DOI:
10.1039/D1EN00955A
(Critical Review)
Environ. Sci.: Nano, 2022,
9, 61-80
A critical review on surface-modified nano-catalyst application for the photocatalytic degradation of volatile organic compounds
Received
19th October 2021
, Accepted 6th December 2021
First published on 8th December 2021
Abstract
Surface modification of nano-catalysts has gained significant attention due to their outstanding photocatalytic performance with minimum secondary pollution. Photocatalytic oxidation (PCO) is a promising technology for removing volatile organic compounds (VOCs) due to its higher activity with minimum secondary pollution. In this review, we have selected literature from the Web of Science database for nearly 10 years, with most of our sources spanning the past 5 years. The current review summarizes the recent reports of the nano-catalyst surface modification technology, including overcoming the internal and external limitations of nano-catalysts and improving the method of photocatalytic degradation of VOCs. Additionally, we found that surface modification greatly enhances the catalytic performance of the nano-catalyst, which is beneficial for the degradation of VOCs. There are some limitations including low catalytic activity and catalyst stability. So, in future research, new methods of preparing catalysts and improving their overall catalyst performance should be managed and paid more attention.
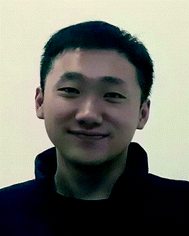 Weichen Zhao | Weichen Zhao obtained a bachelor's degree from the Hebei Agricultural University. He is currently pursuing a master's degree at the China Agricultural University. He is engaged in the research of ecotoxicology and nano-pesticides and is also interested in nano-material photocatalysis. |
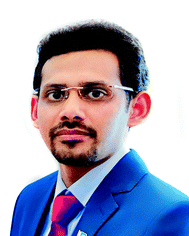 Muhammad Adeel | Dr. Muhammad Adeel serves as a distinguished Associate Professor at Beijing Normal University at Zhuhai. Dr. Adeel has expertise in the accumulation and toxicity of manufactured nano-objects (MNOs) in plants and soil organisms as well as their fate and disposition in the terrestrial environment. He has a broad overview of nano-enabled agriculture; specifically, on the use of nanoscale micronutrients to suppress crop disease and enhance food production. He also has multidisciplinary knowledge including phytoremediation of persistent organic and inorganic pollutants in soil, as well as more generally on the fate of contaminants in soils, sediments, and water. |
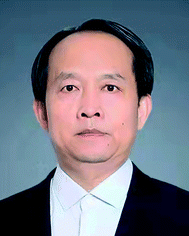 Yukui Rui | Professor Yukui Rui works in the College of Resources and Environment, China Agricultural University. His research interests span food safety, nano toxicology, and environmental remediation. Years of research gave him a deep understanding and unique vision of the application of nanomaterials in agriculture. |
Environmental significance
Photocatalysis is a promising technology in reducing volatile organic compounds (VOCs). However, as a commonly used photocatalyst material, nanomaterials (NMs) have intrinsic and extrinsic factors, which affect photocatalytic activity. Here, we have explained the mechanism behind the photocatalytic degradation of VOC. When the energy of the photon absorbed by the nano-catalyst is equal to or greater than the energy of the bandgap photon, the excited electrons and holes separate and migrate to the surface of the nano-catalyst to participate in the redox reaction. VOC was degraded by O2 and OH˙ into CO2, H2O, and other products. In addition, we have also summarized four nano-catalyst surface modification technologies: doping, surface heterojunction, co-catalyst, and exposing highly active crystal facets. These technologies can improve the efficiency of photocatalytic degradation of VOCs. Further investigations on exploring new high-efficiency nano-catalysts for the degradation of VOCs are warranted.
|
1. Introduction
Air quality has received widespread attention due to its injurious effects on living organisms. Industries, such as petroleum refining,1 chemical production,2 synthetic resin,3 cloth dyeing,4–6 leather processing,7 pharmaceutical industry,2,8 insecticide production,9 coating and adhesive manufacturing,10 spraying,11 printing,4,12 and electronic component manufacturing2,5,13 release a significant amount of volatile organic compounds (VOCs), which ultimately affect the air quality (Fig. 1). Due to easy diffusivity, toxicity, and volatility, VOCs can cause irreversible damage to human health.14–16 The adverse effects of VOCs on human health include not only acute irritation to the eyes and lungs but also chronic diseases such as asthma, gastrointestinal diseases, cardiovascular diseases, and cancer.17–20
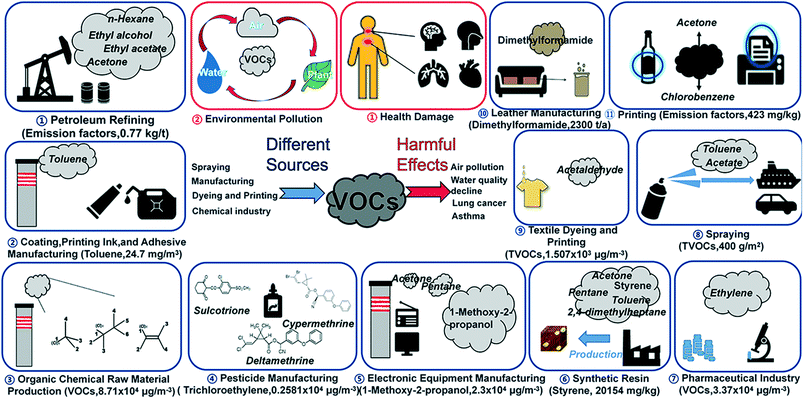 |
| Fig. 1 Illustration of industries releasing VOCs in air, concentration obtained from ref. 47–57. | |
To overcome the devastating effects of VOCs, several efficient purification techniques of VOCs have been developed, such as incineration, condensation, adsorption, photocatalytic oxidation (PCO), and ozone-catalytic oxidation, and membrane separation.21 Compared with these techniques, PCO has many advantages such as room-temperature operation, high activity, and no secondary pollution, which made PCO an auspicious technique.22 Besides, PCO is a powerful air purification technology that destroys VOCs by photocatalysis under the irradiation of ultraviolet (UV) and sunlight, converting them to water, carbon dioxide, and detritus.
Commonly used photocatalyst materials for the purification of VOCs include TiO2, ZnO, WO3, V2O5, ZnS, and CdS.23–25 To date, nanotechnology has made exponential progress.26–28 Nanomaterials (NMs) are widely used in the field of environmental remediation.29 Nowadays, TiO2 has a large number of applications in photocatalysis30–33 due to its high photocatalytic efficiency, stability under extreme conditions, and suitable edge potential to act as active centers for catalytic reactions.34–36 However, the performance of these nano-catalysts is not efficient. For example, compared with other semiconductor materials, TiO2 has a wider band gap and higher carrier recombination rate, which limits the photocatalytic process to the UV region of the spectrum.31,37
Recently, the techniques of modifying nano-catalysts include (i) the use of compound semiconductors i.e., semiconductors made from two or more elements, (ii) catalyst immobilization on solids such as silica or polymeric supports, (iii) use of co-catalysts, (iv) dye sensitization, and (v) surface doping is being applied to fill the shortcomings of nano-catalyst. These techniques not only enable the catalyst to increase visible light utilization efficiency but also increase the lifetime of photoexcited carrier pairs.38–42
Previously published articles give a detailed introduction to the processes for modification of various nano-catalysts.43–46 However, there is no comprehensive review on the impact of modification of nano-catalysts by the different methods on their efficiency and capability to eliminate VOCs. The purpose of this review is thus to classify the techniques according to the surface modification method and review the new features of the modified photocatalyst. Furthermore, new features of the modified photocatalysts are briefly discussed. Photocatalysis fundamentals, factors that affect the catalytic performance of the photocatalyst, and the modification technologies have been illustrated. Through these studies, we can explore the limitations of the current catalysts and use this to further improve the performance of the catalysts, with the overarching goal of contributing to the improved elimination of environmental VOC pollution by nano-catalysis in the future.
2. Mechanism behind photocatalytic oxidation of VOCs
The photocatalytic reaction is a complex process, which begins with the absorption of a large amount of visible light on the surface of the material. When the energy of the absorbed photon is not less than the energy of the semiconductor band gap photon (Eg), the electrons existing in valence bands (VB) will be excited into the empty conduction bands (CB), such that holes are left behind in the VB.58 The following uses TiO2 as an example to analyze the electron and hole generation process: | TiO2 + hv → e−(TiO2) + h+(TiO2) | (1) |
There are three possible processes for electrons and holes: (1) separate and move to the surface of the material to have an opportunity to participate in redox reactions (2) trapped by defect sites. (3) Recombine and release energy. However, the second and the third processes do not promote the photocatalytic reaction, and only the first process can drive reduction and oxidation.59 Before driving the redox reaction, the charge needs to undergo separation, thermalization, trapping, recombination, and transport60 (Fig. 2). Interfacial charge transfer may directly eliminate VOCs through oxidation or generate hydroxyl radicals and superoxides.61 The process can be depicted as the following eqn (2)–(8):62
| h+(TiO2) + H2O → TiO2 + H+OH˙ | (2) |
| h+(TiO2) + OH− → TiO2 + OH˙ | (3) |
| e−(TiO2) + O2 → TiO2 + O2˙− | (4) |
| HO2˙ + HO2˙ → H2O2 + O2 | (6) |
| e−(TiO2) + H2O2 → OH− + OH˙ | (7) |
| VOC + O2 + OH˙ → H2O + CO2 + other products | (8) |
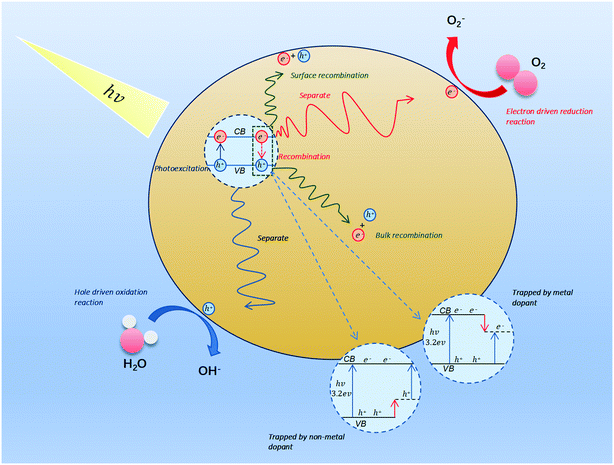 |
| Fig. 2 Schematic of the basic mechanism of photocatalysis. | |
The current model is difficult to explain due to the complex charge transfer process. Understanding the underlying mechanisms will help us to find new photocatalysts for application in VOCs degradation.
3. Factors affecting the photocatalytic activity
The photocatalytic performance is affected by intrinsic and extrinsic factors (Fig. 3). Intrinsic factors affecting photocatalytic ability and VOC degradation have been briefly discussed below.63–65
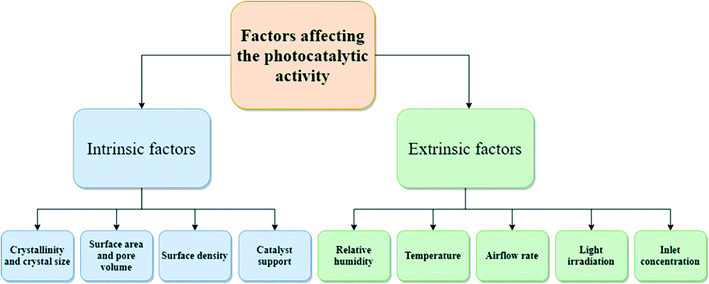 |
| Fig. 3 Illustration of factors affecting photocatalytic activity. | |
3.1 Influence of catalyst characteristics (intrinsic factors) on VOCs degradation
3.1.1 Crystallinity and crystal size.
The presence of defects in the crystal lattice and impurities in the catalyst accelerates the recombination process. To improve the efficiency of photocatalysis, the design and research of high bulk crystallinity have received extensive attention.66
Leite et al.67 claimed that the property of high crystallinity has advantages over disordered polymers in photocatalytic applications. Pleskunov et al.68 used a single-step plasma-based technique to synthesize Ta3NyOx nanoparticles (NPs) with controllable crystallinity. In the visible light range, Ta3NyOx exhibits plasmonic and photoluminescent properties. Katsuki et al.69 found that α-Fe2O3 NPs with high crystallinity are more efficient in PCO. A similar finding was also reported by Li et al.70 They found that the nanorod-shaped photoactive COF containing benzothiadiazole and triazine with good crystallinity exhibited excellent comprehensive performance and good cycle performance in the photocatalytic oxidation reaction. Curtis et al.71 used the two-temperature method to prepare mesoporous silicon NPs. The initial temperature of the reaction is 650 °C and lasted for 0.5 h, and then in the second heating process at 100 °C, 200 °C, and 300 °C for 6 h. They found that the mesoporous silicon NPs prepared at 300 °C have the best photocatalytic performance because of the higher crystallinity of the catalyst. Some studies indicated that synthesizing a new heterogeneous photocatalyst has uniform crystal size and high crystallinity. These advantages accelerate the separation and transfer efficiency of electron–hole pairs.72,73
Besides crystallinity, crystal size also affects photocatalytic activity. Alonso-Tellez et al.74 found that the smaller crystal size of UV100 is the main reason why it is superior to P25 in terms of photocatalytic oxidation. Generally speaking, higher crystallinity and smaller crystal size can promote the reaction rate.
