DOI:
10.1039/D1SC07124F
(Edge Article)
Chem. Sci., 2022,
13, 2783-2788
Electrooxidative palladium- and enantioselective rhodium-catalyzed [3 + 2] spiroannulations†
Received
21st December 2021
, Accepted 9th February 2022
First published on 10th February 2022
Abstract
Despite indisputable progress in the development of electrochemical transformations, electrocatalytic annulations for the synthesis of biologically relevant three-dimensional spirocyclic compounds has as of yet not been accomplished. In sharp contrast, herein, we describe the palladaelectro-catalyzed C–H activation/[3 + 2] spiroannulation of alkynes by 1-aryl-2-naphthols. Likewise, a cationic rhodium(III) catalyst was shown to enable electrooxidative [3 + 2] spiroannulations via formal C(sp3)–H activations. The versatile spiroannulations featured a broad substrate scope, employing electricity as a green oxidant in lieu of stoichiometric chemical oxidants under mild conditions. An array of spirocyclic enones and diverse spiropyrazolones, bearing all-carbon quaternary stereogenic centers were thereby accessed in a user-friendly undivided cell setup, with molecular hydrogen as the sole byproduct.
Introduction
Spirocycles feature inherent three-dimensionality and represent a distinct structural scaffold. This privileged motif has been increasingly utilized in drug discovery, among others.1 Thus, spirocyclic building blocks with an all-carbon quaternary center feature a higher fraction of sp3 hybridization (Fsp3), which was recently regarded as a new parameter for drug-likeness.2 In addition, spirocycles with spirofluorenyl naphthalenone (SFNP) structures exhibit broad applications in organic optoelectronic materials (Fig. 1a).3 However, the synthesis of all-carbon spiro skeletons is not only associated with increased synthetic efforts, but has also been limited by broadly applicable and robust methods.4 Transition metal-catalyzed C–H functionalization5 has emerged as an increasingly efficient tool to construct spirocyclic compounds.6 Enol/enolate-directed ruthenium-,7 rhodium-8 or palladium-catalyzed9 oxidative [3 + 2]/[3 + 3]/[4 + 1] annulations via C–H functionalization10 were thus reported. Similarly, transition metal-catalyzed C–H activation/dearomatization tandem processes of naphthols11 and phenols12 also proved to be viable (Fig. 1b). Despite the indisputable advances, the use of stoichiometric amounts of chemical oxidants has been necessary for the regeneration of the active catalyst. In the past few years, electricity has been recognized as an efficient tool for the assembly of two-dimensional ring scaffolds.13 In sharp contrast, electrocatalytic methods are, thus far, unfortunately not available for the synthesis of 3D spirocyclic rings.14 Within our program on sustainable electrocatalytic C–H functionalizations,15 we have now developed the first electrocatalytically-enabled spiroannulation (Fig. 1c). Notable features of our strategy include (a) unprecedented electrooxidative C(sp2)–H activation/dearomatization by versatile palladium catalysis, (b) enantioselective [3 + 2] spiroannulation with a chiral rhodium catalyst, (c) electricity as a benign oxidant in lieu of stoichiometric chemical oxidants, (d) mild reaction conditions for high chemo- and enenatio-selectivity, (e) and an operationally-convenient undivided cell setup.
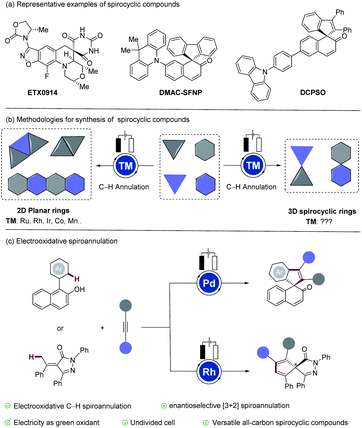 |
| Fig. 1 (a) Representative examples of spirocyclic compounds. (b) Methodologies for synthesis of spirocyclic compounds. (c) Electrooxidative spiroannulations. | |
Results and discussion
Optimization of the reaction conditions
We initiated our studies by probing different catalysts, among which only Pd(OAc)2 performed with encouraging catalytic efficacy. The desired dearomatization product 3aa was thereby obtained in 50% yield. After considerable optimization of the supporting electrolyte (entries 6–13, for details see ESI†), we were pleased to find that the desired transformation was efficiently realized with NMe4Cl and 2.0 equivalents of substrate 1a in an undivided cell setup (entry 13). Replacing the electrolyte NMe4Cl by n-Bu4NOAc led to a slight increase of the yield (entry 14). The conversion decreased significantly, when the catalyst loading was reduced (entry 16). Remarkably, the optimal result was obtained without K2CO3, affording the product 3aa in 87% yield (entry 17). Furthermore, control experiments showed the indispensability of the electricity (entries 18) (Table 1).
