characterized by their antibiotic activity and lower propensity for developing resistance compared to classic antibiotics. While several AMPs have shown activity against antibiotic-sensitive and even multi-drug resistant strains, some bottlenecks to further development and clinical applications are still present, for instance, low antimicrobial activity, instability under physiological conditions, systemic toxicity and the potential for compromising the innate host defense immunity. Conjugation to molecules such as proteins, synthetic polymers, small molecules and nanoparticles are strategies under investigation to boost the therapeutic efficacy of AMPs. This review focuses on the design and application of AMPs’ conjugates. In silico tools for creating new AMPs and AMPs’ conjugates and their clinical development are also discussed. Furthermore, key future considerations regarding the major achievements and challenges of AMPs’ conjugates in the antimicrobial resistance context are presented as a take-home message.
Introduction
Antimicrobial resistance refers to the ability of microorganisms to develop mechanisms to bypass the action of drugs used against them. Infections caused by resistant microorganisms cause a significant increase in the length of stay, morbidity and mortality in hospitals, in addition to limited treatment and the possibility of recurrence.1 More specifically, more than 700
000 deaths are globally estimated to be caused by infections from resistant bacteria.2
With the rapid increase in antimicrobial resistance due to excessive and improper use of antibiotics, alternatives are being sought to overcome this public health problem. According to Mahlapuu et al. (2016), we are moving towards a post-antibiotic era in which current therapeutic strategies might no longer work, generating an urgency for novel categories of therapeutic agents. Several studies point to antimicrobial peptides (AMPs) as promising alternatives3 as they might present rapid bactericidal action,4 low resistance incidence,3 antimicrobial activity in the micromolar range5 and multifunctional mechanism of action.6
AMPs are constituents of the innate immune response and are ubiquitous in nature, in addition to having wide structural and functional diversity.7 Commonly, AMPs are small molecules with less than 50 amino acids.8 Although the peptide structure is diverse in length and primary structure, most AMPs are naturally amphipathic and positively charged. Consequently, the mode of action of AMPs involves permeabilization of the microbial membrane, compromising its integrity and leading to cell lysis. More complex mechanisms of action are also of importance, such as the formation of nanonets and metabolic and translational inhibition.9–11 In addition, AMPs are selective to bacteria, as the combination of their hydrophobic and cationic components interacts more effectively with highly anionic bacterial membranes, and less with more neutral membranes like those of mammalian cells.12 However, challenges regarding the clinical use of AMPs are still present, such as degradation and rapid elimination, which make their clinical use difficult. In this sense, conjugation to molecules such as polymers, small molecules and nanoparticles may increase the therapeutic potential of AMPs, bringing several benefits to the molecules.13
Some recent papers review the important aspects of AMPs’ conjugation to polymers, such as the chemistry employed.14,15 Nonetheless, each day new data are generated and, also, conjugation to other types of molecules is of interest. A compilation of such data is of great help to those investigating the conjugation of AMPs. In this review, the conjugation of AMPs to different types of molecules, such as polymers, lipids, and antibiotics, is presented and discussed, highlighting their positive and negative aspects. AMP nanoparticle systems are also reviewed. In addition, we discuss in silico tools to help the development and understanding of new AMPs and AMP conjugates.
Classification
Usually AMPs are relatively short (10 to 50 amino acids), with an overall positive charge ranging from +2 to +11 and with significant hydrophobicity (usually 50% of the residues).16,17 Based on their secondary structure, AMPs are divided into three groups: α-helical, β-sheet and extended peptides (Fig. 1), with the majority of AMPs belonging to the first two categories (Table 1).18,19 The largest group, the α-helical, corresponds to structures with up to 40 amino acid residues and a positive charge at physiological pH, resulting in an amphipathic character.20 In non-polar environments, such as bacterial membranes, they form the α-helical structure.21 Magainin is one of the most common representatives of α-helical peptides. The β-sheet AMPs present two or more disulfide bridges that stabilize the conformation and result in amphipathic molecules.16 Owing to rigidity, they do not undergo conformational changes after interaction with membranes, as α-helical peptides do.16 Finally, extended AMPs usually form irregular secondary structures and are characterized by the presence of arginine and proline, as well as histidine and/or tryptophan residues.19,22
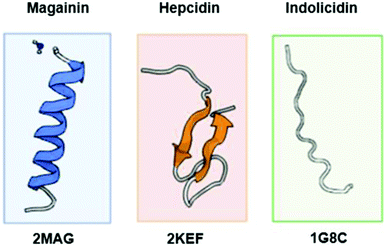 |
| Fig. 1 Examples of different categories of antimicrobial peptides based on the secondary structures: α-helical – magainin, β-sheet – hepcidin and extended AMP – indolicidin, along with their Protein Data Bank identification (PDB ID) codes: 2MAG, 2KEF and 1G8C. | |
Table 1 Classes of antimicrobial peptides based on secondary structures
Category |
Peptide |
Unique structural/sequence feature |
Organism |
PDB ID |
α-Helical peptide |
Piscidin |
— |
Mast cells of striped bass |
2JOS
|
LAH4 |
Histidine rich |
Synthetic construct |
2KJN
|
Acanthaporin |
Five disulfide bonds |
Acanthamoeba culbertsoni
|
2LRD
|
LL-37 |
Amidated C-terminus |
Homo sapiens
|
2K6O
|
Magainins |
— |
Xenopus laevis
|
2MAG
|
Cecropin |
Amidated C-terminus |
Papilio xuthus
|
2LA2
|
Aurein 1–2 |
Amidated C-terminus |
Australian frog
|
1VM5
|
Maculatins |
Amidated C-terminus |
Ranoidea genimaculatus
|
2MMJ
|
|
β-sheet peptide |
Defensins |
|
|
|
– α defensins |
Three disulfide bonds |
Homo sapiens
|
2PM4
|
– β defensins |
Three disulfide bonds |
Homo sapiens
|
1E4Q
|
– θ defensins |
Three disulfide bonds and cyclic |
Homo sapiens
|
2LYE
|
Gomesin |
Cysteine rich and two disulfide bonds |
Acanthoscurria gomesiana
|
1KFP
|
Protegrins |
Two disulfide bonds |
Sus scrofa
|
2NC7
|
Hepcidin |
Four disulfide bonds |
Homo sapiens
|
2KEF
|
LEAP-2 |
Two disulfide bonds |
Homo sapiens
|
2L1Q
|
Capitellacin |
Amidated C-terminus |
Capitella teleta
|
7ALD
|
|
Extended |
Tachyplesin-1 |
Arginine rich |
Tachypleus tridentatus
|
1MA4
|
VG16KRKP |
Lysine rich |
Dengue virus |
2MWL
|
Tritrpticin |
Tryptophan and arginine rich |
Neutrophil granules |
1D6X
|
Indolicidin |
Rich in tryptophan and proline |
Bovine |
1G89
|
Mechanism of action
The interaction of AMPs with membranes is extremely important for antimicrobial activity, both to target the membrane itself, disturbing the physical integrity of the cell, as well as to reach intracellular targets.23–25 AMPs of cationic nature are attracted towards the negative charge of the bacterial membrane composed of lipopolysaccharides and lipoteichoic acid.26,27 On the other hand, mammalian cell membranes consist mainly of zwitterionic phospholipids and, therefore, have an approximately neutral net charge. They also present cholesterol which results in better packing and stabilizes the phospholipid bilayer, reducing the activity of AMPs.16,18,28
Following the electrostatic interaction with the microbial membrane, AMPs adopt a well-defined secondary structure essential for microbial membrane permeabilization, leading to its rupture.29,30 Several models are proposed for membrane permeabilization, in particular the barrel-stave model, the toroidal-pore model and the carpet-like model (Fig. 2).