3.1.2 Surface area.
Surface area is an important structural feature of photocatalysts, which has a great influence on photocatalysis.75 The larger the surface area, the more accessible the active sites, and the higher the photocatalytic efficiency.76
Hajaghazadeh et al.77 found that under steady-state conditions, the conversion rate of methyl ethyl ketone (MEK) using PC500 catalyst was higher than that of PC50 and P25. The experimental results show that the lower surface areas of PC50 and P25 make the activity decrease over time. However, PC500 has a high surface area, and its positive impact offsets the negative impact of electrons and holes on rapid recombination. This finding was also reported by Monteiro's group.78 During the degradation of perchloroethylene by P25 and PC500, it was also noticed that the surface area has a greater impact on the conversion of pollutants. Liu et al.79 found that Ag–ZnO NPs have super high photocatalytic efficiency compared to pure ZnO. Researchers speculate that it may be because the Ag NPs are uniformly distributed and have a large specific surface area. Similar results were also reflected in another experiment. Rajca's group tested the removal efficiency of organic substances in the photocatalytic process of commercial nano-catalysts with different accessible surface areas. The results show that because P90 has a larger surface area, the photocatalytic efficiency of P90 is higher than P25.80
3.1.3 Pore volume and porosity.
In addition to the surface area, another structural feature, pore volume, of the photocatalyst also has a profound effect on the catalytic efficiency (Table 1).74 Another group81 designed Pt nanoclusters similar to 1.8 nm. The catalytic performance of C/Pt@TiO2-3% containing 0.54 wt% Pt is greatly improved because its maximum total pore volume and the average pore diameter is approximately 3 nm. In addition, the mesoporous structure also helps to expose more active sites of the nano-catalyst to promote surface reactions.81
Table 1 Summary of different photocatalysts and photoactivity
Photocatalyst |
Surface area |
Pore volume |
Compound |
Photo activity |
Ref. |
TiO2 USprec |
326 m2 g−1 |
0.484 cm3 g−1 |
Benzyl alcohol |
Conversion 61% |
91
|
P25 |
— |
— |
Benzyl alcohol |
Conversion 100% |
91
|
Brookite/anatase TiO2/g-C3N4 |
37.1 m2 g−1 |
0.2 cm3 g−1 |
Phenol |
Degradation rate 5-fold increase over CN |
92
|
CN |
45.8 m2 g−1 |
0.29 cm3 g−1 |
Phenol |
— |
92
|
Ti3+ doped TiO2/SiO2 |
300 m2 g−1 |
0.35 cm3 g−1 |
Methyl orange |
31.5% |
93
|
TiO2 |
56.8 m2 g−1 |
— |
Methyl orange |
8% |
93
|
TiO2/SiO2 |
228 m2 g−1 |
0.27 cm3 g−1 |
Methyl orange |
16.6% |
93
|
While achieving a porous structure, crystallinity will not be lost. Therefore, the general view is that porous structure is a more important catalyst characteristic than crystallinity.62
Porous materials with superior performance are very suitable for capturing aromatic VOCs in ambient air. In recent years, due to high porosity and strong customization, metal organic frameworks (MOFs) have been studied extensively.82–86 MOFs are rich in organic contents, which makes them have superior inherent advantages in adsorbing aromatic VOCs.87
Xie et al.87 designed and synthesized two MOFs, among which [Zr6(μ3-O)4(μ3-OH)4(BDB)6] (BUT-66) shows superior adsorption performance of benzene. Single-crystal structure analysis shows that the small hydrophobic pores and the less interaction between the adsorption sites make BUT-66 have a high performance of capturing benzene. Wu et al.88 used lab-on-fiber technology and nanotechnology to monitor surface nano-functionalization of VOC adsorption/desorption in zeolitic imidazole frameworks (ZIF)-8. The high porosity plays an important role in VOC sensors. This finding was also reported by Wang et al.89 They found that the shape and size of porous Co3O4 derived from Co-MOF would significantly affect its sensing performance. Besides, the more NPs on the surface, the better the VOC sensing performance. Yu et al.90 proposed a two-step method to prepare In/Ni MOF-derived mesoporous In2O3–NiO composites with a nanosheet hollow spherical (NHS) structure. They observed that mesoporous In2O3–NiO NHS has a high porosity, and this advantage provides sufficient permeation pathways for VOC, a large number of active sites, and the capacity to capture VOC.
3.1.4 Surface density.
Surface density is a key factor as increasing the thickness of the nano-catalyst coating can increase the surface area of the catalyst and reduce competitive adsorption between reactants, thereby increasing the removal rate and the degree of mineralization of the catalyst.94
Singh et al.95 used the atomic layer deposition method for coating TiO2 on fibrous nanosilica (KCC-1). They observed that the KCC-1/TiO2 catalyst coated with TiO2 NPs has a more uniform coating, a higher loading of TiO2, a smaller loss of surface area, and higher active site accessibility than traditional silica catalysts. Wang et al.96 confined the dense Au nanoparticles to a bowl-shaped TiO2 nanoarray doped with N. By adjusting the absorption of light by TiO2 and fully overlapping the plasma band of Au NPs, the photocatalytic efficiency is greatly improved. Roldan et al.97 researched a new type of nanostructured coating system, which includes a layer of SiO2 and a layer of dense anatase TiO2 doped with Ag NPs. The photocatalytic activity has been improved. All in all, increasing the surface density of the nano-catalyst can greatly increase the conversion of VOCs. However, an excessive amount of catalyst will result in a decrease in catalytic efficiency.
3.1.5 Support material.
Fixing the nano photocatalyst on a suitable carrier material can reduce aggregation of NPs, thereby increasing the catalytic efficiency, adsorption capacity, and prolonging the effective life of the photocatalyst.62 In recent years, engineered carbon has been applied to catalyst support due to its high surface area, porous structure, and high-performance adsorption of VOCs.21 Activated carbon (AC) has been applied to the adsorption and recovery of most VOCs. However, AC has some shortcomings that affect its ability to adsorb VOCs: AC is an inherently non-polar adsorbent, which will hinder the adsorption of hydrophilic particles.22 Furthermore, the porous structure of activated carbon is microporous (pore size <2 nm), which makes it difficult for molecules with larger molecular diameters to enter the pores. Furthermore, due to strong diffusion resistance due to irregular pore structure, the adsorption equilibrium is prolonged due to the disordered pores possessed by AC. Zhang et al.98 prepared a new nano-β-FeOOH/Fe3O4/biochar composite material. Through XPS characterization, it is proved that there are Fe–OC bonds between β-FeOOH and biochar. These bonds facilitated the transfer of photo-generated electrons. The connection promotes the rapid interface transfer of light energy electrons between biochar and β-FeOOH. Zhang et al.99 prepared N-doped nano-TiO2-carbon fiber composite material. After TiO2 NPs are irradiated by microwaves, they generate a large number of hydroxyl adsorption sites. Due to the interface formed between TiO2 and carbon fiber, this carbon fiber composite material can effectively catalyze the oxidation reaction of phenol. Graphene has the characteristics of inhibiting the annihilation of electrons and holes and exhibits excellent photoactivity, and so it is widely used in photocatalysis.100–102 Noor et al.103 used graphene oxide (GO) as the support material and randomly dispersed TiO2 and NiO NPS on it. The GO provides fast electronic conductivity and strong oxidation characteristics, which facilitate the separation of carriers.
As can be seen, the support material of the nano-catalyst will affect the removal of VOCs. In short, a suitable carrier can effectively increase the accessible surface area, mechanical strength, and stability of the nano-catalyst.
3.2 Effects of environmental conditions (external factors) on POC of VOCs
The multi-factor synergistic mechanism of photocatalytic degradation of VOCs by adsorption is controlled by two aspects: thermodynamics and dynamics. Considering the complexity of the environment, we briefly review the effects of humidity, airflow rate, light irradiation, concentration of pollutants, and temperature on the photocatalytic degradation of VOCs (Fig. 4). The factors influencing the comprehensive adsorption and photocatalysis capability of modified NMs are discussed in Table 2.
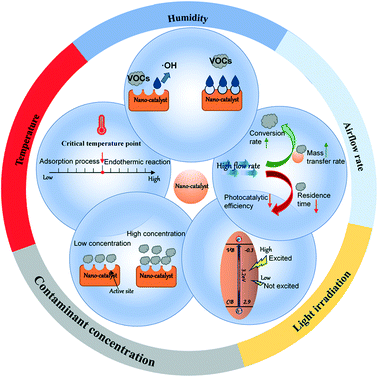 |
| Fig. 4 Illustration of external factors and mechanism of action. | |
Table 2 The key factors affecting VOC photocatalytic performances
Factors |
VOCs |
Humidity |
Temperature |
Airflow rate |
Light |
Inlet concentration |
Removal effificiency |
Ref. |
Humidity |
MEK |
0% |
23 ± 1 °C |
0.015 m3 min−1 |
UV |
2.65 ± 0.3 mg m−3 |
41% |
123
|
20% |
46% |
40% |
44% |
60 ± 1% |
41% |
2-Ethyl-1-hexanol |
20% |
— |
— |
Visible light |
0.1 ppm |
89% |
124
|
50% |
85% |
80% |
70% |
Temperature |
Toluene |
— |
155–160 °C |
30 000 mL g−1 h−1 |
650 mW cm−2 |
200 ppm |
40–50% |
125
|
220–230 °C |
90–95% |
Toluene |
— |
130 °C |
40 000 mL g−1 h−1 |
— |
— |
18–20% |
126
|
180 °C |
57–60% |
Airflow rate |
Acetaldehyde |
20 ± 1% |
25 °C |
20 L min−1 |
UV lamps |
15 ppm |
35–40% |
127
|
40 L min−1 |
20% |
Light |
MEK |
0% |
23 ± 1 °C |
0.015 m3 min−1 |
UV |
2.65 ± 0.3 mg m−3 |
60–70% |
123
|
24 ± 2 °C |
Visible light |
40–50% |
1-Propanol |
10% |
30 °C |
320 mL min−1 |
1.0 mW cm−2 |
400 ppm |
45% |
110
|
2.0 mW cm−2 |
55% |
3.0 mW cm−2 |
65% |
Inlet concentration |
Toluene |
— |
— |
— |
Visible light |
115 ppm |
100% |
128
|
230 ppm |
100% |
460 ppm |
87.1% |
690 ppm |
65.5% |
3.2.1 Relative humidity and temperature.
Water vapor plays a double-sided role in the process of photocatalysis of VOCs and their adsorption onto/interactions with modified NMs.104,105 As a polar molecule, water can provide hydroxyl radicals, which is conducive to the adsorption of more hydrophilic VOCs molecules onto the photocatalyst surface through hydrogen bonds. However, if the moisture content is too high, it will compete with VOCs for the adsorption sites. For some pollutants, the presence of moisture can promote the mineralization of pollutants, but excessive moisture will be adsorbed onto the active sites of the catalyst, thereby reducing the catalytic efficiency of the catalyst.106 Regarding air humidity, modification of the nano-catalyst can reduce the degree of competition between water molecules and VOCs; for example, doped TiO2 has a higher catalytic efficiency than undoped TiO2 because of the introduction of dopants resulting in more oxidant.107–111
Temperature is another key factor affecting photocatalysis. In the adsorption process of VOCs onto the photocatalytic material, low temperature is conducive to adsorption processes dominated by exothermic reactions, but it reduces the diffusion rate of adsorbate molecules. Huang et al.112 pointed out that the efficiency of the PCO of formaldehyde is higher at 60 °C than at 30 °C. This phenomenon shows that a higher temperature promotes the photocatalytic process. At lower temperatures, the VOC adsorption process is dominant, and the rate is higher than the photocatalytic oxidation rate.113
3.2.2 Airflow rate and contaminant concentration.
The airflow rate affects the photocatalytic oxidation process of VOCs and similar to temperature brings advantages and disadvantages. Increasing the airflow rate will increase the transfer rate of VOCs and increase the conversion rate of pollutants. On the contrary, too high an airflow rate will reduce the residence time of VOCs and thus reduce the photodegradation efficiency.114–116 Therefore, optimizing the airflow rate is essential: a low airflow rate can increase the residence time of VOCs so that they can be fully adsorbed on the catalyst surface. Under high flow rates, the residence time is reduced so the removal rate will be reduced. Thus, the flow rate should be optimized for each catalyst–VOC pair to determine the optimal flow rate and maximize the PCO efficiency.
The adsorption capacity of the catalyst is related to the number of active sites. When the concentration of VOCs is low (located in the appropriate range) and the adsorption capacity can meet the adsorption demand, the removal efficiency will be improved. Since the by-products produced by the catalysis compete for adsorption sites, PCO is more suitable for the degradation of low concentration pollutants.117,118 An area for future development is a means to remove the by-products from the adsorption sites or to ensure they have a lower binding affinity for the sites than the target VOC pollutants.
3.2.3 Light irradiation.
It is worth mentioning that light irradiation (wavelength and intensity of light) has a greater impact on the photocatalytic process than the adsorption process. On the one hand, the wavelength of light is related to Eg and the energy of the band gap photon. If Eg is too low (less than the energy band of the catalyst), the electrons will not be excited and the oxidation process of VOCs on the catalyst surface is difficult to occur. On the other hand, under low light intensity conditions, the light intensity is related to the photocatalytic rate, and as the light intensity increases, the rate and light intensity shows a power-law relationship.119 However, in PCO, the energy loss caused by light reflection and transmission is inevitable. Therefore, researchers have used modification methods such as doping, use of semiconductors, and surface modification to use energy as much as possible.106,120–122
4. Surface modification of nano-catalysts as a means to increase PCO efficiency for VOCs
The intrinsic properties of nanomaterial photocatalysts and the extrinsic factors affecting nano-catalysts have a significant role in photocatalytic efficiency. Surface modification methods investigated to date include surface doping, the structure of surface heterojunctions, utilizing a supported co-catalyst, increasing the surface area, and ensuring high reaction surface exposure (Fig. 5). These are discussed below.
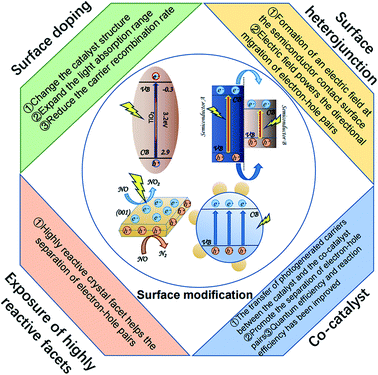 |
| Fig. 5 Schematic illustration of different surface modification methods and effect on photocatalytic efficiency. | |
4.1 Surface doping
Surface doping can introduce electrons into the band gap of the semiconductor, causing an optical response, which in turn produces a significant redshift. This two-step light excitation process excited by low-energy visible photons promotes the visible light activity of the semiconductor.129 By doping with metal elements, Fang et al.130 found that surface doping significantly improves the catalytic efficiency of the catalyst for refractory benzene. However, the lattice defects caused by doping can not only serve as the transfer medium of the interfacial charge but also become the complex center of electron–hole pairs, reducing the catalytic activity.129,131 In addition to a single doped metal or non-metal element, co-doping between metal ions, non-metal elements, or between metal ions and non-metal elements can also effectively extend the wavelength of the photocatalyst excitation light.