Table 1 Optimization of reaction conditionsa
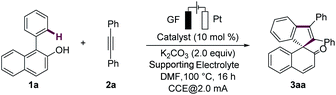
|
Entry |
Catalyst |
Supporting electrolyte |
Yieldb/% |
Reaction conditions: 1a (176 mg, 0.4 mmol), 2a (70 mg, 0.4 mmol), catalyst (10 mol%), supporting electrolyte (1.0 equiv.), base (2.0 equiv.), DMF (4.0 mL) at 100 °C, 16 h, under air.
Yield of isolated product.
Using 1a (0.8 mmol), 2a (0.4 mmol).
8 h.
Using Pd(OAc)2 (5 mol%).
Without K2CO3.
Without current.
|
1 |
[RuCl2(p-cymene)]2 |
n-Bu4NOAc |
Trace |
2 |
[RhCp*Cl2]2 |
n-Bu4NOAc |
Trace |
3 |
[Cp*Co(CO)I2] |
n-Bu4NOAc |
Trace |
4
|
[Cp*Co(MeCN)3](SbF6)2 |
n-Bu4NOAc |
Trace |
5
|
Pd(OAc)2 |
n-Bu4NOAc |
50% |
6 |
Pd(OAc)2 |
n-Bu4NPF6 |
37% |
7 |
Pd(OAc)2 |
n-Bu4NBF4 |
45% |
8
|
Pd(OAc)2 |
NMe4Cl |
46% |
9 |
Pd(OAc)2 |
KCl |
25% |
10 |
Pd(OAc)2 |
n-Bu4NI |
33% |
11 |
Pd(OAc)2 |
Et4NClO4 |
44% |
12 |
Pd(OAc)2 |
n-Bu4NClO4 |
39% |
13
|
Pd(OAc)2 |
NMe4Cl |
71% |
14
|
Pd(OAc)2 |
n-Bu4NOAc |
79% |
15c,d |
Pd(OAc)2 |
n-Bu4NOAc |
64% |
16
,
|
Pd(OAc)2 |
n-Bu4NOAc |
25% |
17
,
|
Pd(OAc)2 |
n-Bu4NOAc |
87% |
18
,
,
|
Pd(OAc)2 |
n-Bu4NOAc |
15% |
Mechanistic studies and gram-scale reaction
Then, we set out to study the working mode of the electrooxidative palladium-catalyzed spiroannulation. First, cyclovoltammetric analysis (Scheme 1a) of the substrates revealed an irreversible oxidation event of the 1-aryl-naphthalenol 1a at Ep = −0.3 V. Compounds 2a and 3aa were found to be stable within the examined potential window. When employing Pt instead of glassy carbon as the working electrode (WE) material, a strong reduction current was observed at −1.5 V, being indicative of an efficient proton reduction as the cathodic event to form molecular hydrogen as the only stoichiometric byproduct (see ESI† for more details). Next, an intermolecular competition reaction was performed to determine the kinetic isotope effect (Scheme 1b). Thus, a KIE kH/kD of 3.0 was observed, suggesting that the C–H scission was involved in the rate-determining step. Finally, the robustness of the electrocatalysis was demonstrated by a gram-scale synthesis (Scheme 1c).
