DOI:
10.1039/D1EE02095A
(Review Article)
Energy Environ. Sci., 2021,
14, 5161-5190
Lessons learned from spiro-OMeTAD and PTAA in perovskite solar cells
Received
7th July 2021
, Accepted 3rd August 2021
First published on 25th August 2021
Abstract
Organic semiconductors have become essential parts of thin-film electronic devices, particularly as hole transport layers (HTLs) in perovskite solar cells (PSCs) where they represent one of the major bottlenecks to further enhancements in both device stability and efficiency. Small molecule 2,2′,7,7′-tetrakis[N,N-di(4-methoxyphenyl)amino]-9,9′-spirobifluorene (spiro-OMeTAD) and polymer poly[bis(4-phenyl)(2,4,6-trimethylphenyl)amine] (PTAA) are two of the first successful HTLs used in PSCs, and have remained at the forefront of developing high efficiency devices for almost a decade. Since their first application, many investigations into the properties of spiro-OMeTAD and PTAA have contributed to a growing understanding of the mechanisms that enable their success as HTLs. This review summarizes and discusses the key electronic and morphological properties, doping strategies and mechanisms, and degradation pathways of both spiro-OMeTAD and PTAA. A critical comparison between the two materials is provided, highlighting both the similarities which explain their enduring popularity as well as key differences in electrical and morphological properties. From this analysis emerges an improved understanding of the fundamental properties that enable the persistent success of HTL materials, which are found to include not only hole conductivity, band gap, and morphology, but also interactions with dopants, the perovskite, and environmental stressors. The knowledge about these properties, which are critically summarized in this review, is also applicable to the many other types of organic electronic devices now employing spiro-OMeTAD and PTAA. A detailed examination of the properties of materials reveals a clear set of guiding principles for the development of future generation HTLs. Applying these design strategies to produce more advanced HTLs will be essential to further improve the stability, efficiency, and commercialization of PSCs.
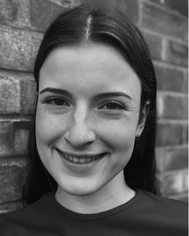
Florine M. Rombach
| Florine M. Rombach is a PhD candidate at the University of Oxford, working in the Photovoltaic and Optoelectronic Device group under Prof. Henry Snaith. She previously completed her MRes in Nanomaterials at Imperial College in London, and her BSc (majoring in Chemistry and Mathematics) at University College Utrecht in the Netherlands. She is interested in finding more efficient ways to create renewable energy, particularly through the investigation of the properties and stability of novel materials for photovoltaics. |
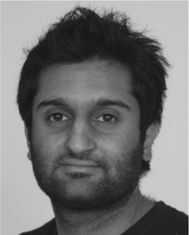
Saif A. Haque
| Saif A. Haque is a Professor of Chemistry at Imperial College London. He is a physical chemist with a particular interest in nanomaterials, molecular electronic materials, photochemistry and solar energy conversion. His research is currently addressing the functional characterization and development of solar cells (photovoltaics) based upon solution processable hybrid inorganic–organic semiconducting materials, inorganic metal chalcogenides and perovskites. Prior to becoming a professor, he was a Royal Society University Research Fellow at Imperial between 2005–2013. |
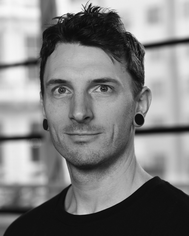
Thomas J. Macdonald
| Thomas J. Macdonald is an 1851 Research Fellow in the Department of Chemistry at Imperial College London. He was previously a Visiting Fellow at University College London, University of Queensland, and Flinders University. Before this, he was a Ramsay Memorial Fellow at University College London working on nanomaterials for solar energy conversion. He obtained his PhD at the University of South Australia in 2016 working in the research group of Prof. Thomas Nann. He has a strong interest in renewable energy and extensive experience in the synthesis of functional nanomaterials and the fabrication of third-generation photovoltaics. |
Broader context
Organic–inorganic lead halide perovskite solar cells (PSCs) are a class of third-generation photovoltaics that have attracted worldwide attention due to their facile solution processability, high efficiency, and low manufacturing costs. Hole transport layers (HTLs) in PSCs are critical to device functionality and represent a major bottleneck to further enhancing device stability and efficiency. Specifically, small molecule 2,2′,7,7′-tetrakis[N,N-di(4-methoxyphenyl)amino]-9,9′-spirobifluorene (spiro-OMeTAD) and polymer poly[bis(4-phenyl)(2,4,6-trimethylphenyl)amine] (PTAA) are two of the first successful HTLs used in PSCs and have remained as the most popular for developing high efficiency devices for over a decade. Investigations into the properties of spiro-OMeTAD and PTAA have contributed to a growing understanding of the mechanisms driving their success as HTLs and as a result, efficiencies of PSCs now routinely surpass 20%. While both HTLs typically use similar inorganic dopants to improve their functionality, several dissimilarities arising from differing physical and electronic properties affect their functionality and longevity in a PSC. Therefore, a detailed overview critically comparing the electronic properties, morphology, dopant and perovskite interactions, and stability of spiro-OMeTAD and PTAA is urgently needed. This will guide the future development of new and improved HTLs and is applicable for optoelectronic applications beyond PSCs.