4.1.1 Metal doping.
At present, the research on doping of metal elements mainly includes noble metals, transition metals, and rare earth metals. However, noble metals cannot be widely used in practice due to their high cost and scarcity of raw materials. Exploring the effects of different types of metal doping on photoactivity, optimal doping dose, and preparing nano-catalysts with the best benefits and efficiency have become the focus of current research (Fig. 6).
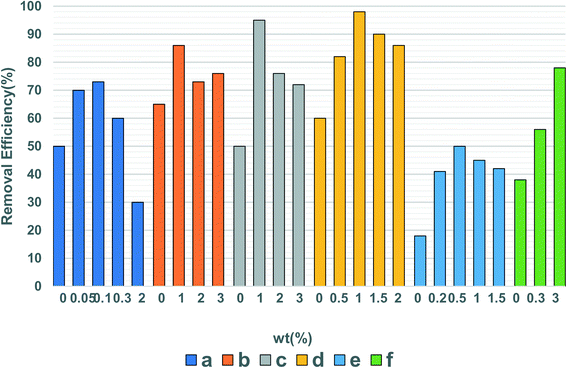 |
| Fig. 6 The effect of different doping amounts on the degradation of VOCs by photocatalysts. (a) Acetaminophen, Sb-doped TiO2.157 (b) 4-Chlorophenol, W-doped TiO2.158 (c) 4-Chlorophenol, Mo-doped TiO2.158 (d) 2,4-Dichlorophenol, Ce-doped CuMgAl.159 (e) MEK, Ce–TiO2.160 (f) Acetaldehyde, Cr–TiO2.161 | |
Generally, the preparation method of the doped catalyst will produce different crystal properties and change the morphology of the photocatalyst. The mechanism behind metal doping can be summarized as follows: (1) noble metals have anti-oxidation and corrosion resistance properties even in humid air. Under the action of noble metal NPs, the recombination of carriers is reduced, which increases the photoactivity on the surface of the photocatalyst. (2) The type and doping amount of transition metals are the two key factors that affect the PCO. If the doping amount is at the optimal value, the dopant can accelerate the separation of carriers. When the optimal value is exceeded, the dopant may become a recombination center, reducing the photocatalytic efficiency. (3) Rare earth metals have incomplete 4f and empty 5d orbitals, which can promote photocatalytic reactions.
Noble metal elements such as platinum (Pt),132 palladium (Pd),133 ruthenium (Ru),134 silver (Ag) have been extensively studied.135 Because of noble metals, the recombination of carriers is reduced, which improves the photoactivity of the catalyst.136 In fact, the doping of noble metal NPs forms a medium for capturing and transferring electrons on the nano-catalyst surface.137 Meng et al.138 doped Pd/PdCl2 onto the surface of the nano-catalyst Bi2WO6 by photoreduction method. Compared with TiO2, the catalyst degrades phenol more efficiently. The researchers concluded that it may be due to the dual factors of the plasmon resonance and the suppression of photo-generated carrier recombination. Xue et al.,139 modified TiO2 doped with Ag and Ag2O. The efficiency of this catalyst to degrade toluene is 50%, which is about 9.7 times higher than TiO2.
Transition metal doping can significantly extend visible light excitation and is more susceptible to doping by other transition metals because of the lower energy required for the substitution process. Thus, there has been extensive research on transition metal doping, such as manganese (Mn),140,141 iron (Fe),142–144 copper (Cu),145 vanadium (V),146,147 and nickel (Ni).148 Afif et al.149 successfully synthesized a highly active Mn-doped Ag3PO4 photocatalyst using the co-precipitation method. Mn doping suppressed hydroxyl defects and oxygen vacancies, increased the atomic ratio of oxygen to silver, and improved the photocatalytic performance under visible light irradiation. Herr et al.150 found that the photochemical properties of the Mn complex reached or approached the performance of Ru and Ir noble metal catalysts in terms of photon absorption. Devaraji group151 incorporated V into the TiO2 crystal lattice to make Ti0.98V0.02O2. Compared with pure TiO2, this catalyst embodies the quantum transition of benzene oxidation, highlighting the importance of V doping for benzene oxidation. Stucchi et al.152 used Mn to replace noble metals such as Au and Ag. Through experiments, it was found that for TiO2 doped with 20% Mn under visible light exposure for 24 h, the degradation efficiency of ethanol reached 35%, which is the peak degradation efficiency. The suppression of defect sites on the catalyst surface and the reduction of electrons of the compound with holes may be the reasons for the excellent photocatalytic activity. Li et al.153 prepared Co-doped TiO2 nanorod array (Co–TiO2@Ti(H2)) with good stability, and the energy barrier for desorption can be effectively reduced by introducing Co with abundant oxygen vacancies. Sajjad et al.154 used Si and Ti to modify magnetic Fe3O4 NPs. It was found that the photodegradation effect was in the order of Ti modified Fe3O4 > Si modified Fe3O4 > Fe3O4.
There are 17 kinds of lanthanides, collectively referred to as rare earth metals. Kumar et al.39 found that lanthanide ion dopants are beneficial to the optical properties of the ZnO structure. Parameters such as material properties and pollutant degradation reaction conditions influence the performance of ZnO. Xiao et al.155 found that Ce-doped TiO2 shows the advantages of stability and higher surface area. Notably, the adsorption capacity of VOCs is also greatly enhanced. The same result also appeared in other experiments. Wang et al.156 synthesized Ce-doped MoS2 nanocomposite by hydrothermal method. Under visible light irradiation, it exhibits excellent photocatalytic activity.
It is worth noting that different cationic dopants have individual effects on the nano-catalyst. Generally speaking, metal doping will produce different properties and also affect the morphology of the nano-catalyst. The lattice defects caused by doping may become the recombination center of carriers, thereby reducing the catalytic activity. Therefore, searching for an optimal amount of doping is still the focus of future work.
4.1.2 Non-metal doping.
Non-metal doping such as nitrogen (N),162–166 carbon (C),167–169 sulfur (S),170–173 boron (B)174,175and fluorine (F)176 has been extensively evaluated previously. In non-metal doping, dopants can change the morphology and improve the photoactive performance of the catalyst. Because the doped state is close to the edge of the VB and is not used as a carrier, the role of the recombination center will be weakened. When the oxygen atom is replaced by other non-metal element atoms, the top energy level of the VB of the oxide will increase, and the semiconductor band gap will be narrowed, thereby extending the excitation wavelength to improve catalytic efficiency.
Table 3 summarizes the synthesized metal and non-metal doped photocatalysts to improve photocatalytic degradation performance. A conclusion can be drawn that after doping, the catalytic efficiency of the catalyst has been improved.
Table 3 Summary of metal and non-metal doped photocatalysts
Contaminant |
Photocatalyst |
Dopant |
Efficiency before doping |
Efficiency after doping |
Ref. |
Toluene |
TiO2 |
Ag/Ag2O |
7.5% |
23.3% |
139
|
Benzene |
OMS-2 |
Mg |
68.4% |
97.2% |
130
|
Benzene |
TiO2 |
V |
0.3% |
12.7% |
151
|
Benzene |
Ti0.98V0.02O2 |
Au |
9% |
18% |
151
|
Acetaldehyde |
TiO2 |
F |
77.3% |
81% |
177
|
Acetaldehyde |
TiO2 |
N |
77.3% |
92.1% |
177
|
Ethylbenzene |
TiO2 |
N |
33% |
38% |
178
|
Awin et al.179 pointed out that the N-doped TiO2 on the Si–OCN support exhibited excellent adsorption properties and high catalytic activity under visible light. Sun et al.180 developed C-doped and oxygen vacancy-rich Bi2WO6 nanospheres mediated by graphene oxide. C-Doping can change the band gap structure and can also promote light absorption. This is because carbon doping in the catalyst acts as an acceptor and electron channel to promote the separation of carriers and the production of active substances. Diao et al.181 synthesized F-doped TiO2 by hydrothermal method and used EPR measurements to prove that F-doped TiO2 has superior degradability to formaldehyde due to the participation of superoxide radical and hydroxyl radical in the process of oxidizing formaldehyde into CO2 and H2O. Ramacharyulu et al.182 noted that compared with undoped TiO2, S-doped TiO2 had a lower band gap value and better photocatalytic activity. Among various doping materials, non-metallic element doping has been tested to be a better way to improve PCO activity.183,184
4.2 Structure of surface heterojunction
When two semiconductors with similar characteristics are in contact, an electric field is formed at the contact interface. The electric field provides the driving force for the directional migration of electron–hole pairs between different semiconductors, which can promote effective separation. This promotes the oxidation–reduction reaction of the nano-catalyst, which in turn facilitates the degradation of VOC. In heterojunction photocatalysis, photogenerated electrons generally migrate from a semiconductor with a higher CB energy level, and a photogenerated hole will migrate from a semiconductor with a lower VB energy level. The mechanism of surface heterojunction is that a well-defined junction can effectively promote charge transfer and hinder the recombination of electrons and holes. Thus, nano-catalysts show high activity and stability.
Table 4 shows the surface heterojunction effect on photoactivity. Obviously, the formation of the heterojunction promotes the degradation efficiency of pollutants.
Table 4 Heterostructure and degradation efficiency of photocatalysts
Heterostructure |
Photocatalyst |
Efficiency before |
Efficiency after |
Ref. |
Z-Scheme |
LaFeO3/g-C3N4 |
37% |
100% |
185
|
Z-Scheme |
Au–TiO2@NH2-UiO-66 |
10% |
85% |
186
|
S-Scheme |
Cu2S/SnO2 |
17.9% |
67.2% |
187
|
Dai et al.188 used a new hydroxylation method to coat BiOI on the TiO2 wall to form the p–n junction of the BiOI/TiO2 nanotube array (Fig. 7A). They found that the photo-electrocatalytic degradation efficiency of BiOI/TiO2 was increased by 3 times. Guo et al.189 successfully prepared an Ag/Ag2O/PbBiO2Br photocatalyst with a broader spectral response through a series of plasma p–sn heterojunctions. Researchers have observed significantly accelerated charge separation, and the degradation efficiency of pollutants has also been significantly improved (Fig. 7B). Huang et al.190 developed the p–n junction BiOI@Bi12O17Cl2 heterostructure by depositing BiOI nanosheets in situ. Due to charge induction, BiOI@Bi12O17Cl2 forms a unique front-side coupling heterostructure. Compared with the pure sample, the obtained BiOI@Bi12O17Cl2 heterostructure can significantly enhance the catalytic performance and degradation of 2,4-dichlorophenol. Iqbal et al.191 studied a new type heterojunction of Al2O3 and GO. FTIR examination showed that the density of hydroxyl on the surface of pure Al2O3 was lower, but after adding GO, the density increased (Fig. 7C). The reason may be related to the interaction between hydroxyl and light-generated holes, which promotes electron transfer and inhibits the recombination of carriers. Because of GO, the recombination of electron–hole pairs is reduced. Through the study of activity, it was found that 15.0% GO/Al2O3 exhibits superior photocatalytic performance. In another study, the same result was observed.192 Wu et al.185 constructed new p-type LaFeO3 microspheres coated with n-type nano-scale graphite carbon nitride nanosheets. The interface effect of charge carriers is separated and transferred more effectively through the solid p–n heterojunction. Yao et al.193 prepared p–n heterojunction of Bi2MoO6/BiOBr, which can promote photocatalysis. In addition, the UV-vis absorption edge of the BMOBB-2 (the mole ratio of Na2MoO4·2H2O to Bi (NO3)3·5H2O is set as 5%) sample has a significant red shift, which is related to the better visible light response of Bi2MoO6 (Fig. 7D). Due to the strong interaction between BiOBr and Bi2MoO6, the binding energy changes in the XPS spectrum. It can be seen that there are carrier transfer and chemical bonds at the heterojunction interface between BiOBr and Bi2MoO6 (Fig. 7E). Another study concluded that the Z-type heterojunction is the main reason for improving the photocatalytic performance of Ag3PO4/Ag/MoS2/TiO2 composites.194 Wang et al.195 developed an electrochemically self-doped WO3/TiO2 nanotube composite film by doping oxygen vacancies into heterojunctions for photocatalytic degradation of exhaust gas. Ding et al.196 synthesized a CoO@TiO2/MXene hybrid with a double heterojunction structure. EPR measurements prove that SO4˙−, ˙O2−, and 1O2 are the main reactive species involved in the photocatalytic degradation of phenol (Fig. 8).