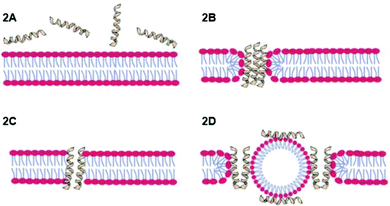 |
| Fig. 2 Mechanisms of action of antimicrobial peptides on bacterial membranes: (A) initial interaction of PAMs; (B) barrel-stave model; (C) toroidal pore model; and (D) carpet-like model. Based on Raheem & Straus (2019).34 | |
According to the barrel-stave model, AMPs are inserted perpendicularly into the bilayer, and line up laterally like strips in a barrel, in which the hydrophobic regions of the peptide interact with the membrane and the hydrophilic regions face inwards pores, forming aqueous transmembrane pores/channels.31 The toroidal-pore model states that AMPs lead to an induction of the positive curvature of the membrane, generating unfavorable deformation energy.32 The peptides are inserted perpendicularly in the membrane, and hydrophobic regions bind to the hydrophobic portions, while hydrophilic regions interact with the headgroups of the phospholipids, resulting in curvature and pore formation.31 Finally, according to the carpet-like model peptides display a detergent-like activity, which causes the formation of micelles and pores in the membrane.31 More specifically, the peptides cover the surface like a carpet and, at a critical concentration, destabilizes the membrane, leading to the formation of mixed micelles (peptide + lipid).33
Antimicrobial peptide conjugates
AMPs have been gaining attention as drug leads and/or drug candidates, especially to treat resistant bacterial infections.35 Nonetheless, some challenges still prevent the clinical use of AMPs, such as (i) degradation by serum and gastrointestinal proteases; (ii) low oral absorption and solubility; (iii) toxicity and immunogenicity; (iv) rapid elimination by renal filtration; (v) low target-specificity and (vi) high production cost.36,37 For the synthetic production of 1 g of peptide, costs can vary from 100 to 600 dollars.38 Toxicity studies have also shown that AMPs could bind directly to mammalian cells, causing undesirable effects. In addition, broad-spectrum AMPs can lead to the elimination of natural microflora.39
Conjugation is a strategy to tackle these drawbacks and the choice of appropriate conjugation must be based on the characteristics of each AMP. The rational design of conjugation needs to overcome the deficiencies in the use of AMPs, in addition to controlling the response of the host to the AMPs, maintaining antibiotic activity in the appropriate range and activity against the chosen microorganism.35 Several papers are found in the literature referring to the development of modified peptides, including hybrid AMPs, immobilized AMPs, conjugated AMPs and nanostructured AMPs (Table 2). All these alternatives will be discussed in this review.35
Table 2 Examples of molecules conjugated to AMPs and its applications
AMP/sequence |
Conjugation molecule(s) |
Treatment/application |
Ref. |
Magainin 2 (GIGKFLHSAKKFGKAFVGEIMNS) |
Vancomycin |
Antimicrobial |
60
|
Dhvar-5 (LLLFLLKKRKKRKY) |
Chitosan |
Antimicrobial |
61
|
CysHHC10 (HCKRWWKWIRW-NH2) |
P(EEP-co-PEP) |
Antimicrobial |
62
|
KR12-NH2 (KRIVQRIKDFLR-NH2) |
n-Alkyl fatty acids (C4–C14) and aromatic acids (benzoic and trans-cinnamic) |
Antimicrobial |
63
|
ILPWRWPWWPWRR-NH2 |
Pluronic F-127 |
Antimicrobial |
64
|
GH12 (variants) |
Methacrylate |
Antimicrobial |
65
|
CysHHC10 |
Chitosan |
Antimicrobial |
66
|
KRFRIRVRV/RWRWRWRW |
Poly(glycolic acid-co-caprolactone) |
Sutures on in vitro wound healing |
67
|
Tet213 (KRWWKWWRRC) |
Alginate/hyaluronic acid/collagen |
Wound dressings for wound healing |
68
|
RWAAC-NH2/CAAWR-NH2/PWKISIHLAAC-NH2 |
Chitosan |
Antimicrobial |
69
|
Daptomycin |
NHS–PEG3400–DSPE |
Antimicrobial |
70
|
Vancomycin |
Transportan 10 |
Antimicrobial |
71
|
Polymyxin |
Porphyrin |
Photobactericidal |
72
|
Aurein 2.2Δ3-cys (GLFDIVKKVVGALC-CONH2) and variants |
Hyperbranched polyglycerol (HPG) |
Antimicrobial/resistance to proteolysis |
73
|
Poly(Lys)x(Ala)y |
6-Arm PEG |
Wound healing |
74
|
LL-37 and indolicidin |
Carbon nanotubes |
Antimicrobial |
75
|
Indolicidin (ILPWKWPWWPWRR) |
Levofloxacin |
Antimicrobial |
76
|
KG18 and VR18 |
Tungsten disulfide (WS2) quantum dots |
Antimicrobial/bioimaging |
77
|
CKRWWKWIRW-NH2 |
PEG-based hydrogels |
Antimicrobial |
78
|
K4 (NH2-KKKKPLFGLFFGLF-COOH) |
Poly(lactic-co-glycolic acid) (PLGA) |
Wound healing |
79
|
Conjugation of AMPs to proteins and peptides
AMPs can be conjugated to other peptides such as cationic cell-penetrating peptides (CPPs). Typically composed of 5 to 30 amino acids, CPPs are able to cross tissues and cell membranes, allowing better systemic distribution and, therefore, antimicrobial activity.40,41 In addition, CPPs can help target anti-cancer drugs in tumor tissues, increasing their effectiveness and reducing the side effects of chemotherapy.42 In a recent study, Lee et al. (2019) conjugated the CPP R9 to the AMP magainin and to the AMP M15; the conjugation resulted in a 2- to 4-fold increase in antimicrobial activity against Gram-positive bacteria and a 4- to 16-fold increase in antimicrobial activity against Gram-negative bacteria.43 Also, to increase the antibiotic activity against Salmonella typhimurium, the marine AMP N2 was conjugated to CPPs bLFcin6 and Tat11 and MICs of 91.4% and 98.9% were reached, respectively, while the MIC of pure N2 was 69.7%.44
Eksteen and colleagues (2020) explored the conjugation of short AMPs to a histidine-rich vector (GHHPH) via the sterically hindered disulfide linker system and found that the AMPs can be converted to anticancer compounds. Accordingly, the positive charge resulting from the HIS vector results in electrostatic interactions between the AMPs and glycosaminoglycans often overexpressed on cancer cell membranes.45 Other forms of carcinogenic cell selectivity are based on the conjugation of an AMP to a low pH Insertion Peptide (pHLIP), in which reachable targeting is based on the acidic microenvironment of the tumor.46 Burns et al. (2016) showed that derivatives of cationic AMP (KLAKLAK)2 conjugated to the C-terminal of pHLIP led to selectivity to breast cancer cells based on the pH.47 Another alternative makes use of the increased levels of matrix metalloproteinases (MMPs) in many types of cancer. Buforin llb is an antitumor CPP/AMP that has been fused to the anionic peptide MMIS (modified magainin intervening sequence) so that the positive charge of AMP is neutralized and rendered inactive through a cleavable MMP ligand; the MMIS:buforin llb conjugate was completely inactive in cells that do not produce MMPs. However, when administered to cancer cells it regained anticancer activity, showing a significant improvement compared to buforin llb alone.48
In more recent studies, AMPs have been conjugated to antibodies for more effective targeting of Gram-negative bacteria. Touti et al. (2018), for example, developed antibody–bactericidal macrocyclic peptide conjugates (ABCs) with low hemolytic activity, preserved bioactivity (in the nanomolar range), and preferential activity against Escherichia coli.49
Conjugation of AMPs to polymers
An interesting strategy to improve the AMP activity and stability is their conjugation to polymers with tailored characteristics for specific applications. The polymer design must take into account the (i) availability of suitable functional groups, (ii) biocompatibility, (iii) biodegradability, and (iv) molecular weight of the conjugate under the renal excretion limit.13,50 A balance between amphiphilicity and hydrophobicity must be established to select the adequate AMP and polymer structures for conjugation. Higher hydrophobicity enhances susceptibility to α-helical folding, resulting in higher antimicrobial and hemolytic activities. In contrast, a balanced amphiphilicity usually improves antimicrobial activity and selectivity.51,52
AMP–polymer conjugates can be synthesized mainly by two methods: grafting to and grafting from.53,54Grafting to is the most common modification and is based on the attachment of a reactive group of the peptide to the pre-formed polymer chain. The major advantage of this technique is that the polymer is synthesized independently of the protein. Yet, the major disadvantages are the low conjugation yields and the difficulty in separating the polymer–peptide from the remaining free polymer.54Grafting from is defined as the modification of a protein or peptide with a small molecule from which the polymerization can start. Some advantages of this method are the ease of purification and a high conjugation yield. However, this method may result in polymers affecting the activity or folding of the conjugate.54
Regarding the polymerization technique itself, priorities are controlling the molecular weight of the polymer, i.e. low polydispersity, and, most importantly, the improvement – or at least the maintenance – of the AMP biological activity. Controlled radical polymerizations (CRPs), especially atom transfer polymerization (ATRP) and radical addition–fragmentation chain transfer (RAFT), are useful alternatives to promote the construction of well-defined polymer architectures with low polydispersity;53,55 ring-opening polymerization allows features like biodegradability to be controlled; anionic polymerization to obtain poly(ethylene glycol) (PEG) is widely used for enhancing stability in aqueous environments.56,57
PEGylation
One of the most common polymers used for conjugation to AMPs is PEG.58 This strategy, known as PEGylation, extends half-life, enhances water solubility, lowers renal clearance, prevents degradation by proteolytic enzymes and lower immunogenicity by shielding antigenic epitopes, overall improving pharmacokinetics. A molecule of PEG can be designed to be conjugated to a specific functional group, and thus the coupling chemistry differs according to the functional group.59 The most common modification takes place in amine groups (N-terminus or lysine), involving mainly N-hydroxysuccinimide (NHS) esters (Fig. 3). NHS groups can bind to available amines with high linkage efficiency.14
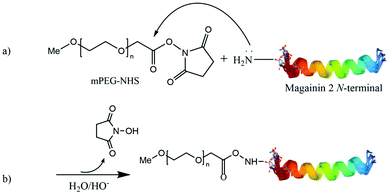 |
| Fig. 3 AMP site specific N-terminal PEGylation reaction using N-hydroxysuccinimidyl ester PEG (mPEG-NHS). (a) Nucleophilic attack by the nucleophile of magainin 2. (b) Conjugation of magainin 2 to mPEG-NHS. | |
Despite the several advantages of PEGylation, purification is still a challenge owing to the excess of unreacted PEG molecules and the similar molecular weight of the reactive PEG and PEGylated AMPs.80 The excess of reactive PEG in the reaction media results from premature hydrolysis before linking to the peptide/protein.81 Another concern refers to random PEGylation at different sites in the AMP and, therefore, polydispersity. To circumvent this drawback, PEGylation is widely implemented attaching a molecule of PEG at site-directed regions such as thiol groups and the N-terminus, for example.
Pelegri-O’Day et al. (2012) showed that the AMP CaLL PEGylated at the N-terminal portion demonstrated increased biocompatibility and preserved membrane binding sites; nonetheless, the antimicrobial activity was found to be 50% of the original activity. In a more recent study, PEGylation was applied to improve the half-life of an arginine-rich AMP by temporarily masking the arginine residues with methoxy poly(ethylene glycol) (mPEG) using phenylglyoxal units.82 The strategy involved the gradual release of AMPs and this method is known as releasable-PEGylation (rPEGylation). The outcome of this study showed that the conjugation was effective to prolong the half-life and shield the AMP from protease degradation. PEGylation can also be adopted for nanogel delivery systems. Nordström (2019) demonstrated the conjugation of 48 ethylene glycol units either at the N- or C-terminal of the AMP KYE28 (and variants). After attachment, the AMPs were bound to poly(ethyl acrylate-co-methacrylic acid) microgels. As a result, KYE28 (and PEGylated variants) was observed to be ordered inside the microgel core and the AMPs formed hydrophobic domains upon microgel loading. However, PEGylation did not play a major role in the release of the AMPs.83
Dennison and collaborators (2021) studied the C-terminal PEGylation of host defense peptides (aurein 2.1, aurein 2.6, and aurein 3.1) and their potential to kill bacteria.84 The PEGylated peptides presented lower bactericidal activity (the minimum lethal concentration was approximately one and a half to threefold lower, but still in the micromolar range), but reduced hemolytic activity (from 10% to 3%) and an enhanced relative therapeutic index (RTI), at least 3–6-fold higher than those of the native aureins. The authors defined the RTI as the ratio of the antibacterial activity to the hemolytic activity.
