DOI:
10.1039/D1EN00870F
(Critical Review)
Environ. Sci.: Nano, 2022,
9, 12-39
Uptake, translocation, and transformation of silver nanoparticles in plants
Received
18th September 2021
, Accepted 24th November 2021
First published on 26th November 2021
Abstract
Silver nanoparticles (AgNPs) have been globally applied in consumer products because of their antimicrobial properties. Broad applications inevitably release AgNPs into the natural environment. Moreover, AgNPs are produced naturally in soils. Plant uptake of AgNPs is the first step of their transfer along food chains, which ultimately raises concerns about human health. This review systematically summarizes the current knowledge on the uptake process of AgNPs in plants which is regulated by the physical properties of nanoparticles (e.g., size, surface coating, and morphology), environmental conditions (e.g., soil redox conditions, soil components, co-contaminants, and symbiotic microorganisms), and plant species (e.g., monocotyledon and dicotyledon). After internalization, AgNPs are translocated within plants and undergo biotransformation, including aggregation, oxidative dissolution, sulfidation, chlorination, and complexation with organic matter such as thiolates. Little is known about whether the internalized AgNPs and the transformed products will be accumulated in edible tissues of plants, which can raise the possibility of Ag to enter the food chains. Knowledge gaps about detailed mechanisms of transformation and translocation of AgNPs in planta still limit our understanding of Ag fate in plant–soil systems. This review intends to give a comprehensive assessment of the interaction between AgNPs and plants, highlighting that an integrated investigation of the full life cycle of AgNPs in plant–soil systems is needed to ensure safe application of nanoparticles.
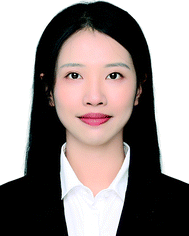 Danyu Huang | Danyu Huang received her Bachelor's degree in Environmental Science from Nanjing University. She is currently a Ph.D. candidate at Nanjing University under the supervision of Prof. Dongmei Zhou. Her research interests include the environmental fate of nanoparticles and the formation of reactive oxygen species in paddy soils. |
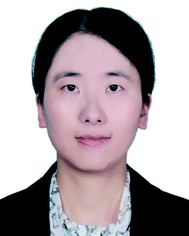 Fei Dang | Fei Dang received her Ph.D. in Marine Environmental Science from Hong Kong University of Science and Technology in 2011. Since 2013 she has been an associate professor in the Institute of Soil Science, Chinese Academy of Sciences. Her research focuses on the biogeochemistry of trace metals and metallic nanoparticles, including their fate, bioavailability and trophic transfer, in the environment, as well as the application of nanotechnology. |
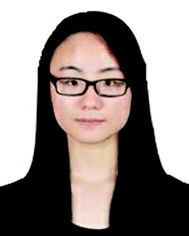 Yingnan Huang | Yingnan Huang received her Bachelor's degree in Environmental Science from Shaanxi Normal University. She is currently a postdoctor at the Institute of Soil Science, Chinese Academy of Sciences, after receiving her Ph.D from the same institute. Her research interests include the biogeochemistry of heavy metals and the environmental fate and implications of nanoparticles and microplastics in soils. |
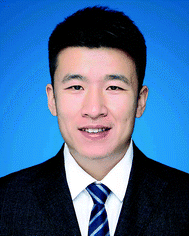 Ning Chen | Ning Chen received his Ph.D. in Environmental Science from the Institute of Soil Science, Chinese Academy of Sciences in 2018. Thereafter, he has been an assistant researcher in the School of Environment at Nanjing University. His research focuses on the formation of reactive oxygen species and its implications for element or contaminant transformation during geochemical processes. |
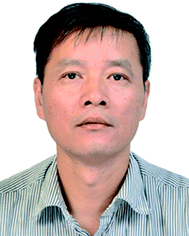 Dongmei Zhou | Dongmei Zhou is a professor at the School of the Environment at Nanjing University. He received his Ph.D. in Chemistry from Nanjing University in 1997, and worked in the Institute of Soil Science, Chinese Academy of Sciences until April, 2020. His current interests include the transportation and transformation of natural and engineered nanoparticles in the subsurface, biogeochemistry of heavy metals in the environment and remediation of soils contaminated with heavy metals and organic contaminants. He has published over 350 SCI papers. |
Environmental significance
The release of engineered AgNPs into the environment has raised concerns about their adverse impacts on plants, the basic components of ecosystems. The uptake of AgNPs in plants (especially crops) changes the fate of Ag in the environment and poses a risk to human health through trophic transfer. In this critical review, we reviewed the mechanisms regarding the uptake, translocation, transformation, and accumulation of AgNPs in plants, and their subsequent transfer along food chains. Critical issues and concerns were put forward to have a better understanding of nanoparticle–plant interaction. These findings will be helpful to explore the fate of AgNPs in terrestrial systems, especially in agricultural soils.
|
1. Introduction
Development of nanomaterial-based agrochemicals, such as nanofertilizers and nanopesticides, has sparked a technological revolution in the sustainable modern agricultural industry.1–7 Nanomaterials offer great potential to enhance agrochemical delivery, suppress pathogen activity, and improve crop yield and quality.8–10 Recently, silver nanoparticles (AgNPs) have been the focus of intense research due to their broad spectrum of antimicrobial properties.11,12 AgNPs are used as nanopesticides in agricultural activities to control plant pathogens, which has led to their direct contact with plants.13–19 Moreover, AgNPs are incorporated into a great number of consumer products including textiles, cosmetics, paints, and food packaging.20,21 More than 50% of the biocidal silver products (e.g., disinfectants, algicides and water filters) registered in the U.S. Environmental Protection Agency contain AgNPs, with an estimated global production of hundreds of tons per year.22,23 Daily use of consumer products introduces AgNPs into wastewater and later, intact AgNPs as well as transformed Ag species (mainly silver sulfide (Ag2S)) can enter the agriculture environment via wastewater irrigation or sludge fertilizer usage.24–29 Overall, the diverse applications inevitably increase AgNP release and anthropogenic input has been regarded as a new source of Ag to natural environments, making soil a major sink for these nanoparticles. Of particular concern are the potential biological impacts of nanoparticles on plants, which act as a major entry point of contaminants into food chains.30–32
Researchers have made efforts to investigate the complex interaction between silver and plants. A bibliometric analysis was performed based on the associated studies from Web of Science (WoS) between 1992 and 2021. The time zone diagram of keywords focusing on the research evolution of AgNPs over 30 years is presented in Fig. 1. From 1992 to 2001, most of these studies focused on the accumulation and toxicity of dissolved silver in plants. From 2002 to 2011, with the rapid expansion of nanotechnology, studies were concentrated on the bioavailability of AgNPs and their plant uptake. From 2012 to 2021, the translocation and transformation of AgNPs in plants have been brought into focus. The uptake of AgNPs by plants plays a crucial role in the fate of Ag in the environment and is influenced by various factors, including exposure pathways, particle properties, soil components, plant species, etc.33–36 However, the possible transformation of AgNPs in biological surfaces makes the uptake processes more complicated. After entering plants via different exposure pathways, AgNPs may aggregate into larger particles, form a biomolecule corona, or transform into various Ag species, such as AgCl, Ag2S and Ag-thiols, which possess different translocation patterns compared with the pristine particles.33,37,38 In the recent decade, models have been expanded from model plants (e.g., Arabidopsis) to crops (e.g., wheat and rice), as shown in Fig. 1, highlighting the possible transfer of AgNPs through crop uptake to humans.39,40 Although the interaction between nanoparticles and plants has attracted much attention, to date, insufficient knowledge still limits our understanding of the uptake, transformation, and translocation of AgNPs in different plants.
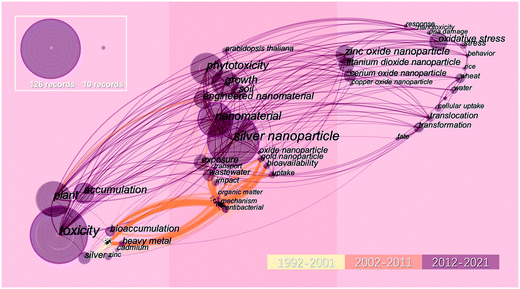 |
| Fig. 1 Time zone diagram of keyword co-occurring networks of papers concerning “AgNPs–plants interaction” between 1992 and 2021. The bibliometric analysis was performed using CiteSpace (version 5.7 R5) based on the database from Web of Science (WOS) between 1992 and 2021. The topic search used syntax “TS = ((silver OR Ag OR “particulate Ag” OR “Nano Ag” OR ((silver or Ag) AND (nanoparticle* OR particle*))) AND (plant) AND (uptake) AND (toxic*))”. Circle size is proportional to the number of publications. Co-citation rings and links within the same decades are shown in a unique color. The inset shows the circle size for the maximum and minimum number of records. | |
The worldwide application of nanotechnology, with the goals of sustainable agriculture and global food security, should be pursued together with comprehensive environmental risk assessments of nanomaterials to ensure ecological safety. Challenges and barriers exist to understand the fate of nanomaterials (such as AgNPs) in agroecosystems, including retention and transformation in soils, plant uptake, in planta transformation, accumulation, and trophic transfer. In this review, we discussed the current state of art and perspectives for the interaction between AgNPs and plants, focusing on the factors that affect uptake. The translocation, biotransformation, and accumulation of AgNPs inside plants have been summarized. Concerns over the incompleteness of experimental methods are also raised. Although research on the trophic transfer of AgNPs is inadequate, a deeper understanding of the fate of AgNPs in planta may be informative to evaluate their phytotoxicity and risks to the environment and human health.
2. Non-anthropogenic sources of AgNPs in plant-soil systems
Engineered AgNPs are introduced into plant-soil systems mainly through the usage of AgNP-containing nanopesticides, wastewater irrigation, and sludge application.41–43 Although anthropogenic input is considered as the major source of AgNPs, the natural generation of AgNPs in soil cannot be neglected. In the environment, Ag exists mainly as Ag2S, which was believed to be chemically inert.44,45 However, the unheeded biotic or abiotic transformation of Ag2S has led to the release of Ag+ and the generation of AgNPs.46,47 Indeed, AgNPs have been discovered in silver-contaminated soils, where anthropogenic input (e.g., AgNPs in medical and consumer products) was unlikely.48–50 These issues raise critical concerns regarding the background levels of naturally generated AgNPs in soils, which may be underestimated and can readily confound the quantification of Ag contamination induced by human activities.51 However, there are few studies on the natural formation of AgNPs in soils. Although natural formation processes and mechanisms of AgNPs have been widely documented in aquatic systems,52–54 extrapolating these results to terrestrial systems is difficult due to significant distinctions between these two media. In this review, we proposed two main mechanisms for natural AgNP formation from Ag+ in soils, involving abiotic and biotic reduction.
Natural generation of AgNPs via abiotic reduction is of great concern, which is governed by multiple factors including light, natural organic matter (NOM), oxygen, temperature, pH, and co-existing ions (e.g., Fe2+/Fe3+).53–61 Particulate organic matter (POM), typically comprising >50% soil organic matter, contributed to 11–31% of natural AgNP generation at the soil surface under natural conditions.62 The redox-active phenolic groups within POM are responsible for the formation of AgNPs both in the dark and under solar irradiation. However, the photoirradiation pathway prevails against the direct electron transfer pathway, due to the production of reductive superoxide anion radicals (O2˙−) from phenol-like groups on insoluble humin under light exposure.62 These findings offer fresh insights into the interplay between POM and Ag, which seems to favor POM-rich soils as a sink for natural AgNPs.
A few studies have revealed the mechanisms of how NOM reduced Ag+ into AgNPs in natural scenarios (especially in water bodies) and found that light irradiation was the crucial factor. NOM can directly reduce Ag+ through ligand-to-metal charge transfer or indirectly by transferring electrons from their phenolic groups to dissolved oxygen to generate O2˙−.55,60,61 Without light irradiation, the redox-active functional group of soil organic matter can directly reduce Ag+ into AgNPs.55,63 Charge transfer from the ligand to the metal has also been observed where Ag+ was firstly bound to the carboxylic group (–COO−) of HA to form Ag-HA complexes and then reduced into AgNPs by the transferred electrons.64 Whether ligand-to-metal transfer or O2˙− dominates the reduction process remains unclear but it is suggested to be relevant to the inherent redox reactivity of NOM.53,55,60,65 Different sources of NOM exhibit distinct reduction abilities, mainly due to the different contents of quinone and phenolic groups.55,60
Given that the standard redox potential of Ag+/Ag0 is 0.8 V, smaller value redox couples (e.g., Fe2+/Fe3+) may be able to reduce Ag+ to AgNPs in thermodynamic feasibility.66–68 Ferrous iron can directly reduce Ag+ under sunlight irradiation or indirectly by generating O2˙− under aerobic conditions.68,69 The Fe2+/Fe3+ redox couple can also act as an electron shuttle to promote the electron transfer from NOM to Ag+, thus enhancing the formation of AgNPs.67,70 Despite dissolved Fe2+, ferrous iron is also presented as Fe(II)-bearing minerals in natural soils.71 Green rusts, layered Fe(II)/Fe(III) hydroxides, have also been found to reduce Ag+ into AgNPs under dark conditions.72,73 Notably, given the heterogeneity in soil environments (NOM associated with minerals), the abiotic pathways for formation of AgNPs could be more complex in nature.
Although not fully understood, the natural formation of AgNPs in soil via biotic pathways also has significant implications for the environmental fate of Ag. The potential contribution of microorganisms has been early discovered in a silver mine, in which the living bacteria reduced Ag+ into AgNPs.74 Later, extracellular production of AgNPs by fungus and protozoan has also been observed.75–78 The biotic reduction mechanisms are complicated but can be divided into two categories: enzyme-mediated and non-enzyme-mediated reduction. AgNPs can be synthesized by enzymes, especially nitrate reductase.79,80 In the extracellular region of microorganisms, reductive O2˙− generated by oxidoreductases (e.g., NAD(P)H oxidases) is responsible for AgNP production.76 Besides, non-enzyme mediated reduction is also suggested because of the redox ability of extracellular polymer substances (EPS).52,81,82 Reducing saccharides containing hemiacetal groups and proteins containing cysteine, as components of EPS, can reduce Ag+ into AgNPs.81,83 However, most of these studies were conducted in pure culture systems; thus it is hard to extrapolate these conclusions to a real soil environment and to evaluate the contribution of microorganisms to natural generation of AgNPs.
In addition to microorganisms, root exudates of plants can promote in vitro AgNP formation at the root surface within a few hours.84–86 For example, the intact root surface of 11 diverse families of angiosperm plants shows potential to reduce Ag+ into AgNPs (5–50 nm) within 24 h, independent of microorganisms.86 Root exudates containing reducing sugars and low molecular weight (0–3 kDa) organic acids have been identified as electron donors for the light-induced formation of AgNPs.85 The released chlorine ions from the root surface could promote these processes by generating photoreactive semiconductor AgCl which is thereby converted to AgNPs under irradiation.61,84,85 With the presence of plant root exudates, the formation of AgNPs was observed to follow a pseudo-first-order (k = 0.3466 h−1)85 or a second-order kinetics reaction (k = 1.11 mM−1 h−1).84 Therefore, generation of AgNPs in the root zone will lead to direct exposure of plants and further AgNP uptake via roots.
3. Uptake of AgNPs in plants
The uptake of nanoparticles in plants has been regarded as their major entry point to food chains.30–32 Thus, it is essential to obtain mechanistic insights into the uptake processes. Progress has been made in elucidating the uptake mechanisms of AgNPs. Multiple influencing factors (e.g., exposure pathway, environmental conditions and plant species) have been demonstrated in Fig. 2.
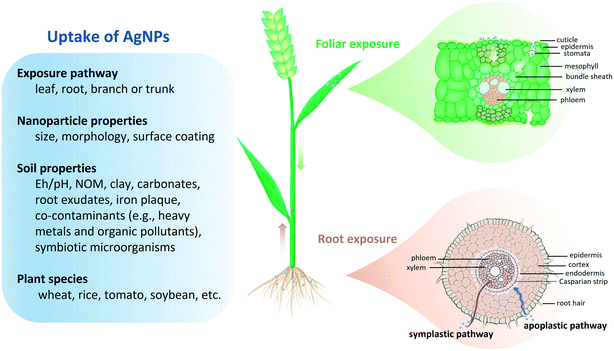 |
| Fig. 2 Schematic diagram of the uptake of AgNPs in plants and the influencing factors. | |
3.1 Uptake pathway of AgNPs
Foliar uptake and root uptake are two major uptake pathways of AgNPs in plants. Spraying agrochemicals directly onto plant leaves is an effective and economic approach in agricultural management.1,87,88 As nanopesticides, AgNPs are applied to fight against plant pathogens and insect pests through foliar exposure.17–19 Natural generation of AgNPs in soils, together with the disposal of Ag-containing sewage sludge or wastewater in agricultural soil, will lead to root exposure to AgNPs.85,89,90 Petiole feeding and trunk injection of nanoparticles are also applied in crop disease control but have not received much attention because of the limited applicable plants (normally trees).91
3.1.1 Foliar uptake.
Plants leaves can take up AgNPs via cuticular and stomatal pathways.92 The waxy hydrophobic cuticle has small polar pores (∼2 nm),93,94 suggesting that particles below 2 nm in size can penetrate directly through the cuticle into plant tissues. For larger particles, the uptake through stomatal openings is supposed to be feasible, because the estimated equivalent pore radius of stomata is larger than 20 nm.93 The observed stomatal size ranges from a few to tens of micrometers, varied between different plant species.95–98 The stomatal density (number of stomata per unit of area) is also dependent on the plant species and environmental conditions (e.g., atmospheric CO2 concentration and light intensity), in ranges of dozens to hundreds of pores per mm.99–102 Other leaf surface features, such as hydathode pores (a few to several μm) that allow direct access of nanoparticles to vasculature,103 and trichomes, although they have not yet been demonstrated as a route for nanoparticle uptake, may also play a role in nanoparticle internalization.104–106
Several studies have shown the presence of AgNPs in leaf stomata and other plant tissues with the aid of high-resolution microscopy (e.g., confocal laser scanning microscopy107 and transmission electron microscopy108), synchrotron-based X-ray imaging techniques (XAS),37 and single-particle inductively coupled plasma mass spectrometry (sp-ICP-MS).109 Using SEM and μ-XANES, Larue et al. observed that AgNPs (38.6 nm) aggregated into larger particles (2–3 μm) on the surface of lettuce (Lactuca sativa L.) leaves and small AgNPs were internalized in leaf tissues including the epidermis, mesophyll, and vascular tissues. They speculated that AgNPs can be entrapped by the cuticle and then enter the leaf through stomata.37 Recently, He et al. reported that stomata were the key to internalizing AgNPs into plant leaves.110 Whether the cuticular or stomatal pathway dominates the AgNP uptake through leaves still needs further investigation for different plant species. Cuticle thickness, stoma size, and density may control the uptake of AgNPs.105 Abundant stomata on leaves may allow rapid and efficient uptake of nanoparticles.111 For instance, an increased uptake of copper oxide nanoparticles (CuO NPs) (up to 6.8–fold) was recorded in wheat leaves with open stomata.112 With stomatal aperture diameter reduction (from ∼9 μm to ∼5 μm), the Zn concentrations in wheat leaf apoplast and cytoplasm decreased by 33.2% and 8.3% upon foliar exposure to zinc oxide nanoparticles (ZnO NPs), indicating the noticeable role of stomata in foliar exposure.113
Leaves can absorb AgNPs with size in the range of 10–40 nm (Table 1), but the precise size exclusion limits for AgNPs in different plants have not been quantitatively determined. The properties of both nanoparticles and the leaf surface regulate their interaction, as reflected in the uptake rate variation.105,114,115 In terms of other NPs, Hu et al. found that low surface tension (∼22 mN m−1) would allow rapid foliar uptake (<10 min) of carbon dots with hydrodynamic size larger than 2 nm.116 Kranjc et al. found that plants with a low foliar surface free energy (SFE) were more likely to retain platinum nanoparticles than plants with high SFE.117 Recently, Gao et al. reported that the SiO2 shell enhanced the uptake of ZnO NPs in tomato (Solanum lycopersicum) leaves while no Zn uptake was observed with pristine ZnO NPs, although the mechanisms were unknown.118 The classical Derjaguin-Landau-Verwey-Overbeek (DLVO) model91,119 or new empirical models such as nanoparticle-leaf interaction empirical models proposed by Hu et al.116 will be helpful to predict the interaction between nanomaterials and the plant leaf surface. Albeit with few publications, systematic investigation on the role of plant leaf physiology (for instance, hydrophobicity/hydrophilicity and roughness) in nanoparticle uptake is needed to determine the factors that control the interaction between AgNPs and the leaf surface and to investigate the uptake mechanisms.