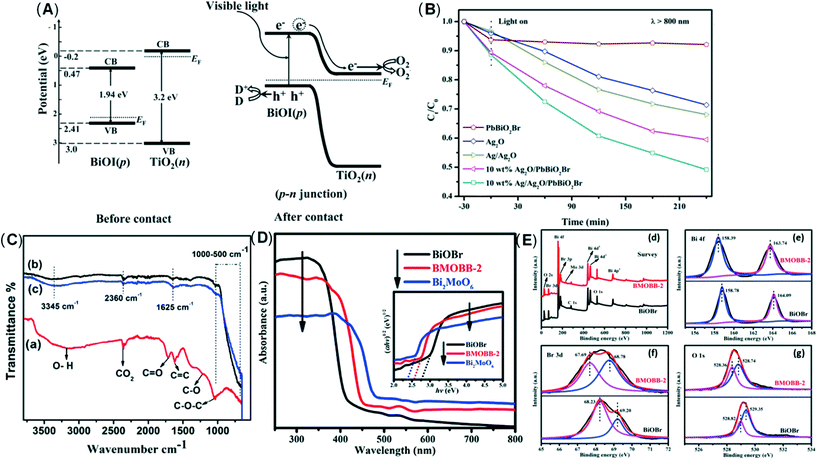 |
| Fig. 7 (A) Schematic diagrams of the energy bands of p-BiOI and n-TiO2 before light irradiation and formation of a p–n junction under visible light irradiation. Adapted with permission from ref. 188 Copyright 2011, American Chemical Society. (B) Photocatalytic degradation of tetracycline with obtained samples under NIR light (λ > 800 nm). Adapted with permission from ref. 189 Copyright 2019, Elsevier. (C) FTIR spectra of various samples; (a) pure GO (b) pure γ-Al2O3 (c) 10.0% GO/Al2O3 composite. Adapted with permission from ref. 191 Copyright 2018, American Chemical Society. (D) UV-vis diffuse reflectance spectra (DRS). Adapted with permission from ref. 193 Copyright 2021, Elsevier. (E) (d) XPS survey spectra and high resolution XPS spectra of (e) Bi 4f, (f) Br 3d, (g) O 1s of BiOBr and BMOBB-2. Adapted with permission from ref. 193 Copyright 2021, Elsevier. | |
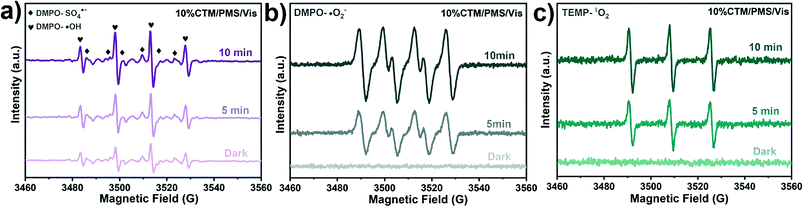 |
| Fig. 8 EPR spectra of 10% CTM/PMS/vis system for (a) 5,5-dimethyl-1-pyrroline N-oxide (DMPO)–˙OH and DMPO–SO4˙−, (b) DMPO–O2˙− and (c) TEMP–1O2. Adapted with permission from ref. 196 Copyright 2021, Elsevier. | |
4.3 Supported co-catalyst
The electron–hole transfer between the co-catalyst and semiconductor not only accelerates the separation of carriers but also realizes the spatial separation of oxidation and reduction reactions so that both quantum efficiency and reaction efficiency are improved. In addition, the co-catalyst also has abundant surface active sites, which can cut back the overpotential of the surface reaction, thereby increasing the surface reaction rate.
Wang et al.197 found that WSe2/g-C3N4 prepared with WSe2 as a co-catalyst promotes both light absorption and improves charge transfer efficiency. Peng et al.161 by comparing the amount of co-catalyst found that the CrxO co-catalyst (3 wt%) is beneficial to improve the removal efficiency of acetaldehyde. Shen et al.198 used the organic molecule oxamide (OA) as a co-catalyst to prepare modified TiO2 samples through wet chemical methods to enhance electron–hole separation and photocatalytic H-2 precipitation on TiO2. Bai et al.199 found that MoS2 as a TiO2 co-catalyst has the following characteristics: (1) No noble metals; (2) high charge transport mobility; (3) many active sites.
4.4 Exposure of highly reactive facets
Crystals have different optical and electronic structures, so the crystals have unique properties, such as adsorption, high activity, and selectivity. The crystal facet can also promote the separation of electrons and holes. Furthermore, the reactivity physical and chemical properties of surface facets are also critical to determine its workability.200
Liang et al.201 prepared a high proportion of active (002) crystal planes (>90%) and high specific surface area ultra-thin WO3 nanosheets, which improved the performance of the catalyst to degrade pollutants. Yu et al.202 synthesized TiO2 nanosheets. The exposed (001) crystal facets are beneficial for the reduction of NOx. The NO conversion rate of the TiO2 sheets prepared by the hydrothermal method is higher than the conversion rate of commercial P25 and TiO2 particles synthesized by the sol–gel method. Li et al.203 prepared Z-scheme rGO/Bi2S3–BiOBr heterojunction, which has an adjustable exposed BiOBr (102) crystal facet. The optimized catalyst has the best photocatalytic oxidation performance in a single system, and the degradation efficiency of 2-nitrophenol reaches 92%. In different photocatalytic applications, the crystal facets promote the separation of carriers, exposing the highly reactive facets to improve the activity of the catalyst and has become a promising method.
5. Summary and outlook
Rapid economic development has posed serious environmental and health problems due to VOCs. They come from a wide range of sources and can cause diseases and even carcinogenesis in the human body. In addition, under light exposure, VOCs generate photochemical smog, and certain halogenated hydrocarbons can cause the destruction of the ozone layer. To date, photocatalysis is being recognized as an effective and clean treatment method for VOC removal as it operates at room temperature, produces no secondary pollution, and has high removal activity. Furthermore, the photocatalytic efficiency has been greatly improved by surface modification of the nano-catalysts.
In this work, we reviewed the influencing mechanism of the intrinsic and extrinsic factors of nano-catalysts on the catalytic degradation of VOCs. In addition, four nano-catalyst surface modification strategies are also discussed: surface doping, surface heterojunction, co-catalyst, and exposure of highly reactive crystal facets, which have been analyzed and evaluated.
From what was discussed, the following conclusions can be drawn: (1) by understanding the basic principles of photocatalysis, it was found that surface modification of the photocatalyst can reduce the recombination of the carrier and improve the photoactivity of the nano-catalyst. (2) The morphology of the catalyst affects the adsorption of VOCs, and the high surface area and porous structure are conducive to the adsorption of VOCs. (3) Temperature and humidity will seriously affect the adsorption of VOC. Low temperature is conducive to adsorption processes dominated by exothermic reactions. High humidity will reduce the adsorption capacity of VOCs. However, photocatalysis also has shortcomings: (1) the photocatalysis is limited to the treatment of low concentrations of pollutants. (2) The performance of the photocatalyst is affected by internal and external factors. (3) The lattice defects caused by doping can reduce the catalytic activity. Therefore, there is often an optimal amount of doping.
Based on our current knowledge about the limitations of PCO technology in removing VOCs, we can make some suggestions for future research (Fig. 9): (1) improve the electronic and chemical properties of the nano-catalyst to improve its photocatalytic activity, adsorption of VOCs, and resistance to deactivation. (2) Explore more stable and more efficient photocatalyst materials, combining different strategies such as facets, heterojunctions, and co-catalysts. (3) Photocatalysts with the visible light response show enormous promise and should be widely researched. (4) More attention should be paid to the development of synthesis methods that contribute to electron trapping mechanisms, efficient structures, and production methods. (5) Increase the rate of adsorption and reduce the competitive adsorption behavior of by-products.
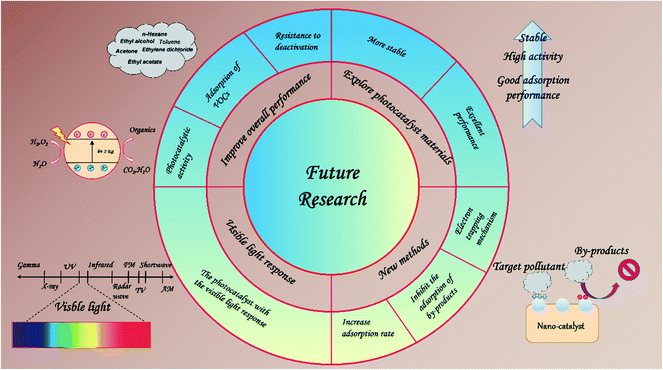 |
| Fig. 9 Prospects for future research. | |
We hope that the presented overview can provide key research progress in the field of photocatalysis of modified NMs, and expect to make greater progress in the design of nano-catalysts in the near future.
Author contributions
Weichen Zhao: writing – original draft, visualization, conceptualization. Muhammad Adeel: writing – review & editing, conceptualization. Peng Zhang, Pingfan Zhou, Lili Huang, Yongwen Zhao, Muhammad Arslan Ahmad, Noman Shakoor, Benzhen Lou, Yaqi Jiang, Iseult Lynch: writing – review & editing. Yukui Rui: writing – review & editing, supervision.
Conflicts of interest
The authors declare that they have no known competing financial interests or personal relationships that could have appeared to influence the work reported in this paper.
Acknowledgements
The project was supported by the National Key R&D Program of China (2017YFD0801300, 2017YFD0801103), National Natural Science Foundation of China (No. 41130526), Professor workstation in Yuhuangmiao Town, Shanghe County, China Agricultural University and Professor workstation in Sunji Town, Shanghe County, China Agricultural University.
References
- P. Amoatey, H. Omidvarborna, M. S. Baawain and A. Al-Mamun, Emissions and exposure assessments of SOX, NOX, PM10/2.5 and trace metals from oil industries: A review study (2000-2018), Process Saf. Environ. Prot., 2019, 123, 215–228 CrossRef CAS.
- B. B. Huang, C. Lei, C. H. Wei and G. M. Zeng, Chlorinated volatile organic compounds (Cl-VOCs) in environment - sources, potential human health impacts, and current remediation technologies, Environ. Int., 2014, 71, 118–138 CrossRef CAS PubMed.
- S. B. Ge, N. L. Ma, S. C. Jiang, Y. S. Ok, S. S. Lam, C. Li, S. Q. Shi, X. Nie, Y. Qiu, D. L. Li, Q. D. Wu, D. C. W. Tsang, W. X. Peng and C. Sonne, Processed Bamboo as a Novel Formaldehyde-Free High-Performance Furniture Biocomposite, ACS Appl. Mater. Interfaces, 2020, 12, 30824–30832 CrossRef CAS PubMed.
- H. K. Yuan, J. Ren, X. H. Ma and Z. L. Xu, Dehydration of ethyl acetate aqueous solution by pervaporation using PVA/PAN hollow fiber composite membrane, Desalination, 2011, 280, 252–258 CrossRef CAS.
- P. I. Beamer, C. E. Luik, L. Abrell, S. Campos, M. E. Martinez and A. E. Saez, Concentration of Trichloroethylene in Breast Milk and Household Water from Nogales, Arizona, Environ. Sci. Technol., 2012, 46, 9055–9061 CrossRef CAS PubMed.
- R. Baran, I. I. Kaminska, A. Śrębowata and S. Dzwigaj, Selective hydrodechlorination of 1,2-dichloroethane on NiSiBEA zeolite catalyst: Influence of the preparation procedure on a high dispersion of Ni centers, Microporous Mesoporous Mater., 2013, 169, 120–127 CrossRef CAS.
- Y. C. Chien, Variations in amounts and potential sources of volatile organic chemicals in new cars, Sci. Total Environ., 2007, 382, 228–239 CrossRef CAS PubMed.
- M. R. Gwinn, D. O. Johns, T. F. Bateson and K. Z. Guyton, A review of the genotoxicity of 1,2-dichloroethane (EDC), Mutat. Res., Rev. Mutat. Res., 2011, 727, 42–53 CrossRef CAS PubMed.
- B. C. McDonald, J. A. de Gouw, J. B. Gilman, S. H. Jathar, A. Akherati, C. D. Cappa, J. L. Jimenez, J. Lee-Taylor, P. L. Hayes, S. A. McKeen, Y. Y. Cui, S.-W. Kim, D. R. Gentner, G. Isaacman-VanWertz, A. H. Goldstein, R. A. Harley, G. J. Frost, J. M. Roberts, T. B. Ryerson and M. Trainer, Volatile chemical products emerging as largest petrochemical source of urban organic emissions, Science, 2018, 359, 760–764 CrossRef CAS PubMed.
- Z. M. Zhong, Q. E. Sha, J. Y. Zheng, Z. B. Yuan, Z. J. Gao, J. M. Ou, Z. Y. Zheng, C. Li and Z. J. Huang, Sector-based VOCs emission factors and source profiles for the surface coating industry in the Pearl River Delta region of China, Sci. Total Environ., 2017, 583, 19–28 CrossRef CAS PubMed.
- J. L. Jiang, X. S. Ding, A. Tasoglou, H. Huber, A. D. Shah, N. Jung and B. E. Boor, Real-Time Measurements of Botanical Disinfectant Emissions, Transformations, and Multiphase Inhalation Exposures in Buildings, Environ. Sci. Technol. Lett., 2021, 8, 558–566 CrossRef CAS.
- X. H. Zhang, Q. L. Liu, Y. Xiong, A. M. Zhu, Y. Chen and Q. G. Zhang, Pervaporation dehydration of ethyl acetate/ethanol/water azeotrope using chitosan/poly (vinyl pyrrolidone) blend membranes, J. Membr. Sci., 2009, 327, 274–280 CrossRef CAS.
- R. J. Keith, J. L. Fetterman, O. A. Orimoloye, Z. Dardari, P. K. Lorkiewicz, N. M. Hamburg, A. P. DeFilippis, M. J. Blaha and A. Bhatnagar, Characterization of Volatile Organic Compound Metabolites in Cigarette Smokers, Electronic Nicotine Device Users, Dual Users, and Nonusers of Tobacco, Nicotine Tob. Res., 2020, 22, 264–272 CrossRef PubMed.
- Z. Zhang, Z. Jiang and W. Shangguan, Low-temperature catalysis for VOCs removal in technology and application: A state-of-the-art review, Catal. Today, 2016, 264, 270–278 CrossRef CAS.
- C. He, J. Cheng, X. Zhang, M. Douthwaite, S. Pattisson and Z. Hao, Recent Advances in the Catalytic Oxidation of Volatile Organic Compounds: A Review Based on Pollutant Sorts and Sources, Chem. Rev., 2019, 119, 4471–4568 CrossRef CAS PubMed.
- U. Poschl and M. Shiraiwa, Multiphase Chemistry at the Atmosphere-Biosphere Interface Influencing Climate and Public Health in the Anthropocene, Chem. Rev., 2015, 115, 4440–4475 CrossRef PubMed.
- C. P. Weisel, Assessing exposure to air toxics relative to asthma, Environ. Health Perspect., 2002, 110, 527–537 CrossRef CAS PubMed.
- S. Weichenthal, R. Kulka, P. Belisle, L. Joseph, A. Dubeau, C. Martin, D. Wang and R. Dales, Personal exposure to specific volatile organic compounds and acute changes in lung function and heart rate variability among urban cyclists, Environ. Res., 2012, 118, 118–123 CrossRef CAS PubMed.
- R. Xiao, J. Mo, Y. Zhang and D. Gao, An in-situ thermally regenerated air purifier for indoor formaldehyde removal, Indoor Air, 2018, 28, 266–275 CrossRef CAS PubMed.