To promote higher therapeutic indices and active AMPs against multi-drug resistant bacteria, Brito and colleagues (2021) PEGylated LyeTxI-b, a synthetic peptide derived from native LyeTx I, originally isolated from Lycosa erythrognatha spider venom, at the cysteine-21 site by Michael addition. The authors used carbapenem-resistant A. baumannii (CRAB) to verify the ability of the PEGylated peptide in reducing resistance. PEGylated LyeTx I-b presented MIC values similar to the non-PEGylated peptide, showed anti-biofilm activity and a synergistic effect with commercial antimicrobial agents, and did not induce resistance.85
The covalent attachment of an AMP to a polymer can be based on PEGylation, ring opening polymerization (ROP), RAFT, and other techniques. But, most importantly, AMPs and polymers to be associated must have functional groups available to react with each other. End-functionalized groups in the polymer are crucial to determine the coupling method applied. Thus, the coupling chemistry can be based on reactions of amidation, urethane bond formation, Michael addition, copper(I)-catalyzed alkyne–azide cycloaddition (CuAAC), thioester linkage, disulfide bond formation and nucleophilic addition.14,54 On the other hand, noncovalent strategies are used for reversible coupling of proteins to polymers. For instance, releasable PEGylation is a common method for the screening of therapeutic proteins in vivo.86,87 The conjugation is based on a cleavable linkage, which is hydrolyzed or reduced (in vivo) by a certain kinetic rate, resulting in the PEG. We believe noncovalent strategies might also be useful to grant AMPs temporal stability under certain conditions (time, pH, temperature).86,88
Amidation is one of the most common coupling techniques, involving several functional groups like activated esters and aldehydes interacting with amine side chains (N-terminus and lysine). Regardless of the success of this method, the number of amine residues in peptides is considerably high, resulting in random conjugations. Nonetheless, amine coupling is still interesting for its ease of synthesis and results in improved pharmacokinetics for AMPs.89
PEGylation by thioester linkage has also been receiving attention more recently. Cysteines are rare in the backbone of peptides and proteins, and therefore when present they provide specific sites for conjugation. Pranantyo and collaborators (2016) used thiol–yne based click chemistry for the conjugation of the AMP HHC10 to phosphoester copolymers.62 The immobilization resulted in slightly lower bactericidal activity but no hemolytic effect at 4000 μg mL−1.
Conjugation to synthetic/natural organic polymers
AMPs can also be associated to antiadhesive polymer brushes to decrease the effect of biofilms.64 Muszanska and collaborators (2014) coupled the amphiphilic copolymer of poly(ethylene oxide)–poly(propylene oxide)–poly(ethylene oxide), Pluronic F127, to an AMP (ILPWRWPWWPWRR-NH2) by amidation at the C-terminal, protecting the AMP from proteolytic degradation.
Xie et al. (2020) demonstrated the conjugation via amidation of the AMP GH12 to the carboxylic acid group of methacrylate (MA). Spacers were inserted to provide flexibility between the peptide and the resin material. The MA–AMP conjugates were copolymerized into dental adhesives and the bactericidal activity was similar to the initial AMPs with the spacers conferring higher stability to the conjugates.65 Kumar et al. (2017) modified the peptide structure of the AMP aurein 2.2 and conjugated it to hyperbranched polyglycerol (HPG) of different molecular weights, resulting in stable low MW HPG conjugates protected from proteolytic enzymes. In particular, peptide 77c (modified from aurein) presented a MIC value of 8 μg mL−1, 4–8 times more active than aurein (MIC 32 μg mL−1), and when conjugated to a 22 kDa HPG, a small loss of microbial activity was observed, with a MIC of 50 μg mL−1. Nonetheless, the conjugate was found to be biocompatible and resistant to tryptic degradation.73 This result is commonly seen in AMP conjugation, i.e. there is a loss of microbial activity, but a considerable gain in biocompatibility, reduced toxicity, and increased half-life. Conjugating to polysaccharides is also an efficient way to improve AMPs and chitosan is one of the most used biopolymers of this class. Its cationic nature leads to an increase in antimicrobial activity. In addition, chitosan is biocompatible and biodegradable, being considered safe for human use.90,91 Different applications of chitosan–AMP conjugates have been investigated. Ju et al. (2020) produced a conjugate of chitosan–poly(ethylene glycol)–peptide AMP LK13 (CS-PEG-LK13) which was more efficient in preventing the formation of P. aeruginosa biofilms in vitro (72.7% of efficiency) than LK13 alone (15.24%) and the antibiotic tobramycin (33.57%). Nonetheless, we cannot clearly attribute the increase in activity to a synergistic effect since the authors did not quantify the antimicrobial activity of chitosan or a simple mixture of chitosan and LK13, and chitosan is well-known for its antimicrobial activity.92 In addition, self-assembled cationic nanoparticles of chitosan-grafted-oligolysine (CSM5-K5) selectively kill bacteria, eliminating multi-drug resistant S. aureus (MRSA) in a murine excisional wound model.93 In another study, thermo-responsive chitosan hydrogels (TCTS) were synthesized associated with different concentrations of the AMP piscidin-1, and the highest concentration of AMP-TCTS (16 μg ml−1) was effective in decreasing the concentration of resistant A. baumannii in vitro.90
Another biopolymer is the thermally responsive elastin-like polypeptide (ELP), which can passively accumulate in tumors, increasing the permeability and retention of drugs conjugated to it. Walker et al. (2012) conjugated the anticancer drug doxorubicin (DOX) to ELP and three different CPPs derived from the bactenecin family of AMPs, also presenting antimicrobial activity, as a strategy to inhibit the growth of tumor cells. All complexes showed phase transition changes under hyperthermic conditions and CPPs increased DOX internalization, highlighting the CPP synB1 that displayed twice as much inhibition as free DOX and reduced toxicity under the same conditions.94
Dextran (Dex) is a polysaccharide available for conjugation to AMPs as its hydroxyl groups bring more solubility to the peptide. Chen et al. (2019) synthesized a series of Dex-g-KnFm copolymers (cationic antimicrobial polymer–AMP derivatives) by a simple thiol–ene click chemistry method and showed that Dex-g-K12.5F12.5–50% was the most promising conjugate, with a MIC of 62.5 μg mL−1, against MRSA. It showed high selectivity and did not induce drug resistance when compared to the aminoglycoside antibiotic amikacin, demonstrating high therapeutic efficacy in sepsis models.95
Conjugation of AMPs to small molecules
Conjugation of peptides to small molecules is of great clinical value and might direct the small molecules to specific tissues, improving pharmacokinetic and pharmacodynamic properties, as well as therapeutic windows.96 Also, AMPs can be conjugated to small molecules to increase antimicrobial activity. Organometallic AMPs (OM-AMPs) are widely studied as materials to potentiate antimicrobial agents. Albada & Metzler-Nolte (2017) described the mechanism of action of OM-AMPs optimized by the use of more active synthetic AMPs (synAMPs), such as the ruthenocene-derived Rc-WRWRW-NH2. Upon interaction with the bacterial membrane, it causes biophysical changes by displacing the vital enzyme MurG and cytochrome c in the cell membrane, thus preventing the formation of cell wall lipids II and making the bacteria more vulnerable.97 In another study by the same authors, the replacement of an N-terminal arginine residue by a metallocenoyl group (such as ferrocene and ruthenocene) in the AMP WRWRW resulted in modulation of the activity against Gram-positive and Gram-negative bacteria with promising results.98
Lipidation of AMPs
Lipidation is acylation with long-chain fatty acids, usually occurring at the N-terminal or lysine residue. It increases the ability to fold short peptides, inducing or stabilizing their secondary structures, improves the antimicrobial activity and promotes the association with cell membranes.99
The short synthetic AMP (Arg-Trp)3 was modified on the lysine side chain positioned at the C- or N-terminal and lipidated in the amino group, increasing the activity against pathogenic Gram-negative bacteria.100 Albada et al. (2013) also optimized AMPs by short lipid chains resulting in lower toxicity, decreased hemolytic activity and improved antimicrobial activity against MRSA. According to the authors, the peptides’ lipophilicity and different combinations of L- and D-amino acid residues affect hemolysis and the antibiotic activity.101
AMPs designed through N-terminal lipidation and Cys–Cys dimerization serve as a model for the development of more potent AMPs, with up to a 10-fold increase in antimicrobial activity, neutralization of endotoxins and membrane permeabilization.102 Singh et al. (2018) developed heterogeneous lipid AMPs to mimic Host Defense Peptides (HDPs) and observed that lipidation, positive charge, hydrophobicity and spatial arrangement are important aspects for selectivity to Gram-negative bacterial cells.103
Lipidation can improve tolerance to various physiological conditions, such as different pH values, salt and protease, and increase the antimicrobial activity, but this is not always the case. Modifications with longer carbon chains significantly decrease the bioactivity of the peptide.104 Thus, antimicrobial activity is dependent on acyl chain length and increasing it leads to an increasing tendency to self-assemble, which decreases the peptide–membrane interaction.105 Kamysz et al. (2020) reinforce how toxicity depends on the length of acyl chains; the longer the chains the higher the cytotoxicity against mammalian cells.63 Furthermore, bioactive lipids are usually susceptible to oxidation, which may increase the instability of lipidated AMPs.106
Conjugation of AMPs to antibiotics
One of the major public health problems today is the antibiotic resistance of pathogens in the ESKAPE group (Enterococcus faecium, Staphylococcus aureus, Klebsiella pneumoniae, Acinetobacter baumannii, Pseudomonas aeruginosa, and Enterobacter species) and conjugation of AMPs to the state-of-the-art antibiotics is an interesting alternative to increase activity and reduce cytotoxicity.107 The antibiotic kanamycin, for example, was linked to the AMP P14LRR, giving rise to the P14KanS conjugate which demonstrated potent antibiotic activity against ESKAPE pathogens, prevented the formation of bacterial biofilms and did not exhibit cytotoxicity.108 In another study, the broad-spectrum antibiotic levofloxacin was conjugated to an amphiphilic cyclic AMP [R4W4] and the resulting molecule significantly reduced the bacterial infection by MRSA and K. pneumoniae.109 Ptaszyńska et al. (2019) studied the conjugation of the AMP HLopt2 to the antibiotics levofloxacin (LVX) and ciprofloxacin (CIP), and the antifungal fluconazole; however, the antimicrobial activity increased only for the CIP conjugate (up to 4-fold). Both LVX and CIP were soluble only at pH 5. Nonetheless, all the drugs conjugated to HLopt2 presented improved solubility regardless of pH, thus overcoming limitations on the administration of these drugs.110
Vancomycin (VAN) is an antibiotic used to treat infections by Gram-positive bacteria; however, cases of vancomycin-resistant S. aureus (VRSA) infections have been reported. To improve activity, VAN was conjugated to the AMP Hecate (Hec) and the conjugate (VAN-Hec) presented broad antibiotic activity against VRSA, in addition to non-toxicity.111 More recently, VAN was conjugated to polycationic peptides, increasing antimicrobial activity up to 1000 times.112
AMP nanoparticle system
The discovery of antimicrobial peptides brought a new spectrum of alternatives to combat disease-causing microbial agents. However, as mentioned before, challenges in clinically implementing these AMPs are still present and justify the short number of drugs in use.36 In this sense, nanostructures have the potential to modulate the pharmacokinetics and pharmacodynamics of drugs, including AMPs,113,114 protect against serum proteases and biodegradation, promote chemical stability, preserve the activity of the drug and minimize side effects.115 A variety of materials can be used to produce nanocarriers for AMPs, including inorganic (metal nanoparticles and carbon tubes) and organic nanomaterials (lipid based) (Fig. 4).