Table 1 Summary of studies on AgNP uptake, translocation, accumulation, and toxic effects in plants
Nanoparticles |
Initial size/nm |
Surface potential/mV |
Plant species |
Exposure condition |
Uptake rates |
Uptake rate constants |
TFshoot/root |
Translocation and accumulation |
Observed toxicity |
Ref. |
“—” represents information is not available.
|
PVP-AgNWs |
43 nm diameter × 1.8 μm length |
— |
Lettuce (Lactuca sativa L.) |
Root exposure, hydroponic, 0.086 mg L−1, 18 d |
|
0.136 ± 0.006 d−1 (particulate Ag) |
0.010 ± 0.001 |
— |
Inhibited the growth of plants significantly with EC50 values ranging from 0.32 to 0.49 mg L−1 |
124
|
PVP-AgNPs |
13.4 ± 4.9 |
−19.1 ± 1.9 |
Wheat (Triticum aestivum L.) |
Root exposure, hydroponic, 0.1–10 mg L−1, 8 h |
— |
4.3 ± 0.6 to 5.2 ± 0.6 L kg−1 h−1 (particulate Ag) |
— |
Translocated from roots to shoots |
— |
126
|
PVP-AgNPs |
14.5 ± 0.7 |
−18.4 ± 2.1 |
Wheat (Triticum aestivum L.) |
Root exposure, hydroponic, 2–30 mg L−1, 8 h |
8.0–39.0 mg kg−1 h−1 (particulate Ag) |
1.1 ± 0.1 L kg−1 h−1 (particulate Ag) |
— |
Aggregated and accumulated in the cytoplasm of root apical meristem cell |
Destroyed plasma membrane integrity and caused cell death in root tips |
123
|
PVP-AgNPs |
28.3 |
−31.5 |
Green alga (Chlorella vulgaris) |
Hydroponic, 0.5–5.0 mg L−1 |
— |
0.233 ± 0.024 L g−1 d−1 (particulate Ag) |
— |
Localised in starch granules within the chloroplast of C. vulgaris |
IC50 value of 9.3 ± 0.1 μg L−1 |
138
|
PEG-AgNPs |
22.0 |
−21.0 |
Green alga (Chlorella vulgaris) |
Hydroponic, 0.5–10 mg L−1 |
— |
0.339 ± 0.020 L g−1 d−1 (particulate Ag) |
— |
Localised in starch granules within the chloroplast of C. vulgaris |
IC50 value of 49.3 ± 5.2 μg L−1 |
138
|
Cit-AgNPs |
22.0 |
−31.6 |
Green alga (Chlorella vulgaris) |
Hydroponic, 0.5–4.0 mg L−1 |
— |
0.246 ± 0.031 L g−1 d−1 (particulate Ag) |
— |
Localised in starch granules within the chloroplast of C. vulgaris |
IC50 value of 9.2 ± 1.0 μg L−1 |
138
|
PVP-AgNPs |
12.2 ± 3.1 |
−18.8 ± 1.8 |
Rice (Oryza sativa L.) |
Root exposure, hydroponic, 10 mg L−1, 2 d |
— |
— |
0.0013 ± 0.0044 |
Translocated from roots to shoots |
— |
188
|
PVP-AgNPs |
9.37 ± 1.83 |
−24.5 ± 0.3 |
Rice (Oryza sativa L.) |
Root exposure, hydroponic, 2–20 mg L−1, 5 d |
— |
— |
0.0024–0.0123 |
Translocated from roots to shoots. Accumulated in the middle lamella of roots |
— |
189
|
PVP-AgNPs |
10 |
— |
Soybean (Glycine max) |
Root exposure, hydroponic, 1 mg L−1, 7 d |
— |
— |
0.05 |
Translocated from roots to stems and leaves |
Decreased transpiration rates, mediated oxidative stress, increased ROS, and MDA contents |
194
|
AgNPs |
15–20 |
−7.54 |
Cowpea (Vigna unguiculata L.) |
Root exposure, hydroponic, 0.6 mg L−1, 14 d |
— |
— |
0.0047 (cowpea) |
Translocated from roots to shoots |
Decreased root elongation rates and fresh biomass |
209
|
Wheat (Triticum aestivum L.) |
0.0134 (wheat) |
PVP-AgNPs |
12.2 ± 3.8 |
−9.25 ± 0.19 |
Rice (Oryza sativa L.) |
Root exposure, hydroponic, 1 mg L−1, 4 h |
34.4 mg kg−1 h−1 (total Ag) |
— |
— |
— |
— |
219
|
AgNPs |
20 |
−15.4–−9.5 |
Lettuce (Lactuca sativa L.) |
Root exposure, hydroponic, 0.1–10 mg L−1, 15 d |
— |
— |
0.009–0.037 |
Translocated from roots to shoots |
Decreased fresh biomass of roots and shoots |
255
|
Increased the content of O2− and MDA in shoots and H2O2 in roots |
PVP-AgNPs |
10 |
— |
Wheat (Triticum aestivum L.) |
Seed exposure, 1–10 mg L−1, 5 d |
— |
— |
0.038 |
Accumulated in the cell wall surface in the outer cells of the root apex |
Reduced length and fresh weight of roots and shoots |
287
|
PVP-AgNPs |
20 |
−8.8 ± 0.65 |
Tomato (Lycopersicon esculentum) |
Root exposure, hydroponic, 10–30 mg L−1, 7 d |
— |
— |
0.38–0.62 (particulate Ag) |
Translocated form roots to shoots and leaves |
Upregulated membrane transporters H+-ATPase, potassium transporter, and sulfate transporter |
283
|
0.75–0.81 (total Ag) |
Aggregated in root cell wall |
Resulted in larger xylem cells |
3.1.2 Root uptake.
After reaching the root surface, smaller AgNPs (<40 nm) can directly pass through the structure of the cell wall,107,120,121 while larger AgNPs may enter the cytoplasm through endocytic uptake, pore formation or wounds,122 but direct evidence is still lacking. It is noteworthy that the size limitation of uptake should be determined by the minimal size of AgNPs in the experimental suspensions but not the average diameter.
A fundamental issue is to differentiate the relative contribution of particles and dissolved ions during root uptake because AgNPs may dissolve in the experimental medium. A variety of methods have been used to prove the direct uptake of AgNPs.123–126 Conventional experiments were conducted by exposing individual plants to AgNPs or Ag+ ions separately. Some studies reported that plants exposed to AgNPs accumulated higher content of Ag compared to those exposed to Ag+ at the same dosages,127,128 while others found that there is more Ag in plant tissue upon exposure to Ag+ and it was the ionic Ag released from the dissolution of AgNPs that were responsible for the uptake.129,130 These contradictory results may be explained by the interference of the possible AgNP dissolution in the exposure medium or at the biological interface. Using dual stable isotope tracing (107AgNO3 and 109AgNPs), Yang et al. confirmed the direct uptake of AgNPs and found that AgNPs were the main Ag species accumulated in rice roots when they were exposed to particles and ions at 50 μg L−1 simultaneously for 14 days.125 They suggested that Ag+ ions mainly located on the root surface, possibly due to the combination of free Ag+ ions with hard and soft ligand residues on the cell wall (e.g., hydroxyl, carboxyl, amino, and thiol groups), thus limiting the internalization of Ag+ ions. Wu et al. also found that the uptake of particles denoted as PVP-coated silver nanowires (AgNWs, 43 nm diameter × 1.8 μm length) accounted for more than 85% of accumulated Ag in lettuce for 18-day exposure.124 Our group found that the direct uptake of AgNPs by 15-day wheat seedlings dominated relative to dissolved Ag uptake when the dissolution of AgNPs was less than 1.2%, suggesting that the relative contribution of particulate uptake varies as a function of AgNP dissolution.126
3.2 Uptake kinetic model
In recent decades, empirical biodynamic models have been developed to describe the uptake kinetics of nanoparticles in organisms (especially invertebrates).131–137 To date, the uptake kinetic models of AgNPs have only been applied to a few plants, including wheat,123,126 lettuce,124 and green alga.138 The linear relationship of accumulated Ag concentration in plant tissues and exposure time was observed in these studies. Thus, a kinetic model for AgNP uptake in plants can be simplified as follows:124,126
d[Ag]plants/dt = ku,total × [Ag]total |
ku,total × [Ag]total = ku,NP × [Ag]NP + ku,diss × [Ag]diss |
where ku,total, ku,NP, and ku,diss represent the overall uptake rate constants of AgNP suspensions, nanoparticles and dissolved Ag+, respectively; [Ag]total, [Ag]NP, and [Ag]diss represent the concentration of total Ag, AgNPs and dissolved Ag+ in the exposure medium, respectively. ku can be calculated as the slope of uptake rates and the corresponding exposure concentrations.139 In this model, the elimination rate of Ag is assumed to be negligible for higher plants. This dynamic model can distinguish the contribution of nanoparticles' uptake and dissolved ions' uptake and is suitable for higher plants. Given the possible elimination process, Ribeiro et al. and Zhang et al. suggested an empirical model for the uptake and elimination of AgNPs in algae:140,141
Uptake: CAlgae(t) = ku/ke × Cmedia × (1 − e(−ke×t)) |
Elimination: CAlgae(t) = ku/ke × Cmedia × (1 − e(−ke×(t−tc)) − e(−ke×t)) |
where CAlgae(t) is the silver concentration in the algae at time t; ku and ke represent the uptake rate constant and elimination rate constant of total silver, respectively; Cmedia is the silver concentration in the exposure media.
The uptake rate constants will help to predict the uptake tendency of different Ag species in plants. Although there are several concerns over the application of uptake kinetic models, for example, whether the uptake rate constants obtained from simplified hydroponic experiments can be used to predict the plant uptake of AgNPs in complex soil matrices, these results are a first step towards the development of a dynamic modeling approach to assess the uptake of AgNPs in plants.
3.3 Factors affecting uptake processes
The physicochemical properties of both particles and soil govern the fate of metal nanoparticles in plant-soil systems. In most studies, hydroponic experiments were conducted to investigate the effect of a certain factor, which have provided a foundation to explore plant uptake of nanoparticles in more complex realistic soil matrices.
3.3.1 Properties of AgNPs.
The physicochemical properties of nanoparticles, for instance, size, morphology, surface coating, and charge, affect their interaction with biological surfaces. Particle size is a crucial factor as smaller nanoparticles have a higher tendency to be internalized by plants.34,38 More Ag accumulated in plant tissues after exposure to smaller particles than larger particles at the same dosage.142,143 For example, poplars (Populus deltoides × nigra) accumulated more Ag upon exposure to 5 nm AgNPs than 25 nm AgNPs (0.1 mg L−1) after 7-day hydroponic exposure.143 Torrent et al. found that lettuce (Lactuca sativa L.) roots accumulated more Ag upon exposure to AgNPs with small size (75 nm PVP-AgNPs and 60 nm citrate-AgNPs) than larger size (100 nm PVP- and citrate-AgNPs).144 Similar results were observed for other metal nanoparticles (e.g., CeO2 NPs and ZnO NPs) in different plants (e.g., cucumber and common bean), indicating that nanoparticles with smaller size are more bioavailable to plants.92,145–147 However, size-specific dissolution of AgNPs has been reported, with greater dissolution for smaller particles.148,149 Thus, higher Ag content in plant tissues upon exposure to smaller AgNPs may be ascribed to the direct uptake of particles as well as the released Ag+.150,151
Coating changes particle surface properties (e.g., surface charge and hydrophobicity/hydrophilicity) to provide electrostatic repulsion between individual nanoparticles to prevent aggregation, which also makes nanoparticles more mobile and more phytoavailable than bare nanoparticles.152–154 Frequently used coating agents in AgNP synthesis include polyvinylpyrrolidone (PVP), gum Arabic (GA), citrate, cetyltrimethylammonium bromide (CTAB), etc.155–157 Surface coating modulates the interaction between nanoparticles and biological surfaces, which affects the potential uptake of nanoparticles.158–160 Due to the negative charge in the root surface,161,162 positively charged nanoparticles are more adhesive to the root surface than negatively charged nanoparticles.119,163,164 For instance, AgNPs modified with a cationic stabilizer such as CTAB (5.6 nm, zeta potential (ζ) = 42.5 mV) can induce more Ag uptake in onion (Allium cepa L.) roots compared to citrate-AgNPs (61.2 nm, ζ = −39.8 mV) and PVP-AgNPs (9.4 nm, ζ = −4.8 mV) after 72 h of hydroponic root exposure.157 The silver content in the leaves of tobacco (Nicotiana tabacum L.) exposed to 100 μM CTAB-AgNPs (56 nm, ζ = 44.67 mV) for 7 days was 8.84 ± 1.33 μg g−1, higher compared to those exposed to citrate-AgNPs (24 nm, ζ = −23.78 mV) (3.65 ± 0.9 μg g−1).165 The uptake rate constants in green algae were similar for PVP-AgNPs (0.233 L g−1 d−1) and citrate-AgNPs (0.246 L g−1 d−1), corresponding to their similar zeta potential (−31.5 mV vs. −31.6 mV), which are also reflected in semblable toxicity.138 A recent study found that the uptake rate constants of polyethylene-coated AgNPs (positively charged) (9.56–13.28 L g−1 h−1) were ∼20 times higher than those of negatively charged AgNPs (negatively charged) (0.36–0.55 L g−1 h−1) for freshwater algae (Chlorella vulgaris).141 Similarly, the uptake rate of CdSe/CdZnS quantum dots with cationic coating was 10 times faster than those with anionic coatings, possibly due to electrostatic attraction to the negatively charged root cell wall.166 Recently, Yan et al. reported that the uptake pathway of AgNPs in a freshwater phytoplankton (Chlamydomonas reinhardtii) depended on the surface coating. Citrate-AgNPs (ζ = −23.75 mV) were internalized mainly through the apical zone of the cell near the flagella, whereas the aggregation-induced emission fluorogen-coated AgNPs (ζ = −46.3 mV) were internalized through endocytosis.167 These findings indicate the critical role of surface coating in the uptake processes of AgNPs in plants.
Multiple morphologies of AgNPs are created in engineered applications including spherical silver nanoparticles,168 silver nanocubes,169 and silver nanowires.170 Besides, naturally formed AgNPs in soil are nearly spherical.62 Studies mainly focused on physiological alterations of plants,171–173 ignoring underlying variations in uptake and accumulation of AgNPs with different morphologies. Morphology-dependent impacts on organisms and communities (including algae and bacteria) have been observed in a lotic ecosystem, highlighting the ecological risk of Ag nanoplates.174 Toxicity differences in ryegrass (Lolium multiflorum) may be ascribed to the increased uptake of a certain morphology of AgNPs.171 Thus, AgNPs may display morphology-based uptake patterns in plants, which has not been explored yet.
Regulation of AgNP uptake in plants becomes possible through manipulating particle properties, especially surface charge. However, although the crucial role of particle properties has been proposed based on laboratory investigation, whether these pristine characteristics have a vital effect on the realistic uptake remains unclear, due to the possible physical and chemical transformations of nanoparticles in natural environments. A recent study based on machine learning found that the composition and size of nanoparticles were the major parameters affecting the root concentration factor (the ratio of nanoparticle concentrations in root versus growth medium) in hydroponic systems, while soil organic matter and clay contents were the most influential ones in soil systems.175 The uptake dependencies of AgNPs on size and surface charge may be weaken by the agglomeration process and over-coating of NOM under realistic environmental conditions.176 Hence, the interplay between nanoparticles and surrounding environments should be fully investigated when extrapolating the results from hydroponic experiments to soil systems.
3.3.2 Environmental factors.
In a typical plant-soil system, the interaction between AgNPs and plants will be affected by multiple environmental factors. Once they entered the soil, AgNPs are retained by soil components or undergo biotic and abiotic processes. Soil physical-chemical properties play a major role in the mobility of AgNPs.177–181 The soil-water distribution coefficient (KD) values of citrate-AgNPs increased from 100 to 10
000 L kg−1 between pH 4 and 8, indicating the strong soil sorption capacity of AgNPs.182 Upon root exposure to 0.15 mg kg−1 AgNPs for 8 days, high Ag fluxes and accumulated Ag were observed in tall fescue (Festuca arundinacea) that grew in loam soil with high carbonate content, whereas the lowest Ag uptake was observed in plants grown in clay loam,183 probably due to the preferential adsorption of surface-charged AgNPs on the oppositely charged sites of clay minerals.184,185
The maximum retention capacity (Qmax = 0.38–0.55 mg g−1), the retained amount of AgNPs on soil, was also positively correlated to iron oxide content.186 For wetland plants, the iron plaque layer formed in the root surface can act as a barrier to inhibit the direct uptake of nanoparticles.187 Decreasing bioconcentration factors (BCFs) of AgNPs were observed for rice (Oryza sativa L.) with an increasing amount of iron plaque on the root surface.188 By contrast, another study found that iron plaque oxidized AgNPs into AgCl and Ag+-thiol complexes, and thus increased Ag uptake in rice seedlings.189 Such contrasting effects of iron plaque were ascribed to its influence on the dissolution of AgNPs. The iron plaque generated in the rhizosphere is a mixture of (oxyhdr)oxides, mainly composed of ferrihydrite, goethite, and lepidocrocite.190,191 Our recent study found that electrostatic attractions between AgNPs and iron oxides (goethite and hematite) led to heteroaggregation of nanoparticles and thus significantly inhibited the dissolution of AgNPs.192 Despite its effects on dissolution, whether iron plaque can act as a physical barrier to inhibit the direct uptake of AgNPs remains to be answered.
Co-existing heavy metals in natural environments potentially influence the uptake of AgNPs. For instance, Cu pre-exposure (0.5–2.5 mg L−1 for 4 days) reduced AgNP uptake rates by 1.3–3.9 times in wheat seedlings, probably due to the cell death in root tips and the decline of non-protein thiols in roots.193 Cao et al. found that the co-exposure of AgNPs (1 mg L−1) and Sb(V) (100 mg L−1) led to significantly higher Ag accumulation in roots, stems and leaves of soybean and speculated that Sb(V) could lead to oxidative dissolution of AgNPs to release more bioavailable Ag+.194 The mutual impacts of AgNPs and heavy metal can be attributed to various physical, chemical and biological processes in the rhizosphere, which ultimately lead to different plant uptakes of both substances.193–196 In agroecosystems, research efforts have also been focused on the co-exposure of nanomaterials with organic contaminants (e.g., organic pesticides and fertilizers).197–202 Co-exposure of organic pollutants can affect the uptake of AgNPs in plants. For example, the accumulated Ag in aerial tissues of zucchini (Cucurbita pepo) exposed to AgNPs alone was 85.4% higher than plants co-exposed to AgNPs and imidacloprid, suggesting that the uptake of AgNPs was suppressed by imidacloprid co-exposure.203 Li et al. found that diclofop-methyl, a common post-emergence herbicide, reduced Ag+ release from AgNPs and thus, the Ag content in shoots and roots of Arabidopsis thaliana was reduced by 15.2% and 9.4% with the presence of 1 mg L−1 diclofop-methyl.198 Therefore, the interaction of AgNPs with coexisting contaminants should be considered for risk assessments in agroecosystems.
In soils, AgNPs will undergo oxidative dissolution and transform into more stable silver complexes such as Ag2S, which was less bioavailable due to its low solubility.29,177,204,205 In flooded soil, most of the Ag was transformed into Ag2S (>95%) within 2 days, due to the decreasing soil Eh.46 Decreased bioavailability of AgNPs with a Ag2S shell has been demonstrated.206–208 The silver bioconcentration factor for ryegrass was 0.061 in soil amended with pristine AgNPs, compared to 0.003 in soil with sulfidized AgNPs.90 Higher Ag content in roots of rice, lettuce, alfalfa, and wheat has been observed upon exposure to AgNPs than Ag2S NPs, which can be explained by the stronger dissolution ability and smaller particle size of the used AgNPs.188,209–211 However, Niu et al. found that sulfate-reducing bacteria (SRB) transformed AgNPs (20 nm) into Ag2S NPs with smaller particle sizes (<10 nm), thus leading to higher concentration of silver-containing nanoparticles with smaller size in the roots and stems of Scirpus triqueter.212 These results highlighted the bioavailability of Ag2S at the nanoscale.89,209,213
Natural organic matter significantly affects the dissolution of nanoparticles.214–218 For example, the thiol group of NOM was effective in reducing AgNP bioavailability by inhibiting particle dissolution.219 A negative relationship was observed between the NOM concentration and AgNP uptake rate for rice (Oryza sativa L.) seedlings: the uptake rate of total Ag decreased from 34.4 to 13.6 mg kg−1 h−1 with increasing NOM concentration from 0 to 79.8 mg L−1.219 Additional humic acids (HA) (10–100 mg L−1) inhibited particle dissolution and reduced Ag accumulation in the roots and leaves of duckweed (Lemna minor L.) exposed to 100 mg L−1 AgNPs for 15 days.220 A recent study found that HA adsorption changed the surface properties of nanoparticles, including the effective size, hydrophobicity, and surface charge.221 NOM may associate with the nanoparticle surfaces, acting as a coating agent to lower the zeta potential of AgNPs, and thus reduce their contact with plant roots via charge repulsion.220,222 In the aquatic environment, HA can complex with AgNPs via carboxylate groups and C–O and C–O–C bonds.220 Yang et al. reported that a NOM-corona can be formed on the AgNP surface within 20 minutes, following a two-step (i.e., fast and slow processes) first-order adsorption model.223 The adsorption equilibrium constants of HA on bare-, citrate-, and PVP-AgNPs were 0.18 ± 0.01, 0.35 ± 0.03, and 0.80 ± 0.18 L mg−1 C, respectively, implying the high affinity of HA toward AgNPs.223 The corona formed by adsorption of diverse biomolecules (e.g., NOM, proteins, and metabolites) on the surface of nanoparticles will influence the retention and plant uptake of AgNPs, as well as other nanoparticles.224,225 For instance, in sandy soil poor in organic matter, Ce fluxes in fescue (Festuca arundinacea) were ∼2.1 times higher (6.6 ± 0.7 ng m2 s−1) following exposure to 1 mg kg−1 citrate-coated CeO2 NPs compared to those exposed to bare CeO2 NPs.154 However, when plants were cultivated in clay soil rich in organic matter, similar phytoavailability of bare and coated CeO2 NPs was observed, indicating that the potential coating of NOM could change the mobility and phytoavailability of nanoparticles.154,226–228 Given that the composition and physicochemical properties of NOM vary greatly as a function of the origin and maturity level of the materials,215,229 the heterogeneity of NOM makes the assessments that are based on the average characteristics of unfractionated NOM unrepresentative to describe interactions between specific components and nanoparticles.230,231
Additionally, the eco-coronas formed at the nanoparticle surface have different molecular components because of the incredible diversity of biomolecules in the soil environment.224 Plant-derived biomolecules (e.g., organic acids, amino acids, and phenolics) in root exudates can also be adsorbed onto particle surfaces to influence the stability of nanoparticles.232,233 For example, a lower zeta potential (−28.7 ± 0.9 mV vs. −14.3 ± 0.7 mV), decreased metal ion release rate (0.002% h−1vs. 0.016 % h−1), and smaller particle size (146.3 ± 17.9 nm vs. 5919.0 ± 696.5 nm) of Mn3O4 NPs were observed in the soybean root exudate medium than in pure water after 5 days of incubation, indicating that the surface charge, dissolution, and aggregation state of NPs were considerably dependent on the constituents of the exposure medium.234 Given that the constituents of root exudates vary with different plants, long-term root exposure under more realistic field conditions is required to decipher the role of root exudates in the fate of nanoparticles.