- K. Rumchev, J. Spickett, M. Bulsara, M. Phillips and S. Stick, Association of domestic exposure to volatile organic compounds with asthma in young children, Thorax, 2004, 59, 746–751 CrossRef CAS PubMed.
- X. Zhang, B. Gao, A. E. Creamer, C. Cao and Y. Li, Adsorption of VOCs onto engineered carbon materials: A review, J. Hazard. Mater., 2017, 338, 102–123 CrossRef CAS PubMed.
- W. X. Zou, B. Gao, Y. S. Ok and L. Dong, Integrated adsorption and photocatalytic degradation of volatile organic compounds (VOCs) using carbon-based nanocomposites: A critical review, Chemosphere, 2019, 218, 845–859 CrossRef CAS PubMed.
- U. I. Gaya and A. H. Abdullah, Heterogeneous photocatalytic degradation of organic contaminants over titanium dioxide: A review of fundamentals, progress and problems, J. Photochem. Photobiol., C, 2008, 9, 1–12 CrossRef CAS.
- M. Tahir, S. Tasleem and B. Tahir, Recent development in band engineering of binary semiconductor materials for solar driven photocatalytic hydrogen production, Int. J. Hydrogen Energy, 2020, 45, 15985–16038 CrossRef CAS.
- T. K. Tseng, Y. S. Lin, Y. J. Chen and H. Chu, A Review of Photocatalysts Prepared by Sol-Gel Method for VOCs Removal, Int. J. Mol. Sci., 2010, 11, 2336–2361 CrossRef CAS PubMed.
- M. Adeel, N. Shakoor, T. Hussain, I. Azeem, P. Zhou, P. Zhang, Y. Hao, J. Rinklebe and Y. Rui, Bio-interaction of nano and bulk lanthanum and ytterbium oxides in soil system: Biochemical, genetic, and histopathological effects on Eisenia fetida, J. Hazard. Mater., 2021, 415, 125574 CrossRef CAS PubMed.
- R. K. Huang, C. Y. Cao, J. Liu, L. R. Zheng, Q. H. Zhang, L. Gu, L. Jiang and W. G. Song, Integration of Metal Single Atoms on Hierarchical Porous Nitrogen-Doped Carbon for Highly Efficient Hydrogenation of Large-Sized Molecules in the Pharmaceutical Industry, ACS Appl. Mater. Interfaces, 2020, 12, 17651–17658 CrossRef CAS PubMed.
- Y. L. Zhao, Y. L. Song, W. G. Song, W. Liang, X. Y. Jiang, Z. Y. Tang, H. X. Xu, Z. X. Wei, Y. Q. Liu, M. H. Liu, L. Jiang, X. H. Bao, L. J. Wan and C. L. Bai, Progress of nanoscience in China, Front. Phys., 2014, 9, 257–288 CrossRef.
- M. M. Rui, C. X. Ma, J. C. White, Y. Hao, Y. Y. Wang, X. L. Tang, J. Yang, F. P. Jiang, A. Ali, Y. K. Rui, W. D. Cao, G. C. Chen and B. S. Xing, Metal oxide nanoparticles alter peanut (Arachis hypogaea L.) physiological response and reduce nutritional quality: a life cycle study, Environ. Sci.: Nano, 2018, 5, 2088–2102 RSC.
- C. Santhosh, V. Velmurugan, G. Jacob, S. K. Jeong, A. N. Grace and A. Bhatnagar, Role of nanomaterials in water treatment applications: A review, Chem. Eng. J., 2016, 306, 1116–1137 CrossRef CAS.
- D. S. Selishchev, T. N. Filippov, M. N. Lyulyukin and D. V. Kozlov, Uranyl-modified TiO2 for complete photocatalytic oxidation of volatile organic compounds under UV and visible light, Chem. Eng. J., 2019, 370, 1440–1449 CrossRef CAS.
- X. Liu, J. Iocozzia, Y. Wang, X. Cui, Y. Chen, S. Zhao, Z. Li and Z. Lin, Noble metal-metal oxide nanohybrids with tailored nanostructures for efficient solar energy conversion, photocatalysis and environmental remediation, Energy Environ. Sci., 2017, 10, 402–434 RSC.
- Q. K. Shen, C. Y. Cao, R. K. Huang, L. Zhu, X. Zhou, Q. H. Zhang, L. Gu and W. G. Song, Single Chromium Atoms Supported on Titanium Dioxide Nanoparticles for Synergic Catalytic Methane Conversion under Mild Conditions, Angew. Chem., Int. Ed., 2020, 59, 1216–1219 CrossRef CAS PubMed.
- A. Suligoj, U. L. Stanger, A. Ristic, M. Mazaj, D. Verhovsek and N. N. Tusar, TiO2-SiO2 films from organic-free colloidal TiO2 anatase nanoparticles as photocatalyst for removal of volatile organic compounds from indoor air, Appl. Catal., B, 2016, 184, 119–131 CrossRef CAS.
- S. Suarez, I. Jansson, B. Ohtani and B. Sanchez, From titania nanoparticles to decahedral anatase particles: Photocatalytic activity of TiO2/zeolite hybrids for VOCs oxidation, Catal. Today, 2019, 326, 2–7 CrossRef CAS.
- C. Karthikeyan, P. Arunachalam, K. Ramachandran, A. M. Al-Mayouf and S. Karuppuchamy, Recent advances in semiconductor metal oxides with enhanced methods for solar photocatalytic applications, J. Alloys Compd., 2020, 828, 15 CrossRef.
- Z. Shayegan, C. S. Lee and F. Haghighat, TiO2 photocatalyst for removal of volatile organic compounds in gas phase - A review, Chem. Eng. J., 2018, 334, 2408–2439 CrossRef CAS.
- Z. J. Zhu, Q. Zhang, X. Xiao, F. Q. Sun, X. X. Zuo and J. M. Nan, Dual-iodine-doped BiOIO3: Bulk and surface co-modification for enhanced visible-light photocatalytic removal of bisphenol AF, Chem. Eng. J., 2021, 404, 13 Search PubMed.
- S. G. Kumar and R. Kavitha, Lanthanide ions doped ZnO based photocatalysts, Sep. Purif. Technol., 2021, 274, 33 Search PubMed.
- K. Li, S. Zhang, Y. Li, J. Fan and K. Lv, MXenes as noble-metal-alternative co-catalysts in photocatalysis, Chin. J. Catal., 2021, 42, 3–14 CrossRef CAS.
- M. Malakootian, A. Nasiri and M. A. Gharaghani, Photocatalytic degradation of ciprofloxacin antibiotic by TiO2 nanoparticles immobilized on a glass plate, Chem. Eng. Commun., 2020, 207, 56–72 CrossRef CAS.
- H. Zangeneh, A. A. L. Zinatizadeh, M. Habibi, M. Akia and M. H. Isa, Photocatalytic oxidation of organic dyes and pollutants in wastewater using different modified titanium dioxides: A comparative review, J. Ind. Eng. Chem., 2015, 26, 1–36 CrossRef CAS.
- Q. Guo, C. Y. Zhou, Z. B. Ma and X. M. Yang, Fundamentals of TiO2 Photocatalysis: Concepts, Mechanisms, and Challenges, Adv. Mater., 2019, 31, 26 Search PubMed.
- H. Yi, L. Qin, D. L. Huang, G. M. Zeng, C. Lai, X. G. Liu, B. S. Li, H. Wang, C. Y. Zhou, F. L. Huang, S. Y. Liu and X. Y. Guo, Nano-structured bismuth tungstate with controlled morphology: Fabrication, modification, environmental application and mechanism insight, Chem. Eng. J., 2019, 358, 480–496 CrossRef CAS.
- C. P. Xu, P. R. Anusuyadevi, C. Aymonier, R. Luque and S. Marre, Nanostructured materials for photocatalysis, Chem. Soc. Rev., 2019, 48, 3868–3902 RSC.
- B. S. Li, C. Lai, G. M. Zeng, D. L. Huang, L. Qin, M. M. Zhang, M. Cheng, X. G. Liu, H. Yi, C. Y. Zhou, F. L. Huang, S. Y. Liu and Y. K. Fu, Black Phosphorus, a Rising Star 2D Nanomaterial in the Post-Graphene Era: Synthesis, Properties, Modifications, and Photocatalysis Applications, Small, 2019, 15, 1804565 CrossRef PubMed.
-
G. Liu, W. Xi, X. You, Y. Zhi and C. Li, VOCs emission from an important industrial park in Tianjin, China, IOP Conference Series: Earth and Environmental Science, 2019, vol. 227, p. 062037 Search PubMed.
- U. B. Celebi and N. Vardar, Investigation of VOC emissions from indoor and outdoor painting processes in shipyards, Atmos. Environ., 2008, 42, 5685–5695 CrossRef CAS.
- H. Wang, L. Nie, J. Li, Y. Wang, G. Wang, J. Wang and Z. Hao, Characterization and assessment of volatile organic compounds (VOCs) emissions from typical industries, Chin. Sci. Bull., 2013, 58, 724–730 CrossRef CAS.
- K. Yang, C. Wang, S. Xue, W. Li, J. Liu and L. Li, The identification, health risks and olfactory effects assessment of VOCs released from the wastewater storage tank in a pesticide plant, Ecotoxicol. Environ. Saf., 2019, 184, 109665 CrossRef CAS PubMed.
- S. M. T. Sendesi, Y. Noh, M. Nuruddin, B. E. Boor, J. A. Howarter, J. P. Youngblood, C. T. Jafvert and A. J. Whelton, An emerging mobile air pollution source: outdoor plastic liner manufacturing sites discharge VOCs into urban and rural areas, Environ. Sci.: Processes Impacts, 2020, 22, 1828–1841 RSC.
- Y. Liu, F. Han, W. Liu, X. Cui, X. Luan and Z. Cui, Process-based volatile organic compound emission inventory establishment method for the petroleum refining industry, J. Cleaner Prod., 2020, 263, 121609 CrossRef CAS.
- X. Zhang, D. Wang, Y. Liu, Y. Cui, Z. Xue, Z. Gao and J. Du, Characteristics and ozone formation potential of volatile organic compounds in emissions from a typical Chinese coking plant, J. Environ. Sci., 2020, 95, 183–189 CrossRef PubMed.
- Q. Li, G. Su, C. Li, M. Wang, L. Tan, L. Gao, M. Wu and Q. Wang, Emission profiles, ozone formation potential and health-risk assessment of volatile organic compounds in rubber footwear industries in China, J. Hazard. Mater., 2019, 375, 52–60 CrossRef CAS PubMed.
- C. T. Chang and K. L. Lin, Assessment of the strategies for reducing VOCs emission from polyurea-formaldehyde resin synthetic fiber leather industry in Taiwan, Resour., Conserv. Recycl., 2006, 46, 321–334 CrossRef.
- N. Cheng, D. Jing, C. Zhang, Z. Chen, W. Li, S. Li and Q. Wang, Process-based VOCs source profiles and contributions to ozone formation and carcinogenic risk in a typical chemical synthesis pharmaceutical industry in China, Sci. Total Environ., 2021, 752, 141899 CrossRef CAS PubMed.
- H.-H. Yang, S. K. Gupta and N. B. Dhital, Emission factor, relative ozone formation potential and relative carcinogenic risk assessment of VOCs emitted from manufacturing industries, Sustainable Environ. Res., 2020, 30, 28 CrossRef CAS.
- H. H. Chen, C. E. Nanayakkara and V. H. Grassian, Titanium Dioxide Photocatalysis in Atmospheric Chemistry, Chem. Rev., 2012, 112, 5919–5948 CrossRef CAS PubMed.
- Q. Guo, C. Zhou, Z. Ma, Z. Ren, H. Fan and X. Yang, Elementary photocatalytic chemistry on TiO2 surfaces, Chem. Soc. Rev., 2016, 45, 3701–3730 RSC.
- M. A. Henderson, A surface science perspective on TiO2 photocatalysis, Surf. Sci. Rep., 2011, 66, 185–297 CrossRef CAS.
- S. H. Zhan, Y. Yang, X. C. Gao, H. B. Yu, S. S. Yang, D. D. Zhu and Y. Li, Rapid degradation of toxic toluene using novel mesoporous SiO2 doped TiO2 nanofibers, Catal. Today, 2014, 225, 10–17 CrossRef CAS.
- A. H. Mamaghani, F. Haghighat and C. S. Lee, Photocatalytic oxidation technology for indoor environment air purification: The state-of-the-art, Appl. Catal., B, 2017, 203, 247–269 CrossRef CAS.
- A. Bazyari, A. A. Khodadadi, A. H. Mamaghani, J. Beheshtian, L. T. Thompson and Y. Mortazavi, Microporous titania-silica nanocomposite catalyst-adsorbent for ultra-deep oxidative desulfurization, Appl. Catal., B, 2016, 180, 65–77 CrossRef CAS.
- V. Puddu, H. Choi, D. D. Dionysiou and G. L. Puma, TiO2 photocatalyst for indoor air remediation: Influence of crystallinity, crystal phase, and UV radiation intensity on trichloroethylene degradation, Appl. Catal., B, 2010, 94, 211–218 CrossRef CAS.
- Z. Long, Q. Li, T. Wei, G. Zhang and Z. Ren, Historical development and prospects of photocatalysts for pollutant removal in water, J. Hazard. Mater., 2020, 395, 122599 CrossRef CAS PubMed.
- S. Wang, Q. Sun, W. Chen, Y. Q. Tang, B. Aguila, Y. X. Pan, A. M. Zheng, Z. Y. Yang, L. Wojtas, S. Q. Ma and F. S. Xiao, Programming Covalent Organic Frameworks for Photocatalysis: Investigation of Chemical and Structural Variations, Matters, 2020, 2, 416–427 CrossRef.
- R. O. Da Silva, R. H. Goncalves, D. G. Stroppa, A. J. Ramirez and E. R. Leite, Synthesis of recrystallized anatase TiO2 mesocrystals with Wulff shape assisted by oriented attachment, Nanoscale, 2011, 3, 1910–1916 RSC.