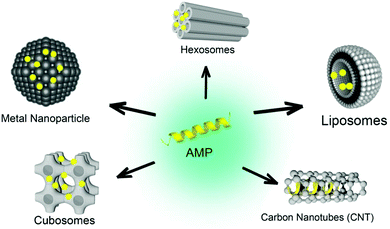 |
| Fig. 4 AMPs conjugated to or encapsulated in nanoparticles. AMPs are represented either as dots and the helicoidal form. Graph designer: João V. Guimarães. | |
Inorganic nanoparticles/metallic nanoparticles
Metallic nanoparticles, such as gold (AuNPs) and silver (AgNPs), present antimicrobial properties, and therefore when combined with AMPs lower doses can be used to decrease the toxicity of nanoparticles to mammalian cells. Alteriis et al. (2018) studied the conjugation of the AMP indolicidin to AuNPs (nano-complex AuNP–indolicidin), which demonstrated significantly higher in vitro activity against C. albicans biofilms (50–65% inhibition) compared to pure AuNPs (10–20% of inhibition) and pure indolicidin (30–40% inhibition), suggesting that the nano-complex is more stable and protects AMP against protease degradation.116 Casciaro et al. (2017) proposed the conjugation of the linear AMPs esculentin-1a and Esc (1–21) to AuNPs via poly(ethylene glycol). In particular, Esc (1–21) is found in frog skin and has strong activity against the bacterium P. aeruginosa. The AuNPs–Esc(1–21) conjugated nano-complex showed a 15-fold increase in the antipseudomonal activity compared to pure Esc (1–21), with no toxicity to human keratinocytes (in vitro) as well as favoring the re-epithelialization and increasing the bioavailability.117
In a study involving the AMP Andersonin-Y1 and variants (CAY1 and AY1C) conjugated to AgNPs, the nano-conjugates also presented higher antibacterial activity than the isolated molecules (peptides and AgNPs), with changes in the cell morphology of the bacterium, followed by rupture of the membrane in multi-resistant strains. AY1C–AgNPs and CAY1–AgNPs presented MIC values of 15 μM and 10 μM against K. pneumonia, and of 12 μM and 10 μM against P. aeruginosa, respectively. More specifically, 80% of bacterial death resulted from the use of these nano-conjugates.118
Carbon nanotubes (CNTs)
CNTs belong to the family of fullerenes; according to the final structure formed by rolled-up tubular shells of graphene, they are classified as the single-walled carbon nanotube (SWCNT) or multiwalled (MWCNT) structure.119 They are promising agents for drug delivery due to their intrinsic stability, structural flexibility and surface functionalization.120 Nonetheless, chronic toxicity is still a concern. Chaudhari and collaborators (2016) proposed the covalent and non-covalent conjugation of the antimicrobial peptide TP359 with SWCNTs-Ag, resulting in FSWCNTs-Ag and SWCNTs-Ag-M nano-complexes, respectively. The antimicrobial activity was measured against S. aureus, S. pyogenes, S. enterica serovar, Typhimurium and E. coli, as well as cellular toxicity by IC50, including the pure peptide and nanoparticles as controls. The nano-conjugate FSWCNTs-Ag was more active at lower concentrations with a MIC of 7.8–3.9 μg mL−1, in comparison to 62.5–31.3 μg mL−1 for SWCNTs-Ag-M (against Gram negative bacteria).121
Conjugation of the AMP indolicidin to CNTs led to the expression of genes related to the innate immune system, also suggesting that the CNT–indolicidin complex protects host cells against bacterial infections at a concentration 1000 times lower compared to free indolicidin. Regarding the gene expression profile, the nano-conjugate is more effective in activating pro-inflammatory genes.122 Recently, Pradhan and colleagues (2017) conjugated LL-37 and indolicidin to short multi-walled carbon nanotubes (SMCNTs); the in vitro immunoregulatory activity of nano-conjugates was significantly higher than that of the dissociated forms of indolicidin and LL-37, and peptide activity was maintained even at a concentration that is 1000 times lower (0.02 μg mL−1 of nano-conjugates and 20 μg mL−1 of free peptides).75
Bioinformatics analysis for discovering new and effective AMP and AMP conjugates
Bioinformatics tools have gained attention in recent years to accelerate and optimize drug development.123–127 Several software programs based on different machine learning methods and databases are available for the prediction and characterization of new antimicrobial peptides.128–133 In general, these tools operate based on the features of amino acid sequences, either by alignment with known sequences with proven activity or by the search for similarity patterns within these sequences.134 Among the most popular databases is CAMP (Collection of Anti-Microbial Peptides), a free online web service available at http://www.bicnirrh.res.in/antimicrobial developed by using the random forest (RF), support vector machine (SVM) and discriminant analysis (DA) machine learning algorithms. It includes experimentally tested peptides, patented peptides and sequences predicted as antimicrobial.135 An evolution of CAMP is CAMPR3 (http://www.camp3.bicnirrh.res.in), comprising sequences, structures, and family-specific signatures of prokaryotic and eukaryotic AMPs.136 The SVM algorithm was also applied in C-PAmP (Collection of Anti-Microbial Peptides), a database comprising high scoring computationally predicted antimicrobial peptides from a great variety of plant species.137 Although plants are a valuable source of peptides, PhytAMP (http://phytamp.pfba-lab-tun.org/statistics.php) is the only database additional to C-PAmP exclusively dedicated to collect validated plant antimicrobial peptides.138 Another essential software program, AmPEP, is of particular interest; while the other tools use a combination of characteristics such as amino acid composition and pseudo-amino acid code, AmPEP is based on several distribution descriptors corresponding to the physicochemical properties of peptides as hydrophobicity, normalized van der Waals volume, polarity, charge, and secondary structure, among others. This tool was developed using the RF classifiers, standing out from the existing methods in terms of performance.139 A recent web platform, ADAPTABLE (Antimicrobial PeptiDescAffold by Property alignmenT. A weB platform for cLustering and dEsign), found at http://gec.u-picardie.fr/adaptable/, is a unified database that allows the design of new antimicrobial peptides active towards a well-defined target organism and predicts the antimicrobial activity of a generic peptide sequence.140 Despite the extensive development of tools and databases to predict AMPs, conjugated AMPs are a different class of molecules. To our knowledge, only two options are available to predict chemically modified AMPs. One of them is CS-AMPPred (Cysteine-Stabilized Antimicrobial Peptides Predictor; http://sourceforge.net/projects/csamppred/), a predictive model generated with the SVM algorithm for antimicrobial activity prediction in cysteine-stabilized peptides.141 Another tool recently created corresponds to AntiMPmod (http://webs.iiitd.edu.in/raghava/antimpmod/), a web server developed with the SVM and RF algorithms to predict the antimicrobial properties of chemically modified peptides based on their tertiary structure.142
The advent of the bioinformatics era has been of great importance, not only for the search and discovery of new peptides with antimicrobial activity but also for the elucidation of mechanisms and structural aspects related to how AMPs act at the molecular level. Regarding that, molecular dynamics simulations and QSAR (quantitative structure–activity relationship) studies offer vast possibilities for the design of more powerful peptides with low toxicity and to understand the structural aspects that define their activity, facilitating detailed visualization of the interactions between peptides and membranes.143–147 These bioinformatics techniques have also been used to generate knowledge about the influence of a particular modification on AMP activity, such as conjugation to nanoparticles.118,148
Additionally, mass spectrometry could be useful as a complementary technique to validate results based on computational methods for identifying new AMPs,149–152 characterizing conjugated AMPs,153 and evaluating susceptibility to proteases.154
Clinical development of AMPs and conjugated AMPs
From the 1980s to now, extensive research in the field of AMPs has been carried out. Thousands of AMPs from different sources, including insects, plants, fungi, bacteria and animals,155 are currently registered in data repositories.156 Despite the advances in this field, only a few AMPs were approved by the Food and Drug Administration (FDA) to treat conditions such as bacterial conjunctivitis, infections by drug-resistant Gram-positive pathogens and skin infections.4,157Table 3 summarizes the currently available AMPs approved by the FDA.
Table 3 List of anti-microbial peptides (AMPs) approved by the FDA
Peptide |
Source |
MW (Da) |
Indication |
Mechanism of action |
Ref. |
Bacitracin |
Homodetic cyclic peptide produced by Bacillus subtilis and Bacillus licheniformis |
1423 |
Skin and eye infections |
Interferes with bacterial cell-wall biosynthesis |
158 and 159
|
Gramicidin |
Heterogeneous mixture of lineal peptides obtained from the soil bacterium Bacillus brevis |
1882 |
Bacterial eye infections |
Formation of small pores in the cell membrane |
160 and 161
|
Dalbavancin |
Semi-synthetic lipoglycopeptide |
1817 |
Acute skin infections |
Induces cell wall lysis |
4 and 162
|
Daptomycin |
Naturally occurring lipopeptide found in the soil saprotroph Streptomyces roseosporus |
1620 |
Complicated skin and skin structure infections |
Membrane disruption |
163 and 164
|
Enfuvirtide |
Synthetic peptide |
4565 |
HIV-1 |
Selective inhibition of viral and cellular membrane fusion |
165
|
Oritavancin |
Semi-synthetic lipoglycopeptide, an analogue of vancomycin |
1793 |
Complicated skin and skin structure infections |
Inhibit bacterial cell wall formation. Membrane disruption |
166
|
Telavancin |
Semisynthetic derivative of vancomycin |
1756 |
Complicated skin and skin structure infections and hospital-acquired/ventilator-associated bacterial pneumonia |
Inhibition of cell wall synthesis and disruption of the bacterial cell membrane |
167 and 168
|
Vancomycin |
Amphoteric glycopeptide antibiotic produced by the soil bacterium Amycolatopsis orientalis |
1449 |
Treatment of serious, life-threatening bacterial infections |
Inhibition of the second stage of cell wall synthesis |
169 and 170
|
Colistin |
Cyclic lipopeptide isolated from Bacillus polymyxa |
1155 |
Treatment of most multidrug-resistant Gram-negative bacteria |
Disruption of the cell membrane |
171 and 172
|
Some peptides are currently undergoing clinical trials and another 10 AMPs have failed during phase II or III of evaluation. Table 4 shows a summary of the main AMPs in clinical trials and their key features. On the other hand, despite several conjugated AMPs developed for different applications, none has reached the clinical investigation stage. Owing to the structural complexity, they require a series of additional characterization tests. Also, antimicrobial peptide conjugates are a relatively new strategy, and we expect to see potential alternatives within the next few years.