Although studies have revealed the impacts of AgNPs on soil and plant-associated microbial communities,235–242 the role of a diverse array of symbiotic microorganisms (including rhizosphere and phyllosphere microbes) in the transformation and uptake of AgNPs in plants is largely unknown. Studies revealed that arbuscular mycorrhizal fungi, which form a symbiotic relationship with over 90% of terrestrial plants, could alleviate AgNP stress (via increased growth and nutrient uptake, etc.) and thus decrease Ag uptake and accumulation in plants.243–245 Noori et al. found that tomatoes inoculated with mycorrhizal fungi (Rhizophagus intraradices) accumulated 14% less Ag compared to non-mycorrhizal tomatoes upon exposure to 36 mg kg−1 of 2 nm AgNPs for 2 weeks, and suggested that mycorrhizal colonization could decrease Ag accumulation via downregulating the expression level of membrane transport proteins.246 The mutual interactions between mycorrhizal fungi and AgNPs are complex because of the large variation in plant and fungal species, as well as the changeable environmental factors.247 Besides, little is known about whether phyllosphere microbial communities (as well as their metabolites) could affect the foliar uptake of AgNPs. Thus, more research is needed to understand how microbes influence nanoparticle uptake in plants in a typical plant–soil system.
3.3.3 Uptake variation between plant species.
Different uptakes of AgNPs were observed in a variety of plant species.36,248,249 The AgNP uptake rates of 15-day wheat (Triticum aestivum L.) seedlings increased from 8.0 to 39.0 mg kg−1 h−1 upon hydroponic exposure to 2 to 30 mg L−1 AgNPs.123 Compared with wheat, 15-day rice (Oryza sativa L.) seedlings had a higher total Ag uptake rate (34.4 mg kg−1 h−1) even at a lower exposure dosage (1 mg L−1 AgNPs).219 The Ag contents in wheat roots were several times higher than those in sunflower roots, suggesting different AgNP uptake potential between monocotyledon and dicotyledon.250 The uptake rate constant of AgNPs of algae (Chlorella vulgaris) (0.233 ± 0.024 L g−1 d−1)138 was higher than of wheat (1.1 ± 0.1 L kg−1 h−1),123 indicating lower AgNP phytoavailability for higher plants. The uptake of other nanoparticles (e.g., CeO2) in plants was also suggested to be species dependent.251 For a given plant, plant age has a minor influence on the uptake of AgNPs because the overall uptake rate constants of 15- and 30-day wheat seedlings were similar (4.3 ± 0.6 L kg−1 h−1vs. 5.2 ± 0.6 L kg−1 h−1),126 but a long-term experiment is required to quantify the uptake at different life stages.
The root surface area and number of lateral roots affect the sensitivity of plants to nanoparticles.187,252 Differences in leaf surface properties (e.g., leaf area, size of stomata, and cuticle thickness) give plants different capacities to take up nanoparticles.92,119,253 These morphological and physiological features are modulated by environmental factors and will change at different growth stages, which consequently change the nanoparticle uptake ability of plants. Many studies were carried out at the initial stage of plant growth, which cannot represent the real behavior of AgNPs in the full life cycle and may exaggerate the adverse effects of AgNPs. In addition, experiments were usually done with small annual plants, such as Arabidopsis, lettuce, rice, wheat, tomato, etc.107,123,211,254,255 The different anatomical features of plant species may result in diverse uptake and translocation patterns. For example, after 48 h of hydroponic exposure to 50 mg Ce per L as CeO2 NPs, Ce existed throughout the leaf of dicot plants (tomato and lettuce) but was mainly located in the vasculature of monocot plants (corn and rice), probably due to the larger airspace volume in dicot leaves.163 The anatomical structure of plant tissues as well as the metabolic processes will change under biotic and abiotic stimuli or stresses (e.g., plant diseases and insect pests, flooding, drought, soil salinity and extreme temperatures),256–258 and thus affect the uptake of nanoparticles. Rossi et al. found that the cerium concentration in leaves of Brassica napus L. was 150% higher in salt stressed (100 mM NaCl) plants than unstressed plants upon exposure to 200 mg kg−1 CeO2 NPs, although the mechanism is unclear.259 Therefore, field trials evaluating the responsive AgNP uptake behaviors of plants under more climatically relevant conditions are needed.1
Studies have observed the toxic effects of AgNPs on plants, such as inhibiting seed germination, reducing biomass, and decreasing transpiration rates (Table 1), and have revealed the underlying mechanisms of AgNP phytotoxicity, including oxidative stress, cytotoxicity and antioxidative responses.260–262 Omics-based approaches offer new opportunities to investigate the molecular mechanisms of nanoecotoxicology, and have succeeded in identifying certain responses that point to potential toxicity pathways and to action modes of nanoparticles.263–265 Recently, Huang et al. observed 21 dysregulated metabolites in corn leaves and 53 in wheat leaves after 3 weeks of root exposure to 200 and 1000 mg MoO3 NPs per kg vermiculite, and demonstrated the value of metabolomics in studying early-stage plant responses to nanoparticle exposure.266 Thus, using multi-omics techniques, a thorough understanding of plant responses to AgNP exposure can also be achieved to optimize both functionality and safety in AgNP application.
4. Translocation and accumulation of AgNPs in plants
Nanoparticles internalized by roots or leaves can be transferred from the exposed surfaces to the vascular system through the apoplastic or symplastic pathway. AgNPs were observed in epidermal cells,252 columella cells,210 or meristem cells in roots,123 typically in the cell wall,33,107,267,268 which serves as a barrier between particles and the protoplast. After crossing the pores of the cell wall, AgNPs can move into the intercellular space between the cell wall and plasma membrane through the apoplastic pathway,269,270 verified by the existence of AgNPs in the middle lamella (i.e., intercellular layer).125,189,268,271 After passing through the epidermis and the cortex via the apoplastic pathway, nanoparticles will be blocked by the Casparian strip, a special structure in the endodermis, but some can penetrate the plasma membrane and enter the cytoplasm of endodermal cells via the symplastic pathway.272–274 Once inside the cells, AgNPs can be transported symplastically through plasmodesmata,107,123 the narrow strands of cytoplasm with 30–50 nm in diameter that penetrate cell walls to interconnect adjacent cells.275–278 The direct cell-to-cell transport through the symplastic pathway can deliver AgNPs to the vascular bundles, the main transportation system throughout the whole plant.107,279,280 In the vascular bundles, AgNPs could be transported upward (from roots to stems or leaves) through the xylem or downward (from leaves to roots) through the phloem.281,282 The perforation plate of the vessel and sieve plate of the sieve tube play a crucial role in the translocation of nanoparticles as they inhibited the transportation of most PVP-AgNPs and citrate-AgNPs but not GA-AgNPs.91
Translocation factors (TFshoot/root, calculated as the ratio of Ag level in shoots to that in roots) differed with particle properties and plant species (Table 1). Small Ag particles tended to transfer upward more feasibly (TFshoot/root = 0.75 ± 0.06 for small particles (20 nm) vs. 0.06 ± 0.007 for bulk Ag) in tomato that were exposed to 10 mg L−1 Ag for 7 days.283 Souza et al. also found that the smallest AgNPs (30 nm), rather than larger AgNPs (85 and 110 nm), could translocate from roots to leaves of Lemna minor.150 The translocation of nanoparticles in plants is also partially governed by their surface properties such as surface charge. For example, several studies have reported that negatively charged nanoparticles had higher TFshoot/root, indicating that these nanoparticles are more advantageous to transport from roots to shoots.163,164,284,285 The TF values in cucumber (Cucumis sativus) exposed to the polyacrylic acid-CeO2 NP (negative charged) group were significantly higher than those exposed to the chitosan-CeO2 NP (positive charged) group at equivalent dosage (50, 200, and 1000 mg L−1) for 14 days.286 Silver content in citrus leaves ranked the highest with branch feeding GA-AgNPs (71.48 ± 108.81 μg kg−1) compared with PVP-AgNPs (58.71 ± 69.15 μg kg−1) and citrate-AgNPs (34.48 ± 27.05 μg kg−1), which indicates that GA coating may enable high transport efficiency for nanoparticles.91 The translocation of AgNPs within plants is also modulated by the internal environment of plants.143,189,287 Generally, the TFshoot/root of total Ag is less than 0.05 in most plants such as wheat (0.013–0.038),34,209,287 soybean (0.017–0.05),34,194 rice (0.001–0.012),188,189 and lettuce (0.009–0.037)124,255 (Table 1). The internalized AgNPs were found to be hard to transport in some plant species. For example, less than 1% of AgNPs were transferred from alfalfa (Medicago sativa) roots to shoots after 6-day root exposure.210 Galazzi et al. found that about 82.5% and 17.2% Ag were maintained in roots and stems while only 0.2% was transferred to leaves of soybean (Glycine max) after exposure to 50 mg kg−1 AgNPs for 14 days.288 Fernandes et al. observed Ag accumulation mainly in belowground tissues of a salt marsh plant, Phragmites (Phragmites australis), after exposure to 10 mg L−1 AgNPs for 7 days.242 On the contrary, high TFshoot/root values of particulate Ag (0.38–0.62) were observed in tomato exposed to 10–30 mg L−1 AgNPs for 7 days.283 For ligneous plants, such as downy oak (Quercus pubescens Willd.), scots pine (Pinus sylvestris L.) and black poplar (Populus nigra L.), more than 50% of the total Ag was accumulated in the stem when exposed to foliar treatment.281 It should be mentioned that, due to the limitation of analytical methods, the TFshoot/root values calculated in most studies were based on the concentrations of total Ag instead of those of AgNPs, which may deviate from the actual translocation of nanoparticles inside plants.
Multi-scale techniques are integrated to trace the distribution and translocation of nanoparticles in plants, such as autoradiography,289 γ-spectrometry,289 surface-enhanced Raman spectroscopy,290,291 laser ablation-single particle-inductively coupled plasma mass spectrometry,292 and synchrotron-based techniques.209 The knowledge on the tissues where AgNPs accumulated is crucial to evaluate the possibility of these nanoparticles to enter food chains. However, few studies have investigated the accumulation of AgNPs in edible parts of plants (e.g., grains and fruits). Although the accessibility of these analytical platforms maybe limited, much work is still required involving the dynamic uptake and translocation of nanoparticles in plants over the life cycle (particularly the maturation stage).
5. Transformation of AgNPs in plants
Within plants, AgNPs will undergo physical or chemical transformation such as aggregation, oxidative dissolution, chlorination, sulfidation and complexation with organic ligands (Fig. 3). Studies have reported that internalized AgNPs aggregated into larger nanoparticles in different plants, including soybean,33 rice,188 wheat,123 lettuce,293 tomato,283 and Arabidopsis,268 while others found that AgNPs were subjected to a range of biochemical reactions, leading to the accumulation of transformed products (Table 2).
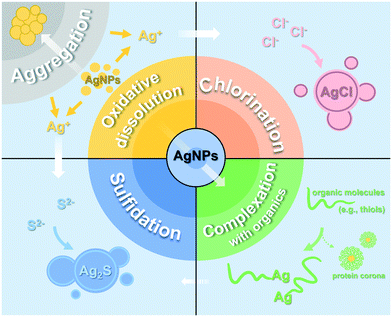 |
| Fig. 3 Schematic diagram of the possible transformation of AgNPs in plants. | |
Table 2 Summary of studies on transformation mechanisms of AgNPs and observed metal species in plants
Nanoparticles |
Plant species |
Exposure pathway |
Qualitative analysis techniques |
Analyzed samples |
Transformation |
Main transformed products |
Ref. |
PVP-AgNPs |
Rice (Oryza sativa L.) |
Root |
sp-ICP-MS |
Leaves |
Aggregation |
AgNPs with larger size (18.6 nm vs. 41.5 nm) |
33
|
PVP-AgNPs |
Soybean (Glycine max) |
Root |
sp-ICP-MS |
Leaves |
Aggregation |
AgNPs with larger size (18.6 nm vs. 30.2 nm) |
33
|
Uncoated AgNPs |
Lettuce (Lactuca sativa L.) |
Foliar |
μXRF |
Leaves |
Aggregation |
Ag agglomerates (38.6 nm vs. 2–3 μm on the leaf surface) |
37
|
PVP-AgNPs |
Wheat (Triticum aestivum L.) |
Root |
sp-ICP-MS |
Roots |
Aggregation |
AgNPs with larger size (15.6 nm vs. 32.9 nm) |
123
|
PVP-AgNPs |
Wheat (Triticum aestivum L.) |
Root |
sp-ICP-MS |
Roots, shoots |
Aggregation |
AgNPs with larger size (13.4 nm vs. 29.1 nm (roots), 34.3 nm (shoots)) |
126
|
PVP-AgNPs |
Rice (Oryza sativa L.) |
Root |
sp-ICP-MS |
Roots, shoots |
Aggregation |
AgNPs with larger size (12.2 nm vs. 24.9 nm (roots), 27.8 nm (shoots)) |
188
|
Citrate-AgNPs |
Arabidopsis (Arabidopsis thaliana) |
Root |
sp-ICP-MS |
Roots |
Aggregation |
AgNPs with larger size (12.8 nm vs. 20.7 nm) |
268
|
PVP-AgNPs |
Tomato (Lycopersicon esculentum) |
Root |
sp-ICP-MS |
Roots, stems, leaves |
Aggregation |
AgNPs with larger size (20 nm vs. 41 nm (roots), 30 nm (stems), 25 nm (leaves)) |
283
|
PVP-AgNPs |
Lettuce (Lactuca sativa L.) |
Foliar, root |
sp-ICP-MS |
Leaves |
Aggregation |
AgNPs with larger size (16.5 nm vs. 27.6–32.5 nm (foliar exposure), 29.8–36.5 nm (root exposure)) |
293
|
PVP-AgNPs |
Rice (Oryza sativa L.) |
Foliar |
TEM-EDS |
Leaves |
Oxidative dissolution, chlorination |
AgCl NPs (33.0–48.9 nm) |
33
|
Uncoated AgNPs |
Lettuce (Lactuca sativa L.) |
Foliar |
μ-XANES |
Leaves |
Oxidative dissolution, chlorination, complexation with organics |
Epidermis: Ag–glutathione (31–85%), AgCl |
37
|
Parenchyma: Ag–glutathione (100%) |
PVP-AgNPs |
Rice (Oryza sativa L.) |
Root |
LC-ICP-MS |
Shoots |
Oxidative dissolution |
Ag+ |
125
|
PVP-AgNPs |
Rice (Oryza sativa L.) |
Root |
XANES |
Roots |
Oxidative dissolution, chlorination, sulfidation, complexation with organics |
Ag2S (22.1%), Ag+–cysteine (9.4%), AgCl (39.8%) |
189
|
AgNPs |
Cowpea (Vigna unguiculata L.) |
Root |
XANES |
Roots, stems, leaves |
Oxidative dissolution, complexation with organics |
Roots: Ag–glutathione (48%), Ag-histidine (21%) |
209
|
Stems: Ag–glutathione (13%) |
Leaves: Ag–glutathione (50–73%) |
AgNPs |
Wheat (Triticum aestivum L.) |
Root |
XANES |
Roots, shoots |
Oxidative dissolution, complexation with organics |
Roots: Ag–glutathione (65%), Ag-histidine (4%) |
209
|
Shoots: Ag–glutathione (72%), Ag-histidine (6%) |
PVP-AgNPs |
Wheat (Triticum aestivum L.) |
Root |
μ-XANES |
Roots |
Oxidative dissolution, complexation with organics |
Cell wall of the endodermis: Ag–thiols (77%), other Ag ionic species (26%) |
252
|
Cell wall of the cortex: Ag–thiols (86%), other Ag ionic species (14%) |
PVP-AgNPs |
Duckweed (Landoltia punctata) |
Foliar, root |
EXAFS |
Roots |
Oxidative dissolution, sulfidation, complexation with organics |
Ag2S (64%), Ag–thiols (53%) |
294
|
PVP-AgNPs |
Green algae (Chlamydomonas reinhardtii) |
Cell |
XANES, EXAFS |
Cytoplasm |
Oxidative dissolution, sulfidation, complexation with organics |
Ag2S (34%), Ag–thiols (51%) |
295
|
AgNPs |
Common bean (Phaseolus vulgaris) |
Seed |
μ-XANES |
Seed coat |
Oxidative dissolution, complexation with organics |
Ag–thiols |
296
|
5.1 Silver species in plants
Using multiple advanced analytical techniques (e.g., XAS), researchers are able to trace the ultimate transformation products of internalized metallic NPs. Several Ag species including Ag2O,38 AgCl,33,37,189 Ag2S,189,294,295 and Ag-thiolate complexes37,189,209,252,294–296 were observed within plants. The presence of Ag(I) indicates the oxidative dissolution of AgNPs. Dissolved oxygen produced by photosynthesis and reactive oxygen species (ROS) generated from oxidative stress may increase the redox levels in plant tissues and oxidize the surface silver of AgNPs into Ag2O (Ag–O– speciation), and finally lead to the release of Ag+.40,297 The effects of other redox-active substances on the oxidative dissolution of AgNPs have not yet been explored in planta. Besides, endogenous organic acids and thiol-containing proteins (e.g., cysteine) inside plants can promote the dissolution of AgNPs.298
Organic thiols, as strongly binding ligands for Ag+ (Kf ≈ 1013),299 will combine with the dissolved Ag to form Ag-thiol complexes. Secondary nanoparticles including AgCl-NPs and Ag2S were also observed, which were considered as stable detoxification products stored in plants.33,37 For instance, the majority of AgNPs primarily transformed into Ag2S and Ag-thiol complexes in duckweed (Landoltia punctata) tissues.294 In rice roots, the content of Ag2S, Ag-thiols, and AgCl counted for >71% of total Ag.189
Moreover, in vivo reduction of Ag+ into metallic Ag nanoparticles is also feasible due to the existence of reducing substances (e.g., polysaccharides, components of the plant cell wall as well as products of photosynthesis)300 and antioxidant compounds in plants.301–303
5.2 Concerns and challenges in investigating AgNP transformation in plants
Remarkably, although different Ag species originating from AgNPs have been determined in plants, the transformation remains a complicated process with many scientific challenges that need to be addressed.
(1) A basic but less noticed criterion for studying nanoparticle biotransformation inside plants is to exclude the interference of ionic species produced from nanoparticle dissolution in the culture medium or at the nano-plant surface. Whether the secondary metal species are derived from directly absorbed nanoparticles or ions remains unclear due to the lack of suitable methodology to monitor the dynamics of Ag species at environmentally relevant concentrations. Besides, the newly formed metallic nanoparticles during the in vivo reduction of Ag+ also bring confusion when investigating the translocation patterns of nanoparticles.
(2) Diverse transformed products observed in plant tissues indicate that the transformation processes are influenced by multiple factors, including the properties of particles and the internal environments, which have been rarely investigated.304 Surface coatings of AgNPs influence their affinity to organic compounds with redox properties that are ubiquitous in plants.119,286,305,306 The secretion of root cells and constituents of sap inside vascular bundles may lead to aggregation, dissolution, and biomolecule corona coating for AgNPs.91 These properties are influenced by plant species, stage of plant growth, and environmental factors.91 It remains unclear how the complex internal environment of plants impacts the transformation of AgNPs. In vitro simulation experiments can be an effective approach to investigate the possible transformation of nanoparticles in plants.
(3) It remains unclear where the transformation of AgNPs takes place. The transformation of AgNPs could occur in the root epidermis with abundant organic acids as well as vascular bundles that contain organic compounds and inorganic salts.252 In AgNP-exposed wheat roots, Ag was mostly present as metallic Ag in the epidermis.252 However, 86% and 14% of Ag was present as Ag-thiols and other ionic Ag species in the cell wall of the cortex and the proportion of these two types of Ag changed to 77% and 26% in the cell wall of the endodermis.252 Another study found that AgNPs can penetrate the seed coats and undergo oxidative dissolution to form Ag-thiolate complexes in parenchyma cells of common bean (Phaseolus vulgaris), probably because of the intrinsic differences in the organic composition and structure between the external and internal layer of the seed coat.296
Due to the lack of methodologies to identify nanoparticles at a subcellular level, less is known about the transformation within plant organelles (e.g., chloroplasts and mitochondria).304,307 In animal cells, lysosomes, the organelles responsible for sequestration of intracellular metal (in both ionic and nanoparticulate forms), may regulate the dissolution of AgNPs.35,307 For example, AgNPs were accumulated in lysosomes of zebrafish cells and then dissolved to release Ag+ because of an acidic microenvironment in lysosomes (pH ≈ 4.5).308 In plant cells, dissolution of nanoparticles may also happen in lytic vacuoles containing hydrolytic enzymes, which is far from being understood. Wang et al. found that Ag2S was formed inside the cytoplasm of algae but no sulfidation of AgNPs occurred outside the cytoplasm, suggesting the presence of sulfidation in the cytoplasm.295 Dai et al. identified the transformation products of CuO NPs in plant cells (Nicotiana tabacum) and found that reduction and sulfidation firstly occurred in the cell wall and then intracellularly in the protoplast and mitochondria.309 Although transformed Ag species have been observed in different plant tissues, the dynamic transformation of AgNPs during transportation in the whole plants, particularly inside plant cells, is not well understood.