|
1. Introduction
In current times of rapid population and economic growth, global energy demand is expected to double by 2050.1 At the same time, the escalating impacts of global warming on both humanity and the world's ecosystem call for an urgent reduction in emissions.2 The amount of sunlight that reaches the earth every hour contains more energy than humanity consumes within one year.3 This makes sunlight our most valuable renewable resource for energy production, which has prompted a worldwide research effort into photovoltaic (PV) energy conversion technologies. Over several decades, crystalline silicon (c-Si) solar cells have dominated the PV market, and although the price of producing electricity from c-Si declined by 76% between 2010–2018, the world is still producing over 70% of electricity by burning fossil fuels.3 Further improvements in the efficiency of solar modules, which will reduce balance of system costs, are necessary to make the switch to solar power economically attractive. Whilst c-Si PV remains the cheapest way to produce solar electricity, they are approaching their theoretical maximum efficiency with only very minor improvements in the past few years,4 and their fabrication is based on non-trivial, high-temperature processes.5
In the last decade, perovskite solar cells (PSCs) have emerged as a new type of solar cell, whose fabrication is low-cost, solution processed, compatible with flexible materials and has the potential to achieve higher efficiencies than c-Si solar cells due to its more ideal band gap.6 Additionally, the band gaps of perovskite materials are highly tuneable,7 making them ideal candidates for multi-junction solar cells which promise significantly higher efficiencies.8 The absorber layer of PSCs typically consists of an ABX3 crystal structure where A is an organic/inorganic cation such as methylammonium (MA), formamidinium (FA), or cesium (Cs), B is a divalent cation such as lead (Pb) and/or tin (Sn), and X is typically one or more halide(s). The first PSC was constructed in 2009 by Kojima et al.,9 who replaced the dye in a typical dye-sensitized solar cell (DSSC) architecture10 with the perovskite absorber methylammonium lead iodide. Moving from a liquid electrolyte to a solid-state hole conductor later raised efficiencies from 3.8% to 9.7%, simultaneously resulting in greatly improved solar cell stability.5,11 The demonstration that perovskite had a high absorption coefficient,12,13 could independently conduct both electrons14 and holes,15 showed exceptionally long electron and hole diffusion lengths16,17 as well as spontaneous exciton dissociation,18 set the pace for the next decade of PSC research. This enabled the further exploration of both mesoporous19 and planar20,21 device architectures in both conventional n–i–p (Fig. 1a) and inverted p–i–n forms, as well as novel processing techniques enabling higher quality perovskite films.22–24 In all architectures, charge carriers are selectively directed to their respective electrodes by charge transport layers (CTLs), where electrons move through an electron transport layer (ETL) and holes through a hole transport layer (HTL).