- P. Pleskunov, T. Kosutova, M. Vaidulych, D. Nikitin, Z. Krtous, S. Ali-Ogly, K. Kishenina, R. Tafiichuk, H. Biederman, I. Gordeev, J. Drewes, I. Barg, F. Faupel, M. Cieslar, R. Yatskiv, Y. Pihosh, V. Nandal, K. Seki, K. Domen and A. Choukourov, The sputter-based synthesis of tantalum oxynitride nanoparticles with architecture and bandgap controlled by design, Appl. Surf. Sci., 2021, 559, 149974 CrossRef CAS.
- T. Katsuki, Z. N. Zahran, K. Tanaka, T. Eo, E. A. Mohamed, Y. Tsubonouchi, M. R. Berber and M. Yagi, Facile Fabrication of a Highly Crystalline and Well-Interconnected Hematite Nanoparticle Photoanode for Efficient Visible-Light-Driven Water Oxidation, ACS Appl. Mater. Interfaces, 2021, 13, 39282–39290 CrossRef CAS PubMed.
- Q. Li, J. Wang, Y. Z. Zhang, L. Ricardez-Sandoval, G. Y. Bai and X. W. Lan, Structural and Morphological Engineering of Benzothiadiazole-Based Covalent Organic Frameworks for Visible Light-Driven Oxidative Coupling of Amines, ACS Appl. Mater. Interfaces, 2021, 13, 39291–39303 CrossRef CAS PubMed.
- I. S. Curtis, R. J. Wills and M. Dasog, Photocatalytic hydrogen generation using mesoporous silicon nanoparticles: influence of magnesiothermic reduction conditions and nanoparticle aging on the catalytic activity, Nanoscale, 2021, 13, 2685–2692 RSC.
- Q. L. Li, T. Song, Y. P. Zhang, Q. Wang and Y. Yang, Boosting Photocatalytic Activity and Stability of Lead-Free Cs3Bi2Br9 Perovskite Nanocrystals via In Situ Growth on Monolayer 2D Ti3C2Tx MXene for C-H Bond Oxidation, ACS Appl. Mater. Interfaces, 2021, 13, 27323–27333 CrossRef CAS PubMed.
- Y. H. Zhang, G. D. Shen, C. H. Sheng, F. Zhang and W. Fan, The effect of piezo-photocatalysis on enhancing the charge carrier separation in BaTiO3/KNbO3 heterostructure photocatalyst, Appl. Surf. Sci., 2021, 562, 12 Search PubMed.
- A. Alonso-Tellez, R. Masson, D. Robert, N. Keller and V. Keller, Comparison of Hombikat UV100 and P25 TiO2 performance in gas-phase photocatalytic oxidation reactions, J. Photochem. Photobiol., A, 2012, 250, 58–65 CrossRef CAS.
- K. Nakata and A. Fujishima, TiO2 photocatalysis: Design and applications, J. Photochem. Photobiol., C, 2012, 13, 169–189 CrossRef CAS.
- M. Xiao, Z. L. Wang, M. Q. Lyu, B. Luo, S. C. Wang, G. Liu, H. M. Cheng and L. Z. Wang, Hollow Nanostructures for Photocatalysis: Advantages and Challenges, Adv. Mater., 2019, 31, 23 Search PubMed.
- M. Hajaghazadeh, V. Vaiano, D. Sannino, H. Kakooei, R. Sotudeh-Gharebagh and P. Ciambelli, Heterogeneous photocatalytic oxidation of methyl ethyl ketone under UV-A light in an LED-fluidized bed reactor, Catal. Today, 2014, 230, 79–84 CrossRef CAS.
- R. A. R. Monteiro, A. M. T. Silva, J. R. M. Ângelo, G. V. Silva, A. M. Mendes, R. A. R. Boaventura and V. J. P. Vilar, Photocatalytic oxidation of gaseous perchloroethylene over TiO2 based paint, J. Photochem. Photobiol., A, 2015, 311, 41–52 CrossRef CAS.
- Y. T. Liu, Q. P. Zhang, M. Xu, H. Yuan, Y. Chen, J. X. Zhang, K. Y. Luo, J. Q. Zhang and B. A. You, Novel and efficient synthesis of Ag-ZnO nanoparticles for the sunlight-induced photocatalytic degradation, Appl. Surf. Sci., 2019, 476, 632–640 CrossRef CAS.
- M. Rajca, NOM (HA and FA) Reduction in Water Using Nano Titanium Dioxide Photocatalysts (P25 and P90) and Membranes, Catalysts, 2020, 10, 13 CrossRef.
- N. Chen, Q. N. Gong, F. Wang, J. Ren, R. X. Wang and W. Z. Jiao, N-doped porous carbon-stabilized Pt in hollow nano-TiO2 with enhanced photocatalytic activity, Int. J. Hydrogen Energy, 2020, 45, 24779–24791 CrossRef CAS.
- W.-H. Li, K. Ding, H.-R. Tian, M.-S. Yao, B. Nath, W.-H. Deng, Y. Wang and G. Xu, Conductive Metal-Organic Framework Nanowire Array Electrodes for High-Performance Solid-State Supercapacitors, Adv. Funct. Mater., 2017, 27, 1702067 CrossRef.
- S. Wang, C. M. McGuirk, A. d'Aquino, J. A. Mason and C. A. Mirkin, Metal-Organic Framework Nanoparticles, Adv. Mater., 2018, 30, 1800202 CrossRef PubMed.
- Y. Zhang, X. Feng, S. Yuan, J. Zhou and B. Wang, Challenges and recent advances in MOF-polymer composite membranes for gas separation, Inorg. Chem. Front., 2016, 3, 896–909 RSC.
- Y. B. Dou, J. Zhou, A. Zhou, J. R. Li and Z. R. Nie, Visible-light responsive MOF encapsulation of noble-metal-sensitized semiconductors for high-performance photoelectrochemical water splitting, J. Mater. Chem. A, 2017, 5, 19491–19498 RSC.
- W. L. Xue, L. Wang, Y. K. Li, H. Chen, K. X. Fu, F. Zhang, T. He, Y. H. Deng, J. R. Li and C. Q. Wan, Reticular Chemistry for Ionic Liquid-Functionalized Metal-Organic Frameworks with High Selectivity for CO2, ACS Sustainable Chem. Eng., 2020, 8, 18558–18567 CrossRef CAS.
- L.-H. Xie, X.-M. Liu, T. He and J.-R. Li, Metal-Organic Frameworks for the Capture of Trace Aromatic Volatile Organic Compounds, Chem, 2018, 4, 1911–1927 CAS.
- J. Y. Wu, C. L. Tang, W. Y. Zhang, X. X. Ma, S. W. Qu, K. X. Chen, T. Hao and S. Chiang, Lab on optical fiber: surface nano-functionalization for real-time monitoring of VOC adsorption/desorption in metal-organic frameworks, Nanophotonics, 2021, 10, 2705–2716 CrossRef.
- M. J. Wang, Z. R. Shen, X. D. Zhao, F. P. Duanmu, H. J. Yu and H. M. Ji, Rational shape control of porous
Co3O4 assemblies derived from MOF and their structural effects on n-butanol sensing, J. Hazard. Mater., 2019, 371, 352–361 CrossRef CAS PubMed.
- Q. Yu, R. R. Jin, L. P. Zhao, T. S. Wang, F. M. Liu, X. Yan, C. G. Wang, P. Sun and G. Y. Lu, MOF-Derived Mesoporous and Hierarchical Hollow-Structured In2O3-NiO Composites for Enhanced Triethylamine Sensing, ACS Sens., 2021, 6, 3451–3461 CrossRef CAS PubMed.
- D. A. Giannakoudakis, A. Qayyum, D. Lomot, M. O. Besenhard, D. Lisovytskiy, T. J. Bandosz and J. C. Colmenares, Boosting the Photoactivity of Grafted Titania: Ultrasound-Driven Synthesis of a Multi-Phase Heterogeneous Nano-Architected Photocatalyst, Adv. Funct. Mater., 2021, 31, 2007115 CrossRef CAS.
- H. Wei, W. A. McMaster, J. Z. Y. Tan, D. H. Chen and R. A. Caruso, Tricomponent brookite/anatase TiO2/g-C3N4 heterojunction in mesoporous hollow microspheres for enhanced visible-light photocatalysis, J. Mater. Chem. A, 2018, 6, 7236–7245 RSC.
- M. Q. Hu, Y. Cao, Z. Z. Li, S. L. Yang and Z. P. Xing, Ti3+ self-doped mesoporous black TiO2/SiO2 nanocomposite as remarkable visible light photocatalyst, Appl. Surf. Sci., 2017, 426, 734–744 CrossRef CAS.
- N. Quici, M. L. Vera, H. Choi, G. L. Puma, D. D. Dionysiou, M. I. Litter and H. Destaillats, Effect of key parameters on the photocatalytic oxidation of toluene at low concentrations in air under 254+185 nm UV irradiation, Appl. Catal., B, 2010, 95, 312–319 CrossRef CAS.
- R. Singh, R. Bapat, L. J. Qin, H. Feng and V. Polshettiwar, Atomic Layer Deposited (ALD) TiO2 on Fibrous Nano-Silica (KCC-1) for Photocatalysis: Nanoparticle Formation and Size Quantization Effect, ACS Catal., 2016, 6, 2770–2784 CrossRef CAS.
- X. N. Wang, R. Long, D. Liu, D. Yang, C. M. Wang and Y. J. Xiong, Enhanced full-spectrum water splitting by confining plasmonic Au nanoparticles in N-doped TiO2 bowl nanoarrays, Nano Energy, 2016, 24, 87–93 CrossRef CAS.
- M. V. Roldan, P. de Ona, Y. Castro, A. Duran, P. Faccendini, C. Lagier, R. Grau and N. S. Pellegri, Photocatalytic and biocidal activities of novel coating systems of mesoporous and dense TiO2-anatase containing silver nanoparticles, Mater. Sci. Eng., C, 2014, 43, 630–640 CrossRef CAS PubMed.
- Z. Zhang, G. Wang, W. Li, L. Zhang, B. Guo, L. Ding and X. Li, Photocatalytic Activity of Magnetic Nano-beta-FeOOH/Fe3O4/Biochar Composites for the Enhanced Degradation of Methyl Orange Under Visible Light, Nanomaterials, 2021, 11, 526 CrossRef CAS PubMed.
- J. J. Zhang, Y. X. Li, L. Li, W. L. Li and C. F. Yang, Dual Functional N-Doped TiO2-Carbon Composite Fibers for Efficient Removal of Water Pollutants, ACS Sustainable Chem. Eng., 2018, 6, 12893–12905 CrossRef CAS.
- S. Shamaila, A. Khan Leghari Sajjad, A. Quart ul, S. Shaheen, A. Iqbal, S. Noor, G. Sughra and U. Ali, A cost effective and eco-friendly green route for fabrication of efficient graphene nanosheets photocatalyst, J. Environ. Chem. Eng., 2017, 5, 5770–5776 CrossRef CAS.
- S. Noor, S. Sajjad, S. A. K. Leghari, C. Flox, T. Kallio, E. I. Kauppinen and S. Ahmad, Electronic transitions of SWCNTs in comparison to GO on Mn3O4/TiO2 nanocomposites for hydrogen energy generation and solar photocatalysis, New J. Chem., 2021, 45, 2431–2442 RSC.
- Z. Yousaf, S. Sajjad, S. Ahmed Khan Leghari, M. Mehboob, A. Kanwal and B. Uzair, Interfacial charge transfer via 2D-NiO and 2D-graphene nanosheets combination for significant visible photocatalysis, J. Solid State Chem., 2020, 291, 121606 CrossRef CAS.
- S. Noor, S. Sajjad, S. A. K. Leghari and M. Long, Energy harvesting for electrochemical OER and solar photocatalysis
via dual functional GO/TiO2-NiO nanocomposite, J. Cleaner Prod., 2020, 277, 123280 CrossRef CAS.
- F. Fresno, M. D. Hernandez-Alonso, D. Tudela, J. M. Coronado and J. Soria, Photocatalytic degradation of toluene over doped and coupled (Ti,M)O-2 (M = Sn or Zr) nanocrystalline oxides: Influence of the heteroatom distribution on deactivation, Appl. Catal., B, 2008, 84, 598–606 CrossRef CAS.
- M. Takeuchi, J. Deguchi, S. Sakai and M. Anpo, Effect of H2O vapor addition on the photocatalytic oxidation of ethanol, acetaldehyde and acetic acid in the gas phase on TiO2 semiconductor powders, Appl. Catal., B, 2010, 96, 218–223 CrossRef CAS.
- M. J. Munoz-Batista, A. Kubacka, M. Natividad Gomez-Cerezo, D. Tudela and M. Fernandez-Garcia, Sunlight-driven toluene photo-elimination using CeO2-TiO2 composite systems: A kinetic study, Appl. Catal., B, 2013, 140, 626–635 CrossRef.
- N. Bouazza, M. A. Lillo-Rodenas and A. Linares-Solano, Photocatalytic activity of TiO2-based materials for the oxidation of propene and benzene at low concentration in presence of humidity, Appl. Catal., B, 2008, 84, 691–698 CrossRef CAS.
- H. Einaga, S. Futamura and T. Ibusuki, Heterogeneous photocatalytic oxidation of benzene, toluene, cyclohexene and cyclohexane in humidified air: comparison of decomposition behavior on photoirradiated TiO2 catalyst, Appl. Catal., B, 2002, 38, 215–225 CrossRef CAS.
- T. Guo, Z. Bai, C. Wu and T. Zhu, Influence of relative humidity on the photocatalytic oxidation (PCO) of toluene by TiO2 loaded on activated carbon fibers: PCO rate and intermediates accumulation, Appl. Catal., B, 2008, 79, 171–178 CrossRef CAS.
- G. Vincent, P. M. Marquaire and O. Zahraa, Photocatalytic degradation of gaseous 1-propanol using an annular reactor: Kinetic modelling and pathways, J. Hazard. Mater., 2009, 161, 1173–1181 CrossRef CAS PubMed.
- R. A. R. Monteiro, A. M. T. Silva, J. R. M. Angelo, G. V. Silva, A. M. Mendes, R. A. R. Boaventura and V. J. P. Vilar, Photocatalytic oxidation of gaseous perchloroethylene over TiO2 based paint, J. Photochem. Photobiol., A, 2015, 311, 41–52 CrossRef CAS.