(4) It is noteworthy that the transformed Ag species have different toxicities.209,249 The observed adverse impacts in plants may be ascribed to the transformed products in some cases, although oxidation of AgNPs and formation of other Ag species are deemed to be the detoxification processes to reduce particle stress.304 Various biotransformation products generated in different plant tissues may lead to dynamic and multiple exposures, which should be further evaluated. In addition, the translocation pattern of metal inside plants may change, due to the difference in translocation ability of transformed products.309 The translocation factors of Ag2S-NPs were lower than those of AgNPs in wheat,209,252 but were higher than those of AgNPs in rice.188 Our group found that Ag2S-NPs were translocated upward to soybean leaves via root exposure and the accumulated Ag2S-NPs in leaves were assimilated by snails, indicating the considerable trophic availability of Ag2S-NPs.32 In view of the complicated transformation in plants, the question remains whether internalized AgNPs or the transformed products will be transferred to edible portions of mature plants, such as fruits and grains.
(5) An increasing number of studies in vitro have found that biomolecules (proteins, lipids, etc.) were ready to form a corona on the surface of AgNPs,310–313 which has not been observed in plants. The corona changes the particle surface properties and has an appreciable impact on the aggregation, dissolution, and biochemical transformation of AgNPs, which has been described in non-plant systems (for example, marine waters and gastrointestinal fluids) elsewhere.314–318 For instance, Miclăuş et al. found that the protein corona could modulate the chemical transformation of AgNPs.297 The strongly attached protein prevents the diffusion of released Ag+ and acts as a site for sulfidation at the surface of AgNPs. On the contrary, the weakly attached proteins transport Ag+ away from the particle surface and thus reduce the formation of Ag2S-NPs.297 As for plants, the abundant proteins, lipids, and carbohydrates in phloem may allow the corona to form around AgNPs.92,105,119 Borgatta et al. observed that a 5.1 ± 0.2 nm thick corona, composed primarily of proteins, was formed around the CuO NPs (50 mg L−1) that were incubated in pumpkin xylem fluid for 3 h.319 Kurepa et al. observed the bio-corona formed on TiO2 NPs in Arabidopsis thaliana leaf and the layer was enriched with metabolites such as flavonoids and lipids.320 Bing et al. reported that the tested plant proteins (i.e., glutenin, gliadin, zein, and soy protein) could form 4–60 nm thick coronas on the TiO2 NP surface and thus changed the surface potential and morphology of the particles.321 The composition and properties of coronas formed in leaves, vascular systems and root tissues are different due to the complex biological matrices inside a wide range of plant species.322 The effects of the corona on the translocation and biotransformation of AgNPs in planta remain to be understood. Sufficient proteomic and metabolomic information of plants, integration of multi-scale analytical techniques, and development of computational analyses are required to predict the possible transformation of nanoparticles in planta and such advances will enable further study of biological effects of nanoparticles on plants.322,323
6. Transfer of AgNPs in food chains and risk of dietary intake for humans
Nanoparticles taken up by plants can be transferred to primary consumers via food chains.324–326 For instance, ryegrass (Lolium perenne) can accumulate up to 0.2 mg kg−1 Ag after growing in AgNP amended soil for 3 weeks and the internalized Ag can be transferred to a primary consumer, snail (Cantareus aspersus).90 AgNPs existed in all aquatic organisms in Taihu Lake of China and the biomagnification of AgNPs with a trophic magnification factor (TMF) of 1.21 was found, proving the trophic transfer of AgNPs in natural aquatic food webs.327 Thus, trophic transfer of AgNPs exists in aquatic and terrestrial systems and acts as a potential pathway of AgNP exposure for organisms at different trophic levels.31,328–330
Parent-progeny transfer of nanoparticles is possible because the internalized nanoparticles via root uptake were detected in the harvested seeds.331,332 The existence of Ag in edible parts (e.g., grains and fruits) leads to the potential risk to food chains.39,197,333 The total Ag content was found to be highest in dry fruits, nuts, and seeds, (734 ng kg−1), followed by cereals and cereal products (375 ng kg−1) and potatoes (292 ng kg−1), and the estimated dietary daily intake of Ag was 0.013 μg kg−1 body weight per day.334 It is worth noting that the safety standard of maximum Ag levels in vegetables, fruits, grains, or other agricultural products is still lacking. The accumulation of engineered nanoparticles in plant tissues has raised the concern about the potential chronic exposure of terrestrial animals and humans to them through food chains.
7. Conclusions and perspectives
The application of engineered nanomaterials in consumer products has increased the concern about their potential risk to the ecosystem and human health. In addition to anthropogenic input, the natural generation of AgNPs in the environment, especially in the root zone, also makes them more accessible to plants. Studies on the ecological risk of AgNPs have revealed the potential transformation of nanoparticles in complex aquatic or terrestrial environments as well as particle-specific toxicity to living organisms through direct contact, but the trophic transfer of nanoparticles after uptake in plants is seldom investigated.
An increasing number of studies have found that multiple factors were governing the plant uptake of AgNPs, including particle properties (e.g., size and surface charge), soil components (e.g., clay, carbonates, iron oxides, NOM, and symbiotic microorganisms) and plant species. A better understanding of AgNPs uptake kinetics in plants will help to predict more accurately the dynamic accumulation of Ag in plants. However, given the shortcomings of the current studies (e.g., short-time exposure and immature plants), long-term exposure experiments, combined with uptake kinetics investigation, are required to verify the role of these factors in natural scenarios.
Internalized AgNPs have been observed in leaves and stems after root exposure, suggesting that AgNPs can be transferred inside plants through the vascular system. However, whether Ag could accumulate in seeds and fruits in the form of nanoparticles is currently unknown due to the lack of investigation. Moreover, the transformed products of AgNPs (e.g., AgCl, Ag2S, and Ag-thiols) may also change the translocation and accumulation of Ag in plants. Although these Ag species have been observed inside plants, little is known about the actual elemental component, content, and morphology of the transformed products. Questions about which factors affect the transformation, where the transformation takes place, and whether these transformed products are accumulated in edible tissues remain to be answered. Given that the transformed products formed at different plant tissues may be variant, the discrimination of Ag species in edible parts is essential to evaluate the risk of Ag entering the food chain.
A combination of innovative qualitative and quantitative techniques, computational simulations, and conceptual models is helpful to deliver in-depth details on the fate of AgNPs inside plants and to reveal the complex mechanisms of nanoparticle-plant interaction. To date, methods to investigate the uptake, translocation, and accumulation of AgNPs (as well as other NPs) inside plants are still limited. As the applications of nanoparticles continue to develop, a comprehensive understanding of the uptake and in planta fate of AgNPs is needed to predict the bioavailability of Ag and its potential to enter food chains, which helps to evaluate risks to wildlife and human health.
Author contributions
All the authors have contributed to the discussion and the writing of this manuscript.
Conflicts of interest
There are no conflicts of interest to declare.
Acknowledgements
This work was supported by the National Natural Science Foundation of China (41430752 and 41771526). We thank the anonymous reviewers for their comments and suggestion on this manuscript.
References
- G. V. Lowry, A. Avellan and L. M. Gilbertson, Opportunities and challenges for nanotechnology in the agri-tech revolution, Nat. Nanotechnol., 2019, 14, 517–522 CrossRef CAS PubMed
.
- M. Kah, N. Tufenkji and J. C. White, Nano-enabled strategies to enhance crop nutrition and protection, Nat. Nanotechnol., 2019, 14, 532–540 CrossRef CAS PubMed
.
- J. P. Giraldo, H. Wu, G. M. Newkirk and S. Kruss, Nanobiotechnology approaches for engineering smart plant sensors, Nat. Nanotechnol., 2019, 14, 541–553 CrossRef CAS PubMed
.
- T. Hofmann, G. V. Lowry, S. Ghoshal, N. Tufenkji, D. Brambilla, J. R. Dutcher, L. M. Gilbertson, J. P. Giraldo, J. M. Kinsella, M. P. Landry, W. Lovell, R. Naccache, M. Paret, J. A. Pedersen, J. M. Unrine, J. C. White and K. J. Wilkinson, Technology readiness and overcoming barriers to sustainably implement nanotechnology-enabled plant agriculture, Nat. Food, 2020, 1, 416–425 CrossRef
.
- P. Zhang, Z. Guo, S. Ullah, G. Melagraki, A. Afantitis and I. Lynch, Nanotechnology and artificial intelligence to enable sustainable and precision agriculture, Nat. Plants, 2021, 7, 864–876 CrossRef PubMed
.
- M. Kah, L. J. Johnston, R. S. Kookana, W. Bruce, A. Haase, V. Ritz, J. Dinglasan, S. Doak, H. Garelick and V. Gubala, Comprehensive framework for human health risk assessment of nanopesticides, Nat. Nanotechnol., 2021, 16, 955–964 CrossRef CAS PubMed
.
- H. Singh, A. Sharma, S. K. Bhardwaj, S. K. Arya, N. Bhardwaj and M. Khatri, Recent advances in the applications of nano-agrochemicals for sustainable agricultural development, Environ. Sci.: Processes Impacts, 2021, 23, 213–239 RSC
.
- I. O. Adisa, V. L. R. Pullagurala, J. R. Peralta-Videa, C. O. Dimkpa, W. H. Elmer, J. L. Gardea-Torresdey and J. C. White, Recent advances in nano-enabled fertilizers and pesticides: A critical review of mechanisms of action, Environ. Sci.: Nano, 2019, 6, 2002–2030 RSC
.
- H. Ur Rahim, M. Qaswar, M. Uddin, C. Giannini, M. L. Herrera and G. Rea, Nano-enable materials promoting sustainability and resilience in modern agriculture, Nanomaterials, 2021, 11, 2068 CrossRef CAS PubMed
.
- Z.-M. Xiu, J. Ma and P. J. J. Alvarez, Differential effect of common ligands and molecular oxygen on antimicrobial activity of silver nanoparticles versus silver ions, Environ. Sci. Technol., 2011, 45, 9003–9008 CrossRef CAS PubMed
.
- A. P. Richter, J. S. Brown, B. Bharti, A. Wang, S. Gangwal, K. Houck, E. A. Cohen Hubal, V. N. Paunov, S. D. Stoyanov and O. D. Velev, An environmentally benign antimicrobial nanoparticle based on a silver-infused lignin core, Nat. Nanotechnol., 2015, 10, 817–823 CrossRef CAS PubMed
.
- C. S. Ward, J.-F. Pan, B. P. Colman, Z. Wang, C. A. Gwin, T. C. Williams, A. Ardis, C. K. Gunsch and D. E. Hunt, Conserved microbial toxicity responses for acute and chronic silver nanoparticle treatments in wetland mesocosms, Environ. Sci. Technol., 2019, 53, 3268–3276 CrossRef CAS PubMed
.
- G. R. Tortella, O. Rubilar, N. Duran, M. C. Diez, M. Martinez, J. Parada and A. B. Seabra, Silver nanoparticles: Toxicity in model organisms as an overview of its hazard for human health and the environment, J. Hazard. Mater., 2020, 390, 121974 CrossRef CAS PubMed
.
- S. P. Deshmukh, S. M. Patil, S. B. Mullani and S. D. Delekar, Silver nanoparticles as an effective disinfectant: A review, Mater. Sci. Eng., C, 2019, 97, 954–965 CrossRef CAS PubMed
.
- C. G. A. Das, V. G. Kumar, T. S. Dhas, V. Karthick, K. Govindaraju, J. M. Joselin and J. Baalamurugan, Antibacterial activity of silver nanoparticles (biosynthesis): A short review on recent advances, Biocatal. Agric. Biotechnol., 2020, 27, 101593 CrossRef
.
- M. S. Sadak, Impact of silver nanoparticles on plant growth, some biochemical aspects, and yield of fenugreek plant (Trigonella foenum-graecum), Bull. Natl. Res. Cent., 2019, 43, 38 CrossRef
.
- H. S. Kim, H. S. Kang, G. J. Chu and H. S. Byun, Antifungal effectiveness of nanosilver colloid against rose powdery mildew in greenhouses, Solid State Phenom., 2008, 135, 15–18 CAS
.
- S. W. Kim, J. H. Jung, K. Lamsal, Y. S. Kim, J. S. Min and Y. S. Lee, Antifungal effects of silver nanoparticles (AgNPs) against various plant pathogenic fungi, Mycobiology, 2012, 40, 53–58 CrossRef CAS PubMed
.
- K. Lamsal, S. W. Kim, J. H. Jung, Y. S. Kim, K. S. Kim and Y. S. Lee, Application of silver nanoparticles for the control of colletotrichum species in vitro and pepper anthracnose disease in field, Mycobiology, 2011, 39, 194–199 CrossRef CAS PubMed
.
- W. Zhang, S. Ke, C. Sun, X. Xu, J. Chen and L. Yao, Fate and toxicity of silver nanoparticles in freshwater from laboratory to realistic environments: A review, Environ. Sci. Pollut. Res., 2019, 26, 7390–7404 CrossRef CAS PubMed
.
- A. Azimzada, N. Tufenkji and K. J. Wilkinson, Transformations of silver nanoparticles in wastewater effluents: Links to Ag bioavailability, Environ. Sci.: Nano, 2017, 4, 1339–1349 RSC
.
- B. Nowack, H. F. Krug and M. Height, 120 years of nanosilver history: Implications for policy makers, Environ. Sci. Technol., 2011, 45, 1177–1183 CrossRef CAS PubMed
.
- J. Pulit-Prociak and M. Banach, Silver nanoparticles - a material of the future…?, Open Chem., 2016, 14, 76–91 CAS
.
- J. Farkas, H. Peter, P. Christian, J. A. Gallego Urrea, M. Hassellöv, J. Tuoriniemi, S. Gustafsson, E. Olsson, K. Hylland and K. V. Thomas, Characterization of the effluent from a nanosilver producing washing machine, Environ. Int., 2011, 37, 1057–1062 CrossRef CAS PubMed
.
- R. Kaegi, A. Voegelin, C. Ort, B. Sinnet, B. Thalmann, J. Krismer, H. Hagendorfer, M. Elumelu and E. Mueller, Fate and transformation of silver nanoparticles in urban wastewater systems, Water Res., 2013, 47, 3866–3877 CrossRef CAS PubMed
.
- E. Lombi, E. Donner, K. G. Scheckel, R. Sekine, C. Lorenz, N. V. Goetz and B. Nowack, Silver speciation and release in commercial antimicrobial textiles as influenced by washing, Chemosphere, 2014, 111, 352–358 CrossRef CAS PubMed
.
- R. Sekine, G. Brunetti, E. Donner, M. Khaksar, K. Vasilev, Å. K. Jämting, K. G. Scheckel, P. Kappen, H. Zhang and E. Lombi, Speciation and lability of Ag-, AgCl-, and Ag2S-nanoparticles in soil determined by X-ray absorption spectroscopy and diffusive gradients in thin films, Environ. Sci. Technol., 2015, 49, 897–905 CrossRef CAS PubMed
.
- E. Donner, K. Scheckel, R. Sekine, R. S. Popelka-Filcoff, J. W. Bennett, G. Brunetti, R. Naidu, S. P. McGrath and E. Lombi, Non-labile silver species in biosolids remain stable throughout 50 years of weathering and ageing, Environ. Pollut., 2015, 205, 78–86 CrossRef CAS PubMed
.
- A. E. Pradas del Real, H. Castillo-Michel, R. Kaegi, B. Sinnet, V. Magnin, N. Findling, J. Villanova, M. Carrière, C. Santaella, A. Fernández-Martínez, C. Levard and G. Sarret, Fate of Ag-NPs in sewage sludge after application on agricultural soils, Environ. Sci. Technol., 2016, 50, 1759–1768 CrossRef CAS PubMed
.
- D. K. Tripathi, A. Tripathi, Shweta, S. Singh, Y. Singh, K. Vishwakarma, G. Yadav, S. Sharma, V. K. Singh, R. K. Mishra, R. G. Upadhyay, N. K. Dubey, Y. Lee and D. K. Chauhan, Uptake, accumulation and toxicity of silver nanoparticle in autotrophic plants, and heterotrophic microbes: A concentric review, Front. Microbiol., 2017, 8, 07 Search PubMed
.
- F. Dang, Y. Huang, Y. Wang, D. Zhou and B. Xing, Transfer and toxicity of silver nanoparticles in the food chain, Environ. Sci.: Nano, 2021, 8, 1519–1535 RSC
.
- F. Dang, Y. Chen, Y. Huang, H. Hintelmann, Y. Si and D. Zhou, Discerning the sources of silver nanoparticle in a terrestrial food chain by sable isotope tracer technique, Environ. Sci. Technol., 2019, 53, 3802–3810 CrossRef CAS PubMed
.
- C. Li, F. Dang, M. Li, M. Zhu, H. Zhong, H. Hintelmann and D. Zhou, Effects of exposure pathways on the accumulation and phytotoxicity of silver nanoparticles in soybean and rice, Nanotoxicology, 2017, 11, 699–709 CrossRef CAS PubMed
.
- B. Quah, C. Musante, J. C. White and X. Ma, Phytotoxicity, uptake, and accumulation of silver with different particle sizes and chemical forms, J. Nanopart. Res., 2015, 17, 277 CrossRef
.
- X. Yang, C. Jiang, H. Hsu-Kim, A. R. Badireddy, M. Dykstra, M. Wiesner, D. E. Hinton and J. N. Meyer, Silver nanoparticle behavior, uptake, and toxicity in Caenorhabditis elegans: Effects of natural organic matter, Environ. Sci. Technol., 2014, 48, 3486–3495 CrossRef CAS PubMed
.
- L. Yin, B. P. Colman, B. M. McGill, J. P. Wright and E. S. Bernhardt, Effects of silver nanoparticle exposure on germination and early growth of eleven wetland plants, PLoS One, 2012, 7, e47674 CrossRef CAS PubMed
.
- C. Larue, H. Castillo-Michel, S. Sobanska, L. Cécillon, S. Bureau, V. Barthès, L. Ouerdane, M. Carrière and G. Sarret, Foliar exposure of the crop Lactuca sativa to silver nanoparticles: Evidence for internalization and changes in Ag speciation, J. Hazard. Mater., 2014, 264, 98–106 CrossRef CAS PubMed
.
- L. Yin, Y. Cheng, B. Espinasse, B. P. Colman, M. Auffan, M. Wiesner, J. Rose, J. Liu and E. S. Bernhardt, More than the ions: The effects of silver nanoparticles on Lolium multiflorum, Environ. Sci. Technol., 2011, 45, 2360–2367 CrossRef CAS PubMed
.
- J. Yang, F. Jiang, C. Ma, Y. Rui, M. Rui, M. Adeel, W. Cao and B. Xing, Alteration of crop yield and quality of wheat upon exposure to silver nanoparticles in a life cycle study, J. Agric. Food Chem., 2018, 66, 2589–2597 CrossRef CAS PubMed
.
- C. O. Dimkpa, J. E. McLean, N. Martineau, D. W. Britt, R. Haverkamp and A. J. Anderson, Silver nanoparticles disrupt wheat (Triticum aestivum L.) growth in a sand matrix, Environ. Sci. Technol., 2013, 47, 1082–1090 CrossRef CAS PubMed
.
- V. L. Pachapur, A. Dalila Larios, M. Cledon, S. K. Brar, M. Verma and R. Y. Surampalli, Behavior and characterization of titanium dioxide and silver nanoparticles in soils, Sci. Total Environ., 2016, 563–564, 933–943 CrossRef CAS PubMed
.
- T. M. Benn and P. Westerhoff, Nanoparticle silver released into water from commercially available sock fabrics, Environ. Sci. Technol., 2008, 42, 4133–4139 CrossRef CAS PubMed
.
- P. Wang, N. W. Menzies, P. G. Dennis, J. Guo, C. Forstner, R. Sekine, E. Lombi, P. Kappen, P. M. Bertsch and P. M. Kopittke, Silver nanoparticles entering soils via the wastewater-sludge-soil pathway pose low risk to plants but elevated Cl concentrations increase Ag bioavailability, Environ. Sci. Technol., 2016, 50, 8274–8281 CrossRef CAS PubMed
.
- P. M. Potter, J. Navratilova, K. R. Rogers and S. R. Al-Abed, Transformation of silver nanoparticle consumer products during simulated usage and disposal, Environ. Sci.: Nano, 2019, 6, 592–598 RSC
.
- G. V. Lowry, B. P. Espinasse, A. R. Badireddy, C. J. Richardson, B. C. Reinsch, L. D. Bryant, A. J. Bone, A. Deonarine, S. Chae, M. Therezien, B. P. Colman, H. Hsu-Kim, E. S. Bernhardt, C. W. Matson and M. R. Wiesner, Long-term transformation and fate of manufactured Ag nanoparticles in a simulated large scale freshwater emergent wetland, Environ. Sci. Technol., 2012, 46, 7027 CrossRef CAS PubMed
.
- M. Li, P. Wang, F. Dang and D. Zhou, The transformation and fate of silver nanoparticles in paddy soil: Effects of soil organic matter and redox conditions, Environ. Sci.: Nano, 2017, 4, 919–928 RSC
.
- Y. Yin, W. Xu, Z. Tan, Y. Li, W. Wang, X. Guo, S. Yu, J. Liu and G. Jiang, Photo- and thermo-chemical transformation of AgCl and Ag2S in environmental matrices and its implication, Environ. Pollut., 2017, 220, 955–962 CrossRef CAS PubMed
.
- L. Settimio, M. J. McLaughlin, J. K. Kirby, K. A. Langdon, E. Lombi, E. Donner and K. G. Scheckel, Fate and lability of silver in soils: Effect of ageing, Environ. Pollut., 2014, 191, 151 CrossRef CAS PubMed
.
- M. Reich, S. L. Chryssoulis, A. Deditius, C. Palacios, A. Zúñiga, M. Weldt and M. Alvear, “Invisible” silver and gold in supergene digenite (Cu1.8S), Geochim. Cosmochim. Acta, 2010, 74, 6157–6173 CrossRef CAS
.