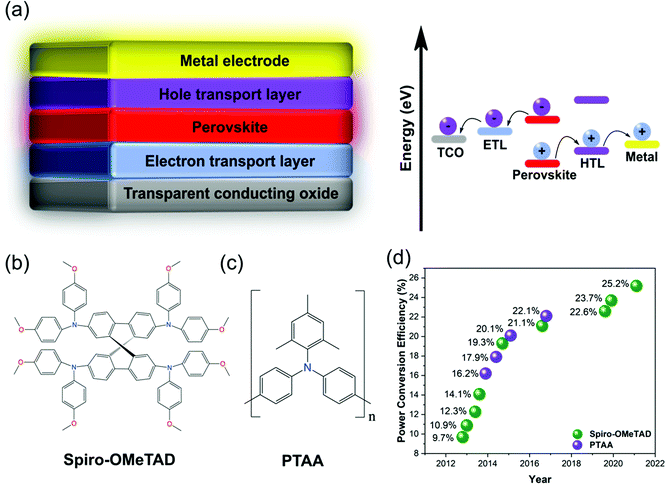 |
| Fig. 1 Introduction to spiro-OMeTAD and PTAA. (a) Architecture of a planar n–i–p PSC and its energy alignment. Chemical structures of (b) spiro-OMeTAD and (c) PTAA. (d) HTLs used in high-efficiency solar cells over time. Reprinted with permission.59 Copyright 2017, Wiley-VCH. | |
Over the last decade, significant progress has been made in understanding and improving both the efficiency25–31 and stability32–37 of PSCs by tuning the properties of the perovskite absorber and/or CTLs. Over time, PSCs have become increasingly limited in both efficiency and stability by their CTLs.38 A renewed focus on understanding and optimizing their functionality is hence essential to pushing the efficiency of PSCs from the current record of 25.6%39 to the ∼31% thermodynamic efficiency limit,6 whilst also developing sufficient stability for commercialization.
The highest efficiencies in n–i–p PSCs are most often achieved using p-doped spiro-OMeTAD40,41 (2,2′,7,7′-tetrakis(N,N-p-dimethoxyphenylamino)-9,9′-spirobifluorene, Fig. 1b), which possesses a long-standing reputation as an effective solid-state HTL.42 However, the more recently introduced43 p-doped PTAA (poly[bis(4-phenyl)(2,4,6-trimethylphenyl)amine], Fig. 1c) has also become popular and been used to achieve record efficiencies.44 Despite extensive attempts to develop more efficient and/or stable alternative HTL materials,45–47 spiro-OMeTAD and PTAA remain the most popular HTLs for high-efficiency n–i–p PSCs (Fig. 1d).48 This review provides an in-depth analysis and critical comparison of the development, key electronic and morphological properties, conventional and alternative doping schemes and mechanisms, and degradation pathways of both spiro-OMeTAD and PTAA. This knowledge is highly applicable to device fabrication and explains the root of various HTL-related device failures.
Whilst this review focuses on applications of spiro-OMeTAD and PTAA as HTLs in PSCs, most of the properties discussed remain equally relevant for other electronic devices in which spiro-OMeTAD and PTAA are used as organic semiconductors. For example, they are also popular as HTLs in perovskite light emitting diodes (LED),49,50 organic LEDs,51 and dye sensitized solar cells (DSSC),52 in which familiarity with the energetics, conductivity, doping, and stability of these materials remains equally important. Additionally, PTAA remains very widely used in organic field effect transistors (OFET),53 and both materials have also been applied in photodetectors.54,55 Researchers from any of these fields will find useful information about the intrinsic properties, doping schemes, behaviors under environmental stressing, and degradation pathways of spiro-OMeTAD and PTAA in this review. These are relevant to any electronic devices employing spiro-OMeTAD and PTAA as organic semiconductors.
When looking specifically at the performance of spiro-OMeTAD and PTAA in PSCs, this review focuses on HTLs in n–i–p architectures as these still retain the highest efficiencies. Additionally, recent investigations have suggested that the properties of charge transfer at the perovskite/HTL interface are the major efficiency and stability-limiting factors in high-efficiency n–i–p PSCs.56–58 Hence, this review focuses solely on the properties of HTLs in n–i–p architectures and does not cover the wetting or crystallographic growth of perovskite materials on top of them.