- Y. Huang, H. Hu, S. Wang, M.-S. Balogun, H. Ji and Y. Tong, Low concentration nitric acid facilitate rapid electron-hole separation in vacancy-rich bismuth oxyiodide for photo-thermo-synergistic oxidation of formaldehyde, Appl. Catal., B, 2017, 218, 700–708 CrossRef CAS.
- Y. Tao, C.-Y. Wu and D. W. Mazyck, Removal of methanol from pulp and paper mills using combined activated carbon adsorption and photocatalytic regeneration, Chemosphere, 2006, 65, 35–42 CrossRef CAS PubMed.
- B. M. da Costa Filho, A. L. P. Araujo, G. V. Silva, R. A. R. Boaventura, M. M. Dias, J. C. B. Lopes and V. J. P. Vilar, Intensification of heterogeneous TiO2 photocatalysis using an innovative micro-meso-structured-photoreactor for n-decane oxidation at gas phase, Chem. Eng. J., 2017, 310, 331–341 CrossRef CAS.
- Z. Han, V.-W. Chang, X. Wang, T.-T. Lim and L. Hildemann, Experimental study on visible-light induced photocatalytic oxidation of gaseous formaldehyde by polyester fiber supported photocatalysts, Chem. Eng. J., 2013, 218, 9–18 CrossRef CAS.
- M. Hussain, N. Russo and G. Saracco, Photocatalytic abatement of VOCs by novel optimized TiO2 nanoparticles, Chem. Eng. J., 2011, 166, 138–149 CrossRef CAS.
- F. Moulis and J. Krysa, Photocatalytic degradation of several VOCs (n-hexane, n-butyl acetate and toluene) on TiO2 layer in a closed-loop reactor, Catal. Today, 2013, 209, 153–158 CrossRef CAS.
- L. Zhong, F. Haghighat, C.-S. Lee and N. Lakdawala, Performance of ultraviolet photocatalytic oxidation for indoor air applications: Systematic experimental evaluation, J. Hazard. Mater., 2013, 261, 130–138 CrossRef CAS PubMed.
- Y. X. Deng, Developing a Langmuir-type excitation equilibrium equation to describe the effect of light intensity on the kinetics of the photocatalytic oxidation, Chem. Eng. J., 2018, 337, 220–227 CrossRef CAS.
- L. Pinho, M. Rojas and M. J. Mosquera, Ag-SiO2-TiO2 nanocomposite coatings with enhanced photoactivity for self-cleaning application on building materials, Appl. Catal., B, 2015, 178, 144–154 CrossRef CAS.
- S. Sun, J. Ding, J. Bao, C. Gao, Z. Qi, X. Yang, B. He and C. Li, Photocatalytic degradation of gaseous toluene on Fe-TiO2 under visible light irradiation: A study on the structure, activity and deactivation mechanism, Appl. Surf. Sci., 2012, 258, 5031–5037 CrossRef CAS.
- R. Daghrir, P. Drogui and D. Robert, Modified TiO2 For Environmental Photocatalytic Applications: A Review, Ind. Eng. Chem. Res., 2013, 52, 3581–3599 CrossRef CAS.
- Z. Shayegan, F. Haghighat and C. S. Lee, Carbon-doped TiO2 film to enhance visible and UV light photocatalytic degradation of indoor environment volatile organic compounds, J. Environ. Chem. Eng., 2020, 8, 14 Search PubMed.
- H.-H. Chun and W.-K. Jo, Adsorption and photocatalysis of 2-ethyl-1-hexanol over graphene oxide-TiO2 hybrids post-treated under various thermal conditions, Appl. Catal., B, 2016, 180, 740–750 CrossRef CAS.
- E. Q. Yu, J. Chen and H. P. Jia, Enhanced light-driven photothermocatalytic activity on selectively dissolved LaTi1-xMnxO3+delta perovskites by photoactivation, J. Hazard. Mater., 2020, 399, 11 Search PubMed.
- Y. Zhang, Y. X. Liu, S. H. Xie, H. B. Huang, G. S. Guo, H. X. Dai and J. G. Deng, Supported ceria-modified silver catalysts with high activity and stability for toluene removal, Environ. Int., 2019, 128, 335–342 CrossRef CAS PubMed.
- F. Montecchio, M. U. Babler and K. Engvall, Development of an irradiation and kinetic model for UV processes in volatile organic compounds abatement applications, Chem. Eng. J., 2018, 348, 569–582 CrossRef CAS.
- M. J. Tian, F. Liao, Q. F. Ke, Y. J. Guo and Y. P. Guo, Synergetic effect of titanium dioxide ultralong nanofibers and activated carbon fibers on adsorption and photodegradation of toluene, Chem. Eng. J., 2017, 328, 962–976 CrossRef CAS.
- X. Li, J. G. Yu, J. X. Low, Y. P. Fang, J. Xiao and X. B. Chen, Engineering heterogeneous semiconductors for solar water splitting, J. Mater. Chem. A, 2015, 3, 2485–2534 RSC.
- S. M. Fang, Y. Z. Li, Y. Yang, J. Chen, H. H. Liu and X. J. Zhao, Mg-doped OMS-2 nanorods: a highly efficient catalyst for purification of volatile organic compounds with full solar spectrum irradiation, Environ. Sci.: Nano, 2017, 4, 1798–1807 RSC.
- X. Li, J. Q. Wen, J. X. Low, Y. P. Fang and J. G. Yu, Design and fabrication of semiconductor photocatalyst for photocatalytic reduction of CO2 to solar fuel, Sci. China Mater., 2014, 57, 70–100 CrossRef.
- F. Zhang, Y. Zhu, Q. Lin, L. Zhang, X. Zhang and H. Wang, Noble-metal single-atoms in thermocatalysis, electrocatalysis, and photocatalysis, Energy Environ. Sci., 2021, 14, 2954–3009 RSC.
- C. H. Nguyen, C. C. Fu and R. S. Juang, Degradation of methylene blue and methyl orange by palladium-doped TiO2 photocatalysis for water reuse: Efficiency and degradation pathways, J. Cleaner Prod., 2018, 202, 413–427 CrossRef CAS.
- P. Garcia-Ramirez, E. Ramirez-Morales, J. C. S. Cortazar, I. Sires and S. Silva-Martinez, Influence of ruthenium doping on UV- and visible-light photoelectrocatalytic color removal from dye solutions using a TiO2 nanotube array photoanode, Chemosphere, 2021, 267, 10 CrossRef PubMed.
- M. Perez-Gonzalez and S. A. Tomas, Surface chemistry of TiO2-ZnO thin films doped with Ag. Its role on the photocatalytic degradation of methylene blue, Catal. Today, 2021, 360, 129–137 CrossRef CAS.
- A. P. Manuel and K. Shankar, Hot Electrons in TiO2-Noble Metal Nano-Heterojunctions: Fundamental Science and Applications in Photocatalysis, Nanomaterials, 2021, 11, 55 CrossRef PubMed.
- S. I. Mogal, V. G. Gandhi, M. Mishra, S. Tripathi, T. Shripathi, P. A. Joshi and D. O. Shah, Single-Step Synthesis of Silver-Doped Titanium Dioxide: Influence of Silver on Structural, Textural, and Photocatalytic Properties, Ind. Eng. Chem. Res., 2014, 53, 5749–5758 CrossRef CAS.
- X. C. Meng, H. N. Qin and Z. S. Zhang, New insight into the enhanced visible light-driven photocatalytic activity of Pd/PdCl2-doped Bi2WO6 photocatalysts, J. Colloid Interface Sci., 2018, 513, 877–890 CrossRef CAS PubMed.
- X. Xue, X. Chen and X. Gong, Fast electron transfer and enhanced visible light photocatalytic activity of silver and Ag2O co-doped titanium dioxide with the doping of electron mediator for removing gaseous toluene, Mater. Sci. Semicond. Process., 2021, 132, 105901 CrossRef CAS.
- C. V. Reddy, I. N. Reddy, B. Akkinepally, V. V. N. Harish, K. R. Reddy and S. Jaesool, Mn-doped ZrO2 nanoparticles prepared by a template-free method for electrochemical energy storage and abatement of dye degradation, Ceram. Int., 2019, 45, 15298–15306 CrossRef CAS.
- T. Tsuzuki, R. He, A. Dodd and M. Saunders, Challenges in Determining the Location of Dopants, to Study the Influence of Metal Doping on the Photocatalytic Activities of ZnO Nanopowders, Nanomaterials, 2019, 9, 481 CrossRef CAS PubMed.
- X. Wang, M. Hong, F. Zhang, Z. Zhuang and Y. Yu, Recyclable Nanoscale Zero Valent Iron Doped g-C3N4/MoS2 for Efficient Photocatalysis of RhB and Cr(VI) Driven by Visible Light, ACS Sustainable Chem. Eng., 2016, 4, 4055–4062 CrossRef CAS.
- P. K. Boruah, B. Sharma, I. Karbhal, M. V. Shelke and M. R. Das, Ammonia-modified graphene sheets decorated with magnetic Fe3O4 nanoparticles for the photocatalytic and photo-Fenton degradation of phenolic compounds under sunlight irradiation, J. Hazard. Mater., 2017, 325, 90–100 CrossRef CAS PubMed.
- H. R. Rajabi, O. Khani, M. Shamsipur and V. Vatanpour, High-performance pure and Fe3+−ion doped ZnS quantum dots as green nanophotocatalysts for the removal of malachite green under UV-light irradiation, J. Hazard. Mater., 2013, 250, 370–378 CrossRef PubMed.
- J. N. Qu, Y. Du, P. H. Ji, Z. F. Li, N. Jiang, X. Y. Sun, L. Xue, H. Y. Li and G. L. Sun, Fe, Cu co-doped BiOBr with improved photocatalytic ability of pollutants degradation, J. Alloys Compd., 2021, 881, 10 CrossRef.
- H. Bantawal, U. S. Shenoy and D. K. Bhat, Vanadium-Doped SrTiO3 Nanocubes: Insight into role of vanadium in improving the photocatalytic activity, Appl. Surf. Sci., 2020, 513, 7 CrossRef.
- T. Wang, X. Q. Liu, D. L. Han, C. C. Ma, M. B. Wei, P. W. Huo and Y. S. Yan, Biomass derived the V-doped carbon/Bi2O3 composite for efficient photocatalysts, Environ. Res., 2020, 182, 9 Search PubMed.
- I. N. Reddy, C. V. Reddy, J. Shim, B. Akkinepally, M. Y. Cho, K. Yoo and D. Kim, Excellent visible-light driven photocatalyst of (Al, Ni) co-doped ZnO structures for organic dye degradation, Catal. Today, 2020, 340, 277–285 CrossRef CAS.
- M. Afif, U. Sulaeman, A. Riapanitra, R. Andreas and S. Yin, Use of Mn doping to suppress defect sites in Ag3PO4: Applications in photocatalysis, Appl. Surf. Sci., 2019, 466, 352–357 CrossRef CAS.
- P. Herr, C. Kerzig, C. B. Larsen, D. Haussinger and O. S. Wenger, Manganese(i) complexes with metal-to-ligand charge transfer luminescence and photoreactivity, Nat. Chem., 2021, 13, 956–962 CrossRef CAS PubMed.
- P. Devaraji, N. K. Sathu and C. S. Gopinath, Ambient Oxidation of Benzene to Phenol by Photocatalysis on Au/Ti0.98V0.02O2: Role of Holes, ACS Catal., 2014, 4, 2844–2853 CrossRef CAS.
- M. Stucchi, D. C. Boffito, E. Pargoletti, G. Cerrato, C. L. Bianchi and G. Cappelletti, Nano-MnO2 Decoration of TiO2 Microparticles to Promote Gaseous Ethanol Visible Photoremoval, Nanomaterials, 2018, 8, 686 CrossRef PubMed.
- R. C. Li, B. H. Hu, T. W. Yu, Z. P. Shao, Y. Wang and S. Q. Song, New TiO2-Based Oxide for Catalyzing Alkaline Hydrogen Evolution Reaction with Noble Metal-Like Performance, Small Methods, 2021, 5, 9 Search PubMed.
- S. Shamaila, T. Bano and A. K. L. Sajjad, Efficient visible light magnetic modified iron oxide photocatalysts, Ceram. Int., 2017, 43, 14672–14677 CrossRef CAS.
- J. R. Xiao, T. Y. Peng, R. Li, Z. H. Peng and C. H. Yan, Preparation, phase transformation and photocatalytic activities of cerium-doped mesoporous titania nanoparticles, J. Solid State Chem., 2006, 179, 1161–1170 CrossRef CAS.
- H. G. Wang, F. F. Wen, X. Y. Li, X. R. Gan, Y. N. Yang, P. Chen and Y. Zhang, Cerium-doped MoS2 nanostructures: Efficient visible photocatalysis for Cr(VI) removal, Sep. Purif. Technol., 2016, 170, 190–198 CrossRef CAS.
- H. Gandelman, A. L. da Silva, B. Ramos and D. Gouvea, Interface excess on Sb-doped TiO2 photocatalysts and its influence on photocatalytic activity, Ceram. Int., 2021, 47, 619–625 CrossRef CAS.
- O. Aviles-Garcia, J. Espino-Valencia, R. Romero, J. L. Rico-Cerda, M. Arroyo-Albiter and R. Natividad, W and Mo doped TiO2: Synthesis, characterization and photocatalytic activity, Fuel, 2017, 198, 31–41 CrossRef CAS.
- K. Goswami and R. Ananthakrishnan, Ce-Doped CuMgAl Oxide as a Redox Couple Mediated Catalyst for Visible Light Aided Photooxidation of Organic Pollutants, ACS Appl. Nano Mater., 2019, 2, 6030–6039 CrossRef CAS.
- Z. Shayegan, F. Haghighat and C.-S. Lee, Surface fluorinated Ce-doped TiO2 nanostructure photocatalyst: A trap and remove strategy to enhance the VOC removal from indoor air environment, Chem. Eng. J., 2020, 401, 125932 CrossRef CAS.
- X. F. Peng, C. H. Wang, Y. Y. Li, H. Ma, F. Yu, G. S. Che, J. Y. Yan, X. T. Zhang and Y. C. Liu, Revisiting cocatalyst/TiO2 photocatalyst in blue light photothermalcatalysis, Catal. Today, 2019, 335, 286–293 CrossRef CAS.