- J. A. Gómez-Caballero, M. G. Villaseñor-Cabral, P. Santiago-Jacinto and F. Ponce-Abad, Hypogene Ba-rich todorokite and associated nanometric native silver in the San Miguel Tenango mining area, ZACATLÁN, PUEBLA, MEXICO, Can. Mineral., 2010, 48, 1237–1253 CrossRef
.
- D. Lu, Q. Liu, T. Zhang, Y. Cai, Y. Yin and G. Jiang, Stable silver isotope fractionation in the natural transformation process of silver nanoparticles, Nat. Nanotechnol., 2016, 11, 682–686 CrossRef CAS PubMed
.
- X. Zhang, C. Yang, H. Yu and G. Sheng, Light-induced reduction of silver ions to silver nanoparticles in aquatic environments by microbial extracellular polymeric substances (EPS), Water Res., 2016, 106, 242–248 CrossRef CAS PubMed
.
- Y. Yin, M. Shen, X. Zhou, S. Yu, J. Chao, J. Liu and G. Jiang, Photoreduction and stabilization capability of molecular weight fractionated natural organic matter in transformation of silver Ion to metallic nanoparticle, Environ. Sci. Technol., 2014, 48, 9366–9373 CrossRef CAS PubMed
.
- A. Wimmer, A. Kalinnik and M. Schuster, New insights into the formation of silver-based nanoparticles under natural and semi-natural conditions, Water Res., 2018, 141, 227–234 CrossRef CAS PubMed
.
- F. Dong, C. Wu, A. Miao and K. Pan, Reduction of silver ions to form silver nanoparticles by redox-active organic molecules: Coupled impact of the redox state and environmental factors, Environ. Sci.: Nano, 2021, 8, 269–281 RSC
.
- Z. Tan, X. Guo, Y. Yin, B. Wang, Q. Bai, X. Li, J. Liu and G. Jiang, Freezing facilitates formation of silver nanoparticles under natural and simulated sunlight conditions, Environ. Sci. Technol., 2019, 53, 13802–13811 CrossRef CAS PubMed
.
- N. Akaighe, R. I. MacCuspie, D. A. Navarro, D. S. Aga, S. Banerjee, M. Sohn and V. K. Sharma, Humic acid-induced silver nanoparticle formation under environmentally relevant conditions, Environ. Sci. Technol., 2011, 45, 3895–3901 CrossRef CAS PubMed
.
- N. F. Adegboyega, V. K. Sharma, K. Siskova, R. Zbořil, M. Sohn, B. J. Schultz and S. Banerjee, Interactions of aqueous Ag+ with fulvic acids: Mechanisms of silver nanoparticle formation and investigation of stability, Environ. Sci. Technol., 2013, 47, 757–764 CrossRef CAS PubMed
.
- N. F. Adegboyega, V. K. Sharma, L. Cizmas and C. M. Sayes, UV light induces Ag nanoparticle formation: Roles of natural organic matter, iron, and oxygen, Environ. Chem. Lett., 2016, 14, 353–357 CrossRef CAS
.
- Y. Yin, J. Liu and G. Jiang, Sunlight-induced reduction of ionic Ag and Au to metallic nanoparticles by dissolved organic matter, ACS Nano, 2012, 6, 7910–7919 CrossRef CAS PubMed
.
- H. Liu, X. Gu, C. Wei, H. Fu, P. J. J. Alvarez, Q. Li, S. Zheng, X. Qu and D. Zhu, Threshold concentrations of silver ions exist for the sunlight-induced formation of silver nanoparticles in the rresence of natural organic matter, Environ. Sci. Technol., 2018, 52, 4040–4050 CrossRef CAS PubMed
.
- Y. Huang, T. Qian, F. Dang, Y. Yin, M. Li and D. Zhou, Significant contribution of metastable particulate organic matter to natural formation of silver nanoparticles in soils, Nat. Commun., 2019, 10, 3775 CrossRef PubMed
.
- H. Peng, H. Guo, P. Gao, Y. Zhou, B. Pan and B. Xing, Reduction of silver ions to silver nanoparticles by biomass and biochar: Mechanisms and critical factors, Sci. Total Environ., 2021, 779, 146326 CrossRef CAS PubMed
.
- B. Dong, G. Liu, J. Zhou, J. Wang and R. Jin, Transformation of silver ions to silver nanoparticles mediated by humic acid under dark conditions at ambient temperature, J. Hazard. Mater., 2020, 383, 121190 CrossRef CAS PubMed
.
-
V. K. Sharma and R. Zboril, in Bioactivity of Engineered Nanoparticles, ed. B. Yan, H. Zhou and J. L. Gardea-Torresdey, Springer Singapore, Singapore, 2017, pp. 239–258 DOI:10.1007/978-981-10-5864-6_10
.
- A. Henglein, Non-metallic silver clusters in aqueous solution: Stabilization and chemical reactions, Chem. Phys. Lett., 1989, 154, 473–476 CrossRef CAS
.
- Y. Yin, D. Han, C. Tai, Z. Tan, X. Zhou, S. Yu, J. Liu and G. Jiang, Catalytic role of iron in the formation of silver nanoparticles in photo-irradiated Ag+-dissolved organic matter solution, Environ. Pollut., 2017, 225, 66–73 CrossRef CAS PubMed
.
- L. Li, Q. Zhou, F. Geng, Y. Wang and G. Jiang, Formation of nanosilver from silver sulfide nanoparticles in natural waters by photoinduced Fe(II, III) redox cycling, Environ. Sci. Technol., 2016, 50, 13342–13350 CrossRef CAS PubMed
.
- D. He, S. Garg, Z. Wang, L. Li, H. Rong, X. Ma, G. Li, T. An and T. D. Waite, Silver sulfide nanoparticles in aqueous environments: Formation, transformation and toxicity, Environ. Sci.: Nano, 2019, 6, 1674–1687 RSC
.
- N. F. Adegboyega, V. K. Sharma, K. M. Siskova, R. Vecerova, M. Kolar, R. Zbořil and J. L. Gardea-Torresdey, Enhanced formation of silver nanoparticles in Ag+-NOM-Iron(II, III) systems and antibacterial activity studies, Environ. Sci. Technol., 2014, 48, 3228–3235 CrossRef CAS PubMed
.
- N. Chen, D. Huang, G. Liu, L. Chu, G. Fang, C. Zhu, D. Zhou and J. Gao, Active iron species driven hydroxyl radicals formation in oxygenation of different paddy soils: Implications to polycyclic aromatic hydrocarbons degradation, Water Res., 2021, 203, 117484 CrossRef CAS PubMed
.
- E. J. O'Loughlin, S. D. Kelly, K. M. Kemner, R. Csencsits and R. E. Cook, Reduction of AgI, AuIII, CuII, and HgII by FeII/FeIII hydroxysulfate green rust, Chemosphere, 2003, 53, 437–446 CrossRef
.
- S. Ayadi, C. Perca and L. Legrand, New one-pot synthesis of Au and Ag nanoparticles using green rust reactive particle as a micro-reactor, Nanoscale Res. Lett., 2013, 8, 95 CrossRef PubMed
.
- T. Klaus, R. Joerger, E. Olsson and C.-G. Granqvist, Silver-based crystalline nanoparticles, microbially fabricated, Proc. Natl. Acad. Sci. U. S. A., 1999, 96, 13611 CrossRef CAS PubMed
.
- K. Juganson, M. Mortimer, A. Ivask, K. Kasemets and A. Kahru, Extracellular conversion of silver ions into silver nanoparticles by protozoan Tetrahymena thermophila, Environ. Sci.: Processes Impacts, 2013, 15, 244–250 RSC
.
- Y. Yin, X. Yang, L. Hu, Z. Tan, L. Zhao, Z. Zhang, J. Liu and G. Jiang, Superoxide-mediated extracellular biosynthesis of silver nanoparticles by the fungus Fusarium oxysporum, Environ. Sci. Technol. Lett., 2016, 3, 160–165 CrossRef CAS
.
- P. Mukherjee, A. Ahmad, D. Mandal, S. Senapati, S. R. Sainkar, M. I. Khan, R. Parishcha, P. V. Ajaykumar, M. Alam, R. Kumar and M. Sastry, Fungus-mediated synthesis of silver nanoparticles and their immobilization in the mycelial matrix: A novel biological approach to nanoparticle synthesis, Nano Lett., 2001, 1, 515–519 CrossRef CAS
.
- L. Sintubin, W. De Windt, J. Dick, J. Mast, D. van der Ha, W. Verstraete and N. Boon, Lactic acid bacteria as reducing and capping agent for the fast and efficient production of silver nanoparticles, Appl. Microbiol. Biotechnol., 2009, 84, 741–749 CrossRef CAS PubMed
.
- S. Anil Kumar, M. K. Abyaneh, S. W. Gosavi, S. K. Kulkarni, R. Pasricha, A. Ahmad and M. I. Khan, Nitrate reductase-mediated synthesis of silver nanoparticles from AgNO3, Biotechnol. Lett., 2007, 29, 439–445 CrossRef CAS PubMed
.
- R. Vaidyanathan, S. Gopalram, K. Kalishwaralal, V. Deepak, S. R. K. Pandian and S. Gurunathan, Enhanced silver nanoparticle synthesis by optimization of nitrate reductase activity, Colloids Surf., B, 2010, 75, 335–341 CrossRef CAS PubMed
.
- F. Kang, P. J. Alvarez and D. Zhu, Microbial extracellular polymeric substances reduce Ag+ to silver nanoparticles and antagonize bactericidal activity, Environ. Sci. Technol., 2014, 48, 316–322 CrossRef CAS PubMed
.
- A. R. Badireddy, J. Farner Budarz, S. M. Marinakos, S. Chellam and M. R. Wiesner, Formation of silver nanoparticles in visible light-illuminated waters: Mechanism and possible impacts on the persistence of AgNPs and bacterial lysis, Environ. Eng. Sci., 2014, 31, 338–349 CrossRef CAS
.
- P. Mukherjee, M. Roy, B. P. Mandal, G. K. Dey, P. K. Mukherjee, J. Ghatak, A. K. Tyagi and S. P. Kale, Green synthesis of highly stabilized nanocrystalline silver particles by a non-pathogenic and agriculturally important fungusT. asperellum, Nanotechnology, 2008, 19, 075103 CrossRef CAS PubMed
.
- H. Guo, C. Ma, L. Thistle, M. Huynh, C. Yu, D. Clasby, B. Chefetz, T. Polubesova, J. C. White, L. He and B. Xing, Transformation of Ag ions into Ag nanoparticle-loaded AgCl microcubes in the plant root zone, Environ. Sci.: Nano, 2019, 6, 1099–1110 RSC
.
- H. Guo, F. Han, H. Shang, S. Xiong, M. Huynh, L. Thistle, L. Meng, L. He and B. Xing, New insight into naturally formed nanosilver particles: Role of plant root exudates, Environ. Sci.: Nano, 2021, 8, 1580–1592 RSC
.
- P. Pardha-Saradhi, G. Yamal, T. Peddisetty, P. Sharmila, S. Nagar, J. Singh, R. Nagarajan and K. S. Rao, Reducing strength prevailing at root surface of plants promotes reduction of Ag+ and generation of Ag0/Ag2O nanoparticles exogenously in aqueous phase, PLoS One, 2014, 9, e106715 CrossRef PubMed
.
- N. K. Fageria, M. P. B. Filho, A. Moreira and C. M. Guimarães, Foliar fertilization of crop plants, J. Plant Nutr., 2009, 32, 1044–1064 CrossRef CAS
.
- W. Wang, J. C. Tarafdar and P. Biswas, Nanoparticle synthesis and delivery by an aerosol route for watermelon plant foliar uptake, J. Nanopart. Res., 2013, 15, 1417 CrossRef
.
- K. Schlich, M. Hoppe, M. Kraas, J. Schubert, M. Chanana and K. Hund-Rinke, Long-term effects of three different silver sulfide nanomaterials, silver nitrate and bulk silver sulfide on soil microorganisms and plants, Environ. Pollut., 2018, 242, 1850–1859 CrossRef CAS PubMed
.
- P. Courtois, A. de Vaufleury, A. Grosser, C. Lors and F. Vandenbulcke, Transfer of sulfidized silver from silver nanoparticles, in sewage sludge, to plants and primary consumers in agricultural soil environment, Sci. Total Environ., 2021, 777, 145900 CrossRef CAS PubMed
.
- Y. Su, V. E. T. M. Ashworth, N. K. Geitner, M. R. Wiesner, N. Ginnan, P. Rolshausen, C. Roper and D. Jassby, Delivery, fate, and mobility of silver nanoparticles in citrus trees, ACS Nano, 2020, 14, 2966–2981 CrossRef CAS PubMed
.
- A. Avellan, J. Yun, Y. Zhang, E. Spielman-Sun, J. M. Unrine, J. Thieme, J. Li, E. Lombi, G. Bland and G. V. Lowry, Nanoparticle size and coating chemistry control foliar uptake pathways, translocation, and leaf-to-rhizosphere transport in wheat, ACS Nano, 2019, 13, 5291–5305 CrossRef CAS PubMed
.
- T. Eichert and H. E. Goldbach, Equivalent pore radii of hydrophilic foliar uptake routes in stomatous and astomatous leaf surfaces - further evidence for a stomatal pathway, Physiol. Plant., 2008, 132, 491–502 CrossRef CAS PubMed
.
- T. Eichert, A. Kurtz, U. Steiner and H. E. Goldbach, Size exclusion limits and lateral heterogeneity of the stomatal foliar uptake pathway for aqueous solutes and water-suspended nanoparticles, Physiol. Plant., 2008, 134, 151–160 CrossRef CAS PubMed
.
- G. Uzu, S. Sobanska, G. Sarret, M. Muñoz and C. Dumat, Foliar lead uptake by lettuce exposed to atmospheric fallouts, Environ. Sci. Technol., 2010, 44, 1036–1042 CrossRef CAS PubMed
.
- H. Giday, K. H. Kjaer, D. Fanourakis and C.-O. Ottosen, Smaller stomata require less severe leaf drying to close: A case study in Rosa hydrida, J. Plant Physiol., 2013, 170, 1309–1316 CrossRef CAS PubMed
.
- L. Kübarsepp, L. Laanisto, Ü. Niinemets, E. Talts and T. Tosens, Are stomata in ferns and allies sluggish? Stomatal responses to CO2, humidity and light and their scaling with size and density, New Phytol., 2020, 225, 183–195 CrossRef PubMed
.
- A. M. Hetherington and F. I. Woodward, The role of stomata in sensing and driving environmental change, Nature, 2003, 424, 901–908 CrossRef CAS PubMed
.
- I. Poole, J. D. B. Weyers, T. Lawson and J. A. Raven, Variations in stomatal density and index: Implications for palaeoclimatic reconstructions, Plant, Cell Environ., 1996, 19, 705–712 CrossRef
.
- W. Konrad, A. Roth-Nebelsick and M. Grein, Modelling of stomatal density response to atmospheric CO2, J. Theor. Biol., 2008, 253, 638–658 CrossRef CAS PubMed
.
- S. S. Sugano, T. Shimada, Y. Imai, K. Okawa, A. Tamai, M. Mori and I. Hara-Nishimura, Stomagen positively regulates stomatal density in Arabidopsis, Nature, 2010, 463, 241–244 CrossRef CAS PubMed
.
- Z. Xu and G. Zhou, Responses of leaf stomatal density to water status and its relationship with photosynthesis in a grass, J. Exp. Bot., 2008, 59, 3317–3325 CrossRef CAS PubMed
.
- A. B. Bombo, A. E. S. Pereira, M. G. Lusa, E. de Medeiros Oliveira, J. L. de Oliveira, E. V. R. Campos, M. B. de Jesus, H. C. Oliveira, L. F. Fraceto and J. L. S. Mayer, A mechanistic view of interactions of a nanoherbicide with target organism, J. Agric. Food Chem., 2019, 67, 4453–4462 CrossRef CAS PubMed
.
- E. Spielman-Sun, A. Avellan, G. D. Bland, E. T. Clement, R. V. Tappero, A. S. Acerbo and G. V. Lowry, Protein coating composition targets nanoparticles to leaf stomata and trichomes, Nanoscale, 2020, 12, 3630–3636 RSC
.
- A. Avellan, J. Yun, B. P. Morais, E. T. Clement, S. M. Rodrigues and G. V. Lowry, Critical review: Role of inorganic nanoparticle properties on their foliar uptake and in planta translocation, Environ. Sci. Technol., 2021, 55(20), 13417–13431 CrossRef CAS PubMed
.
- C. Li, P. Wang, E. Lombi, M. Cheng, C. Tang, D. L. Howard, N. W. Menzies and P. M. Kopittke, Absorption of foliar-applied Zn fertilizers by trichomes in soybean and tomato, J. Exp. Bot., 2018, 69, 2717–2729 CrossRef CAS PubMed
.
- J. Geisler-Lee, Q. Wang, Y. Yao, W. Zhang, M. Geisler, K. Li, Y. Huang, Y. Chen, A. Kolmakov and X. Ma, Phytotoxicity, accumulation and transport of silver nanoparticles by Arabidopsis thaliana, Nanotoxicology, 2013, 7, 323–337 CrossRef CAS PubMed
.
- W. M. Lee, J. I. Kwak and Y. J. An, Effect of silver nanoparticles in crop plants Phaseolus radiatus and Sorghum bicolor: Media effect on phytotoxicity, Chemosphere, 2012, 86, 491–499 CrossRef CAS PubMed
.
- M. Li, H. Liu, F. Dang, H. Hintelmann, B. Yin and D. Zhou, Alteration of crop yield and quality of three vegetables upon exposure to silver nanoparticles in sludge-amended soil, ACS Sustainable Chem. Eng., 2020, 8, 2472–2480 CrossRef CAS
.
- J. He, L. Zhang, S. Y. He, E. T. Ryser, H. Li and W. Zhang, Stomata facilitate foliar sorption of silver nanoparticles by Arabidopsis thaliana, Environ. Pollut., 2022, 292, 118448 CrossRef CAS PubMed
.
- J. Kurepa, T. Paunesku, S. Vogt, H. Arora, B. M. Rabatic, J. Lu, M. B. Wanzer, G. E. Woloschak and J. A. Smalle, Uptake and distribution of ultrasmall anatase TiO2 Alizarin red S nanoconjugates in Arabidopsis thaliana, Nano Lett., 2010, 10, 2296–2302 CrossRef CAS PubMed
.
- P. Patel, P. Kumar Jatav, R. Jain, S. Gupta, S. L. Kothari and S. Kachhwaha, Humidity induced opening of stomata leads to enhanced uptake of copper nanoparticles in Triticum aestivum L., Mater. Today: Proc., 2021, 43, 3191–3196 CAS
.
- J. Zhu, J. Li, Y. Shen, S. Liu, N. Zeng, X. Zhan, J. C. White, J. Gardea-Torresdey and B. Xing, Mechanism of zinc oxide nanoparticle entry into wheat seedling leaves, Environ. Sci.: Nano, 2020, 7, 3901–3913 RSC
.
- M. Auffan, J. Rose, J.-Y. Bottero, G. V. Lowry, J.-P. Jolivet and M. R. Wiesner, Towards a definition of inorganic nanoparticles from an environmental, health and safety perspective, Nat. Nanotechnol., 2009, 4, 634–641 CrossRef CAS PubMed
.
- M. Yu, J. Yao, J. Liang, Z. Zeng, B. Cui, X. Zhao, C. Sun, Y. Wang, G. Liu and H. Cui, Development of functionalized abamectin poly(lactic acid) nanoparticles with regulatable adhesion
to enhance foliar retention, RSC Adv., 2017, 7, 11271–11280 RSC
.
- P. Hu, J. An, M. M. Faulkner, H. Wu, Z. Li, X. Tian and J. P. Giraldo, Nanoparticle charge and size control foliar delivery efficiency to plant cells and organelles, ACS Nano, 2020, 14, 7970–7986 CrossRef CAS PubMed
.
- E. Kranjc, D. Mazej, M. Regvar, D. Drobne and M. Remškar, Foliar surface free energy affects platinum nanoparticle adhesion, uptake, and translocation from leaves to roots in arugula and escarole, Environ. Sci.: Nano, 2018, 5, 520–532 RSC
.
- X. Gao, A. Kundu, V. Bueno, A. A. Rahim and S. Ghoshal, Uptake and translocation of mesoporous SiO2-coated ZnO nanoparticles to Solanum lycopersicum following foliar application, Environ. Sci. Technol., 2021, 55(20), 13551–13560 CrossRef CAS PubMed
.
- Y. Su, V. Ashworth, C. Kim, A. S. Adeleye, P. Rolshausen, C. Roper, J. White and D. Jassby, Delivery, uptake, fate, and transport of engineered nanoparticles in plants: A critical review and data analysis, Environ. Sci.: Nano, 2019, 6, 2311–2331 RSC
.
- A. Tripathi, S. Liu, P. K. Singh, N. Kumar, A. C. Pandey, D. K. Tripathi, D. K. Chauhan and S. Sahi, Differential phytotoxic responses of silver nitrate (AgNO3) and silver nanoparticle (AgNPs) in Cucumis sativus L, Plant Gene, 2017, 11, 255–264 CrossRef CAS
.
- C. G. Castro-González, L. Sánchez-Segura, F. C. Gómez-Merino and J. J. Bello-Bello, Exposure of stevia (Stevia rebaudiana B.) to silver nanoparticles in vitro: Transport and accumulation, Sci. Rep., 2019, 9, 10372 CrossRef PubMed
.
- F. Schwab, G. Zhai, M. Kern, A. Turner, J. L. Schnoor and M. R. Wiesner, Barriers, pathways and processes for uptake, translocation and accumulation of nanomaterials in plants-critical review, Nanotoxicology, 2016, 10, 257–278 CrossRef CAS PubMed
.