Table 4 Main physicochemical and biological properties of antimicrobial peptides in the clinical stage
Peptide |
Source |
M
W (Da) |
Length (aa) |
Indication |
Mechanism of action |
Company |
Ref. |
Phase I clinical trials |
hLF1-11 |
Derived from the N-terminus of human lactoferrin |
1374 |
11 |
Systemic infections with C. albicans, S. aureus, and A. baumannii strains |
Modulatory activities on monocytes, thus enhancing their actions in innate immune responses |
AM-Pharma |
173–175
|
WAP-8294A |
Synthetic cyclic lipodepsipeptides |
1563 |
12 |
Potent activity against methicillin-resistant S. aureus |
Membrane disruption |
aRigen |
176 and 177
|
Phase II clinical trials |
CZEN-002 |
Dimeric peptide derived from α-melanocyte-stimulating hormone |
971 |
8 |
Vaginal candidiasis |
cAMP accumulation in Candida albicans and disrupts related signaling pathways |
Zengen |
7
|
DPK-060 |
Synthetic peptide structurally derived from human protein kininogen |
2503 |
20 |
Broad-spectrum activity in vitro against both Gram-positive and Gram-negative bacteria. Study of topical application for atopic dermatitis |
Membrane disruption and immunomodulation |
ProMore Pharma |
178–180
|
PAC-113 |
Derived from natural histatin found in human saliva |
1564 |
12 |
Candida infections. Fungal/yeast infections. Inflammation and ulceration |
Membrane disruption and immunomodulation |
Demegen |
181 and 182
|
Lytixar |
Synthetic host defense peptide- mimicking |
788 |
3 |
Skin infection caused by Gram-positive bacteria, impetigo and nasally colonized with S. aureus |
Membrane-degrading peptide |
Lytix biopharma |
183 and 184
|
LL-37 |
Human cathelicidin-derived peptide |
4491 |
37 |
Wound healing, regeneration and angiogenesis |
Immunomodulation and pore formation on the bacterial membrane |
ProMore Pharma |
185–187
|
Novexatin |
Synthetic cyclic peptide based on human α- and β-defensins |
1093 |
7 |
Topical treatment of recalcitrant fungal infections in toenails |
Membrane disruption/permeabilization |
NovaBiotics |
188 and 189
|
Glutoxim |
Synthetic thiopetin analog of key metabolites |
657 |
6 |
Tuberculosis |
Immunomodulation |
Pharma BAM |
190 and 191
|
Phase II clinical trials |
Omiganan pentahydro-chloride |
Synthetic analog of indolicidin |
1961 |
12 |
Prevention of catheter-related infections. Treatment of acne and rosacea |
Membrane disruption and immunomodulation |
Cutanea life sciences |
192–194
|
Summary and future outlook
Modified AMPs should be designed considering three fundamental issues: stability, toxicity, and costs, since usually bioconjugation increases the price of the final product. As discussed in this review, different strategies can lead to stability enhancement, lower toxicity, less susceptibility to proteolytic attack and reduction of immunogenicity. In some cases, increased antimicrobial activity can also be obtained, but not frequently. We believe that sustained-release dosage forms can also be achieved through a variety of formulations of AMPs including liposomes and polymer conjugates. The controlled release of AMPs not only prolongs the antimicrobial activity but might also keep drug levels within the therapeutic window to avoid potentially harmful peaks of drug concentration following ingestion or injection. In situ tools are another ally for the design of new tailor-made AMP conjugates with a specific improvement over the original drug. Nonetheless, the increased cost associated to AMPs’ conjugation and drug delivery systems is still a challenge.
Concerns regarding the safety of peptides when compared to conventional and widely used antibiotics are still present.195 A considerable number of AMPs failed in clinical trials due to side effects, for example, NVB-302 (phase I), POL7080 (phase II), and Iseganan, Omiganan, Surotomycin (phase III).196–198 This has drawn the efforts of the pharmaceutical industry towards investing on AMPs for topical use rather than parenteral application. However, conjugation and/or encapsulation could be alternatives to improve safety, as presented in this review. In this sense maintaining the AMP integrity, identifying the suitable polymer for conjugation, and achieving optimum drug loading are key issues to consider while developing the process.
While several conjugation and bioconjugation techniques have been explored so far, novel nanobiotechnological approaches show high potential for creating follow-on antimicrobial peptide drugs. Genetic engineering advances allow the development of effective AMP conjugates that can be real alternatives to treat antibiotic-resistant bacterial infections and septic shock, to preserve food, or to sanitize surfaces both in vitro and in vivo. The attachment of cleavable PEGs, conjugation to FDA-approved smart polymers and the use of nanoparticles will be hot trends.
Conflicts of interest
There are no conflicts to declare.
Acknowledgements
The authors are grateful for the financial support of the São Paulo Research Foundation (FAPESP, Process #2018/25994-2), the Brazilian National Council for Scientific and Technological Development (CNPq, Process # 301832/2017-0) and the Coordination for the Improvement of Higher Education Personnel – Brazil (CAPES, Finance Code 001).
References
- E. Christaki, M. Marcou and A. Tofarides, J. Mol. Evol., 2020, 88, 26–40 CrossRef CAS PubMed.
- World Health Organization, Interagency Coordination Group on Antimicrobial Resistance. No time to wait: securing the future from drug-resistant infections, Report to the secretary-general of the united nations, 2019, 1, 28 Search PubMed.
- M. C. Wolfgang, C. M. Dozois, M. Schmelcher, E. Zurich, M. M. Margitmahlapuu, M. Mahlapuu, J. Håkansson, L. Ringstad and C. Björn, Front. Cell. Infect. Microbiol., 2016, 6, 194 Search PubMed.
- J. Lei, L. C. Sun, S. Huang, C. Zhu, P. Li, J. He, V. Mackey, D. H. Coy and Q. Y. He, Am. J. Transl. Res., 2019, 11, 3919–3931 CAS.
- W. Aoki and M. Ueda, Pharmaceuticals, 2013, 6, 1055–1081 CrossRef PubMed.
- J. Li, J. J. Koh, S. Liu, R. Lakshminarayanan, C. S. Verma and R. W. Beuerman, Front. Neurosci., 2017, 11, 73 Search PubMed.
- C. D. Fjell, J. A. Hiss, R. E. W. Hancock and G. Schneider, Nat. Rev. Drug Discovery, 2012, 11, 37–51 CrossRef CAS PubMed.
- R. Manteghi, E. Pallagi, G. Olajos and I. Csóka, Eur. J. Pharm. Sci., 2020, 144, 105197 CrossRef PubMed.
- K. Loth, A. Vergnes, C. Barreto, S. N. Voisin, H. Meudal, J. Da Silva, A. Bressan, N. Belmadi, E. Bachère, V. Aucagne, C. Cazevielle, H. Marchandin, R. D. Rosa, P. Bulet, L. Touqui, A. F. Delmas and D. Destoumieux-Garzón, mBio, 2019, 10, 1819–1821 CrossRef PubMed.
- T. Florin, C. Maracci, M. Graf, P. Karki, D. Klepacki, O. Berninghausen, R. Beckmann, N. Vázquez-Laslop, D. N. Wilson, M. V. Rodnina and A. S. Mankin, Nat. Publ. Gr., 2017, 24, 752–757 CAS.
- M. G. Gagnon, R. N. Roy, I. B. Lomakin, T. Florin, A. S. Mankin and T. A. Steitz, Nucleic Acids Res., 2016, 44, 2439–2450 CrossRef CAS PubMed.
- N. Mookherjee, M. A. Anderson, H. P. Haagsman and D. J. Davidson, Nat. Rev. Drug Discovery, 2020, 19, 311–332 CrossRef CAS PubMed.
- Z. Cui, Q. Luo, M. S. Bannon, V. P. Gray, T. G. Bloom, M. F. Clore, M. A. Hughes, M. A. Crawford and R. A. Letteri, Biomater. Sci., 2021, 9, 5069–5091 RSC.
- H. Sun, Y. Hong, Y. Xi, Y. Zou, J. Gao and J. Du, Biomacromolecules, 2018, 19, 1701–1720 CrossRef CAS PubMed.
- I. W. Hamley, Biomacromolecules, 2014, 15, 1543–1559 CrossRef CAS PubMed.
- M. R. Yeaman and N. Y. Yount, Pharmacol. Rev., 2003, 55, 27–55 CrossRef CAS PubMed.
- M. Pasupuleti, A. Schmidtchen and M. Malmsten, Crit. Rev. Biotechnol., 2012, 32, 143–171 CrossRef CAS PubMed.
-
M. Zasloff, Antimicrobial Peptides in Health and Disease Washington, DC, 2002 Search PubMed.
- L. T. Nguyen, E. F. Haney and H. J. Vogel, Trends Biotechnol., 2011, 29, 464–472 CrossRef CAS PubMed.
- K. A. Brogden, Nat. Rev. Microbiol., 2005, 3, 238–250 CrossRef CAS PubMed.
- L. J. Zhang and R. L. Gallo, Curr. Biol., 2016, 26, 14–19 CrossRef PubMed.
- D. Takahashi, S. K. Shukla, O. Prakash and G. Zhang, Biochimie, 2010, 92, 1236–1241 CrossRef CAS PubMed.
- R. E. W. Hancock and H. G. Sahl, Nat. Biotechnol., 2006, 24, 1551–1557 CrossRef CAS PubMed.
- A. T. Y. Yeung, S. L. Gellatly and R. E. W. Hancock, Cell. Mol. Life Sci., 2011, 68, 2161–2176 CrossRef CAS PubMed.
- M. Malmsten, Curr. Top. Med. Chem., 2016, 16, 16–24 CrossRef CAS PubMed.
- M. G. Scott, H. Yan and R. E. W. Hancock, Infect. Immun., 1999, 67, 2005–2009 CrossRef CAS PubMed.
- M. G. Scott, M. R. Gold and R. E. W. Hancock, Infect. Immun., 1999, 67, 6445–6453 CrossRef CAS PubMed.
- Y. Lai and R. L. Gallo, Trends Immunol., 2009, 30, 131–141 CrossRef CAS PubMed.
- T. Ebenhan, O. Gheysens, H. G. Kruger, J. R. Zeevaart and M. M. Sathekge, Biomed Res. Int., 2014, 867381 Search PubMed.
- J. P. da Costa, M. Cova, R. Ferreira and R. Vitorino, Appl. Microbiol. Biotechnol., 2015, 99, 2023–2040 CrossRef PubMed.
- B. Mojsoska and H. Jenssen, Pharmaceuticals, 2015, 8, 366–415 CrossRef CAS PubMed.
- S. J. Ludtke, K. He, W. T. Heller, T. A. Harroun, L. Yang and H. W. Huang, Biochemistry, 1996, 35, 13723–13728 CrossRef CAS PubMed.
- Y. Pouny, D. Rapaport, Y. Shai, A. Mor and P. Nicolas, Biochemistry, 1992, 31, 12416–12423 CrossRef CAS PubMed.
- N. Raheem and S. K. Straus, Front. Microbiol., 2019, 2866 CrossRef PubMed.
- N. K. Brogden and K. A. Brogden, Int. J. Antimicrob. Agents, 2011, 38, 217–225 CAS.
- A. I. Gomaa, C. Martinent, R. Hammami, I. Fliss and M. Subirade, Front. Chem., 2017, 5, 103 CrossRef PubMed.