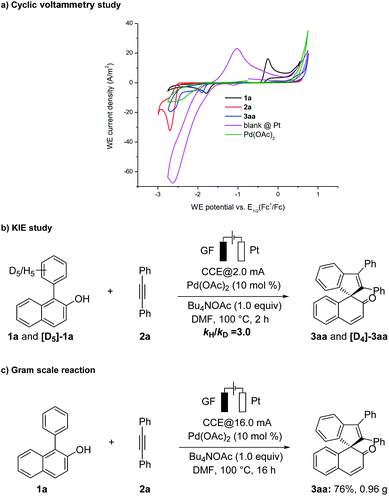 |
| Scheme 1 (a) Cyclic voltammetry, (b) KIE study, (c) gram-scale reaction. | |
Substrate scope
With the optimal reaction conditions in hand, we explored the generality of the approach by testing different alkynes 2 (Scheme 2). Substrates with electron-donating groups, such as methyl and methoxy substituents, afforded products 3ab and 3ac in high yields. Alkynes with the electron-deficient trifluoromethyl and alkoxycarbonyl groups on the tolane could also be converted into the desired spirocycles 3ad and 3ae, albeit with slightly diminished yields due to the relatively low conversion of the alkynes. However, when the halogen substituents (3af–3ah) were examined, the bromoaryl-alkyne showed an apparent drop in the yield as compared with the fluoro or chloro analogs. Next, a series of alkynes bearing substituents at the meta- or otho-position of the arene were found to be suitable to give products 3ai–3am. In addition, the unsymmetrical alkyne 2n was utilized for the dearomative spiroannulation, and the corresponding product 3an was selectively obtained, while products 3o and 3p were not observed under otherwise identical reaction conditions.
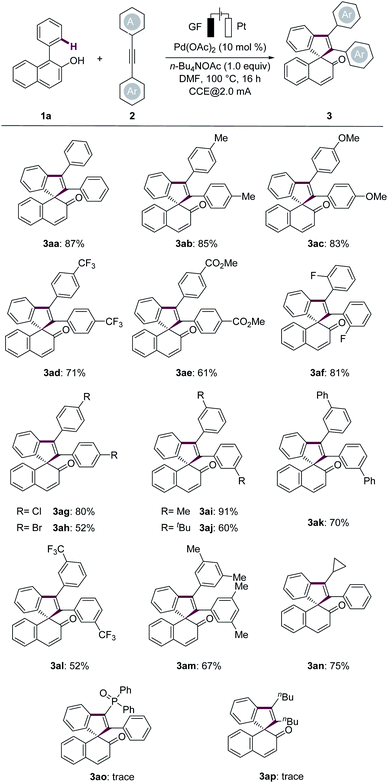 |
| Scheme 2 Palladaelectro-catalyzed spiroannulation with alkynes 2. | |
Subsequently, the reaction scope was further examined by varying the substitution pattern on the naphthols 1 (Scheme 3). An array of electron-donating groups on the arene motif was found to be compatible with the electrocatalytic process (3ba–3bc). It is noteworthy that synthetically-useful electron-withdrawing groups, such as cyano (3bd), acetyl (3be), formyl (3bf), ester (3bg), nitro (3bh) chloro (3bi), and different fluoro-containing groups were tolerated well. The reaction of substrates with meta-substituents occurred regioselectively at the less sterically hindered position, delivering products 3bm and 3bn in 80% and 82% yield, respectively. The chloro group remained intact in products 3bi and 3bn, thus offering handles for further late-stage manipulations. The electrocatalysis enabled the transformation of ortho-fluoro-substituted substrate, affording the product 3bo in 54% yield. When binaphthalen-2-ol was subjected to the pallada-electrocatalysis, the spirocycle 3bp was furnished in excellent yield. An aryl-substituent on the naphthyl ring was tolerated likewise (3bq).
