DOI:
10.1039/D1AN01817E
(Paper)
Analyst, 2022,
147, 40-47
Copper fumarate with high-bifunctional nanozyme activities at different pH values for glucose and epinephrine colorimetric detection in human serum†
Received
8th October 2021
, Accepted 16th November 2021
First published on 16th November 2021
Abstract
Metal–organic frameworks (MOFs) have attracted extensive attention for the development of colorimetric detection methods due to their ease of modification and high density of active sites. However, most of the reported colorimetric sensors based on MOFs show only a single nanozyme activity. Herein, the bifunctional enzyme activities of a hexagonal prism Cu MOF with fumaric acid as the ligand (Cu FMA), namely laccase-like activity under alkaline conditions (pH = 8) and peroxidase-like activity under acidic conditions (pH = 4), were verified. The specificity of Cu FMA at different pH values may be due to the presence of the Cu+ active center introduced by the weak reducibility of FMA. At pH = 8, Cu+ active centers are beneficial for dissociating the H–O bonds of phenolic compounds for the laccase system. In contrast, the dissociation of H–O is weakened at pH = 4, which prompts the breaking of the O–O bonds of H2O2 as a Fenton-like reaction for the peroxidase system. Based on the dual enzyme activities, Cu FMA sensors exhibit outstanding detectability for epinephrine and glucose with linear ranges of 2.7–54.6 μM and 0.01–0.8 mM and detection limits of 1.1 μM and 2.28 × 10−7 M, respectively. The Cu FMA colorimetric sensor can be applied for detecting and measuring glucose and epinephrine in human serum samples. This work paves the way for Cu MOFs to be used as the basis for rational regulation of the activity of dual nanozymes and for multifunctional applications under completely independent conditions.
Introduction
Natural enzymes, as highly selective and high-performance biocatalytic components, play a vital role in biological processes. They have been widely used in various applications such as biomedical analysis, the food industry and environmental protection.1–3 However, their high cost, inherent instability and difficulty of recycling have hindered their potential applications.4–6 Therefore, a lot of research has been devoted to the development of nanozymes as cheap and stable candidates.7,8 For example, previous works found that CuO, CuS, Cu3(PO4)2, Cu-based metal–organic frameworks (MOFs), etc. have peroxidase-like activity and can be applied as sensors for the detection of biomolecules.9,10 Liang reported that Cu/guanosine monophosphate amorphous MOFs exhibit outstanding laccase-like catalytic activity.11 Hence, Cu MOFs have been considered as one of the most promising materials with nanozyme activity due to their active Cu sites and their uniform nanoholes, exposing a large number of catalytic active sites. However, the nanozyme system based on Cu MOF nanozymes has not been fully utilized due to the mutual interference of multiple enzyme properties. To solve this problem, it is important to further develop a new Cu MOF with multifunctional enzyme activities and good anti-interference properties.
Epinephrine and glucose are both very important biomolecules in the human body, and can act as markers for pheochromocytoma and diabetes.12–14 Various methods for the quantitative determination of these two substances have been reported, such as fluorescence,13,15 electrochemistry,16–18 liquid chromatography13,19 and chemiluminescence.13,20,21 However, most of these methods are not conducive for rapid on-site detection, especially in remote areas, due to their high cost and cumbersome operation. Colorimetric measurement is a visual inspection technology that can be used in routine analysis without any special equipment.22 Unfortunately, colorimetric detection methods based on nanozymes are usually capable of detecting only a single substrate, which makes them difficult to apply for multichannel point-of-care testing (POCT). Therefore, the development of a dual-mode colorimetric method that enables specific detection of epinephrine and glucose could greatly simplify the detection process and have strong practical significance.
In this work, the hexagonal prism-shaped Cu FMA was synthesized by a one-step hydrothermal method with CuCl2 as the copper source and fumaric acid as an organic ligand. Then the enzyme activities of Cu FMA under different conditions (different substrates, pH, reaction temperature, reaction time, etc.) were explored. The experimental results demonstrate that Cu FMA exhibits dual enzyme activities under different pH conditions due to the presence of Cu2+ and Cu+ active centers on the Cu FMA surface. Based on these properties, a dual-mode colorimetric detection technology based on Cu FMA was proposed, which was applied to the colorimetric detection of glucose and epinephrine under different conditions. To verify the reliability of Cu FMA as a colorimetric sensor, the contents of glucose and epinephrine in human serum were also evaluated. For the different catalytic mechanisms of Cu FMA, measurement of the Michaelis–Menten constant was used to characterize the affinity between catalysts and substrates. Moreover, the surface electron properties of Cu FMA were tested by X-ray photoelectron spectroscopy to perform a thorough inquiry into the real active sites on the surface of materials.