- W. Zhang, Q. Wang, M. Li, F. Dang and D. Zhou, Nonselective uptake of silver and gold nanoparticles by wheat, Nanotoxicology, 2019, 13, 1073–1086 CrossRef CAS PubMed
.
- J. Wu, Q. Yu, T. Bosker, M. G. Vijver and W. J. G. M. Peijnenburg, Quantifying the relative contribution of particulate versus dissolved silver to toxicity and uptake kinetics of silver nanowires in lettuce: Impact of size and coating, Nanotoxicology, 2020, 14, 1399–1414 CrossRef CAS PubMed
.
- Q. Yang, W. Shan, L. Hu, Y. Zhao, Y. Hou, Y. Yin, Y. Liang, F. Wang, Y. Cai, J. Liu and G. Jiang, Uptake and transformation of silver nanoparticles and ions by rice plants revealed by dual stable isotope tracing, Environ. Sci. Technol., 2019, 53, 625–633 CrossRef CAS PubMed
.
- F. Dang, Q. Wang, W. Cai, D. Zhou and B. Xing, Uptake kinetics of silver nanoparticles by plant: Relative importance of particles and dissolved ions, Nanotoxicology, 2020, 14, 654–666 CrossRef CAS PubMed
.
- P. P. Stefanic, P. Cvjetko, R. Biba, A. M. Domijan, I. Letofsky-Papst, M. Tkalec, S. Sikic, M. Cindric and B. Balen, Physiological, ultrastructural and proteomic responses of tobacco seedlings exposed to silver nanoparticles and silver nitrate, Chemosphere, 2018, 209, 640–653 CrossRef CAS PubMed
.
- P. M. G. Nair and I. M. Chung, Assessment of silver nanoparticle-induced physiological and molecular changes in Arabidopsis thaliana, Environ. Sci. Pollut. Res., 2014, 21, 8858–8869 CrossRef CAS PubMed
.
- T. Vinkovic, O. Novak, M. Strnad, W. Goessler, D. D. Jurasin, N. Paradikovic and I. V. Vrcek, Cytokinin response in pepper plants (Capsicum annuum L.) exposed to silver nanoparticles, Environ. Res., 2017, 156, 10–18 CrossRef CAS PubMed
.
- L. Zhang and W. Wang, Dominant role of silver ions in silver nanoparticle toxicity to a unicellular alga: Evidence from luminogen imaging, Environ. Sci. Technol., 2019, 53, 494–502 CrossRef CAS PubMed
.
- F. R. Khan, K. B. Paul, A. D. Dybowska, E. Valsami-Jones, J. R. Lead, V. Stone and T. F. Fernandes, Accumulation dynamics and acute toxicity of silver nanoparticles to daphnia magna and lumbriculus variegatus: Implications for metal modeling approaches, Environ. Sci. Technol., 2015, 49, 4389–4397 CrossRef CAS PubMed
.
- F. C. F. Santos, P. S. Tourinho, J. J. Scott-Fordsmand, C. A. M. van Gestel and M. J. B. Amorim, Toxicokinetics of Ag (nano)materials in the soil model Enchytraeus crypticus (Oligochaeta) – impact of aging and concentration, Environ. Sci.: Nano, 2021, 8, 2629–2640 RSC
.
- C. Zhao and W. Wang, Biokinetic uptake and efflux of silver nanoparticles in Daphnia magna, Environ. Sci. Technol., 2010, 44, 7699–7704 CrossRef CAS PubMed
.
- B. Xiao, X. Wang, J. Yang, K. Wang, Y. Zhang, B. Sun, T. Zhang and L. Zhu, Bioaccumulation kinetics and tissue distribution of silver nanoparticles in zebrafish: The mechanisms and influence of natural organic matter, Ecotoxicol. Environ. Saf., 2020, 194, 110454 CrossRef CAS PubMed
.
- Z. Khodaparast, C. A. M. van Gestel, A. G. Papadiamantis, S. F. Gonçalves, I. Lynch and S. Loureiro, Toxicokinetics of silver nanoparticles in the mealworm Tenebrio molitor exposed via soil or food, Sci. Total Environ., 2021, 777, 146071 CrossRef CAS PubMed
.
- C. Jiang, B. T. Castellon, C. W. Matson, G. R. Aiken and H. Hsu-Kim, Relative contributions of copper oxide nanoparticles and dissolved copper to Cu uptake kinetics of gulf killifish (Fundulus grandis) embryos, Environ. Sci. Technol., 2017, 51, 1395–1404 CrossRef CAS PubMed
.
- Z. Shao and W. Wang, Biodynamics of silver nanoparticles in an estuarine oyster revealed by 110mAgNP tracing, Environ. Sci. Technol., 2020, 54, 965–974 CrossRef CAS PubMed
.
- J. Kalman, K. B. Paul, F. R. Khan, V. Stone and T. F. Fernandes, Characterisation of bioaccumulation dynamics of three differently coated silver nanoparticles and aqueous silver in a simple freshwater food chain, Environ. Chem., 2015, 12, 662–672 CrossRef CAS
.
- W. Fan, L. Liu, R. Peng and W. Wang, High bioconcentration of titanium dioxide nanoparticles in Daphnia magna determined by kinetic approach, Sci. Total Environ., 2016, 569–570, 1224–1231 CrossRef CAS PubMed
.
- F. Ribeiro, J. A. Gallego-Urrea, R. M. Goodhead, C. A. M. Van Gestel, J. Moger, A. M. V. M. Soares and S. Loureiro, Uptake and elimination kinetics of silver nanoparticles and silver nitrate by Raphidocelis subcapitata: The influence of silver behaviour in solution, Nanotoxicology, 2015, 9, 686–695 CrossRef CAS PubMed
.
- J. Zhang, Q. Xiang, L. Shen, J. Ling, C. Zhou, J. Hu and L. Chen, Surface charge-dependent bioaccumulation dynamics of silver nanoparticles in freshwater algae, Chemosphere, 2020, 247, 125936 CrossRef CAS PubMed
.
- P. Thuesombat, S. Hannongbua, S. Akasit and S. Chadchawan, Effect of silver nanoparticles on rice (Oryza sativa L. cv. KDML 105) seed germination and seedling growth, Ecotoxicol. Environ. Saf., 2014, 104, 302–309 CrossRef CAS PubMed
.
- J. Wang, Y. Koo, A. Alexander, Y. Yang, S. Westerhof, Q. Zhang, J. L. Schnoor, V. L. Colvin, J. Braam and P. J. J. Alvarez, Phytostimulation of poplars and Arabidopsis exposed to silver nanoparticles and Ag+ at sublethal concentrations, Environ. Sci. Technol., 2013, 47, 5442–5449 CrossRef CAS PubMed
.
- L. Torrent, M. Iglesias, E. Marguí, M. Hidalgo, D. Verdaguer, L. Llorens, A. Kodre, A. Kavčič and K. Vogel-Mikuš, Uptake, translocation and ligand of silver in Lactuca sativa exposed to silver nanoparticles of different size, coatings
and concentration, J. Hazard. Mater., 2020, 384, 121201 CrossRef CAS PubMed
.
- N. K. Geitner, J. L. Cooper, A. Avellan, B. T. Castellon, B. G. Perrotta, N. Bossa, M. Simonin, S. M. Anderson, S. Inoue, M. F. Hochella, C. J. Richardson, E. S. Bernhardt, G. V. Lowry, P. L. Ferguson, C. W. Matson, R. S. King, J. M. Unrine, M. R. Wiesner and H. Hsu-Kim, Size-based differential transport, uptake, and mass distribution of ceria (CeO2) nanoparticles in wetland mesocosms, Environ. Sci. Technol., 2018, 52, 9768–9776 CrossRef CAS PubMed
.
- Z. Zhang, X. He, H. Zhang, Y. Ma, P. Zhang, Y. Ding and Y. Zhao, Uptake and distribution of ceria nanoparticles in cucumber plants, Metallomics, 2011, 3, 816–822 CrossRef CAS PubMed
.
- T. N. M. da Cruz, S. M. Savassa, M. H. F. Gomes, E. S. Rodrigues, N. M. Duran, E. de Almeida, A. P. Martinelli and H. W. P. de Carvalho, Shedding light on the mechanisms of absorption and transport of ZnO nanoparticles by plants via in vivo X-ray spectroscopy, Environ. Sci.: Nano, 2017, 4, 2367–2376 RSC
.
- T. S. Peretyazhko, Q. Zhang and V. L. Colvin, Size-controlled dissolution of silver nanoparticles at neutral and acidic pH conditions: Kinetics and size changes, Environ. Sci. Technol., 2014, 48, 11954–11961 CrossRef CAS PubMed
.
- R. Ma, C. Levard, S. M. Marinakos, Y. Cheng, J. Liu, F. M. Michel, G. E. Brown and G. V. Lowry, Size-controlled dissolution of organic-coated silver nanoparticles, Environ. Sci. Technol., 2012, 46, 752–759 CrossRef CAS PubMed
.
- L. R. R. Souza, T. Z. Corrêa, A. T. Bruni and M. A. M. S. da Veiga, The effects of solubility of silver nanoparticles, accumulation, and toxicity to the aquatic plant Lemna minor, Environ. Sci. Pollut. Res., 2021, 28, 16720–16733 CrossRef CAS PubMed
.
- M. Thwala, S. Klaine and N. Musee, Exposure media and nanoparticle size influence on the fate, bioaccumulation, and toxicity of silver nanoparticles to higher plant Salvinia minima, Molecules, 2021, 26, 2305 CrossRef CAS PubMed
.
- K. M. Koczkur, S. Mourdikoudis, L. Polavarapu and S. E. Skrabalak, Polyvinylpyrrolidone (PVP) in nanoparticle synthesis, Dalton Trans., 2015, 44, 17883–17905 RSC
.
- V. K. Sharma, K. M. Siskova, R. Zboril and J. L. Gardea-Torresdey, Organic-coated silver nanoparticles in biological and environmental conditions: Fate, stability and toxicity, Adv. Colloid Interface Sci., 2014, 204, 15–34 CrossRef CAS PubMed
.
- C. Layet, M. Auffan, C. Santaella, C. Chevassus-Rosset, M. Montes, P. Ortet, M. Barakat, B. Collin, S. Legros, M. N. Bravin, B. Angeletti, I. Kieffer, O. Proux, J.-L. Hazemann and E. Doelsch, Evidence that soil properties and organic coating drive the phytoavailability of cerium oxide nanoparticles, Environ. Sci. Technol., 2017, 51, 9756–9764 CrossRef CAS PubMed
.
- B. J. R. Thio, M. O. Montes, M. A. Mahmoud, D.-W. Lee, D. Zhou and A. A. Keller, Mobility of capped silver nanoparticles under environmentally relevant conditions, Environ. Sci. Technol., 2012, 46, 6985–6991 CrossRef CAS PubMed
.
- J. E. Song, T. Phenrat, S. Marinakos, Y. Xiao, J. Liu, M. R. Wiesner, R. D. Tilton and G. V. Lowry, Hydrophobic interactions increase attachment of gum arabic- and PVP-coated Ag nanoparticles to hydrophobic surfaces, Environ. Sci. Technol., 2011, 45, 5988–5995 CrossRef CAS PubMed
.
- P. Cvjetko, A. Milosic, A.-M. Domijan, I. Vinkovic Vrcek, S. Tolic, P. Peharec Stefanic, I. Letofsky-Papst, M. Tkalec and B. Balen, Toxicity of silver ions and differently coated silver nanoparticles in Allium cepa roots, Ecotoxicol. Environ. Saf., 2017, 137, 18–28 CrossRef CAS PubMed
.
- F. Wu, B. J. Harper and S. L. Harper, Differential dissolution and toxicity of surface functionalized silver nanoparticles in small-scale microcosms: Impacts of community complexity, Environ. Sci.: Nano, 2017, 4, 359–372 RSC
.
- K. W. H. Kwok, W. Dong, S. M. Marinakos, J. Liu, A. Chilkoti, M. R. Wiesner, M. Chernick and D. E. Hinton, Silver nanoparticle toxicity is related to coating materials and disruption of sodium concentration regulation, Nanotoxicology, 2016, 10, 1306–1317 CrossRef CAS PubMed
.
- L. Zhao, J. R. Peralta-Videa, A. Varela-Ramirez, H. Castillo-Michel, C. Li, J. Zhang, R. J. Aguilera, A. A. Keller and J. L. Gardea-Torresdey, Effect of surface coating and organic matter on the uptake of CeO2 NPs by corn plants grown in soil: Insight into the uptake mechanism, J. Hazard. Mater., 2012, 225–226, 131–138 CrossRef CAS PubMed
.
- Z. Liu, H. Wang and R. Xu, The effects of root surface charge and nitrogen forms on the adsorption of aluminum ions by the roots of rice with different aluminum tolerances, Plant Soil, 2016, 408, 43–53 CrossRef CAS
.
- Y. Wu and W. H. Hendershot, Cation exchange capacity and proton binding properties of pea (Pisum sativum L.) roots, Water, Air, Soil Pollut., 2009, 200, 353–369 CrossRef CAS
.
- E. Spielman-Sun, A. Avellan, G. D. Bland, R. V. Tappero, A. S. Acerbo, J. M. Unrine, J. P. Giraldo and G. V. Lowry, Nanoparticle surface charge influences translocation and leaf distribution in vascular plants with contrasting anatomy, Environ. Sci.: Nano, 2019, 6, 2508–2519 RSC
.
- Z. Zhu, H. Wang, B. Yan, H. Zheng, Y. Jiang, O. R. Miranda, V. M. Rotello, B. Xing and R. W. Vachet, Effect of surface charge on the uptake and distribution of gold nanoparticles in four plant species, Environ. Sci. Technol., 2012, 46, 12391–12398 CrossRef CAS PubMed
.
- P. Peharec Štefanić, K. Košpić, D. M. Lyons, L. Jurković, B. Balen and M. Tkalec, Phytotoxicity of silver nanoparticles on tobacco plants: Evaluation of coating effects on photosynthetic performance and chloroplast ultrastructure, Nanomaterials, 2021, 11, 744 CrossRef PubMed
.
- J. Wang, Y. Yang, H. Zhu, J. Braam, J. L. Schnoor and P. J. J. Alvarez, Uptake, translocation, and transformation of quantum dots with cationic versus anionic coatings by Populus deltoides × nigra cuttings, Environ. Sci. Technol., 2014, 48, 6754–6762 CrossRef CAS PubMed
.
- N. Yan and W.-X. Wang, Novel imaging of silver nanoparticle uptake by a unicellular alga and trophic transfer to Daphnia magna, Environ. Sci. Technol., 2021, 55, 5143–5151 CrossRef CAS PubMed
.
- M. Rai, A. Yadav and A. Gade, Silver nanoparticles as a new generation of antimicrobials, Biotechnol. Adv., 2009, 27, 76–83 CrossRef CAS PubMed
.
- A. R. Siekkinen, J. M. McLellan, J. Chen and Y. Xia, Rapid synthesis of small silver nanocubes by mediating polyol reduction with a trace amount of sodium sulfide or sodium hydrosulfide, Chem. Phys. Lett., 2006, 432, 491–496 CrossRef CAS PubMed
.
- A. R. Madaria, A. Kumar and C. Zhou, Large scale, highly conductive and patterned transparent films of silver nanowires on arbitrary substrates and their application in touch screens, Nanotechnology, 2011, 22, 245201 CrossRef PubMed
.
- D. E. Gorka, J. S. Osterberg, C. A. Gwin, B. P. Colman, J. N. Meyer, E. S. Bernhardt, C. K. Gunsch, R. T. DiGulio and J. Liu, Reducing environmental toxicity of silver nanoparticles through shape control, Environ. Sci. Technol., 2015, 49, 10093–10098 CrossRef CAS PubMed
.
- Y.-y. Syu, J.-H. Hung, J.-C. Chen and H.-w. Chuang, Impacts of size and shape of silver nanoparticles on Arabidopsis plant growth and gene expression, Plant Physiol. Biochem., 2014, 83, 57–64 CrossRef CAS PubMed
.
- D. E. Gorka and J. Liu, Effect of direct contact on the phytotoxicity of silver nanomaterials, Environ. Sci. Technol., 2016, 50, 10370–10376 CrossRef CAS PubMed
.
- M. Auffan, C. Santaella, L. Brousset, M. Tella, E. Morel, P. Ortet, M. Barakat, C. Chaneac, J. Issartel, B. Angeletti, C. Levard, J. L. Hazemann, M. Wiesner, J. Rose, A. Thiéry and J. Y. Bottero, The shape and speciation of Ag nanoparticles drive their impacts on organisms in a lotic ecosystem, Environ. Sci.: Nano, 2020, 7, 3167–3177 RSC
.
- X. Wang, L. Liu, W. Zhang and X. Ma, Prediction of plant uptake and translocation of engineered metallic nanoparticles by machine learning, Environ. Sci. Technol., 2021, 55, 7491–7500 CrossRef CAS PubMed
.
- C. Svendsen, L. A. Walker, M. Matzke, E. Lahive, S. Harrison, A. Crossley, B. Park, S. Lofts, I. Lynch, S. Vázquez-Campos, R. Kaegi, A. Gogos, C. Asbach, G. Cornelis, F. von der Kammer, N. W. van den Brink, C. Mays and D. J. Spurgeon, Key principles and operational practices for improved nanotechnology environmental exposure assessment, Nat. Nanotechnol., 2020, 15, 731–742 CrossRef CAS PubMed
.
- P. Wang, N. W. Menzies, P. G. Dennis, J. Guo, C. Forstner, R. Sekine, E. Lombi, P. Kappen, P. M. Bertsch and P. M. Kopittke, Silver nanoparticles entering soils via the wastewater–sludge–soil pathway pose low risk to plants but elevated Cl concentrations increase Ag bioavailability, Environ. Sci. Technol., 2016, 50, 8274–8281 CrossRef CAS PubMed
.
- J. Liu, Y. S. Hwang and J. J. Lenhart, Heteroaggregation of bare silver nanoparticles with clay minerals, Environ. Sci.: Nano, 2015, 2, 528–540 RSC
.
- Y. Liang, S. A. Bradford, J. Simunek, M. Heggen, H. Vereecken and E. Klumpp, Retention and remobilization of stabilized silver nanoparticles in an undisturbed loamy sand soil, Environ. Sci. Technol., 2013, 47, 12229–12237 CrossRef CAS PubMed
.
- D. Wang, D. P. Jaisi, J. Yan, Y. Jin and D. Zhou, Transport and retention of polyvinylpyrrolidone-coated silver nanoparticles in natural soils, Vadose Zone J., 2015, 14, 1–13 CAS
.
- Y. Liang, Y. Luo, Z. Lu, E. Klumpp, C. Shen and S. A. Bradford, Evidence on enhanced transport and release of silver nanoparticles by colloids in soil due to modification of grain surface morphology and co-transport, Environ. Pollut., 2021, 276, 116661 CrossRef CAS PubMed
.
- N. Saleeb, R. Gooneratne, J. Cavanagh, C. Bunt, A. K. M. M. Hossain, S. Gaw and B. Robinson, The mobility of silver nanoparticles and silver ions in the soil-plant system, J. Environ. Qual., 2019, 48, 1835–1841 CrossRef CAS
.
- C. Layet, C. Santaella, M. Auffan, C. Chevassus-Rosset, M. Montes, C. Levard, P. Ortet, M. Barakat and E. Doelsch, Phytoavailability of silver at predicted environmental concentrations: Does the initial ionic or nanoparticulate form matter?, Environ. Sci.: Nano, 2019, 6, 127–135 RSC
.
- D. Zhou, A. I. Abdel-Fattah and A. A. Keller, Clay particles destabilize engineered nanoparticles in aqueous environments, Environ. Sci. Technol., 2012, 46, 7520–7526 CrossRef CAS PubMed
.
- M. Hoppe, R. Mikutta, J. Utermann, W. Duijnisveld and G. Guggenberger, Retention of sterically and electrosterically stabilized silver nanoparticles in soils, Environ. Sci. Technol., 2014, 48, 12628–12635 CrossRef CAS PubMed
.
- R. Wang, H. Du, Y. Wang, D. Wang, Q. Sun and D. Zhou, Retention of silver nanoparticles and silver ion to natural soils: Effects of soil physicochemical properties, J. Soils Sediments, 2018, 18, 2491–2499 CrossRef CAS
.
- C. Peng, S. Chen, C. Shen, M. He, Y. Zhang, J. Ye, J. Liu and J. Shi, Iron plaque: A barrier layer to the uptake and translocation of copper oxide nanoparticles by rice plants, Environ. Sci. Technol., 2018, 52, 12244–12254 CrossRef CAS PubMed
.
- Y. Wu, L. Yang, H. Gong, F. Dang and D. Zhou, Contrasting effects of iron plaque on the bioavailability of metallic and sulfidized silver nanoparticles to rice, Environ. Pollut., 2020, 260, 113969 CrossRef CAS PubMed
.
- Q. Yang, W. Xu, G. Liu, M. Song, Z. Tan, Y. Mao, Y. Yin, Y. Cai, J. Liu and G. Jiang, Transformation and uptake of silver nanoparticles and silver ions in rice plant (Oryza sativa L.): The effect of iron plaque and dissolved iron, Environ. Sci.: Nano, 2020, 7, 599–609 RSC
.
- W. J. Liu, Y. G. Zhu, Y. Hu, P. N. Williams, A. G. Gault, A. A. Meharg, J. M. Charnock and F. A. Smith, Arsenic sequestration in iron plaque, its accumulation and speciation in mature rice plants (Oryza Sativa L.), Environ. Sci. Technol., 2006, 40, 5730–5736 CrossRef CAS PubMed
.