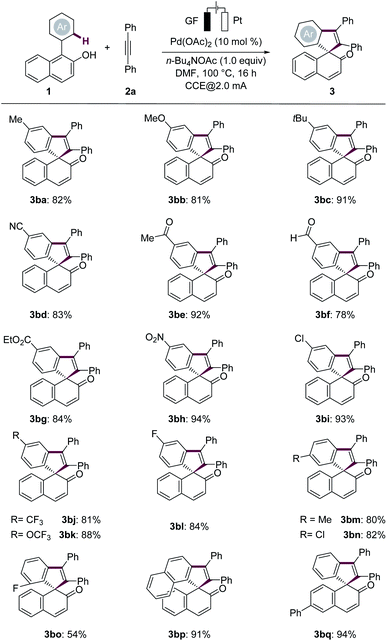 |
| Scheme 3 Palladaelectro-catalyzed spiroannulation with 1-aryl-naphthalenol 1. | |
Given the topical interest in enantio-selective electrocatalyzed C–H activation,16 we explored an asymmetric electrooxidative palladium-catalyzed spiroannulation (Table 2). Thus, amino acid ligand 1 and chiral phosphoric acid diester ligand 2 were inter alia initially probed (entries 1 and 2). Unfortunately, no desired product was formed. As an alternative, chiral rhodium catalyst [Rh]-1 was examined, but exhibited no catalytic efficiency. Meanwhile, and inspired by a rhodium(III)-catalyzed enantioselective spiroannulation,8c the substrate α-arylidene pyrazolone 1b was, among others, considered for the envisioned rhodaelectro-catalyzed oxidative formal [3 + 2] annulation. It is noteworthy that the cationic rhodium(III) catalyst proved effective for this electrocatalytic transformation. Thereby, the desired biorelevant spiropyrazolone 4a could be obtained in 67% yield (entry 4).17 Importantly, a minor adjustment of the catalytic system, set the stage for an enantioselective transformation. An excellent enantio-selectivity and a high conversion were hence observed under mild reaction conditions with the catalyst [Rh]-1 (entry 5).
Table 2 Optimization of enantioselective electrocatalysis
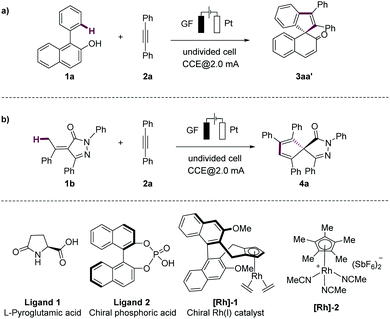
|
Entry |
1a/1b |
Catalyst |
Additive |
Yield/% |
Reaction conditions: 1a (0.8 mmol, 2.0 equiv.), 2a (0.4 mmol), PdCl2 (10 mol%), ligand 1 (20 mol%), NMe4Cl (1.0 equiv.), DMF (4.0 mL) at 100 °C, 16 h, under air.
Reaction conditions: 1a (0.8 mmol, 2.0 equiv.), 2a (0.4 mmol), PdCl2 (10 mol%), ligand 2 (20 mol%), NMe4Cl (1.0 equiv.), DMF (4.0 mL) at 100 °C, 16 h, under air.
Reaction conditions: 1a (0.8 mmol, 2.0 equiv.), 2a (0.4 mmol), [Rh]-1 (5.0 mol%), (BzO)2 (5.0 mol%), n-Bu4NOAc (1.0 equiv.), 1,4-dioxane/H2O (3 : 1) (4.0 mL) at 100 °C, 16 h, under air.
Reaction conditions: 1b (1.5 equiv., 0.3 mmol), 2a (0.2 mmol), [Rh]-2 (2.5 mol%), KOAc (2.0 equiv.), AdCO2H (10 mol%), MeOH (4.0 mL), at 35 °C, 6 h, under air.
Reaction conditions: 1b (1.5 equiv., 0.15 mmol), 2a (0.1 mmol), [Rh]-1 (10 mol%), (BzO)2 (10 mol%), n-Bu4NPF6 (3.0 equiv.), MeOH (4.0 mL), at 35 °C, 6 h, under air.
|
1a |
1a
|
PdCl2 |
Ligand 1
|
Trace |
2b |
1a
|
PdCl2 |
Ligand 2
|
Trace |
3c |
1a
|
[Rh]-1
|
(BzO)2 |
Trace |
4d |
1b
|
[Rh]-2
|
AdCO2H |
67% |
5e |
1b
|
[Rh]-1
|
(BzO)2 |
57%/93:7 er |
Next, we investigated the functional group tolerance of the enantioselective rhodaelectrocatalysis (Scheme 4). Halogen groups, such as fluoro and chloro substituents, proved compatible with the rhoda-electrocatalysis (4b and 4b′). Likewise, different substituents at the para- or meta-position were examined, featuring high catalytic performance and selectivities (4c–4e, 4c′–4e′). In addition, an unsymmetrically-substituted alkyne was tested to afford the product 4f with excellent yield, while no conversion was found for the enantioselective electrocatalysis.