- Á. Martin-Serrano, R. Gómez, P. Ortega and F. J. de la Mata, Pharmaceutics, 2019, 11, 448 CrossRef PubMed.
- S. J. Kang, S. J. Park, T. Mishig-Ochir and B. J. Lee, Expert Rev. Anti-Infect. Ther., 2014, 12, 1477–1486 CrossRef CAS PubMed.
- R. Zheng, B. Yao, H. Yu, H. Wang, J. Bian and F. Feng, Peptides, 2010, 31, 1674–1677 CrossRef CAS PubMed.
- G. Guidotti, L. Brambilla and D. Rossi, Trends Pharmacol. Sci., 2017, 38, 406–424 CrossRef CAS PubMed.
- D. Raucher and J. S. Ryu, Trends Mol. Med., 2015, 21, 560–570 CrossRef CAS PubMed.
- M. Zorko, S. Jones and Ü. Langel, Adv. Drug Delivery Rev., 2022, 180, 114044 CrossRef CAS PubMed.
- H. Lee, S. I. Lim, S. H. Shin, Y. Lim, J. W. Koh and S. Yang, ACS Omega, 2019, 4, 15694–15701 CrossRef CAS PubMed.
- Z. Li, X. Wang, D. Teng, R. Mao, Y. Hao, N. Yang, H. Chen, X. Wang and J. Wang, Eur. J. Med. Chem., 2018, 145, 263–272 CrossRef CAS PubMed.
- J. J. Eksteen, D. Ausbacher, T. Vasskog, Ø. Rekdal and J. S. M. Svendsen, ACS Omega, 2020, 5, 4937–4942 CrossRef CAS PubMed.
- Y. K. Reshetnyak, O. A. Andreev, M. Segala, V. S. Markin and D. M. Engelman, Proc. Natl. Acad. Sci. U. S. A., 2008, 105, 15340–15345 CrossRef CAS PubMed.
- K. E. Burns, T. P. McCleerey and D. Thévenin, Sci. Rep., 2016, 6, 1–10 CrossRef PubMed.
- J. H. Jang, M. Y. Kim, J. W. Lee, S. C. Kim and J. H. Cho, Peptides, 2011, 32, 895–899 CrossRef CAS PubMed.
- F. Touti, G. Lautrette, K. D. Johnson, J. C. Delaney, A. Wollacott, H. Tissire, K. Zacharys, S. K. Mong, A. J. Mijalis, O. J. Plante and B. L. Pentelute, ChemBioChem, 2018, 19, 2039–2044 CrossRef CAS PubMed.
- J. Y. Shu, B. Panganiban and T. Xu, Annu. Rev. Phys. Chem., 2013, 64, 631–657 CrossRef CAS PubMed.
- N. Wiradharma, M. Y. S. Sng, M. Khan, Z.-Y. Ong and Y.-Y. Yang, Macromol. Rapid Commun., 2013, 34, 74–80 CrossRef CAS PubMed.
- M. Fernández-Vidal, S. Jayasinghe, A. S. Ladokhin and S. H. White, J. Mol. Biol., 2007, 370, 459–470 CrossRef PubMed.
- G. N. Grover and H. D. Maynard, Curr. Opin. Chem. Biol., 2010, 14, 818–827 CrossRef CAS PubMed.
- E. M. Pelegri-O’day and H. D. Maynard, Acc. Chem. Res., 2016, 49, 1777–1785 CrossRef PubMed.
- Z. P. Tolstyka and H. D. Maynard, Polymer Science: A Comprehensive Reference, 2012, 9, 317–337 Search PubMed.
- U. C. Palmiero, M. Sponchioni, N. Manfredini, M. Maraldi and D. Moscatelli, Polym. Chem., 2018, 9, 4084–4099 RSC.
- M. S. Messina, K. M. M. Messina, A. Bhattacharya, H. R. Montgomery and H. D. Maynard, Prog. Polym. Sci., 2020, 100, 101186 CrossRef CAS PubMed.
- F. M. Veronese and G. Pasut, Drug Discovery Today, 2005, 10, 1451–1458 CrossRef CAS PubMed.
- G. Pasut and F. M. Veronese, J. Controlled Release, 2012, 161, 461–472 CrossRef CAS PubMed.
- C. J. Arnusch, R. J. Pieters and E. Breukink, PLoS One, 2012, 7, 39768 CrossRef PubMed.
- M. Barbosa, N. Vale, F. M. T. A. Costa, M. C. L. Martins and P. Gomes, Carbohydr. Polym., 2017, 165, 384–393 CrossRef CAS PubMed.
- D. Pranantyo, L. Q. Xu, E. T. Kang, M. K. Mya and M. B. Chan-Park, Biomacromolecules, 2016, 17, 4037–4044 CrossRef CAS PubMed.
- E. Kamysz, E. Sikorska, M. Jaśkiewicz, M. Bauer, D. Neubauer, S. Bartoszewska, W. Barańska-Rybak and W. Kamysz, Int. J. Mol. Sci., 2020, 21, 887 CrossRef CAS PubMed.
- A. K. Muszanska, E. T. J. Rochford, A. Gruszka, A. A. Bastian, H. J. Busscher, W. Norde, H. C. Van Der Mei and A. Herrmann, Biomacromolecules, 2014, 15, 2019–2026 CrossRef CAS PubMed.
- S.-X. Xie, L. Song, E. Yuca, K. Boone, R. Sarikaya, S. K. VanOosten, A. Misra, Q. Ye, P. Spencer and C. Tamerler, ACS Appl. Polym. Mater., 2020, 2, 1134–1144 CrossRef CAS PubMed.
- D. Pranantyo, L. Q. Xu, E. T. Kang and M. B. Chan-Park, Biomacromolecules, 2018, 19, 2156–2165 CrossRef CAS PubMed.
-
Z. Muganli, G. Onak, U. K. Ercan and O. Karaman, Medical Technologies Congress (TIPTEKNO), IEEE, 2019, pp. 1–4 Search PubMed.
- Z. Lin, T. Wu, W. Wang, B. Li, M. Wang, L. Chen, H. Xia and T. Zhang, Int. J. Biol. Macromol., 2019, 140, 330–342 CrossRef CAS PubMed.
- T. H. C. Petrin, V. Fadel, D. B. Martins, S. A. Dias, A. Cruz, L. M. Sergio, M. Arcisio-Miranda, M. A. R. B. Castanho and M. P. Dos Santos Cabrera, Biomacromolecules, 2019, 20, 2743–2753 CrossRef CAS PubMed.
- H. Jiang, M. Xiong, Q. Bi, Y. Wang and C. Li, Acta Pharm. Sin. B, 2016, 6, 319–328 CrossRef PubMed.
- J. Ruczyński, I. Rusiecka, K. Turecka, A. Kozłowska, M. Alenowicz, I. Gągało, A. Kawiak, P. Rekowski, K. Waleron and I. Kocić, Sci. Rep., 2019, 9, 1–15 Search PubMed.
- F. Le Guern, V. Sol, C. Ouk, P. Arnoux, C. Frochot and T. S. Ouk, Bioconjugate Chem., 2017, 28, 2493–2506 CrossRef CAS PubMed.
- P. Kumar, A. Takayesu, U. Abbasi, M. T. Kalathottukaren, S. Abbina, J. N. Kizhakkedathu and S. K. Straus, ACS Appl. Mater. Interfaces, 2017, 9, 37575–37586 CrossRef CAS PubMed.
- A. Song, A. A. Rane and K. L. Christman, Acta Biomater., 2012, 8, 41–50 CrossRef CAS PubMed.
- B. Pradhan, D. Guha, K. C. Murmu, A. Sur, P. Ray, D. Das and P. Aich, J. Nanobiotechnol., 2017, 15, 44 CrossRef PubMed.
- K. Abdul Ghaffar, W. M. Hussein, Z. G. Khalil, R. J. Capon, M. Skwarczynski and I. Toth, Curr. Drug Delivery, 2015, 12, 108–114 CrossRef CAS PubMed.
- S. A. Mohid, A. Ghorai, H. Ilyas, K. H. Mroue, G. Narayanan, A. Sarkar, S. K. Ray, K. Biswas, A. K. Bera, M. Malmsten, A. Midya and A. Bhunia, Colloids Surf., B, 2019, 176, 360–370 CrossRef PubMed.
- X. Y. Cai, J. Z. Li, N. N. Li, J. C. Chen, E. T. Kang and L. Q. Xu, Biomater. Sci., 2016, 4, 1663–1672 RSC.
- A. Vijayan, P. P. James, C. K. Nanditha and G. S. Vinod Kumar, Int. J. Nanomed., 2019, 14, 2253–2263 CrossRef CAS PubMed.
- C. Sánchez-Trasviña, M. Rito-Palomares and J. González-Valdez, Adv. Polym. Technol., 2019, 2019, 1–10 CrossRef.
- F. M. Veronese, Biomaterials, 2001, 22, 405–417 CrossRef CAS PubMed.
- E. M. Pelegri-O’Day, N. M. Matsumoto, K. Tamshen, E. D. Raftery, U. Y. Lau and H. D. Maynard, Bioconjugate Chem., 2018, 29, 3739–3745 CrossRef PubMed.
- R. Nordström, L. Nyström, H. Ilyas, H. S. Atreya, B. C. Borro, A. Bhunia and M. Malmsten, Colloids Surf., A, 2019, 565, 8–15 CrossRef.
- S. R. Dennison, S. M. Reddy, L. H. G. Morton, F. Harris, K. Badiani and D. A. Phoenix, Biochim. Biophys. Acta, Biomembr., 2022, 1864, 183806 CrossRef CAS PubMed.
- J. César Moreira Brito, W. G. Lima, J. M. Resende, D. Cristina Sampaio de Assis, D. Boff, V. N. Cardoso, F. A. Amaral, E. M. Souza-Fagundes, S. O. A. Fernandes and M. Elena de Lima, Int. J. Pharm., 2021, 609, 121156 CrossRef PubMed.
- F. M. Veronese and G. Pasut, Drug Discovery Today: Technol., 2008, 5, 57–64 CrossRef PubMed.
- I. W. Hamley, Biomacromolecules, 2014, 15, 1543–1559 CrossRef CAS PubMed.
- D. Yadav and H. K. Dewangan, J. Biomater. Sci., Polym. Ed., 2020, 1–15 Search PubMed.
- R. M. Broyer, G. N. Grover and H. D. Maynard, Chem. Commun., 2011, 47, 2212–2226 RSC.
- N. Rezaei, H. G. Hamidabadi, S. Khosravimelal, M. Zahiri, Z. A. Ahovan, M. N. Bojnordi, B. S. Eftekhari, A. Hashemi, F. Ganji, S. Darabi and M. Gholipourmalekabadi, Int. J. Biol. Macromol., 2020, 164, 855–862 CrossRef CAS PubMed.