- R. D. Tripathi, P. Tripathi, S. Dwivedi, A. Kumar, A. Mishra, P. S. Chauhan, G. J. Norton and C. S. Nautiyal, Roles for root iron plaque in sequestration and uptake of heavy metals and metalloids in aquatic and wetland plants, Metallomics, 2014, 6, 1789–1800 CrossRef CAS PubMed
.
- R. Wang, F. Dang, C. Liu, D. Wang, P. Cui, H. Yan and D. Zhou, Heteroaggregation and dissolution of silver nanoparticles by iron oxide colloids under environmentally relevant conditions, Environ. Sci.: Nano, 2019, 6, 195–206 RSC
.
- W. Cai, Y. Wang, F. Dang and D. Zhou, Copper pre-exposure reduces AgNP bioavailability to wheat, Sci. Total Environ., 2020, 707, 136084 CrossRef CAS PubMed
.
- W. Cao, J. Gong, G. Zeng, B. Song, P. Zhang, J. Li, S. Fang, L. Qin, J. Ye and Z. Cai, Mutual effects of silver nanoparticles and antimony(iii)/(v) co-exposed to Glycine max (L.) Merr. in hydroponic systems: Uptake, translocation, physiochemical responses, and potential mechanisms, Environ. Sci.: Nano, 2020, 7, 2691–2707 RSC
.
- L. Rossi, H. Sharifan, W. Zhang, A. P. Schwab and X. Ma, Mutual effects and in planta accumulation of co-existing cerium oxide nanoparticles and cadmium in hydroponically grown soybean (Glycine max (L.) Merr.), Environ. Sci.: Nano, 2018, 5, 150–157 RSC
.
- T. Zhou, S. Prasher, Z. Qi, S. George, A. Mawof, C. Nzediegwu, J. Dhiman and R. Patel, Impact of silver nanoparticles in wastewater on heavy metal transport in soil and uptake by radish plants, Water, Air, Soil Pollut., 2021, 232, 267 CrossRef CAS
.
- R. De La Torre-Roche, J. Hawthorne, C. Musante, B. Xing, L. A. Newman, X. Ma and J. C. White, Impact of Ag nanoparticle exposure on p,p'-DDE bioaccumulation by Cucurbita pepo (Zucchini) and Glycine max (Soybean), Environ. Sci. Technol., 2013, 47, 718–725 CrossRef CAS PubMed
.
- X. Li, M. Ke, M. Zhang, W. J. G. M. Peijnenburg, X. Fan, J. Xu, Z. Zhang, T. Lu, Z. Fu and H. Qian, The interactive effects of diclofop-methyl and silver nanoparticles on Arabidopsis thaliana: Growth, photosynthesis and antioxidant system, Environ. Pollut., 2018, 232, 212–219 CrossRef CAS PubMed
.
- J. Zhu, Z. Zou, Y. Shen, J. Li, S. Shi, S. Han and X. Zhan, Increased ZnO nanoparticle toxicity to wheat upon co-exposure to phenanthrene, Environ. Pollut., 2019, 247, 108–117 CrossRef CAS PubMed
.
- C. Ma, H. Liu, G. Chen, Q. Zhao, B. Eitzer, Z. Wang, W. Cai, L. A. Newman, J. C. White, O. P. Dhankher and B. Xing, Effects of titanium oxide nanoparticles on tetracycline accumulation and toxicity in Oryza sativa (L.), Environ. Sci.: Nano, 2017, 4, 1827–1839 RSC
.
- Y. Wen, L. Zhang, Z. Chen, X. Sheng, J. Qiu and D. Xu, Co-exposure of silver nanoparticles and chiral herbicide imazethapyr to Arabidopsis thaliana: Enantioselective effects, Chemosphere, 2016, 145, 207–214 CrossRef CAS PubMed
.
- R. De La Torre-Roche, J. Hawthorne, Y. Deng, B. Xing, W. Cai, L. A. Newman, C. Wang, X. Ma and J. C. White, Fullerene-enhanced accumulation of p,p'-DDE in agricultural crop species, Environ. Sci. Technol., 2012, 46, 9315–9323 CrossRef CAS PubMed
.
- R. De La Torre Roche, L. Pagano, S. Majumdar, B. D. Eitzer, N. Zuverza-Mena, C. Ma, A. D. Servin, N. Marmiroli, O. P. Dhankher and J. C. White, Co-exposure of imidacloprid and nanoparticle Ag or CeO2 to Cucurbita pepo (zucchini): Contaminant bioaccumulation and translocation, NanoImpact, 2018, 11, 136–145 CrossRef
.
- P. Courtois, A. Rorat, S. Lemiere, R. Guyoneaud, E. Attard, C. Levard and F. Vandenbulcke, Ecotoxicology of silver nanoparticles and their derivatives introduced in soil with or without sewage sludge: A review of effects on microorganisms, plants and animals, Environ. Pollut., 2019, 253, 578–598 CrossRef CAS PubMed
.
- R. Sekine, M. Khaksar, G. Brunetti, E. Donner, K. G. Scheckel, E. Lombi and K. Vasilev, Surface immobilization of engineered nanomaterials for in situ study of their environmental transformations and fate, Environ. Sci. Technol., 2013, 47, 9308–9316 CrossRef CAS PubMed
.
- B. Thalmann, A. Voegelin, E. Morgenroth and R. Kaegi, Effect of humic acid on the kinetics of silver nanoparticle sulfidation, Environ. Sci.: Nano, 2016, 3, 203–212 RSC
.
- C. Levard, E. M. Hotze, B. P. Colman, A. L. Dale, L. Truong, X. Y. Yang, A. J. Bone, G. E. Brown, R. L. Tanguay, R. T. Di Giulio, E. S. Bernhardt, J. N. Meyer, M. R. Wiesner and G. V. Lowry, Sulfidation of silver nanoparticles: Natural antidote to their toxicity, Environ. Sci. Technol., 2013, 47, 13440 CrossRef CAS PubMed
.
- D. L. Starnes, J. M. Unrine, C. P. Starnes, B. E. Collin, E. K. Oostveen, R. Ma, G. V. Lowry, P. M. Bertsch and O. V. Tsyusko, Impact of sulfidation on the bioavailability and toxicity of silver nanoparticles to Caenorhabditis elegans, Environ. Pollut., 2015, 196, 239–246 CrossRef CAS PubMed
.
- P. Wang, N. W. Menzies, E. Lombi, R. Sekine, F. P. C. Blamey, M. C. Hernandez-Soriano, M. Cheng, P. Kappen, W. J. G. M. Peijnenburg, C. Tang and P. M. Kopittke, Silver sulfide nanoparticles (Ag2S-NPs) are taken up by plants and are phytotoxic, Nanotoxicology, 2015, 9, 1041–1049 CrossRef PubMed
.
- J. P. Stegemeier, F. Schwab, B. P. Colman, S. M. Webb, M. Newville, A. Lanzirotti, C. Winkler, M. R. Wiesner and G. V. Lowry, Speciation matters: Bioavailability
of silver and silver sulfide nanoparticles to alfalfa (Medicago sativa), Environ. Sci. Technol., 2015, 49, 8451–8460 CrossRef CAS PubMed
.
- C. L. Doolette, M. J. McLaughlin, J. K. Kirby and D. A. Navarro, Bioavailability of silver and silver sulfide nanoparticles to lettuce (Lactuca sativa): Effect of agricultural amendments on plant uptake, J. Hazard. Mater., 2015, 300, 788–795 CrossRef CAS PubMed
.
- Z. Niu, Y. Yang, F. Tou, X. Guo, R. Huang, J. Xu, Y. Chen, L. Hou, M. Liu and M. F. Hochella, Sulfate-reducing bacteria (SRB) can enhance the uptake of silver-containing nanoparticles by a wetland plant, Environ. Sci.: Nano, 2020, 7, 912–925 RSC
.
- P. Wang, E. Lombi, S. Sun, K. G. Scheckel, A. Malysheva, B. A. McKenna, N. W. Menzies, F. Zhao and P. M. Kopittke, Characterizing the uptake, accumulation and toxicity of silver sulfide nanoparticles in plants, Environ. Sci.: Nano, 2017, 4, 448–460 RSC
.
- M. Azodi, Y. Sultan and S. Ghoshal, Dissolution behavior of silver nanoparticles and formation of secondary silver nanoparticles in municipal wastewater by single-particle ICP-MS, Environ. Sci. Technol., 2016, 50, 13318–13327 CrossRef CAS PubMed
.
- I. L. Gunsolus, M. P. S. Mousavi, K. Hussein, P. Bühlmann and C. L. Haynes, Effects of humic and fulvic acids on silver nanoparticle stability, dissolution, and toxicity, Environ. Sci. Technol., 2015, 49, 8078–8086 CrossRef CAS PubMed
.
- X. Gao, S. M. Rodrigues, E. Spielman-Sun, S. Lopes, S. Rodrigues, Y. Zhang, A. Avellan, R. Duarte, A. Duarte, E. A. Casman and G. V. Lowry, Effect of soil organic matter, soil pH, and moisture content on solubility and dissolution rate of CuO NPs in soil, Environ. Sci. Technol., 2019, 53, 4959–4967 CrossRef CAS PubMed
.
- Y. Li, H. Chen, F. Wang, F. Zhao, X. Han, H. Geng, L. Gao, H. Chen, R. Yuan and J. Yao, Environmental behavior and associated plant accumulation of silver nanoparticles in the presence of dissolved humic and fulvic acid, Environ. Pollut., 2018, 243, 1334–1342 CrossRef CAS PubMed
.
- X. Huang, Y. Li, K. Chen, H. Chen, F. Wang, X. Han, B. Zhou, H. Chen and R. Yuan, NOM mitigates the phytotoxicity of AgNPs by regulating rice physiology, root cell wall components and root morphology, Environ. Pollut., 2020, 260, 113942 CrossRef CAS PubMed
.
- M. Li, F. Dang, Q. Fu, D. Zhou and B. Yin, Effects of molecular weight-fractionated natural organic matter on the phytoavailability of silver nanoparticles, Environ. Sci.: Nano, 2018, 5, 969–979 RSC
.
- Y. Ding, X. Bai, Z. Ye, D. Gong, J. Cao and Z. Hua, Humic acid regulation of the environmental behavior and phytotoxicity of silver nanoparticles to Lemna minor, Environ. Sci.: Nano, 2019, 6, 3712–3722 RSC
.
- S. Li, S. Wang, B. Yan and T. Yue, Surface properties of nanoparticles dictate their toxicity by regulating adsorption of humic acid molecules, ACS Sustainable Chem. Eng., 2021, 9, 13705–13716 CrossRef CAS
.
- T. Zhu, D. F. Lawler, Y. Chen and B. L. T. Lau, Effects of natural organic matter and sulfidation on the flocculation and filtration of silver nanoparticles, Environ. Sci.: Nano, 2016, 3, 1436–1446 RSC
.
- C.-W. Yang, L. Yuan, H.-Z. Zhou, X. Zhang and G.-P. Sheng, Coating ligand-mediated dynamic formation of natural organic matter (NOM) corona on engineered nanoparticles in natural environments, Environ. Sci.: Nano, 2021, 8, 1029–1041 RSC
.
- K. E. Wheeler, A. J. Chetwynd, K. M. Fahy, B. S. Hong, J. A. Tochihuitl, L. A. Foster and I. Lynch, Environmental dimensions of the protein corona, Nat. Nanotechnol., 2021, 16, 617–629 CrossRef CAS PubMed
.
- X. Wang, W. Fan, Z. Dong, D. Liang and T. Zhou, Interactions of natural organic matter on the surface of PVP-capped silver nanoparticle under different aqueous environment, Water Res., 2018, 138, 224–233 CrossRef CAS PubMed
.
- A. R. Whitley, C. Levard, E. Oostveen, P. M. Bertsch, C. J. Matocha, F. V. D. Kammer and J. M. Unrine, Behavior of Ag nanoparticles in soil: Effects of particle surface coating, aging and sewage sludge amendment, Environ. Pollut., 2013, 182, 141 CrossRef CAS PubMed
.
- J. Hou, M. Zhang, P. Wang, C. Wang, L. Miao, Y. Xu, G. You, B. Lv, Y. Yang and Z. Liu, Transport, retention, and long-term release behavior of polymer-coated silver nanoparticles in saturated quartz sand: The impact of natural organic matters and electrolyte, Environ. Pollut., 2017, 229, 49–59 CrossRef CAS PubMed
.
- C. M. Park, J. Heo, N. Her, K. H. Chu, M. Jang and Y. Yoon, Modeling the effects of surfactant, hardness, and natural organic matter on deposition and mobility of silver nanoparticles in saturated porous media, Water Res., 2016, 103, 38–47 CrossRef CAS PubMed
.
- J. Lehmann and M. Kleber, The contentious nature of soil organic matter, Nature, 2015, 528, 60–68 CrossRef CAS PubMed
.
- A. T. Sutton, R. D. Arrua, S. C. Thickett, E. Lombi and E. F. Hilder, Understanding the interaction of gold and silver nanoparticles with natural organic matter using affinity capillary electrophoresis, Environ. Sci.: Nano, 2019, 6, 1351–1362 RSC
.
- M. Delay, T. Dolt, A. Woellhaf, R. Sembritzki and F. H. Frimmel, Interactions and stability of silver nanoparticles in the aqueous phase: Influence of natural organic matter (NOM) and ionic strength, J. Chromatogr. A, 2011, 1218, 4206–4212 CrossRef CAS PubMed
.
- X.-D. Sun, X.-Z. Yuan, Y. Jia, L.-J. Feng, F.-P. Zhu, S.-S. Dong, J. Liu, X. Kong, H. Tian, J.-L. Duan, Z. Ding, S.-G. Wang and B. Xing, Differentially charged nanoplastics demonstrate distinct accumulation in Arabidopsis thaliana, Nat. Nanotechnol., 2020, 15, 755–760 CrossRef CAS PubMed
.
- L. Wei, Q. Zhang, X. Hou, G. Qu, J. Liu and G. Jiang, Species-dependent eco-corona dictates the aggregation of black phosphorus nanosheets: The role of protein and calcium, Environ. Sci.: Nano, 2021, 8, 3098–3109 RSC
.
- P. Cervantes-Avilés, X. Huang and A. A. Keller, Dissolution and aggregation of metal oxide nanoparticles in root exudates and soil leachate: Implications for nanoagrochemical application, Environ. Sci. Technol., 2021, 55, 13443–13451 CrossRef PubMed
.
- S. He, Y. Feng, J. Ni, Y. Sun, L. Xue, Y. Feng, Y. Yu, X. Lin and L. Yang, Different responses of soil microbial metabolic activity to silver and iron oxide nanoparticles, Chemosphere, 2016, 147, 195–202 CrossRef CAS PubMed
.
- P. Courtois, A. Rorat, S. Lemiere, R. Guyoneaud, E. Attard, M. Longepierre, F. Rigal, C. Levard, P. Chaurand, A. Grosser, A. Grobelak, M. Kacprzak, C. Lors, A. Richaume and F. Vandenbulcke, Medium-term effects of Ag supplied directly or via sewage sludge to an agricultural soil on Eisenia fetida earthworm and soil microbial communities, Chemosphere, 2021, 269, 128761 CrossRef CAS PubMed
.
- J. Wang, K. Shu, L. Zhang and Y. Si, Effects of silver nanoparticles on soil microbial communities and bacterial nitrification in suburban vegetable soils, Pedosphere, 2017, 27, 482–490 CrossRef CAS
.
- X. Zou, P. Li, X. Wang, S. Zheng, F. Dai and H. Zhang, Silver nanoparticle and Ag+-induced shifts of microbial communities in natural brackish waters: Are they more pronounced under oxic conditions than anoxic conditions?, Environ. Pollut., 2020, 258, 113686 CrossRef CAS PubMed
.
- H. Zhang, M. Huang, W. Zhang, J. L. Gardea-Torresdey, J. C. White, R. Ji and L. Zhao, Silver nanoparticles alter soil microbial community compositions and metabolite profiles in unplanted and cucumber-planted soils, Environ. Sci. Technol., 2020, 54, 3334–3342 CrossRef CAS PubMed
.
- G. Montes de Oca-Vásquez, F. Solano-Campos, J. R. Vega-Baudrit, R. López-Mondéjar, A. Vera, J. L. Moreno and F. Bastida, Organic amendments exacerbate the effects of silver nanoparticles on microbial biomass and community composition of a semiarid soil, Sci. Total Environ., 2020, 744, 140919 CrossRef PubMed
.
- C. A. Ottoni, M. C. Lima Neto, P. Leo, B. D. Ortolan, E. Barbieri and A. O. De Souza, Environmental impact of biogenic silver nanoparticles in soil and aquatic organisms, Chemosphere, 2020, 239, 124698 CrossRef CAS PubMed
.
- J. P. Fernandes, A. P. Mucha, T. Francisco, C. R. Gomes and C. M. R. Almeida, Silver nanoparticles uptake by salt marsh plants - Implications for phytoremediation processes and effects in microbial community dynamics, Mar. Pollut. Bull., 2017, 119, 176–183 CrossRef CAS PubMed
.
- Y. Feng, X. Cui, S. He, G. Dong, M. Chen, J. Wang and X. Lin, The role of metal nanoparticles in influencing arbuscular mycorrhizal fungi effects on plant growth, Environ. Sci. Technol., 2013, 47, 9496–9504 CrossRef CAS PubMed
.
- F. Wang, K. Li and Z. Shi, Phosphorus fertilization and mycorrhizal colonization change silver nanoparticle impacts on maize, Ecotoxicology, 2021, 30, 118–129 CrossRef CAS PubMed
.
- J. Cao, Y. Feng, X. Lin and J. Wang, A beneficial role of arbuscular mycorrhizal fungi in influencing the effects of silver nanoparticles on plant-microbe systems in a soil matrix, Environ. Sci. Pollut. Res., 2020, 27, 11782–11796 CrossRef CAS PubMed
.
- A. Noori, J. C. White and L. A. Newman, Mycorrhizal fungi influence on silver uptake and membrane protein gene expression following silver nanoparticle exposure, J. Nanopart. Res., 2017, 19, 66 CrossRef
.
- J. Cao, Y. Feng, S. He and X. Lin, Silver nanoparticles deteriorate the mutual interaction between maize (Zea mays L.) and arbuscular mycorrhizal fungi: A soil microcosm study, Appl. Soil Ecol., 2017, 119, 307–316 CrossRef
.
- M. Pittol, D. Tomacheski, D. N. Simões, V. F. Ribeiro and R. M. C. Santana, Macroscopic effects of silver nanoparticles and titanium dioxide on edible plant growth, Environ. Nanotechnol. Monit. Manag., 2017, 8, 127–133 Search PubMed
.
- L. Yuan, C. J. Richardson, M. Ho, C. W. Willis, B. P. Colman and M. R. Wiesner, Stress responses of aquatic plants to silver nanoparticles, Environ. Sci. Technol., 2018, 52, 2558–2565 CrossRef CAS PubMed
.
- P. Pardha-Saradhi, N. Shabnam, P. Sharmila, A. K. Ganguli and H. Kim, Differential sensitivity of light-harnessing photosynthetic events in wheat and sunflower to exogenously applied ionic and nanoparticulate silver, Chemosphere, 2018, 194, 340–351 CrossRef CAS PubMed
.
- P. Zhang, Y. Ma, C. Xie, Z. Guo, X. He, E. Valsami-Jones, I. Lynch, W. Luo, L. Zheng and Z. Zhang, Plant species-dependent transformation and translocation of ceria nanoparticles, Environ. Sci.: Nano, 2019, 6, 60–67 RSC
.
- A. E. Pradas del Real, V. Vidal, M. Carrière, H. Castillo-Michel, C. Levard, P. Chaurand and G. Sarret, Silver nanoparticles and wheat roots: A complex interplay, Environ. Sci. Technol., 2017, 51, 5774–5782 CrossRef CAS PubMed
.
- J. Lv, P. Christie and S. Zhang, Uptake, translocation, and transformation of metal-based nanoparticles in plants: Recent advances and methodological challenges, Environ. Sci.: Nano, 2019, 6, 41–59 RSC
.
- A. Noori, T. Donnelly, J. Colbert, W. Cai, L. A. Newman and J. C. White, Exposure of tomato (Lycopersicon esculentum) to silver nanoparticles and silver nitrate: Physiological and molecular response, Int. J. Phytorem., 2020, 22, 40–51 CrossRef CAS PubMed
.
- J. Wu, G. Wang, M. G. Vijver, T. Bosker and W. J. G. M. Peijnenburg, Foliar versus root exposure of AgNPs to lettuce: Phytotoxicity, antioxidant responses and internal translocation, Environ. Pollut., 2020, 261, 114117 CrossRef CAS PubMed
.
- J. Bailey-Serres, J. E. Parker, E. A. Ainsworth, G. E. D. Oldroyd and J. I. Schroeder, Genetic strategies for improving crop yields, Nature, 2019, 575, 109–118 CrossRef CAS PubMed
.
- W. Wang, B. Vinocur and A. Altman, Plant responses to drought, salinity and extreme temperatures: Towards genetic engineering for stress tolerance, Planta, 2003, 218, 1–14 CrossRef CAS PubMed
.
- L. Zhao, L. Lu, A. Wang, H. Zhang, M. Huang, H. Wu, B. Xing, Z. Wang and R. Ji, Nano-biotechnology in agriculture: Use of nanomaterials to promote plant growth and stress tolerance, J. Agric. Food Chem., 2020, 68, 1935–1947 CrossRef CAS PubMed
.
- L. Rossi, W. Zhang, L. Lombardini and X. Ma, The impact of cerium oxide nanoparticles on the salt stress responses of Brassica napus L, Environ. Pollut., 2016, 219, 28–36 CrossRef CAS PubMed
.
- A. Cox, P. Venkatachalam, S. Sahi and N. Sharma, Silver and titanium dioxide nanoparticle toxicity in plants: A review of current research, Plant Physiol. Biochem., 2016, 107, 147–163 CrossRef CAS PubMed
.