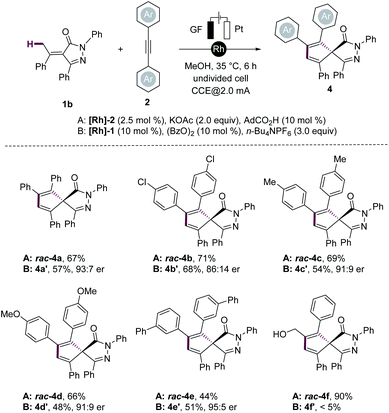 |
| Scheme 4 Enantio-selective rhoda-electrocatalyzed spiroannulation of pyrazolone 1b. | |
Conclusions
We have developed the first pallada-electrocatalyzed C–H activation/dearomative spiroannulation of 1-aryl-2-naphthols, as well as rhoda-electrocatalyzed enantioselective [3 + 2] spiroannulation. The electrocatalysis provided a facile route towards the assembly of a structurally distinct three-dimensional spirocyclic compounds. The unprecedented electrocatalysis was characterized by high chemo-selectivity as well as ample substrate scope, forming molecular hydrogen as the sole byproduct.
Data availability
All experimental data, procedures for data analysis and pertinent data sets are provided in the ESI.†
Author contributions
W. W. and L. A. conceived the project. W. W. conducted the experiments. A. S. performed the CV studies. W. W. and L. A. wrote the manuscript. All authors discussed the results.
Conflicts of interest
There are no conflicts to declare.
Acknowledgements
Generous support by the CSC (fellowship to W. W.), the ERC Advanced Grant no. 101021358, and the DFG (Gottfried-Wilhelm-Leibniz prize to L. A.) is gratefully acknowledged.
Notes and references
-
(a) K. Hiesinger, D. Dar’in, E. Proschak and M. Krasavin, J. Med. Chem., 2021, 64, 150–183 CrossRef CAS PubMed;
(b) T. T. Talele, J. Med. Chem., 2020, 63, 13291–13315 CrossRef CAS PubMed;
(c) Y.-J. Zheng and C. M. Tice, Expert Opin. Drug Discovery, 2016, 11, 831–834 CrossRef PubMed;
(d) Y. Zheng, C. M. Tice and S. B. Singh, Bioorg. Med. Chem. Lett., 2014, 24, 3673–3682 CrossRef CAS PubMed.
- W. Wei, S. Cherukupalli, L. Jing, X. Liu and P. Zhan, Drug Discovery Today, 2020, 25, 1839–1845 CrossRef CAS PubMed.
-
(a) T. P. I. Saragi, T. Spehr, A. Siebert, T. Fuhrmann-Lieker and J. Salbeck, Chem. Rev., 2007, 107, 1011–1065 CrossRef CAS PubMed;
(b) C. Poriel and J. Rault-Berthelot, J. Mater. Chem. C, 2017, 5, 3869–3897 RSC.
-
(a) A. J. Boddy and J. A. Bull, Org. Chem. Front., 2021, 8, 1026–1084 RSC;
(b) L. Zheng and R. Hua, Front. Chem., 2020, 8, 580355 CrossRef CAS PubMed.