Experimental
Regents and instruments
CuCl2·2H2O, fumaric acid (FMA), 3,3′,5,5′-tetramethylbenzidine (TMB), hydrogen peroxide (H2O2), glucose, glucose oxidase (GOx), CH3COOH (HAc), CH3COONa (NaAc), epinephrine, 2,4-dichlorophenol (2,4-DP), 4-aminoantipyrine (4-AAP), 2-[4-(2-hydroxyethyl)piperazin-1-yl]ethanesulfonic acid (HEPES) buffer solution, tert-butanol (t-butanol) and benzoquinone (BQ) were purchased from Aladdin Chemistry Co. Ltd (Shanghai, China). Deionized (DI) water, which was used in all processes, was obtained from a Milli-Q plus water purification system (Millipore Co. Ltd, USA; 18 MΩ cm). Human serum was taken from the school clinic at Fuzhou University.
Synthesis of copper fumarate
The synthetic process of copper fumarate (Cu FMA) was as follows: 0.5 g of CuCl2·2H2O and 0.5 g of C4H4O4 were dissolved in 30 ml of aqueous solution and stirred for 30 min to form the homogeneous solution. After the solvothermal reaction (60 °C for 24 h), the product was washed with water and centrifuged three times, and finally dried at 60 °C overnight.
Peroxidase-like activity and colorimetric detection of glucose based on Cu FMA
Cu FMA was ultrasonically dispersed in deionized water for 10 min to prepare the 5 mg mL−1 dispersion solution. To assess its peroxidase-like activity, 50 μL of Cu FMA solution was added to the catalytic substrate in the presence of 50 μL of TMB (5 mM), 50 μL of H2O2 (1.5 M) and 850 μL of NaAc–HAc buffer solution (pH = 4) at 30 °C and reacted for 45 min. In addition, for analysis and comparison, two samples without H2O2 or Cu FMA were set as a blank control group. Using the UV–vis technique, the absorbance values of the reaction system were measured at 652 nm under different conditions.
The colorimetric detection process of glucose based on Cu FMA was as follows: 50 μL of 2 mg mL−1 GOx and 50 μL of different concentrations of glucose were added to 100 μL of PBS (pH = 7.4) at 37 °C and reacted for 30 min. Then, 700 μL of 0.1 M NaAc–HAc buffer, 50 μL of Cu FMA (5 mg mL−1) and 50 μL of TMB (5 mM) were added to the previous reaction system at 30 °C and reacted for 45 min. Finally, the absorbance at 652 nm was measured.
Laccase-like activity and colorimetric detection of epinephrine based on Cu FMA
2,4-Dichlorophenol (2,4-DP) was used as a common laccase substrate to evaluate the catalytic activity of the enzyme and 4-aminoantipyrine (4-AAP) was used as a chromogenic dye for the evaluation of laccase activity.23 One hundred microliters of 5 mg mL−1 Cu FMA, 100 μL of 2,4-DP (5 mg mL−1) and 100 μL of 4-AAP (1 mg mL−1) were added to 700 μL HEPES buffer solution and reacted at 45 °C for 45 min. In addition, a blank control group was set up without 2,4-DP or Cu FMA. Using UV–vis measurement, the absorbance value of the reaction system at 510 nm was determined under different conditions.
The colorimetric detection process for epinephrine based on Cu FMA was as follows: 100 μL of different concentrations of epinephrine (0.5–10 μg) and 100 μL of 5 mg mL−1 Cu FMA dispersion solution were added to 800 μL of HEPES buffer (pH = 8), reacted at 45 °C for 45 min and then the absorbance at 485 nm collected using UV–vis spectroscopy.
Evaluation of catalytic mechanism using the steady-state kinetic constant
Cu FMA bi-enzyme steady-state kinetic constants, the Michaelis–Menten constant (Km) and the maximal catalytic velocity (Vmax)24,25 were used in the Michaelis–Menten equation (eqn (1) and (2)) to calculate the absorbance change rate of different substrates at 652 and 510 nm: | 1/V = (Km/Vmax) (1/[S]) + 1/Vmax. | (2) |
where V is initial catalytic velocity and [S] is the substrate concentration.1
The catalytic constant (kcat) directly reflects the enzyme's catalytic activity,23,26 and was calculated using:
where
kcat is the maximum number of substrate reactants converted to product per unit time and [
E] is the molar concentration of Cu FMA (1.98 × 10
−12 M), HRP or laccase.
Characterization
X-ray diffraction analysis (XRD) was performed using a Rigaku-minifex II (Rigaku Company, Japan) diffractometer operating with monochromatic Cu Kα1 (λ = 0.154056 nm) radiation at 30 kV and 15 mA. Data were collected over a 2θ range of 5–80° at a scanning speed of 0.02° s−1. The morphology and size were characterized by field-emission scanning electron microscopy (FESEM; Hitachi S4800, Hitachi Company, Japan). The surface properties of all samples were characterized using X-ray photoelectron spectroscopy (XPS) (ESCALAB 250, Thermo Fisher Scientific, China). All binding energies were calibrated with the C 1s peak at 284.8 eV corresponding to adventitious carbon found on the surface. The Fourier transform infrared (FTIR) spectrum was produced on a Nicolet iS50 IR spectrophotometer (Thermo Fisher, USA). The UV–vis absorption spectroscopy was conducted using a Genesys 10S spectrophotometer (Thermo Fisher Scientific, China).