This analysis reveals a range of fundamental properties that enable the persistent success of a HTL, which are found to include not only high hole mobility and good morphology, but also interactions with dopants, the perovskite, and environmental stressors. A detailed examination of both doping and degradation mechanisms reveals general rules for selecting optimal dopants and ensuring good stability, which apply even to materials beyond spiro-OMeTAD and PTAA (especially other organic HTLs). As the efficiencies of PSCs approach fundamental limits, optimization of hole transport layers will only become more important. Understanding the doping strategies, morphological and molecular adjustments that can be used to optimize device performance is essential for the development of the next generation of HTLs.
2. Hole transport layers in perovskite solar cells
The way charges are transferred to and through the CTLs in PSCs affects every important solar cell parameter and can significantly limit power conversion efficiency (PCE). The choice and processing of the CTLs is central to PSC performance, as they strongly effect the energetic alignment,57,58,60 charge transit time,61 recombination kinetics,38,58,62 and charge extraction efficiency63 in the device. Their morphological, mechanical and chemical properties can strongly affect device stability, where their abundance and cost determine the commercialization of a PSC architecture.
In high-efficiency n–i–p PSCs, open-circuit voltage (Voc) losses have been found to correspond very well to interfacial recombination currents at the perovskite/HTL interface, which depend strongly on the HTL used (Fig. 2a).57 Gelmetti et al. demonstrated that recombination between holes in the HTL and electrons in the perovskite is the main recombination loss mechanism in these PSCs.58 The magnitude of this interfacial recombination can be influenced by the electronic coupling between the HTL and the perovskite,64 the number of trap states in the HTL as determined by its energetic disorder,38 and the HTL conductivity, which when it is much lower than that of the perovskite can cause accumulation of high densities of holes at the interface.65 Glowienka et al. suggested that the chemical interaction between the HTL and the perovskite creates a chemically altered ‘dead zone’ in the perovskite in which Shockley–Read–Hall recombination rates are very high.66 However, some HTLs have also been shown to actively passivate defects at the perovskite interface.67
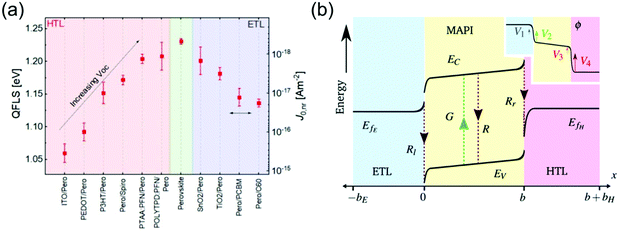 |
| Fig. 2 Impact of HTL energetics on PSCs. (a) Trend of quasi Fermi level splitting (QFLS) in a variety of HTLs and ETLs, illustrating impact of CTLs on PSC Voc. Reprinted with permission.57 Copyright 2019, Royal Society of Chemistry. (b) Energy diagram of a PSC, illustrating band bending at CTL interfaces as well as impact of energetic offsets. Inset shows potential distribution throughout ETL/MAPI/HTL, with severe potential changes at the 4 Debye layers as described by the surface polarization model. Reprinted with permission.60 Copyright 2019, Royal Society of Chemistry. | |
Additionally, a large offset between the valence band (VB) of the perovskite and the highest occupied molecular orbital (HOMO) of the HTL decreases the Voc in PSCs due to hole transport losses.38 Although the ideal offset remains a subject of discussion,62 Westbrook et al. have suggested that only a small energy offset of 0.07 eV between the HTL HOMO and the perovskite VB is needed to drive charge separation at the interface.63 The quasi-Fermi level of the perovskite must also be aligned with the Fermi level of the HTL, as an offset exponentially increases the hole density within the HTL, increasing the interfacial recombination rate.38,57 The energetics of HTLs in n–i–p PSCs have also been shown to determine the distribution of internal electric potential in the cell, thought to be key to device performance as it can drive charges either towards or away from interfaces (Fig. 2b).60