-
(a) T. Rogge, N. Kaplaneris, N. Chatani, J. Kim, S. Chang, B. Punji, L. L. Schafer, D. G. Musaev, J. Wencel-Delord, C. A. Roberts, R. Sarpong, Z. E. Wilson, M. A. Brimble, M. J. Johansson and L. Ackermann, Nat. Rev. Methods Primers, 2021, 1, 43 CrossRef CAS;
(b) B. Liu, A. M. Romine, C. Z. Rubel, K. M. Engle and B.-F. Shi, Chem. Rev., 2021, 121, 14957–15074 CrossRef PubMed;
(c) L. Guillemard, N. Kaplaneris, L. Ackermann and M. J. Johansson, Nat. Rev. Chem., 2021, 5, 522–545 CrossRef CAS;
(d) S. Rej, Y. Ano and N. Chatani, Chem. Rev., 2020, 120, 1788–1887 CrossRef CAS PubMed;
(e) P. Gandeepan, L. H. Finger, T. H. Meyer and L. Ackermann, Chem. Soc. Rev., 2020, 49, 4254–4272 RSC;
(f) C. Sambiagio, D. Schönbauer, R. Blieck, T. Dao-Huy, G. Pototschnig, P. Schaaf, T. Wiesinger, M. F. Zia, J. Wencel-Delord, T. Besset, B. U. W. Maes and M. Schnürch, Chem. Soc. Rev., 2018, 47, 6603–6743 RSC;
(g) Y. Park, Y. Kim and S. Chang, Chem. Rev., 2017, 117, 9247–9301 CrossRef CAS PubMed;
(h) Z. Dong, Z. Ren, S. J. Thompson, Y. Xu and G. Dong, Chem. Rev., 2017, 117, 9333–9403 CrossRef CAS PubMed;
(i) B. Ye and N. Cramer, Acc. Chem. Res., 2015, 48, 1308–1318 CrossRef CAS PubMed;
(j) T. Satoh and M. Miura, Chem.–Eur. J., 2010, 16, 11212–11222 CrossRef CAS PubMed;
(k) D. A. Colby, R. G. Bergman and J. A. Ellman, Chem. Rev., 2010, 110, 624–655 CrossRef CAS PubMedY. Li and S. Xu, Chem.–Eur. J., 2018, 24, 16218–16245 CrossRef CAS PubMed.
- Y. Li and S. Xu, Chem.–Eur. J., 2018, 24, 16218–16245 CrossRef CAS PubMed.
- S. Reddy Chidipudi, I. Khan and H. W. Lam, Angew. Chem., Int. Ed., 2012, 51, 12115–12119 CrossRef CAS PubMed.
-
(a) Y. Wang, X. Huang, Q. Wang, Y. Tang, S. Xu and Y. Li, Org. Lett., 2021, 23, 757–761 CrossRef CAS PubMed;
(b) L. Kong, X. Han, S. Liu, Y. Zou, Y. Lan and X. Li, Angew. Chem., Int. Ed., 2020, 59, 7188–7192 CrossRef CAS PubMed;
(c) H. Li, R. Gontla, J. Flegel, C. Merten, S. Ziegler, A. P. Antonchick and H. Waldmann, Angew. Chem., Int. Ed., 2019, 58, 307–311 CrossRef CAS PubMed;
(d) J. Zheng, S.-B. Wang, C. Zheng and S.-L. You, Angew. Chem., Int. Ed., 2017, 56, 4540–4544 CrossRef CAS PubMed;
(e) J. Zheng, P. Li, M. Gu, A. Lin and H. Yao, Org. Lett., 2017, 19, 2829–2832 CrossRef CAS PubMed;
(f) S. Reddy Chidipudi, D. J. Burns, I. Khan and H. W. Lam, Angew. Chem., Int. Ed., 2015, 54, 13975–13979 CrossRef CAS PubMed;
(g) D. J. Burns, D. Best, M. D. Wieczysty and H. W. Lam, Angew. Chem., Int. Ed., 2015, 54, 9958–9962 CrossRef CAS PubMed;
(h) A. Seoane, N. Casanova, N. Quiñones, J. L. Mascareñas and M. Gulías, J. Am. Chem. Soc., 2014, 136, 7607–7610 CrossRef CAS PubMed.
-
(a) I. Khan, S. R. Chidipudi and H. W. Lam, Chem. Commun., 2015, 51, 2613–2616 RSC;
(b) J. D. Dooley, S. Reddy Chidipudi and H. W. Lam, J. Am. Chem. Soc., 2013, 135, 10829–10836 CrossRef CAS PubMed.
- W. K. Yuan and B. F. Shi, Angew. Chem., Int. Ed., 2021, 60, 23187–23192 CrossRef CAS PubMed.