Results and discussion
Structural and morphological characterization
Cu FMA was synthesized using the hydrothermal synthesis method with cupric chloride dihydrate as the copper source and fumaric acid as the organic ligand. To determine the crystal structure of Cu FMA, XRD patterns of Cu FMA and simulated Cu FMA were compared (Fig. 1a), and were in good agreement. Cu FMA exhibited a monoclinic crystal structure with a P21/c space group (a = 3.39690(6) Å, b = 10.34413(24) Å, c = 8.80754(16) Å, α = 90°, β = 95.5452(22)° and γ = 90°), indicating the successful synthesis of Cu FMA. This result was also confirmed by FT-IR spectroscopy (Fig. 1b), showing the C–H bond belonging to FMA to be located near 3085 cm−1. Furthermore, no significant characteristic peak of unprotonated –COOH was observed around 1658 cm−1 in the Cu FMA spectrum. The strong bands at 1568.8 and 1386.5 cm−1 in Cu FMA were assigned to the asymmetric and symmetric stretching modes of the coordinated (OCO) group, indicating that the carboxylic acid group is in non-monodentate coordination with Cu FMA.27 These results further confirm the structure of Cu FMA. SEM images (Fig. 1c and d) indicate that the Cu FMA has a hexagonal cross-section of 500 nm and a longitudinal size of 3–6 μm.
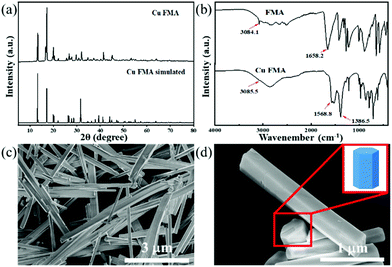 |
| Fig. 1 (a) XRD pattern of Cu FMA, (b) FT-IR spectra of FMA (up) and Cu FMA (down), and (c and d) SEM images of Cu FMA. | |
XPS technique was used to explore the surface chemical compositions and valence states of elements in Cu FMA. As shown in Fig. 2a, the spectrum Cu 2p splits into Cu 2p3/2 (934.1 eV) and Cu 2p1/2 (953.6 eV), corresponding to the formation of the coordination compound between Cu2+ and fumaric acid.28 The fitting results indicate the existence of an unsaturated Cu node on Cu FMA, which has been reported to be beneficial for dissociating the H–O bonds under alkaline conditions.29 Furthermore, the Cu LMM Auger spectrum (Fig. 2b) was deconvoluted into two peaks at 573.2 and 567.4 eV that were assigned to Cu(I) and Cu(II), respectively, which again demonstrates the presence of a Cu+ center on Cu FMA. The formation of Cu+ in the structure of Cu FMA was due to the weak reducing ability of FMA.30,31 We further compared the O 1s and C 1s spectra of Cu FMA. The O 1s spectra were composed of four peaks (Fig. 2c) and the binding energies at 533.1, 532.3, 531.5 and 530.7 eV were assigned to water peaks, C
O, carboxylic acid group (COO–) and M–O peak, respectively.32,33 The C 1s spectrum in Fig. 2d was deconvoluted into two peaks at 288.4 and 284.4 eV, corresponding to O
C–O and C
C from the coordination between Cu2+ and fumaric acid, which further proves the formation of Cu FMA.33
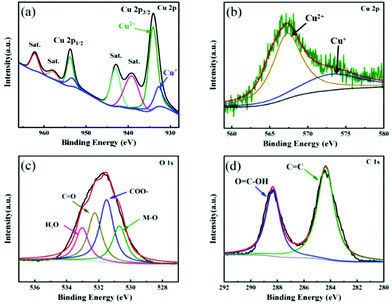 |
| Fig. 2 XPS spectra of (a) Cu 2p, (b) Cu LMM, (c) O 1s, and (d) C 1s for Cu FMA. | |
Analysis of Cu FMA with peroxidase-like and laccase-like activities
To investigate the peroxidase-like activity of Cu FMA, Cu FMA was mixed with peroxidase substrates TMB and H2O2 in a 0.1 M acetic acid buffer solution (pH = 4). In Fig. 3, the mixture presents an obvious color change (Fig. 3a1), with an absorption peak at 652 nm (Fig. 3b1), indicating that Cu FMA has inherent peroxidase-like activity. For comparison, three control experiments were carried out under the same conditions. In the absence of Cu FMA (Fig. 3a2 and b2), no obvious non-specific color change and no absorption peak at 652 nm could be identified. On the other hand, the results of the control experiment of Cu FMA (Fig. 3a3 and b3) also revealed no oxidation reaction in the absence of H2O2, indicating that the color change of TMB comes from the peroxidase-like activity of Cu FMA. At the same time, to ensure that the peroxidase-like activity of Cu FMA derived from the structure itself, rather than from free ions, experiments were performed using the supernatant solution of Cu FMA solution under different pH conditions. In Fig. 3a4 and b4, a slight colour change and UV absorption can be observed, indicating that the peroxidase-like activity of Cu FMA is mainly related to its nanostructure rather than the metallic ion leaching from Cu FMA. Similarly, the above methods were used to explore the laccase-like activity of Cu FMA. Cu FMA was mixed with the laccase substrate 2,4-DP, 4-AAP and the HEPES buffer solution (pH = 8). Similarly, this mixture showed a significant color change (Fig. 3c1) and a significant UV absorption at 510 nm (Fig. 3d1), demonstrating that Cu FMA has laccase-like activity under weakly alkaline conditions. The results from a series of control experiments (Fig. 3c and d) prove that the laccase-like activity arises from the structure of Cu FMA. Therefore, the above results demonstrate that Cu FMA shows dual enzyme activities, namely peroxidase-like activity at pH = 4 and laccase-like activity at pH = 8.