Fast charge transit through the HTL is also important to PSC efficiency, and the conductivity of hybrid perovskites is orders of magnitude larger than that of most commonly used HTLs.61,68 Hence, decreasing the transit time of charges through the HTL, either by making it thinner or increasing its conductivity, significantly improves both the fill factor (FF) and Voc of the device by improving charge extraction65,69 and reducing the likelihood of recombination events in the HTL.69 In addition to this, parasitic absorption of light by HTLs can also reduce device efficiency by negatively impacting the short-circuit current density (Jsc).70
Hence, the energetics, hole conductivity and morphology of the HTL all greatly influence overall device efficiency.71 The HTL also plays a crucial role in the stability and lifetime of PSCs as it sits directly on top of the active perovskite layer, and can either slow down or accelerate degradation mechanisms.72 Finally, the HTL should also be compatible with low-cost and solution processable manufacturing techniques. HTLs ideally require (a) high charge carrier mobilities and conductivities, (b) appropriate HOMO and quasi Fermi levels for efficient hole transfer from perovskite to HTL, (c) high LUMO for good electron blocking, (d) thermal, moisture, UV and chemical stability, (e) pinhole-free morphology and good morphological contact with the perovskite. Additionally, the HTLs should be made with (f) low-cost and abundant materials, (g) cheap, solution-based fabrication methods using solvents that do not disturb the perovskite layer, and (h) an easily reproducible synthesis. As a range of dopants or additives is normally used to improve both the hole conductivity in HTLs and their electronic alignment with the perovskite, these should also align with each of these properties.
A wide range48 of potential HTLs from organic small molecules45–47 to polymers73,74 to inorganic compounds75,76 has been explored over the past decade, with the inclusion of various additives. However, the most popular hole transport materials for high efficiency PSCs remain spiro-OMeTAD and PTAA as demonstrated in Fig. 1a. They are both based on the triarylamine moiety, which has been used in organic semiconductors since the late 1990's and benefits from an exceptionally stable radical cation77 and facile tuning of optical, electrical, and morphological properties.64 The review examines how and to what extent spiro-OMeTAD and PTAA fulfill the requirements for ideal HTLs, and explains how this translates to their good functionality as HTL materials. However, it also reveals their limitations and makes recommendations for research practices around the design of future HTLs.
3. Spiro-OMeTAD
3.1 Pristine spiro-OMeTAD properties
Spiro-OMeTAD was initially synthesized as a high conductivity, stably amorphous, small molecule HTL in the 1990's42,78 and first applied in PSCs in 2012 demonstrating an impressive efficiency of 9.7% and dramatically improved stability compared to liquid junction PSCs.11 The use of spiro-OMeTAD proved to be critical to the evolution of solid-state heterojunction PSCs from DSSCs, and spiro-OMeTAD was the HTL of choice for many early PSC efficiency records.22,25,32,79 The enduring popularity of spiro-OMeTAD as a HTL in PSCs can be largely ascribed to its ease of processing, somewhat favorable HOMO-VB match with the most popular perovskite light absorbers, and good hole conductivity when doped. The use of an appropriate solvent, specifically chloroform,80 enables a smooth, homogenous and pinhole-free layer of pristine spiro-OMeTAD to be deposited from solution, which is a morphology strongly correlated to overall device efficiency.81
Schulz et al. investigated the band alignment of spiro-OMeTAD at the perovskite interface using direct spectroscopic methods, determining a HOMO of −5.0 eV ± 0.1 eV and a LUMO of −1.5 eV.82 The LUMO of spiro-OMeTAD is positioned more than 2 eV above the conduction band (CB) of a range of different perovskites, which indicates good electron blocking abilities. However, the offset between the spiro-OMeTAD HOMO and the perovskite VB, which lies between −5.2 eV and −5.9 eV for a wide range of photoactive perovskites,82,83 lies on the order of 100s of meV, which is large enough to cause significant voltage losses.82