-
(a) J. Wu, L. Bai, L. Han, J. Liu and X. Luan, Chem. Commun., 2021, 57, 1117–1120 RSC;
(b) W. Liang, Y. Yang, M. Yang, M. Zhang, C. Li, Y. Ran, J. Lan, Z. Bin and J. You, Angew. Chem., Int. Ed., 2021, 60, 3493–3497 CrossRef CAS PubMed;
(c) J. Hao, Y. Ge, L. Yang, J. Wang and X. Luan, Tetrahedron Lett., 2021, 71, 153050 CrossRef CAS;
(d) B. Tan, L. Liu, H. Zheng, T. Cheng, D. Zhu, X. Yang and X. Luan, Chem. Sci., 2020, 11, 10198–10203 RSC;
(e) L. Ding, W.-T. Wu, L. Zhang and S.-L. You, Org. Lett., 2020, 22, 5861–5865 CrossRef CAS PubMed;
(f) B. Tan, L. Bai, P. Ding, J. Liu, Y. Wang and X. Luan, Angew. Chem., Int. Ed., 2019, 58, 1474–1478 CrossRef CAS PubMed;
(g) L. Han, H. Wang and X. Luan, Org. Chem. Front., 2018, 5, 2453–2457 RSC;
(h) G. Duarah, P. P. Kaishap, B. Sarma and S. Gogoi, Chem.–Eur. J., 2018, 24, 10196–10200 CrossRef CAS PubMed;
(i) L. Ding and S.-L. You, Org. Lett., 2018, 20, 6206–6210 CrossRef CAS PubMed;
(j) J. Zheng, S.-B. Wang, C. Zheng and S.-L. You, J. Am. Chem. Soc., 2015, 137, 4880–4883 CrossRef CAS PubMed;
(k) H. Zheng, L. Bai, J. Liu, J. Nan, Z. Zuo, L. Yang, Y. Wang and X. Luan, Chem. Commun., 2015, 51, 3061–3064 RSC;
(l) S. Gu, L. Luo, J. Liu, L. Bai, H. Zheng, Y. Wang and X. Luan, Org. Lett., 2014, 16, 6132–6135 CrossRef CAS PubMed;
(m) J. Nan, Z. Zuo, L. Luo, L. Bai, H. Zheng, Y. Yuan, J. Liu, X. Luan and Y. Wang, J. Am. Chem. Soc., 2013, 135, 17306–17309 CrossRef CAS PubMed.
-
(a) Z. Zuo, J. Wang, J. Liu, Y. Wang and X. Luan, Angew. Chem., Int. Ed., 2020, 59, 653–657 CrossRef CAS PubMed;
(b) Y. Li, Z. Tang, J. Zhang and L. Liu, Chem. Commun., 2020, 56, 8202–8205 RSC;
(c) Y. Ge, C. Qin, L. Bai, J. Hao, J. Liu and X. Luan, Angew. Chem., Int. Ed., 2020, 59, 18985–18989 CrossRef CAS PubMed;
(d) P.-P. Lin, X.-L. Han, G.-H. Ye, J.-L. Li, Q. Li and H. Wang, J. Org. Chem., 2019, 84, 12966–12974 CrossRef CAS PubMed;
(e) J. Nan, Y. Yuan, L. Bai, J. Liu and X. Luan, Org. Lett., 2018, 20, 7731–7734 CrossRef CAS PubMed;
(f) W. Hu, H. Wang, L. Bai, J. Liu and X. Luan, Org. Lett., 2018, 20, 880–883 CrossRef CAS PubMed;
(g) L. Luo, H. Zheng, J. Liu, H. Wang, Y. Wang and X. Luan, Org. Lett., 2016, 18, 2082–2085 CrossRef CAS PubMed;
(h) Z. Zuo, X. Yang, J. Liu, J. Nan, L. Bai, Y. Wang and X. Luan, J. Org. Chem., 2015, 80, 3349–3356 CrossRef CAS PubMed;
(i) L. Yang, H. Zheng, L. Luo, J. Nan, J. Liu, Y. Wang and X. Luan, J. Am. Chem. Soc., 2015, 137, 4876–4879 CrossRef CAS PubMed;
(j) S. Kujawa, D. Best, D. J. Burns and H. W. Lam, Chem.–Eur. J., 2014, 20, 8599–8602 CrossRef CAS PubMed.