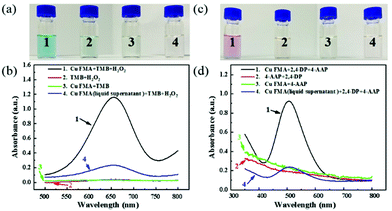 |
| Fig. 3 (a) Photographs of various reaction systems. (b) Typical UV–vis absorption of various reaction systems in H2O at (1) Cu FMA + TMB + H2O2, (2) TMB + H2O2, (3) Cu FMA + TMB and (4) Cu FMA liquid supernatant + TMB + H2O2. (c) Photographs of various reaction systems. (d) Typical UV–vis absorption of various reaction systems in H2O at (1) Cu FMA + 2,4-DP + 4-AAP, (2) 2,4-DP + 4-AAP, (3) Cu FMA + 4-AAP and (4) Cu FMA liquid supernatant + 2,4-DP + 4-AAP. | |
Similar to natural enzymes, the dual enzyme activities of Cu FMA are affected by various factors such as temperature, pH, concentration and reaction time. To determine the optimal peroxidase-like reaction conditions of Cu FMA, the reaction was carried out under different conditions of pH (2–5), temperature (25–40 °C) and H2O2 concentration (0.01–3 M). The results are illustrated in Fig. S1.† The relative activity of the reaction is maximum at pH = 4.0 (Fig. S1a†). With the increase in H2O2 concentration, the Cu FMA peroxidase-like activity reached the optimal point at 1.5 M, and then gradually decreased (Fig. S1b†). Fig. S1c† displays the effect of incubation time. In the 0–45 min incubation time, the light absorption intensity increased rapidly. Then experiments were conducted on the reaction activity at different temperatures, and 30 °C was found to be the optimal reaction temperature (Fig. S1d†). For Cu FMA laccase-like activity, the same method was used and the results are displayed in Fig. S2.† It obtained its maximum reactivity under the conditions of pH = 8, 45 °C, 45 min and 2,4-DP concentration of 5 mg mL−1.
To further study the steady-state kinetic characteristics of the dual-enzyme activity of Cu FMA, a series of enzyme-like kinetic data were measured in different substrate concentration ranges under the optimal reaction conditions for peroxidase-like and laccase-like activities (Fig. 4). The Km calculated by the Michaelis–Menten equation is a key parameter for evaluating the affinity between the enzyme and the substrate.1 When H2O2, TMB and 2,4-DP are the reaction substrates, the Km values are 0.34 mM (Fig. 4a),1.10 mM (Fig. 4b) and 0.45 mM (Fig. 4c), respectively. The above results indicate that Cu FMA has a strong binding affinity to H2O2 and 2,4-DP. Furthermore, the catalytic constant (kcat) (eqn (3)) is also a key factor for understanding the catalytic performance of the nanozyme.23,26 In Table S1,† the kcat value of Cu FMA with substrate TMB or H2O2 is higher than that with substrate HRP, indicating the better catalytic efficiency of Cu MOFs. Likewise, the kcat value of Cu FMA was much larger than that of laccase, which suggests that Cu FMA has a higher catalytic efficiency for oxidation of 2,4-DP than laccase.