- A. Yan and Z. Chen, Impacts of silver nanoparticles on plants: A focus on the phytotoxicity and underlying mechanism, Int. J. Mol. Sci., 2019, 20, 1003 CrossRef CAS PubMed
.
- F. Mirzajani, H. Askari, S. Hamzelou, Y. Schober, A. Römpp, A. Ghassempour and B. Spengler, Proteomics study of silver nanoparticles toxicity on Oryza sativa L, Ecotoxicol. Environ. Saf., 2014, 108, 335–339 CrossRef CAS PubMed
.
- B. Fadeel, L. Farcal, B. Hardy, S. Vázquez-Campos, D. Hristozov, A. Marcomini, I. Lynch, E. Valsami-Jones, H. Alenius and K. Savolainen, Advanced tools for the safety assessment of nanomaterials, Nat. Nanotechnol., 2018, 13, 537–543 CrossRef CAS PubMed
.
- M. Revel, A. Châtel and C. Mouneyrac, Omics tools: New challenges in aquatic nanotoxicology?, Aquat. Toxicol., 2017, 193, 72–85 CrossRef CAS PubMed
.
- R. Ruotolo, E. Maestri, L. Pagano, M. Marmiroli, J. C. White and N. Marmiroli, Plant response to metal-containing engineered nanomaterials: An omics-based perspective, Environ. Sci. Technol., 2018, 52, 2451–2467 CrossRef CAS PubMed
.
- X. Huang, P. Cervantes-Avilés, W. Li and A. A. Keller, Drilling into the metabolomics to enhance insight on corn and wheat responses to molybdenum trioxide nanoparticles, Environ. Sci. Technol., 2021, 55, 13452–13464 CrossRef CAS PubMed
.
- J. Geisler-Lee, M. Brooks, J. R. Gerfen, Q. Wang, C. Fotis, A. Sparer, X. Ma, R. H. Berg and M. Geisler, Reproductive toxicity and life history study of silver nanoparticle effect, uptake and transport in Arabidopsis thaliana, Nanomaterials, 2014, 4, 301–318 CrossRef PubMed
.
- D. Bao, Z. G. Oh and Z. Chen, Characterization of silver nanoparticles internalized by Arabidopsis plants using single particle ICP-MS analysis, Front. Plant Sci., 2016, 7, 32 Search PubMed
.
- X. Ma, J. Geisler-Lee, Y. Deng and A. Kolmakov, Interactions between engineered nanoparticles (ENPs) and plants: Phytotoxicity, uptake and accumulation, Sci. Total Environ., 2010, 408, 3053–3061 CrossRef CAS PubMed
.
- F. Mirzajani, H. Askari, S. Hamzelou, M. Farzaneh and A. Ghassempour, Effect of silver nanoparticles on Oryza sativa L. and its rhizosphere bacteria, Ecotoxicol. Environ. Saf., 2013, 88, 48–54 CrossRef CAS PubMed
.
- P. Cvjetko, M. Zovko, P. P. Štefanić, R. Biba, M. Tkalec, A.-M. Domijan, I. V. Vrček, I. Letofsky-Papst, S. Šikić and B. Balen, Phytotoxic effects of silver nanoparticles in tobacco plants, Environ. Sci. Pollut. Res., 2018, 25, 5590–5602 CrossRef CAS PubMed
.
- D. Lin and B. Xing, Root uptake and phytotoxicity of ZnO nanoparticles, Environ. Sci. Technol., 2008, 42, 5580–5585 CrossRef CAS PubMed
.
- C. Peng, D. Duan, C. Xu, Y. Chen, L. Sun, H. Zhang, X. Yuan, L. Zheng, Y. Yang, J. Yang, X. Zhen, Y. Chen and J. Shi, Translocation and biotransformation of CuO nanoparticles in rice (Oryza sativa L.) plants, Environ. Pollut., 2015, 197, 99–107 CrossRef CAS PubMed
.
- J. Lv, S. Zhang, L. Luo, J. Zhang, K. Yang and P. Christie, Accumulation, speciation and uptake pathway of ZnO nanoparticles in maize, Environ. Sci.: Nano, 2015, 2, 68–77 RSC
.
- S. Wolf, W. J. Lucas, C. M. Deom and R. N. Beachy, Movement protein of tobacco mosaic virus modifies plasmodesmatal size exclusion limit, Science, 1989, 246, 377 CrossRef CAS PubMed
.
- K. Bell and K. Oparka, Imaging plasmodesmata, Protoplasma, 2011, 248, 9–25 CrossRef PubMed
.
- K. Ehlers and R. Kollmann, Primary and secondary plasmodesmata: Structure, origin, and functioning, Protoplasma, 2001, 216, 1 CrossRef CAS PubMed
.
- B. C. Reagan, E. E. Ganusova, J. C. Fernandez, T. N. McCray and T. M. Burch-Smith, RNA on the move: The plasmodesmata perspective, Plant Sci., 2018, 275, 1–10 CrossRef CAS PubMed
.
- Y. Ma, X. He, P. Zhang, Z. Zhang, Y. Ding, J. Zhang, G. Wang, C. Xie, W. Luo, J. Zhang, L. Zheng, Z. Chai and K. Yang, Xylem and phloem based transport of CeO2 nanoparticles in hydroponic cucumber plants, Environ. Sci. Technol., 2017, 51, 5215–5221 CrossRef CAS PubMed
.
- N. A. Anjum, M. A. M. Rodrigo, A. Moulick, Z. Heger, P. Kopel, O. Zítka, V. Adam, A. S. Lukatkin, A. C. Duarte, E. Pereira and R. Kizek, Transport phenomena of nanoparticles in plants and animals/humans, Environ. Res., 2016, 151, 233–243 CrossRef CAS PubMed
.
- C. Cocozza, A. Perone, C. Giordano, M. C. Salvatici, S. Pignattelli, A. Raio, M. Schaub, K. Sever, J. L. Innes, R. Tognetti and P. Cherubini, Silver nanoparticles enter the tree stem faster through leaves than through roots, Tree Physiol., 2019, 39, 1251–1261 CrossRef CAS PubMed
.
- A. Pérez-de-Luque, Interaction of nanomaterials with plants: What do we need for real applications in agriculture?, Front. Environ. Sci., 2017, 5, 12 Search PubMed
.
- A. Noori, A. Ngo, P. Gutierrez, S. Theberge and J. C. White, Silver nanoparticle detection and accumulation in tomato (Lycopersicon esculentum), J. Nanopart. Res., 2020, 22, 131 CrossRef CAS
.
- E. Spielman-Sun, E. Lombi, E. Donner, D. Howard, J. M. Unrine and G. V. Lowry, Impact of surface charge on cerium oxide nanoparticle uptake and translocation by wheat (Triticum aestivum), Environ. Sci. Technol., 2017, 51, 7361–7368 CrossRef CAS PubMed
.
- A. Avellan, F. Schwab, A. Masion, P. Chaurand, D. Borschneck, V. Vidal, J. Rose, C. Santaella and C. Levard, Nanoparticle uptake in plants: Gold nanomaterial localized in roots of Arabidopsis thaliana by X-ray computed nanotomography and hyperspectral imaging, Environ. Sci. Technol., 2017, 51, 8682–8691 CrossRef CAS PubMed
.
- M. Liu, S. Feng, Y. Ma, C. Xie, X. He, Y. Ding, J. Zhang, W. Luo, L. Zheng, D. Chen, F. Yang, Z. Chai, Y. Zhao and Z. Zhang, Influence of surface charge on the phytotoxicity, transformation, and translocation of CeO2 nanoparticles in cucumber plants, ACS Appl. Mater. Interfaces, 2019, 11, 16905–16913 CrossRef CAS PubMed
.
- C. Vannini, G. Domingo, E. Onelli, F. De Mattia, I. Bruni, M. Marsoni and M. Bracale, Phytotoxic and genotoxic effects of silver nanoparticles exposure on germinating wheat seedlings, J. Plant Physiol., 2014, 171, 1142–1148 CrossRef CAS PubMed
.
- R. M. Galazzi and M. A. Z. Arruda, Evaluation of changes in the macro and micronutrients homeostasis of transgenic and non-transgenic soybean plants after cultivation with silver nanoparticles through ionomic approaches, J. Trace Elem. Med. Biol., 2018, 48, 181–187 CrossRef CAS PubMed
.
- T. L. Read, C. L. Doolette, N. R. Howell, P. M. Kopittke, T. Cresswell and E. Lombi, Zinc accumulates in the nodes of wheat following the foliar application of 65Zn oxide nano- and microparticles, Environ. Sci. Technol., 2021, 55, 13523–13531 CrossRef CAS PubMed
.
- M. Yilmaz, A. Yilmaz, A. Karaman, F. Aysin and O. Aksakal, Monitoring chemically and green-synthesized silver nanoparticles in maize seedlings via surface-enhanced Raman spectroscopy (SERS) and their phytotoxicity evaluation, Talanta, 2021, 225, 121952 CrossRef CAS PubMed
.
- K. K. Das, Y. You, M. Torres, F. Barrios-Masias, X. Wang, S. Tao, B. Xing and Y. Yang, Development and application of a digestion-Raman analysis approach for studying multiwall carbon nanotube uptake in lettuce, Environ. Sci.: Nano, 2018, 5, 659–668 RSC
.
- D. Metarapi, J. T. van Elteren, M. Šala, K. Vogel-Mikuš, I. Arčon, V. S. Šelih, M. Kolar and S. B. Hočevar, Laser ablation-single-particle-inductively coupled plasma mass spectrometry as a multimodality bioimaging tool in nano-based omics, Environ. Sci.: Nano, 2021, 8, 647–656 RSC
.
- W. Li, T. Qing, C. Li, F. Li, F. Ge, J. Fei and W. J. G. M. Peijnenburg, Integration of subcellular partitioning and chemical forms to understand silver nanoparticles toxicity to lettuce (Lactuca sativa L.) under different exposure pathways, Chemosphere, 2020, 258, 127349 CrossRef CAS PubMed
.
- J. P. Stegemeier, B. P. Colman, F. Schwab, M. R. Wiesner and G. V. Lowry, Uptake and distribution of silver in the aquatic plant Landoltia punctata (duckweed) exposed to silver and silver sulfide nanoparticles, Environ. Sci. Technol., 2017, 51, 4936–4943 CrossRef CAS PubMed
.
- S. Wang, J. Lv, J. Ma and S. Zhang, Cellular internalization and intracellular biotransformation of silver nanoparticles in Chlamydomonas reinhardtii, Nanotoxicology, 2016, 10, 1129–1135 CrossRef CAS PubMed
.
- S. M. Savassa, H. Castillo-Michel, A. E. Pradas del Real, J. Reyes-Herrera, J. P. R. Marques and H. W. P. de Carvalho, Ag nanoparticles enhancing Phaseolus vulgaris seedling development: Understanding nanoparticle migration and chemical transformation across the seed coat, Environ. Sci.: Nano, 2021, 8, 493–501 RSC
.
- T. Miclăuş, C. Beer, J. Chevallier, C. Scavenius, V. E. Bochenkov, J. J. Enghild and D. S. Sutherland, Dynamic protein coronas revealed as a modulator of silver nanoparticle sulphidation in vitro, Nat. Commun., 2016, 7, 11770 CrossRef PubMed
.
- L. Sigg and U. Lindauer, Silver nanoparticle dissolution in the presence of ligands and of hydrogen peroxide, Environ. Pollut., 2015, 206, 582–587 CrossRef CAS PubMed
.
- R. A. Bell and J. R. Kramer, Structural chemistry and geochemistry of silver-sulfur compounds: Critical review, Environ. Toxicol. Chem., 1999, 18, 9–22 CAS
.
- K. H. Caffall and D. Mohnen, The structure, function, and biosynthesis of plant cell wall pectic polysaccharides, Carbohydr. Res., 2009, 344, 1879–1900 CrossRef CAS PubMed
.
- L. Marchiol, A. Mattiello, F. Pošćić, C. Giordano and R. Musetti,
In vivo synthesis of nanomaterials in plants: Location of silver nanoparticles and plant metabolism, Nanoscale Res. Lett., 2014, 9, 101 CrossRef PubMed
.
- K. S. Siddiqi, A. Husen and R. A. K. Rao, A review on biosynthesis of silver nanoparticles and their biocidal properties, J. Nanobiotechnol., 2018, 16, 14 CrossRef PubMed
.
- A. K. Saim, F. N. Kumah and M. N. Oppong, Extracellular and intracellular synthesis of gold and silver nanoparticles by living plants: A review, Nanotechnol. Environ. Eng., 2020, 6, 1 Search PubMed
.
- A. Malysheva, A. Ivask, C. L. Doolette, N. H. Voelcker and E. Lombi, Cellular binding, uptake and biotransformation of silver nanoparticles in human T lymphocytes, Nat. Nanotechnol., 2021, 16, 926–932 CrossRef CAS PubMed
.
- J. Zhao, W. Ren, Y. Dai, L. Liu, Z. Wang, X. Yu, J. Zhang, X. Wang and B. Xing, Uptake, distribution, and transformation of CuO NPs in a floating plant Eichhornia crassipes and related stomatal responses, Environ. Sci. Technol., 2017, 51, 7686–7695 CrossRef CAS PubMed
.
- A. D. Dwivedi, H. Yoon, J. P. Singh, K. H. Chae, S.-c. Rho, D. S. Hwang and Y.-S. Chang, Uptake, distribution, and transformation of zerovalent iron nanoparticles in the edible plant Cucumis sativus, Environ. Sci. Technol., 2018, 52, 10057–10066 CrossRef CAS PubMed
.
- L. Wang, T. Zhang, P. Li, W. Huang, J. Tang, P. Wang, J. Liu, Q. Yuan, R. Bai, B. Li, K. Zhang, Y. Zhao and C. Chen, Use of synchrotron radiation-analytical techniques to reveal chemical origin of silver-nanoparticle cytotoxicity, ACS Nano, 2015, 9, 6532–6547 CrossRef CAS PubMed
.
- N. Yan, B. Z. Tang and W. Wang, Intracellular trafficking of silver nanoparticles and silver ions determined their specific mitotoxicity to the zebrafish cell line, Environ. Sci.: Nano, 2021, 8, 1364–1375 RSC
.
- Y. Dai, J. Zhao, X. Liu, X. Yu, Z. Jiang, Y. Bu, Z. Xu, Z. Wang, X. Zhu and B. Xing, Transformation and species identification of CuO nanoparticles in plant cells (Nicotiana tabacum), Environ. Sci.: Nano, 2019, 6, 2724–2735 RSC
.
- S. Argentiere, C. Cella, M. Cesaria, P. Milani and C. Lenardi, Silver nanoparticles in complex biological media: Assessment of colloidal stability and protein corona formation, J. Nanopart. Res., 2016, 18, 253 CrossRef
.
- T. A. Jorge de Souza, L. R. Rosa Souza and L. P. Franchi, Silver nanoparticles: An integrated view of green synthesis methods, transformation in the environment, and toxicity, Ecotoxicol. Environ. Saf., 2019, 171, 691–700 CrossRef CAS PubMed
.
- L. Pinďáková, V. Kašpárková, K. Kejlová, M. Dvořáková, D. Krsek, D. Jírová and L. Kašparová, Behaviour of silver nanoparticles in simulated saliva and gastrointestinal fluids, Int. J. Pharm., 2017, 527, 12–20 CrossRef PubMed
.
- Z. Zhao, G. Li, Q. S. Liu, W. Liu, G. Qu, L. Hu, Y. Long, Z. Cai, X. Zhao and G. Jiang, Identification and interaction mechanism of protein corona on silver nanoparticles with different sizes and the cellular responses, J. Hazard. Mater., 2021, 414, 125582 CrossRef CAS PubMed
.
- M. Levak, P. Burić, M. Dutour Sikirić, D. Domazet Jurašin, N. Mikac, N. Bačić, R. Drexel, F. Meier, Ž. Jakšić and D. M. Lyons, Effect of protein corona on silver nanoparticle stabilization and ion release kinetics in artificial seawater, Environ. Sci. Technol., 2017, 51, 1259–1266 CrossRef CAS PubMed
.
- W. Wu, R. Zhang, D. J. McClements, B. Chefetz, T. Polubesova and B. Xing, Transformation and speciation analysis of silver nanoparticles of dietary supplement in simulated human gastrointestinal tract, Environ. Sci. Technol., 2018, 52, 8792–8800 CrossRef CAS PubMed
.
- W. Liu, I. Worms and V. I. Slaveykova, Interaction of silver nanoparticles with antioxidant enzymes, Environ. Sci.: Nano, 2020, 7, 1507–1517 RSC
.
- A. Bhargava, A. Dev, S. J. Mohanbhai, V. Pareek, N. Jain, S. R. Choudhury, J. Panwar and S. Karmakar, Pre-coating of protein modulate patterns of corona formation, physiological stability and cytotoxicity of silver nanoparticles, Sci. Total Environ., 2021, 772, 144797 CrossRef CAS PubMed
.
- D. J. Boehmler, Z. J. O'Dell, C. Chung and K. R. Riley, Bovine serum albumin enhances silver nanoparticle dissolution kinetics in a size- and concentration-dependent manner, Langmuir, 2020, 36, 1053–1061 CrossRef CAS PubMed
.
- J. R. Borgatta, C. A. Lochbaum, W. H. Elmer, J. C. White, J. A. Pedersen and R. J. Hamers, Biomolecular corona formation on CuO nanoparticles in plant xylem fluid, Environ. Sci.: Nano, 2021, 8, 1067–1080 RSC
.
- J. Kurepa, T. E. Shull and J. A. Smalle, Metabolomic analyses of the bio-corona formed on TiO2 nanoparticles incubated with plant leaf tissues, J. Nanobiotechnol., 2020, 18, 28 CrossRef CAS PubMed
.
- J. Bing, X. Xiao, D. J. McClements, Y. Biao and C. Chongjiang, Protein corona formation around inorganic nanoparticles: Food plant proteins-TiO2 nanoparticle interactions, Food Hydrocolloids, 2021, 115, 106594 CrossRef CAS
.
- E. Voke, R. L. Pinals, N. S. Goh and M. P. Landry,
In planta nanosensors: Understanding biocorona formation for functional design, ACS Sens., 2021, 6, 2802–2814 CrossRef CAS PubMed
.
- M. R. Findlay, D. N. Freitas, M. Mobed-Miremadi and K. E. Wheeler, Machine learning provides predictive analysis into silver nanoparticle protein corona formation from physicochemical properties, Environ. Sci.: Nano, 2018, 5, 64–71 RSC
.
- J. D. Judy, J. M. Unrine and P. M. Bertsch, Evidence for biomagnification of gold nanoparticles within a terrestrial food chain, Environ. Sci. Technol., 2011, 45, 776–781 CrossRef CAS PubMed
.
- Y. Dai, Z. Wang, L. Zhang, Z. Jiang, S. Pu, Q. Fan, J. Zhao and B. Xing, Transfer and transformation of CeO2 NPs along a terrestrial trophic food chain, Environ. Sci.: Nano, 2020, 7, 588–598 RSC
.
- C. M. Rico, S. Majumdar, M. Duarte-Gardea, J. R. Peralta-Videa and J. L. Gardea-Torresdey, Interaction of nanoparticles with edible plants and their possible implications in the food chain, J. Agric. Food Chem., 2011, 59, 3485–3498 CrossRef CAS PubMed
.
- B. Xiao, Y. Zhang, X. Wang, M. Chen, B. Sun, T. Zhang and L. Zhu, Occurrence and trophic transfer of nanoparticulate Ag and Ti in the natural aquatic food web of Taihu Lake, China, Environ. Sci.: Nano, 2019, 6, 3431–3441 RSC
.
- J. I. Kwak and Y.-J. An, Trophic transfer of silver nanoparticles from earthworms disrupts the locomotion of springtails (Collembola), J. Hazard. Mater., 2016, 315, 110–116 CrossRef CAS PubMed
.
- D. Cleveland, S. E. Long, P. L. Pennington, E. Cooper, M. H. Fulton, G. I. Scott, T. Brewer, J. Davis, E. J. Petersen and L. Wood, Pilot estuarine mesocosm study on the environmental fate of silver nanomaterials leached from consumer products, Sci. Total Environ., 2012, 421–422, 267–272 CrossRef CAS PubMed
.
- N. Yan and W. Wang, Novel imaging of silver nanoparticle uptake by a unicellular alga and trophic transfer to Daphnia magna, Environ. Sci. Technol., 2021, 55, 5143–5151 CrossRef CAS PubMed
.
- Z. Wang, L. Xu, J. Zhao, X. Wang, J. C. White and B. Xing, CuO nanoparticle interaction with Arabidopsis thaliana: Toxicity, parent-progeny transfer, and gene expression, Environ. Sci. Technol., 2016, 50, 6008–6016 CrossRef CAS PubMed
.
- C. Peng, C. Xu, Q. Liu, L. Sun, Y. Luo and J. Shi, Fate and transformation of CuO nanoparticles in the soil–rice system during the life cycle of rice plants, Environ. Sci. Technol., 2017, 51, 4907–4917 CrossRef CAS PubMed
.
- P. Das, S. Barua, S. Sarkar, S. K. Chatterjee, S. Mukherjee, L. Goswami, S. Das, S. Bhattacharya, N. Karak and S. S. Bhattacharya, Mechanism of toxicity and transformation of silver nanoparticles: Inclusive assessment in earthworm-microbe-soil-plant system, Geoderma, 2018, 314, 73–84 CrossRef CAS
.
- T. Filippini, S. Tancredi, C. Malagoli, M. Malavolti, A. Bargellini, L. Vescovi, F. Nicolini and M. Vinceti, Dietary estimated intake of trace elements: Risk assessment in an Italian population, Exposure Health, 2020, 12, 641–655 CrossRef CAS
.
|
This journal is © The Royal Society of Chemistry 2022 |
Click here to see how this site uses Cookies. View our privacy policy here.