-
(a) K. Yamamoto, M. Kuriyama and O. Onomura, Acc. Chem. Res., 2020, 53, 105–120 CrossRef CAS PubMed;
(b) F. Wang and S. S. Stahl, Acc. Chem. Res., 2020, 53, 561–574 CrossRef CAS PubMed;
(c) J. C. Siu, N. Fu and S. Lin, Acc. Chem. Res., 2020, 53, 547–560 CrossRef CAS PubMed;
(d) J. L. Röckl, D. Pollok, R. Franke and S. R. Waldvogel, Acc. Chem. Res., 2020, 53, 45–61 CrossRef PubMed;
(e) Q. Jing and K. D. Moeller, Acc. Chem. Res., 2020, 53, 135–143 CrossRef CAS PubMed;
(f) K.-J. Jiao, Y.-K. Xing, Q.-L. Yang, H. Qiu and T.-S. Mei, Acc. Chem. Res., 2020, 53, 300–310 CrossRef CAS PubMed;
(g) T. Fuchigami and S. Inagi, Acc. Chem. Res., 2020, 53, 322–334 CrossRef CAS PubMed;
(h) P. Xiong and H.-C. Xu, Acc. Chem. Res., 2019, 52, 3339–3350 CrossRef CAS PubMed;
(i) H. Wang, X. Gao, Z. Lv, T. Abdelilah and A. Lei, Chem. Rev., 2019, 119, 6769–6787 CrossRef CAS PubMed;
(j) M. Elsherbini and T. Wirth, Acc. Chem. Res., 2019, 52, 3287–3296 CrossRef CAS PubMed;
(k) S. Tang, Y. Liu and A. Lei, Chem, 2018, 4, 27–45 CrossRef CAS;
(l) G. S. Sauer and S. Lin, ACS Catal., 2018, 8, 5175–5187 CrossRef CAS;
(m) J. E. Nutting, M. Rafiee and S. S. Stahl, Chem. Rev., 2018, 118, 4834–4885 CrossRef CAS PubMed;
(n) C. Ma, P. Fang and T.-S. Mei, ACS Catal., 2018, 8, 7179–7189 CrossRef CAS;
(o) M. Yan, Y. Kawamata and P. S. Baran, Chem. Rev., 2017, 117, 13230–13319 CrossRef CAS PubMed;
(p) R. Francke and R. D. Little, Chem. Soc. Rev., 2014, 43, 2492–2521 RSC;
(q) A. Jutand, Chem. Rev., 2008, 108, 2300–2347 CrossRef CAS PubMed.
-
(a) Y. Zhang, C. Ma, J. Struwe, J. Feng, G. Zhu and L. Ackermann, Chem. Sci., 2021, 12, 10092–10096 RSC;
(b) W. C. Yang, M. M. Zhang, Y. Sun, C. Y. Chen and L. Wang, Org. Lett., 2021, 23, 6691–6696 CrossRef CAS PubMed;
(c) D. F. Chen, T. Y. He, Y. C. Jin and S. L. Huang, Adv. Synth. Catal., 2022, 364, 286–290 CrossRef CAS.
-
(a) R. C. Samanta, T. H. Meyer, I. Siewert and L. Ackermann, Chem. Sci., 2020, 11, 8657–8670 RSC;
(b) T. H. Meyer, I. Choi, C. Tian and L. Ackermann, Chem, 2020, 6, 2484–2496 CrossRef CAS;
(c) N. Sauermann, T. H. Meyer, Y. Qiu and L. Ackermann, ACS Catal., 2018, 8, 7086–7103 CrossRef CAS.
-
(a) U. Dhawa, T. Wdowik, X. Hou, B. Yuan, J. C. A. Oliveira and L. Ackermann, Chem. Sci., 2021, 12, 14182–14188 RSC;
(b) U. Dhawa, C. Tian, T. Wdowik, J. C. A. Oliveira, J. Hao and L. Ackermann, Angew. Chem., Int. Ed., 2020, 59, 13451–13457 CrossRef CAS PubMed.
- During the preparation of our manuscript, Mei and coworkers reported on an elegant electrochemical rhodium(III)-catalyzed enantioselective C–H activation through alkyne annulation, Y.-Q. Huang, Z.-J. Wu, L. Zhu, Q. Gu, X. Lu, S.-L. You and T.-S. Mei, CCS Chem., 2021, 3, 3501–3509 CrossRef.
Footnote |
† Electronic supplementary information (ESI) available. See DOI: 10.1039/d1sc07124f |
|
This journal is © The Royal Society of Chemistry 2022 |
Click here to see how this site uses Cookies. View our privacy policy here.