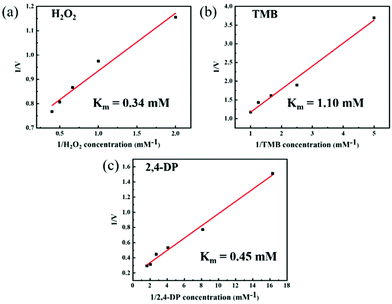 |
| Fig. 4 Lineweaver–Burk plot for Cu FMA in (a) H2O2, (b) TMB and (c) 2,4-DP. | |
Analysis of the catalytic mechanism of Cu FMA dual enzyme activity
To reveal its peroxidase-like activity mechanism, 1 mM p-benzoquinone (BQ) and 1 mM tert-butyl alcohol were added as free radical scavengers into the Cu FMA + H2O2 + TMB reaction system.34 After 45 min (Fig. 5a), the absorbance decreased with the addition of tert-butanol. After adding BQ, there was no signal change (Fig. 5a). This phenomenon indicates that ˙OH plays a major role in peroxidase activity, indicating that a Fenton-like reaction occurs on Cu FMA. Specifically, ˙OH is formed via a Fenton-like reaction on the Cu+ active sites introduced by the weak reducibility of FMA, which oxidizes the TMB into oxTMB (eqn (4)):4 | Cu+ + H2O2 → Cu2+ + OH− + ˙OH | (4) |
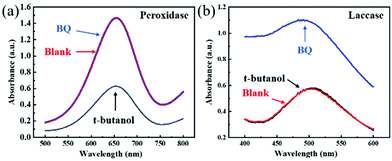 |
| Fig. 5 (a) Reaction activities of the Cu FMA + H2O2 + TMB system and (b) the Cu FMA + 2,4-DP + 4-AAP system with BQ and t-butanol. | |
For the mechanism of laccase-like activity, Fig. 5b shows no change in absorbance for the experimental group added with tert-butyl alcohol, while an increased signal in absorbance for BQ was observed due to the oxidation of Cu FMA. Such results indicate that no free radicals were involved in the reaction. Hence, as Solomon reports, to simplify the catalytic mechanism, analysis was performed using p-phenol as a substrate. As shown in Fig. 6, the Cu FMA laccase-like catalytic mechanism is proposed to be as follows:35 (i) p-phenol is adsorbed on Cu+ active sites; (ii) p-phenol is oxidized to generate p-benzoquinone and Cu+ is converted to form Cu0; (iii) Cu2+ is reduced to Cu+ while Cu0 is oxidized to Cu+ by electron transfer; and (iv) Cu+ is finally oxidized by oxygen in the air to Cu2+. Cheng et al. have demonstrated that coordinatively unsaturated Cu+ active centers could effectively accelerate the dissociation of the H–O bonds under alkaline conditions. Hence, combining the above analysis, under weakly alkaline conditions, Cu+ active centers created by the weak reducibility of FMA are beneficial for dissociating the H–O bonds of phenolic compounds. In contrast, the dissociation of H–O is weakened under weakly acidic conditions, which prompts the breaking of the O–O bonds of H2O2 with the formation of ˙OH.
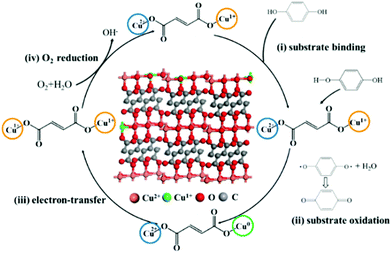 |
| Fig. 6 Catalytic mechanism of Cu FMA laccase-like activity. | |
Glucose detection based on Cu FMA peroxidase-like activity
H2O2, as an important oxidation product of glucose oxidase (GOx), is considered as a detection marker for the colorimetric detection of glucose. Utilizing the peroxidase-like properties of Cu FMA, a biosensor application for the colorimetric detection of glucose was constructed. In detail, as shown in Fig. 7a, GOx catalyzes the generation of H2O2 from glucose. Then Cu FMA's peroxidase-like activity further catalyzes the oxidation of TMB. As the glucose concentration increases, the color changes and the concentration of glucose can be clearly distinguished (Fig. 7b). The absorbance at 652 nm belonging to oxTMB increases in the UV–vis system (Fig. 7c). The calibration curve (Fig. 7c) shows that the detection range is 0.01–0.3 mM, the linear regression equation is A = 4.06C (mM) + 0.166 (R2 = 0.947) and the detection limit (LOD) is calculated as 2.28 × 10−7 M (S/N = 3) (LOD can be defined as LOD = 3σ/S, where σ is the response standard deviation and S is the slope of the calibration curve). For comparison with other glucose colorimetric methods, the Cu FMA colorimetric detection method has a better linear range and detection limit than other nanozymes (Table S2†).
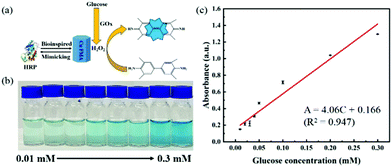 |
| Fig. 7 (a) Schematic mechanism of glucose detection colorimetry; (b) photo of catalytic activity of Cu FMA at different concentrations of glucose; and (c) absorbance at 652 nm versus concentration of glucose catalyzed by Cu FMA. | |
Epinephrine detection based on Cu FMA laccase-like activity
Epinephrine is a hormone produced by the adrenal medulla following external stimulation. It is used as a medicine to treat arrhythmia, anaphylactic shock and bronchial asthma. Therefore, the quantitative detection of epinephrine is essential for disease diagnosis and drug analysis. Cu FMA can catalyze the oxidation of epinephrine to produce pinkish-orange oxidation products. Different concentrations of epinephrine (2.7–54.6 μM) were mixed with Cu FMA and the absorbances of the oxidation products were collected at 485 nm by UV–vis. As shown in Fig. 8, the absorbance increased with increasing concentration of epinephrine (Fig. 8a), clearly distinguished by the color changes with different concentrations (Fig. 8b). The detection range is 2.7–54.6 μM, and the linear regression equation is A = 0.0032C (μM) + 0.0283 (R2 = 0.939). The LOD was calculated as 1.1 μM (S/N = 3). In addition, compared with other epinephrine colorimetric methods, the Cu FMA colorimetric detection method shows a satisfactory linear range and detection limit (Table S2†).