- H. Mellegård, S. P. Strand, B. E. Christensen, P. E. Granum and S. P. Hardy, Int. J. Food Microbiol., 2011, 148, 48–54 CrossRef PubMed.
- X. Ju, J. Chen, M. Zhou, M. Zhu, Z. Li, S. Gao, J. Ou, D. Xu, M. Wu, S. Jiang, Y. Hu, Y. Tian and Z. Niu, ACS Appl. Mater. Interfaces, 2020, 12, 13731–13738 CrossRef CAS PubMed.
- Z. Hou, Y. V. Shankar, Y. Liu, F. Ding, J. L. Subramanion, V. Ravikumar, R. Zamudio-Vázquez, D. Keogh, H. Lim, M. Y. F. Tay, S. Bhattacharjya, S. A. Rice, J. Shi, H. Duan, X.-W. Liu, Y. Mu, N. S. Tan, K. C. Tam, K. Pethe and M. B. Chan-Park, ACS Appl. Mater. Interfaces, 2017, 9, 38288–38303 CrossRef CAS PubMed.
- L. Walker, E. Perkins, F. Kratz and D. Raucher, Int. J. Pharm., 2012, 436, 825–832 CrossRef CAS PubMed.
- Y. Chen, L. Yu, B. Zhang, W. Feng, M. Xu, L. Gao, N. Liu, Q. Wang, X. Huang, P. Li and W. Huang, Biomacromolecules, 2019, 20, 2230–2240 CrossRef CAS PubMed.
- P. M. Drake and D. Rabuka, Curr. Opin. Chem. Biol., 2015, 28, 174–180 CrossRef CAS PubMed.
- B. Albada and N. Metzler-Nolte, Acc. Chem. Res., 2017, 50, 2510–2518 CrossRef CAS PubMed.
- H. Bauke Albada, A. I. Chiriac, M. Wenzel, M. Penkova, J. E. Bandow, H. G. Sahl and N. Metzler-Nolte, Beilstein J. Org. Chem., 2012, 8, 1753–1764 CrossRef PubMed.
- B. P. Ward, N. L. Ottaway, D. Perez-Tilve, D. Ma, V. M. Gelfanov, M. H. Tschöp and R. D. DiMarchi, Mol. Metab., 2013, 2, 468–479 CrossRef CAS PubMed.
- H. B. Albada, P. Prochnow, S. Bobersky, S. Langklotz, P. Schriek, J. E. Bandow and N. Metzler-Nolte, ACS Med. Chem. Lett., 2012, 3, 980–984 CrossRef CAS PubMed.
- H. B. Albada, P. Prochnow, S. Bobersky, S. Langklotz, J. E. Bandow and N. Metzler-Nolte, ACS Comb. Sci., 2013, 15, 585–592 CrossRef CAS PubMed.
- A. Datta, P. Kundu and A. Bhunia, J. Colloid Interface Sci., 2016, 461, 335–345 CrossRef CAS PubMed.
- S. Singh, A. Nimmagadda, M. Su, M. Wang, P. Teng and J. Cai, Eur. J. Med. Chem., 2018, 155, 398–405 CrossRef CAS PubMed.
- C. Zhong, T. Liu, S. Gou, Y. He, N. Zhu, Y. Zhu, L. Wang, H. Liu, Y. Zhang, J. Yao and J. Ni, Eur. J. Med. Chem., 2019, 182, 111636 CrossRef CAS PubMed.
- E. Grimsey, D. W. P. Collis, R. Mikut and K. Hilpert, Biochim. Biophys. Acta, Biomembr., 2020, 1862, 183195 CrossRef CAS PubMed.
- C. C. Berton-Carabin, M. H. Ropers and C. Genot, Compr. Rev. Food Sci. Food Saf., 2014, 13, 945–977 CrossRef CAS.
- I. S. Hwang, J. S. Hwang, J. H. Hwang, H. Choi, E. Lee, Y. Kim and D. G. Lee, Curr. Microbiol., 2013, 66, 56–60 CrossRef CAS PubMed.
- M. F. Mohamed, A. Brezden, H. Mohammad, J. Chmielewski and M. N. Seleem, Biochim. Biophys. Acta, Gen. Subj., 2017, 1861, 848–859 CrossRef CAS PubMed.
- N. Riahifard, K. Tavakoli, J. Yamaki, K. Parang and R. Tiwari, Molecules, 2017, 22, 957 CrossRef PubMed.
- N. Ptaszyńska, K. Gucwa, K. Olkiewicz, A. Łegowska, J. Okońska, J. Ruczyński, A. Gitlin-Domagalska, D. Debowski, S. Milewski and K. Rolka, ACS Chem. Biol., 2019, 14, 2233–2242 Search PubMed.
- P. Jelinkova, Z. Splichal, A. M. J. Jimenez, Y. Haddad, A. Mazumdar, V. P. Sur, V. Milosavljevic, P. Kopel, H. Buchtelova, R. Guran, O. Zitka, L. Richtera, D. Hegerova, Z. Heger, A. Moulick and V. Adam, Infect. Drug Resist., 2018, 11, 1807–1817 CrossRef CAS PubMed.
- F. Umstätter, C. Domhan, T. Hertlein, K. Ohlsen, E. Mühlberg, C. Kleist, S. Zimmermann, B. Beijer, K. D. Klika, U. Haberkorn, W. Mier and P. Uhl, Angew. Chem., Int. Ed., 2020, 59, 8823–8827 CrossRef PubMed.
- A. C. Apolinário, J. De Almeida Pachioni-Vasconcelos, A. Pessoa and C. De Oliveira Rangel-Yagui, Quim. Nova, 2017, 40, 810–817 Search PubMed.
- F. U. Din, W. Aman, I. Ullah, O. S. Qureshi, O. Mustapha, S. Shafique and A. Zeb, Int. J. Nanomed., 2017, 12, 7291–7309 CrossRef PubMed.
- A. Wicki, D. Witzigmann, V. Balasubramanian and J. Huwyler, J. Controlled Release, 2015, 200, 138–157 CrossRef CAS PubMed.
- E. de Alteriis, V. Maselli, A. Falanga, S. Galdiero, F. M. Di Lella, R. Gesuele, M. Guida and E. Galdiero, Infect. Drug
Resist., 2018, 11, 915–925 CrossRef CAS PubMed.
- B. Casciaro, M. Moros, S. Rivera-Fernández, A. Bellelli, J. M. de la Fuente and M. L. Mangoni, Acta Biomater., 2017, 47, 170–181 CrossRef CAS PubMed.
- I. Pal, D. Bhattacharyya, R. K. Kar, D. Zarena, A. Bhunia and H. S. Atreya, Sci. Rep., 2019, 9, 4485 CrossRef PubMed.
- N. Saifuddin, A. Z. Raziah and A. R. Junizah, J. Chem., 2013, 676815 Search PubMed.
- D. Lombardo, M. A. Kiselev and M. T. Caccamo, J. Nanomater., 2019, 1–26 Search PubMed.
- A. A. Chaudhari, D. Ashmore, S. Deb Nath, K. Kate, V. Dennis, S. R. Singh, D. R. Owen, C. Palazzo, R. D. Arnold, M. E. Miller and S. R. Pillai, J. Nanobiotechnol., 2016, 14, 1–15 CrossRef PubMed.
- A. Sur, B. Pradhan, A. Banerjee and P. Aich, PLoS One, 2015, 10, 0123905 CrossRef PubMed.
- S. Gill, A. Christopher, V. Gupta and P. Bansal, Perspect. Clin. Res., 2016, 7, 115 CrossRef PubMed.
- J. Vamathevan, D. Clark, P. Czodrowski, I. Dunham, E. Ferran, G. Lee, B. Li, A. Madabhushi, P. Shah, M. Spitzer and S. Zhao, Nat. Rev. Drug Discovery, 2019, 18, 463–477 CrossRef CAS PubMed.
- X. Xia, Curr. Top. Med. Chem., 2017, 17, 1709–1726 CrossRef CAS PubMed.
- X. Lin, X. Li and X. Lin, Molecules, 2020, 25, 1375 CrossRef CAS PubMed.
- S. Dibyajyoti, E. T. Bin and P. Swati, Gall. Med. J., 2013, 18, 44 CrossRef.
- Z. Wang and G. Wang, Nucleic Acids Res., 2004, 32, 590–592 CrossRef PubMed.
- G. Wang, X. Li and Z. Wang, Nucleic Acids Res., 2009, 37, 933–937 CrossRef PubMed.
- X. Zhao, H. Wu, H. Lu, G. Li and Q. Huang, PLoS One, 2013, 8, 66557 CrossRef PubMed.
- J. H. Jhong, Y. H. Chi, W. C. Li, T. H. Lin, K. Y. Huang and T. Y. Lee, Nucleic Acids Res., 2019, 47, D285–D297 CrossRef CAS PubMed.
- X. Kang, F. Dong, C. Shi, S. Liu, J. Sun, J. Chen, H. Li, H. Xu, X. Lao and H. Zheng, Sci. Data, 2019, 6, 1–10 CrossRef CAS PubMed.
- G. Wang, X. Li and Z. Wang, Nucleic Acids Res., 2016, 44, 1087–1093 CrossRef PubMed.
- W. F. Porto, A. S. Pires and O. L. Franco, Biotechnol. Adv., 2017, 35, 337–349 CrossRef CAS PubMed.
- S. Thomas, S. Karnik, R. S. Barai, V. K. Jayaraman and S. Idicula-Thomas, Nucleic Acids Res., 2009, 38, 774–780 CrossRef PubMed.
- F. H. Waghu, R. S. Barai, P. Gurung and S. Idicula-Thomas, Nucleic Acids Res., 2016, 44, 1094–1097 CrossRef PubMed.
- A. Niarchou, A. Alexandridou, E. Athanasiadis and G. Spyrou, PLoS One, 2013, 8, 79728 CrossRef PubMed.
- R. Hammami, J. Ben Hamida, G. Vergoten and I. Fliss, Nucleic Acids Res., 2009, 37, 963–968 CrossRef PubMed.
- P. Bhadra, J. Yan, J. Li, S. Fong and S. W. I. Siu, Sci. Rep., 2018, 8, 1–10 CAS.
- F. Ramos-Martín, T. Annaval, S. Buchoux, C. Sarazin and N. D’Amelio, Life Sci. Alliance, 2019, 2, 1–11 Search PubMed.
- W. F. Porto, Á. S. Pires and O. L. Franco, PLoS One, 2012, 7, 51444 CrossRef PubMed.
- P. Agrawal and G. P. S. Raghava, Front. Microbiol., 2018, 9, 2551 CrossRef PubMed.
- A. Langham and Y. N. Kaznessis, Methods Mol. Biol., 2010, 618, 267–285 CrossRef CAS PubMed.
- D. A. Phoenix, S. R. Dennison and F. Harris, Antimicrob. Pept., 2013, 223–231 Search PubMed.