- Y. Huang, Z. Guo, H. Liu, S. Zhang, P. Wang, J. Lu and Y. Tong, Heterojunction Architecture of N-Doped WO3 Nanobundles with Ce2S3 Nanodots Hybridized on a Carbon Textile Enables a Highly Efficient Flexible Photocatalyst, Adv. Funct. Mater., 2019, 29, 1903490 CrossRef CAS.
- H. Che, G. Che, P. Zhou, C. Liu, H. Dong, C. Li, N. Song and C. Li, Nitrogen doped carbon ribbons modified g-C3N4 for markedly enhanced photocatalytic H-2-production in visible to near-infrared region, Chem. Eng. J., 2020, 382, 122870 CrossRef CAS.
- S. Wu, H. Hu, Y. Lin, J. Zhang and Y. H. Hu, Visible light photocatalytic degradation of tetracycline over TiO2, Chem. Eng. J., 2020, 382, 122842 CrossRef CAS.
- G. S. Jamila, S. Sajjad, S. A. K. Leghari and M. Long, Nitrogen doped carbon quantum dots and GO modified WO3 nanosheets combination as an effective visible photo catalyst, J. Hazard. Mater., 2020, 382, 121087 CrossRef CAS PubMed.
- G. S. Jamila, S. Sajjad, S. A. K. Leghari and T. Mahmood, Role of nitrogen doped carbon quantum dots on CuO nano-leaves as solar induced photo catalyst, J. Phys. Chem. Solids, 2020, 138, 109233 CrossRef CAS.
- J. Lu, Y. Wang, J. Huang, J. Fei, L. Cao and C. Li, In situ synthesis of mesoporous C-doped TiO2 single crystal with oxygen vacancy and its enhanced sunlight photocatalytic properties, Dyes Pigm., 2017, 144, 203–211 CrossRef CAS.
- J. Matos, J. Ocares-Riquelme, P. S. Poon, R. Montana, X. Garcia, K. Campos, J. C. Hernandez-Garrido and M. M. Titirici, C-doped anatase TiO2: Adsorption kinetics and photocatalytic degradation of methylene blue and phenol, and correlations with DFT estimations, J. Colloid Interface Sci., 2019, 547, 14–29 CrossRef CAS PubMed.
- S. Wang, X. Zhang, S. Li, Y. Fang, L. Pan and J.-J. Zou, C-doped ZnO ball-in-ball hollow microspheres for efficient
photocatalytic and photoelectrochemical applications, J. Hazard. Mater., 2017, 331, 235–245 CrossRef CAS PubMed.
- Z. Ma, Y. Li, Y. Lv, R. Sa, Q. Li and K. Wu, Synergistic Effect of Doping and Compositing on Photocatalytic Efficiency: A Case Study of La2Ti2O7, ACS Appl. Mater. Interfaces, 2018, 10, 39327–39335 CrossRef CAS PubMed.
- C. Feng, L. Tang, Y. Deng, G. Zeng, J. Wang, Y. Liu, Z. Chen, J. Yu and J. Wang, Enhancing optical absorption and charge transfer: Synthesis of S-doped h-BN with tunable band structures for metal-free visible-light-driven photocatalysis, Appl. Catal., B, 2019, 256, 117827 CrossRef CAS.
- M. A. Baghchesara, H. R. Azimi, A. G. Shiravizadeh, M. A. M. Teridi and R. Yousefi, Improving the intrinsic properties of rGO sheets by S-doping and the effects of rGO improvements on the photocatalytic performance of Cu3Se2/rGO nanocomposites, Appl. Surf. Sci., 2019, 466, 401–410 CrossRef CAS.
- R. Wang, D. Li, H. Wang, C. Liu and L. Xu, Preparation, Characterization, and Performance Analysis of S-Doped Bi2MoO6 Nanosheets, Nanomaterials, 2019, 9, 1341 CrossRef CAS PubMed.
- Y. Shi, G. Zhan, H. Li, X. Wang, X. Liu, L. Shi, K. Wei, C. Ling, Z. Li, H. Wang, C. Mao, X. Liu and L. Zhang, Simultaneous Manipulation of Bulk Excitons and Surface Defects for Ultrastable and Highly Selective CO2 Photoreduction, Adv. Mater., 2021, 33, 2100143 CrossRef CAS PubMed.
- Y. Dong, D. Xu, Q. Wang, G. Zhang, Q. Zhang, Z. Zhang, L. Lv, Y. Xia, Z. Ren and P. Wang, Tailoring the electronic structure of ultrathin 2D Bi3O4Cl sheets by boron doping for enhanced visible light environmental remediation, Appl. Surf. Sci., 2021, 542, 148521 CrossRef CAS.
- Q. Wang, B. Rhimi, H. Wang and C. Y. Wang, Efficient photocatalytic degradation of gaseous toluene over F-doped TiO2/exfoliated bentonite, Appl. Surf. Sci., 2020, 530, 12 Search PubMed.
- A. Khalilzadeh and S. Fatemi, Spouted bed reactor for VOC removal by modified nano-TiO2 photocatalytic particles, Chem. Eng. Res. Des., 2016, 115, 241–250 CrossRef CAS.
- M. Kamaei, H. Rashedi, S. M. M. Dastgheib and S. Tasharrofi, Comparing Photocatalytic Degradation of Gaseous Ethylbenzene Using N-doped and Pure TiO2 Nano-Catalysts Coated on Glass Beads under Both UV and Visible Light Irradiation, Catalysts, 2018, 8, 466 CrossRef.
- E. W. Awin, A. Lale, K. C. Hari Kumar, S. Bernard and R. Kumar, Disordered mesoporous polymer derived N-doped TiO2/Si-O-C-N nanocomposites with nanoscaled heterojunctions towards enhanced adsorption and harnessing of visible light, Appl. Surf. Sci., 2020, 508, 144953 CrossRef CAS.
- M. L. Sun, X. A. Dong, B. Lei, J. Y. Li, P. Chen, Y. X. Zhang and F. Dong, Graphene oxide mediated co-generation of C-doping and oxygen defects in Bi2WO6 nanosheets: a combined DRIFTS and DFT investigation, Nanoscale, 2019, 11, 20562–20570 RSC.
- W. Diao, J. Xu, X. Rao and Y. Zhang, Facile Synthesis of Fluorine Doped Rutile TiO2 Nanorod Arrays for Photocatalytic Removal of Formaldehyde, Catal. Lett., 2021 DOI:10.1007/s10562-021-03700-x.
- P. Ramacharyulu, J. P. Kumar, G. K. Prasad and B. Sreedhar, Sulphur doped nano TiO2: Synthesis, characterization and photocatalytic degradation of a toxic chemical in presence of sunlight, Mater. Chem. Phys., 2014, 148, 692–698 CrossRef CAS.
- R. Asahi, T. Morikawa, H. Irie and T. Ohwaki, Nitrogen-Doped Titanium Dioxide as Visible-Light-Sensitive Photocatalyst: Designs, Developments, and Prospects, Chem. Rev., 2014, 114, 9824–9852 CrossRef CAS PubMed.
- S. L. Yang, L. Peng, P. P. Huang, X. S. Wang, Y. B. Sun, C. Y. Cao and W. G. Song, Nitrogen, Phosphorus, and Sulfur Co-Doped Hollow Carbon Shell as Superior Metal-Free Catalyst for Selective Oxidation of Aromatic Alkanes, Angew. Chem., Int. Ed., 2016, 55, 4016–4020 CrossRef CAS PubMed.
- Y. Wu, H. Wang, W. Tu, Y. Liu, Y. Z. Tan, X. Z. Yuan and J. W. Chew, Quasi-polymeric construction of stable perovskite-type LaFeO3/g-C3N4 heterostructured photocatalyst for improved Z-scheme photocatalytic activity via solid p-n heterojunction interfacial effect, J. Hazard. Mater., 2018, 347, 412–422 CrossRef CAS PubMed.
- H. L. Liu, X. Y. Chang, X. X. Liu, G. Y. Li, W. P. Zhang and T. C. An, Boosting the photocatalytic degradation of ethyl acetate by a Z-scheme Au-TiO2@NH2-UiO-66 heterojunction with ultrafine Au as an electron mediator, Environ. Sci.: Nano, 2021, 8, 2542–2553 RSC.
- A. Enesca and L. Isac, Photocatalytic Activity of Cu2S/WO3 and Cu2S/SnO2 Heterostructures for Indoor Air Treatment, Materials, 2021, 14, 3656 CrossRef CAS PubMed.
- G. P. Dai, J. G. Yu and G. Liu, Synthesis and Enhanced Visible-Light Photoelectrocatalytic Activity of p-n Junction BiOI/TiO2 Nanotube Arrays, J. Phys. Chem. C, 2011, 115, 7339–7346 CrossRef CAS.
- H. Guo, C. G. Niu, D. W. Huang, N. Tang, C. Liang, L. Zhang, X. J. Wen, Y. Yang, W. J. Wang and G. M. Zeng, Integrating the plasmonic effect and p-n heterojunction into a novel Ag/Ag2O/PbBiO2Br photocatalyst: Broadened light absorption and accelerated charge separation co-mediated highly efficient visible/NIR light photocatalysis, Chem. Eng. J., 2019, 360, 349–363 CrossRef CAS.
- H. W. Huang, K. Xiao, Y. He, T. R. Zhang, F. Dong, X. Du and Y. H. Zhang, In situ assembly of BiOI@Bi12O17Cl2 p-n junction: charge induced unique front-lateral surfaces coupling heterostructure with high exposure of BiOI {001} active facets for robust and nonselective photocatalysis, Appl. Catal., B, 2016, 199, 75–86 CrossRef CAS.
- A. Iqbal, S. Sajjad and S. A. K. Leghari, Low Cost Graphene Oxide Modified Alumina Nanocomposite as Solar Light Induced Photocatalyst, ACS Appl. Nano Mater., 2018, 1, 4612–4621 CrossRef CAS.
- S. Sajjad, M. Khan, S. A. K. Leghari, N. A. Ryma and S. A. Farooqi, Potential visible WO3/GO composite photocatalyst, Int. J. Appl. Ceram. Technol., 2019, 16, 1218–1227 CrossRef CAS.
- Z. Yao, H. Sun, S. Xiao, Y. Hu, X. Liu and Y. Zhang, Synergetic piezo-photocatalytic effect in a Bi2MoO6/BiOBr composite for decomposing organic pollutants, Appl. Surf. Sci., 2021, 560, 150037 CrossRef CAS.
- J. Q. Pan, C. Y. Chi, M. Z. You, Z. Y. Jiang, W. J. Zhao, M. Zhu, C. S. Song, Y. Y. Zheng and C. R. Li, The three dimensional Z-scheme Ag3PO4/Ag/MoS2/TiO2 nano-heterojunction and its sunlight photocatalytic performance enhancement, Mater. Lett., 2018, 227, 205–208 CrossRef CAS.
- X. G. Wang, M. H. Sun, M. Murugananthan, Y. R. Zhang and L. Z. Zhang, Electrochemically self-doped WO3/TiO2 nanotubes for photocatalytic degradation of volatile organic compounds, Appl. Catal., B, 2020, 260, 11 Search PubMed.
- M. Ding, W. Ao, H. Xu, W. Chen, L. Tao, Z. Shen, H. Liu, C. Lu and Z. Xie, Facile construction of dual heterojunction CoO@TiO2/MXene hybrid with efficient and stable catalytic activity for phenol degradation with peroxymonosulfate under visible light irradiation, J. Hazard. Mater., 2021, 420, 126686 CrossRef CAS PubMed.
- W. J. Wang, W. Q. Gu, G. Y. Li, H. J. Xie, P. K. Wong and T. C. An, Few-layered tungsten selenide as a co-catalyst for visible-light-driven photocatalytic production of hydrogen peroxide for bacterial inactivation, Environ. Sci.: Nano, 2020, 7, 3877–3887 RSC.
- J. Shen, R. Wang, Q. Q. Liu, X. F. Yang, H. Tang and J. Yang, Accelerating photocatalytic hydrogen evolution and pollutant degradation by coupling organic co-catalysts with TiO2, Chin. J. Catal., 2019, 40, 380–389 CrossRef CAS.
- S. Bai, L. M. Wang, X. Y. Chen, J. T. Du and Y. J. Xiong, Chemically exfoliated metallic MoS2 nanosheets: A promising supporting co-catalyst for enhancing the photocatalytic performance of TiO2 nanocrystals, Nano Res., 2015, 8, 175–183 CrossRef CAS.
- L. Q. Ye, Y. R. Su, X. L. Jin, H. Q. Xie and C. Zhang, Recent advances in BiOX (X = Cl, Br and I) photocatalysts: synthesis, modification, facet effects and mechanisms, Environ. Sci.: Nano, 2014, 1, 90–112 RSC.
- Y. Liang, Y. Yang, C. W. Zou, K. Xu, X. F. Luo, T. Luo, J. Y. Li, Q. Yang, P. Y. Shi and C. L. Yuan, 2D ultra-thin WO3 nanosheets with dominant {002} crystal facets for high-performance xylene sensing and methyl orange photocatalytic degradation, J. Alloys Compd., 2019, 783, 848–854 CrossRef CAS.
- J. C. C. Yu, V. H. Nguyen, J. Lasek and J. C. S. Wu, Titania nanosheet photocatalysts with dominantly exposed (001) reactive facets for photocatalytic NOx abatement, Appl. Catal., B, 2017, 219, 391–400 CrossRef CAS.
- H. Li, F. Deng, Y. Zheng, L. Hua, C. H. Qu and X. B. Luo, Visible-light-driven Z-scheme rGO/Bi2S3-BiOBr heterojunctions with tunable exposed BiOBr (102) facets for efficient synchronous photocatalytic degradation of 2-nitrophenol and Cr(vi) reduction, Environ. Sci.: Nano, 2019, 6, 3670–3683 RSC.
Footnote |
† These authors contributed equally to this work. |
|
This journal is © The Royal Society of Chemistry 2022 |
Click here to see how this site uses Cookies. View our privacy policy here.