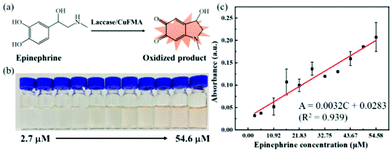 |
| Fig. 8 (a) Schematic mechanism of epinephrine detection colorimetry; (b) photo of the catalytic activity of Cu FMA at different concentrations of epinephrine; and (c) response for absorbance at 485 nm versus concentration of epinephrine catalyzed by Cu FMA. | |
Selective performance without interference for glucose and epinephrine colorimetric detection
Selectivity evaluation is an important step to determine the feasibility of using Cu FMA sensors. To examine the selectivity of the glucose detection method, some glucose analogues (1 mM fructose, galactose, sucrose and lactose) with twice the concentration of glucose were tested under the same optimal conditions. It can be seen from Fig. 9a that the response of these glucose analogues was negligible and less than 10% of the response of glucose under the same conditions. To further evaluate the anti-interference performance of the Cu FMA sensors, those glucose analogues with twice the concentration of glucose were mixed into a glucose solution. Fig. 9b shows that there was no significant change in the response of glucose analogues to the sensor, indicating that Cu FMA-based sensor has excellent anti-interference performance for glucose.
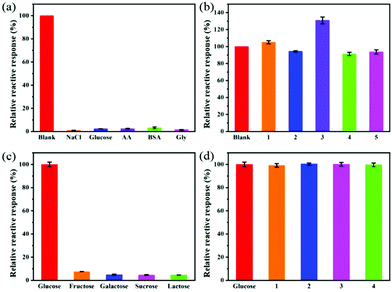 |
| Fig. 9 (a) Selectivity of glucose detection with glucose analogues, independently; (b) anti-interference performance of glucose detection with a mixture of glucose analogues and glucose; (c) selectivity of epinephrine detection with potential interferers, independently; and (d) anti-interference performance of epinephrine detection with a mixture of epinephrine potential interferers and epinephrine. | |
To explore the selectivity towards epinephrine, the responses of the Cu FMA sensor to 1 mM sodium chloride, glucose, ascorbic acid (AA), glycine (Gly), bovine serum albumin (BSA) and 0.5 mM epinephrine were tested under the same optimal conditions. Fig. 9c illustrates that the absorbance of these interfering substances produces a negligible response, less than 5% of that of epinephrine under the same conditions. In order to further evaluate the anti-interference performance of the sensor, twice the concentration of the interfering substance was added to the epinephrine solution; there was no significant change except for ascorbic acid (Fig. 9d). The substantial increase in ascorbic acid was due to the reduction of Cu2+ to Cu+, which accelerated electron transfer and improved the catalytic activity of Cu FMA. Overall, the Cu FMA sensor showed outstanding selectivity and anti-interference performance for glucose under weakly acid conditions and for epinephrine under weakly alkaline conditions.
To evaluate the reproducibility, Cu FMA was cyclically tested eight times, and each time Cu FMA was centrifuged, washed with DI water and reused. In Fig. S3,† it can be seen that, after eight cycles, Cu FMA maintained 83% of its relative activity while natural enzymes like HRP and laccase cannot be recycled.
To verify the reliability of Cu FMA as a colorimetric sensor, the contents of glucose and epinephrine in human serum were determined. Different concentrations of glucose and epinephrine were added to the serum sample and diluted 100 times without further processing. The experimental results are shown in Table 1. The recovery rates were between 92 and 110%, and the relative standard deviation (RSD) was less than 5%, which proves that the Cu FMA colorimetric sensor is suitable for the actual analysis of glucose and epinephrine. Furthermore, to verify the stability of Cu FMA as a colorimetric sensor, the contents of glucose and epinephrine in human serum were retested after 5 months. Experimental UV–vis spectra with spiked serum samples are illustrated in the Fig. S4† and Table S3,† showing almost no matrix effect. Note that the amount of the addition is basically consistent with the amount calculated, which proves the reliability and stability of Cu FMA.