- Y. Wang, D. E. Schlamadinger, J. E. Kim and J. A. McCammon, Biochim. Biophys. Acta, Biomembr., 2012, 1818, 1402–1409 CrossRef CAS PubMed.
- M. Faya, R. S. Kalhapure, H. M. Kumalo, A. Y. Waddad, C. Omolo and T. Govender, J. Drug Delivery Sci. Technol., 2018, 44, 153–171 CrossRef CAS.
- G. Kasetty, P. Papareddy, M. Kalle, V. Rydengård, M. Mörgelin, B. Albiger, M. Malmsten and A. Schmidtchen, Antimicrob. Agents Chemother., 2011, 55, 2880–2890 CrossRef CAS PubMed.
- A. F. Ferreira, A. Rai, L. Ferreira and P. N. Simões, Eur. Biophys. J., 2017, 46, 247–256 CrossRef CAS PubMed.
- M. L. Juba, P. S. Russo, M. Devine, S. Barksdale, C. Rodriguez, K. A. Vliet, J. M. Schnur, M. L. Van Hoek and B. M. Bishop, J. Proteome Res., 2015, 14, 4282–4295 CrossRef CAS PubMed.
- Y. Gao, D. Wu, X. Xi, Y. Wu, C. Ma, M. Zhou, L. Wang, M. Yang, T. Chen and C. Shaw, Molecules, 2016, 21, 1667 CrossRef CAS PubMed.
- A. Sharma, R. Nigam, A. Kumar and S. Singh, Protein Pept. Lett., 2019, 27, 225–235 CrossRef PubMed.
- M. Preianò, G. Maggisano, M. Murfuni, C. Villella, C. Colica, A. Fregola, C. Pelaia, N. Lombardo, G. Pelaia, R. Savino and R. Terracciano, Int. J. Mol. Sci., 2018, 19, 4005 CrossRef.
- N. Ardila-Chantré, A. K. Hernández-Cardona, H. M. Pineda-Castañeda, S. M. Estupiñan-Torres, A. L. Leal-Castro, R. Fierro-Medina, Z. J. Rivera-Monroy and J. E. García-Castañeda, RSC Adv., 2020, 10, 29580–29586 RSC.
-
M. Bagheri and R. E. W. Hancock, Methods in Molecular Biology, Humana Press Inc., 2017, vo. 1548, pp. 61–71 Search PubMed.
- A. Bahar and D. Ren, Pharmaceuticals, 2013, 6, 1543–1575 CrossRef PubMed.
- L. Fan, J. Sun, M. Zhou, J. Zhou, X. Lao, H. Zheng and H. Xu, Sci. Rep., 2016, 6, 1–7 CrossRef PubMed.
- C. H. Chen and T. K. Lu, Antibiotics, 2020, 9, 24 CrossRef CAS PubMed.
- N. J. Economou, S. Cocklin and P. J. Loll, Proc. Natl. Acad. Sci. U. S. A., 2013, 110, 14207–14212 CrossRef CAS PubMed.
- I. M. Lockhart and E. P. Abraham, Biochem. J., 1954, 58, 633–647 CrossRef CAS PubMed.
- N. Kessler, H. Schuhmann, S. Morneweg, U. Linne and M. A. Marahiel, J. Biol. Chem., 2004, 279, 7413–7419 CrossRef CAS PubMed.
- F. Wang, L. Qin, C. J. Pace, P. Wong, R. Malonis and J. Gao, ChemBioChem, 2012, 13, 51–55 CrossRef CAS PubMed.
- A. Malabarba and B. P. Goldstein, J. Antimicrob. Chemother., 2005, 55, 15–20 CrossRef PubMed.
- W. R. Miller, A. S. Bayer and C. A. Arias, Cold Spring Harbor Perspect. Med., 2016, 6, 026997 Search PubMed.
- J. N. Steenbergen, J. Alder, G. M. Thorne and F. P. Tally, J. Antimicrob. Chemother., 2005, 55, 283–288 CrossRef CAS PubMed.
- M. C. Jamjian and I. R. McNicholl, Am. J. Heal. Pharm., 2004, 61, 1242–1247 CrossRef CAS PubMed.
- S. Rosenthal, A. G. Decano, A. Bandali, D. Lai, G. E. Malat and T. E. Bias, Pharm. Ther., 2018, 43, 143 Search PubMed.
- L. D. Saravolatz, G. E. Stein and L. B. Johnson, Clin. Infect. Dis., 2009, 49, 1908–1914 CrossRef CAS PubMed.
- J. A. Karlowsky, K. Nichol and G. G. Zhanel, Clin. Infect. Dis., 2015, 61, S58–S68 CrossRef CAS PubMed.
- C. Watanakunakorn, J. Antimicrob. Chemother., 1984, 14, 7–18 CrossRef CAS PubMed.
- E. Binda, F. Marinelli and G. Marcone, Antibiotics, 2014, 3, 572–594 CrossRef PubMed.
- S. Biswas, J. M. Brunel, J. C. Dubus, M. Reynaud-Gaubert and J. M. Rolain, Expert Rev. Anti-Infect. Ther., 2012, 10, 917–934 CrossRef CAS PubMed.
- A. Z. Bialvaei and H. Samadi Kafil, Curr. Med. Res. Opin., 2015, 31, 707–721 CrossRef CAS PubMed.
- A. M. van der Does, P. J. Hensbergen, S. J. Bogaards, M. Cansoy, A. M. Deelder, H. C. van Leeuwen, J. W. Drijfhout, J. T. van Dissel and P. H. Nibbering, J. Immunol., 2012, 188, 5012–5019 CrossRef CAS PubMed.
- A. Lupetti, C. P. J. M. Brouwer, S. J. P. Bogaards, M. M. Welling, E. de Heer, M. Campa, J. T. van Dissel, R. H. E. Friesen and P. H. Nibbering, J. Infect. Dis., 2007, 196, 1416–1424 CrossRef CAS PubMed.
- P. H. Nibbering, E. Ravensbergen, M. M. Welling, L. A. Van Berkel, P. H. C. Van Berkel, E. K. J. Pauwels and J. H. Nuijens, Infect. Immun., 2001, 69, 1469–1476 CrossRef CAS PubMed.
- H. Itoh, K. Tokumoto, T. Kaji, A. Paudel, S. Panthee, H. Hamamoto, K. Sekimizu and M. Inoue, J. Org. Chem., 2018, 83, 6924–6935 CrossRef CAS PubMed.
- X. Chen, S. Li, L. Yu, A. Miller and L. Du, Microb. Biotechnol., 2019, 12, 1430–1440 CrossRef CAS PubMed.
- A. Schmidtchen, M. Pasupuleti, M. Mörgelin, M. Davoudi, J. Alenfall, A. Chalupka and M. Malmsten, J. Biol. Chem., 2009, 284, 17584–17594 CrossRef CAS PubMed.
- R. Nordström, L. Nyström, O. C. J. Andrén, M. Malkoch, A. Umerska, M. Davoudi, A. Schmidtchen and M. Malmsten, J. Colloid Interface Sci., 2018, 513, 141–150 CrossRef PubMed.
- A. L. Harvey, R. Edrada-Ebel and R. J. Quinn, Nat. Rev. Drug Discovery, 2015, 14, 111–129 CrossRef CAS PubMed.
-
D. J. Cheng, F. G. Oppenheim and E. J. Helmerhorst, WO2009005798A3, 2009.
- H. B. Koo and J. Seo, Pept. Sci., 2019, 111, e24122 Search PubMed.
- M. Wang, T. Odom and J. Cai, Expert Opin. Ther. Pat., 2020, 30, 303–305 CrossRef CAS PubMed.
- M. F. Mohamed, A. Abdelkhalek and M. N. Seleem, Sci. Rep., 2016, 6, 1–14 CrossRef PubMed.
- R. Esfandiyari, R. Halabian, E. Behzadi, H. Sedighian, R. Jafari and A. A. Imani Fooladi, Heliyon, 2019, 5, 02652 CrossRef PubMed.
- R. Koczulla, G. Von Degenfeld, C. Kupatt, F. Krötz, S. Zahler, T. Gloe, K. Issbrücker, P. Unterberger, M. Zaiou, C. Lebherz, A. Karl, P. Raake, A. Pfosser, P. Boekstegers, U. Welsch, P. S. Hiemstra, C. Vogelmeier, R. L. Gallo, M. Clauss and R. Bals, J. Clin. Invest., 2003, 111, 1665–1672 CrossRef CAS PubMed.
- D. Xhindoli, S. Pacor, M. Benincasa, M. Scocchi, R. Gennaro and A. Tossi, Biochim. Biophys. Acta, Biomembr., 2016, 1858, 546–566 CrossRef CAS PubMed.
- R. Roudi, N. L. Syn and M. Roudbary, Front. Immunol., 2017, 8, 1320 CrossRef PubMed.
- D. K. Mercer, C. S. Stewart, L. Miller, J. Robertson, V. M. S. Duncan and D. A. O’Neil, Antimicrob. Agents Chemother., 2019, 63, 2117–2118 CrossRef PubMed.
- L. A. Kozhemyakin, N. I. Chalisova, O. S. Ketlinskaya, V. A. Penniyainen, A. V. Komashnya and A. D. Nozdrachev, Dokl. Biol. Sci., 2004, 398, 376–378 CrossRef CAS PubMed.
- S. Döşler, İstanbul J. Pharm., 2017, 47, 72–76 CrossRef.
- H. S. Sader, K. A. Fedler, R. P. Rennie, S. Stevens and R. N. Jones, Antimicrob. Agents Chemother., 2004, 48, 3112–3118 CrossRef CAS PubMed.
- H. W. Grievink, S. M. G. Jirka, T. D. Woutman, M. Schoonakker, R. Rissmann, K. E. Malone, G. Feiss and M. Moerland, Clin. Transl. Sci., 2020, 13, 891–895 CAS.
- E. Rubinchik, D. Dugourd, T. Algara, C. Pasetka and H. D. Friedland, Int. J. Antimicrob. Agents, 2009, 34, 457–461 CrossRef CAS PubMed.
- L. Otvos and J. D. Wade, Front. Chem., 2014, 2, 62 Search PubMed.
- P. Kosikowska and A. Lesner, Expert Opin. Ther. Pat., 2016, 26, 689–702 CrossRef CAS PubMed.
- K. E. Greber and M. Dawgul, Curr. Top. Med. Chem., 2017, 17, 620–628 CrossRef CAS PubMed.
- J. M. Sierra, E. Fusté, F. Rabanal, T. Vinuesa and M. Viñas, Expert Opin. Biol. Ther., 2017, 17, 663–676 CrossRef PubMed.
|
This journal is © The Royal Society of Chemistry 2022 |
Click here to see how this site uses Cookies. View our privacy policy here.