Table 1 Determination of glucose and epinephrine added to diluted human blood serum with the proposed Cu FMA colorimetric method
|
Added (μM) |
Found (μM) |
Recovery (%) |
RSD (%, n = 3) |
Glucose |
20.00 |
20.38 |
101.90 |
3.60 |
30.00 |
27.77 |
92.57 |
2.80 |
40.00 |
38.16 |
95.40 |
4.30 |
50.00 |
48.30 |
96.60 |
1.60 |
60.00 |
57.97 |
96.62 |
3.20 |
Epinephrine |
5.00 |
4.67 |
93.40 |
4.20 |
10.00 |
11.04 |
110.40 |
2.90 |
15.00 |
15.78 |
105.20 |
4.30 |
20.00 |
18.67 |
93.35 |
3.70 |
25.00 |
24.30 |
97.20 |
2.30 |
Conclusions
In conclusion, using CuCl2 as the copper source and fumaric acid as the organic ligand, a one-step hydrothermal method was used to synthesize hexagonal prism-shaped Cu FMA nanomaterials without using any surfactant. The experimental results reveal that Cu FMA exhibits different catalytic activities under different pH conditions due to the presence of Cu+ active centers on the Cu FMA surface which are introduced by the weak reducibility of FMA. For peroxidase-like activity, Cu+ and H2O2 undergo a Fenton-like reaction to form ˙OH. The laccase-like activity of Cu FMA comes from the electron transfer process at Cu+/Cu2+. Based on the above strategy, a dual-mode colorimetric detection technology using Cu FMA was proposed, which was applied to the colorimetric detection of glucose and epinephrine under different conditions. The results prove that under weakly acidic conditions, the colorimetric method for detecting glucose displays a good linear relationship in the range of 0.01–0.3 mM with A = 4.06C (mM) + 0.166 (R2 = 0.947) with a detection limit of 2.28 × 10−7 M (S/N = 3). Under weakly alkaline conditions, the colorimetric method for detecting epinephrine in the range of 2.7–54.6 μM shows a good linear relationship with A = 0.0032C (μM) + 0.0283 (R2 = 0.939) and a detection limit of 1.1 μM (S/N = 3). Moreover, the colorimetric detection in different modes of Cu FMA exhibits good selectivity and anti-interference performance. Note that the Cu FMA colorimetric sensor is suitable for detecting and measuring glucose and epinephrine in human serum samples. This work paves the way for Cu MOF to be used as the basis for rationally regulating the activity of dual nanozymes and for multifunctional applications under completely independent conditions, which shows great prospects in the fields of catalysis and analysis.
Author contributions
M. H. Ying, G. Z. Yang, H. B. Pan and M. Du designed the experiments. M. H. Ying, G. Z. Yang, Y. J. Xu and H. L. Ye performed the materials synthesis, sample measurement and characterization. M. H. Ying, G. Z. Yang, X. Lin, Y. Lu and Y. Bai carried out the data analysis.
Conflicts of interest
The authors declare no competing financial interest.
Acknowledgements
This work was supported by the National Natural Science Foundation of China (no. 51872048), the Natural Science Foundation of Fujian Province (no. 2016J01222, 2018J01518) and the International Cooperation Foundation of Fujian Province (no. 2009I0016).
References
- Y. Chong, Q. Liu and C. Ge, Nano Today, 2021, 37, 101076 CrossRef CAS.
- Q. Liu, A. Zhang, R. Wang, Q. Zhang and D. Cui, Nano-Micro Lett., 2021, 13, 154 CrossRef CAS.
- Z. Wang, R. Zhang, X. Yan and K. Fan, Mater. Today, 2020, 41, 81–119 CrossRef CAS.
- T. Kang, Y. G. Kim, D. Kim and T. Hyeon, Coord. Chem. Rev., 2020, 403, 213092 CrossRef CAS.
- J. Wu, X. Wang, Q. Wang, Z. Lou, S. Li, Y. Zhu, L. Qin and H. Wei, Chem. Soc. Rev., 2019, 48, 1004–1076 RSC.
- W. Song, B. Zhao, C. Wang, Y. Ozaki and X. Lu, J. Mater. Chem. B, 2019, 7, 850–875 RSC.
- M. K. Masud, J. Na, M. Younus, M. S. A. Hossain, Y. Bando, M. J. A. Shiddiky and Y. Yamauchi, Chem. Soc. Rev., 2019, 48, 5717–5751 RSC.
- R. Bhattacharjee, S. Tanaka, S. Moriam, M. K. Masud, J. Lin, S. M. Alshehri, T. Ahamad, R. R. Salunkhe, N.-T. Nguyen, Y. Yamauchi, M. S. A. Hossain and M. J. A. Shiddiky, J. Mater. Chem. B, 2018, 6, 4783–4791 RSC.
- Y. Huang, M. Zhao, S. Han, Z. Lai, J. Yang, C. Tan, Q. Ma, Q. Lu, J. Chen, X. Zhang, Z. Zhang, B. Li, B. Chen, Y. Zong and H. Zhang, Adv. Mater., 2017, 29, 1700102 CrossRef.
- J. Zhu, W. Nie, Q. Wang, J. Li, H. Li, W. Wen, T. Bao, H. Xiong, X. Zhang and S. Wang, Carbon, 2018, 129, 29–37 CrossRef CAS.
- H. Liang, F. Lin, Z. Zhang, B. Liu, S. Jiang, Q. Yuan and J. Liu, ACS Appl. Mater. Interfaces, 2017, 9, 1352–1360 CrossRef CAS PubMed.
- J. Tashkhourian, S. F. Nami-Ana and M. Shamsipur, J. Mol. Liq., 2018, 266, 548–556 CrossRef CAS.
- Z. Chen, Y. Hu, Q. Yang, C. Wan, Y. Tan and H. Ma, Sens. Actuators, B, 2015, 207, 277–280 CrossRef CAS.
- N. H. Cho, J. E. Shaw, S. Karuranga, Y. Huang, J. D. da Rocha Fernandes, A. W. Ohlrogge and B. Malanda, Diabetes Res. Clin. Pract., 2018, 138, 271–281 CrossRef CAS PubMed.
- F. Hussain, D. J. S. Birch and J. C. Pickup, Anal. Biochem., 2005, 339, 137–143 CrossRef CAS PubMed.
- J. Wang, Chem. Rev., 2008, 108, 814–825 CrossRef CAS.
- E. Sehit and Z. Altintas, Biosens. Bioelectron., 2020, 159, 112165 CrossRef CAS PubMed.
- N. Nasirizadeh, Z. Shekari, H. R. Zare, S. M. Reza, A. R. Fakhari and H. Ahmar, Biosens. Bioelectron., 2013, 41, 608–614 CrossRef CAS.
- R. E. Foreman, A. L. George, F. Reimann, F. M. Gribble and R.
G. Kay, J. Proteome Res., 2021, 20, 3782–3797 CrossRef CAS PubMed.
- Y. Gao, Y. Huang, J. Chen, Y. Liu, Y. Xu and X. Ning, Anal. Chem., 2021, 93, 10593–10600 CrossRef CAS PubMed.
- N. Coppedè, G. Tarabella, M. Villani, D. Calestani, S. Iannotta and A. Zappettini, J. Mater. Chem. B, 2014, 2, 5620–5626 RSC.
- M. K. Masud, S. Yadav, M. N. Islam, N.-T. Nguyen, C. Salomon, R. Kline, H. R. Alamri, Z. A. Alothman, Y. Yamauchi, M. S. A. Hossain and M. J. A. Shiddiky, Anal. Chem., 2017, 89, 11005–11013 CrossRef CAS PubMed.
- J. Wang, R. Huang, W. Qi, R. Su, B. P. Binks and Z. He, Appl. Catal., B, 2019, 254, 452–462 CrossRef CAS.
- S. Tanaka, M. K. Masud, Y. V. Kaneti, M. J. A. Shiddiky, A. Fatehmulla, A. M. Aldhafiri, W. A. Farooq, Y. Bando, M. S. A. Hossain and Y. Yamauchi, ChemNanoMat, 2019, 5, 506–513 CrossRef CAS.
- M. K. Masud, J. Kim, M. M. Billah, K. Wood, M. J. A. Shiddiky, N.-T. Nguyen, R. K. Parsapur, Y. V. Kaneti, A. A. Alshehri, Y. G. Alghamidi, K. A. Alzahrani, M. Adharvanachari, P. Selvam, M. S. A. Hossain and Y. Yamauchi, J. Mater. Chem. B, 2019, 7, 5412–5422 RSC.
- Y.-Z. Li, T.-T. Li, W. Chen and Y.-Y. Song, ACS Appl. Mater. Interfaces, 2017, 9, 29881–29888 CrossRef CAS PubMed.
- C. Serre, F. Millange, S. Surble and G. Ferey, Angew. Chem., Int. Ed., 2004, 43, 6285–6289 CrossRef PubMed.
- A. A. Koutinas, A. Vlysidis, D. Pleissner, N. Kopsahelis, I. Lopez Garcia, I. K. Kookos, S. Papanikolaou, T. H. Kwan and C. S. K. Lin, Chem. Soc. Rev., 2014, 43, 2587–2627 RSC.
- W. Cheng, H. Zhang, D. Luan and X. Lou, Sci. Adv., 2021, 7, eabg2580 CrossRef CAS PubMed.
- R. M. Mitacek, Y. Ke, J. E. Prenni, R. Jadeja, D. L. VanOverbeke, G. G. Mafi and R. Ramanathan, J. Food Sci., 2019, 84, 38–50 CrossRef CAS PubMed.
- Y. R. Uhm, J. C. Lim and S. M. Choi, Int. J. Anal. Chem., 2017, 2017, 9321896 Search PubMed.
- Z. Liu, Z. Zhao, Y. Wang, S. Dou, D. Yan, D. Liu, Z. Xia and S. Wang, Adv. Mater., 2017, 29, 1606207 CrossRef PubMed.
- X. Liao, F. Wang, F. Wang, Y. Cai, Y. Yao, B.-T. Teng, Q. Hao and L. Shuxiang, Appl. Catal., B, 2019, 259, 118064 CrossRef CAS.
- Y. Li, J. Jiang, Y. Fang, Z. Cao, D. Chen, N. Li, Q. Xu and J. Lu, ACS Sustainable Chem. Eng., 2018, 6, 16186–16197 CrossRef CAS.
- S. M. Jones and E. I. Solomon, Cell. Mol. Life Sci., 2015, 72, 869–883 CrossRef CAS PubMed.
Footnote |
† Electronic supplementary information (ESI) available. See DOI: 10.1039/d1an01817e |
|
This journal is © The Royal Society of Chemistry 2022 |
Click here to see how this site uses Cookies. View our privacy policy here.