Although hole mobility generally increases with crystallinity for organic semiconductors,84 the amorphous spiro-OMeTAD phase is important for maintaining good contact at the HTL/perovskite and HTL/electrode interfaces.85 Poplavvsky and Nelson86 determined the hole mobility in pristine spiro-OMeTAD to be 2 × 10−4 cm2 V−1 s−1, but it is worth noting that this mobility is sometimes measured to be lower in other papers, down to a magnitude of 10−5 cm2 V−1 s−1.87,88 This variation is likely due to differences in solvent or spiro-OMeTAD purity89 and film deposition techniques,86 resulting in varying degrees of energetic disorder in the film,84 but could also be caused by the use of different mobility measurement techniques and models.90 Conductivity is a product of the hole mobility and hole density in the HTL. The intrinsic hole density in pristine spiro-OMeTAD has been cited as 8.67 × 1014 cm−3
89 and a range of values for its conductivity can be found in literature, ranging from 2.5 × 10−7 S cm−1
88 (derived from I–V characteristics) to ∼10−8 S cm−1 (derived from 4-point probe measurements).91 These variations in conductivity measurements can be due to variations in the concentration of oxidized spiro-OMeTAD+ in the pristine film caused by differing amounts of exposure to oxygen or light.92
The hole conductivity of spiro-OMeTAD is smaller than that of perovskites by multiple orders of magnitude, which means that it limits charge transit times68 (Fig. 3a) and particularly the FF in PSCs.61,68 Multiple groups have found that using a thinner layer of spiro-OMeTAD (down to 180 nm) significantly improves the FF of PSCs (Fig. 3b), as holes have to traverse a shorter distance which means that the limited conductivity of spiro-OMeTAD impacts device performance less.93,94 However, devices with spiro-OMeTAD layers this thin were found to be less reproducible due to a reduced uniformity of coverage on the relatively rough perovskite layer, which enabled occasional direct contact between perovskite and Au. These contacts increase recombination and are detrimental to Voc (Fig. 3b). Spiro-OMeTAD hence has an optimal thickness around 20093,94–370 nm68 depending on perovskite roughness. Additionally, a smooth HTL/Au interface was found to improve the current generation in PSCs by enhanced light reflection.94
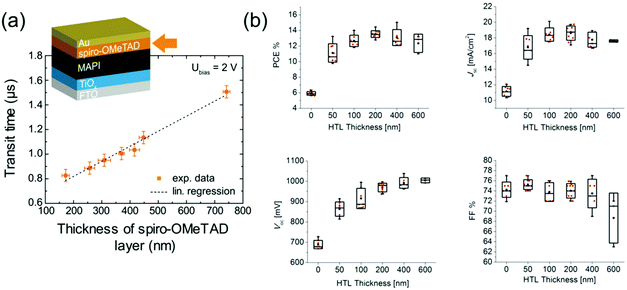 |
| Fig. 3 Impact of spiro-OMeTAD thickness on PSC performance. (a) Transit time of charges through a PSC with different spiro-OMeTAD HTL thicknesses. Reprinted with permission.68 Copyright 2017, American Chemical Society. (b) PSC parameters with different spiro-OMeTAD HTL thicknesses. Reprinted with permission.94 Copyright 2015, American Chemical Society. | |
In addition to this, spiro-OMeTAD has been found to show very fast hole transfer on the sub-ps scale from perovskites (undoped)95 and particularly low recombination rates (in its doped form) in DSSCs as compared to other HTLs.96 This could be due to the relatively weak electronic coupling between spiro-OMeTAD and the perovskite because of its bulky molecular structure.97
3.2 Spiro-OMeTAD doping
Spiro-OMeTAD is conventionally doped with multiple additives which enhance both its electronic and physical properties. Doping is a well-established strategy to improve the conductivity of semiconductors, where an impurity is added to the semiconductor in order to increase the concentration of majority charge carriers and improve conductivity.98 Spiro-OMeTAD is p-doped by oxidation to spiro-OMeTAD+.99 This also deepens its HOMO by 420 meV, potentially providing better energetic alignment at the spiro-OMeTAD/perovskite interface.100 Although the oxidation of spiro-OMeTAD has been detected even without the addition of any p-dopants, this was at a rate too low to significantly affect conductivity.101 The most commonly used additives are p-dopants lithium bistrifluoromethanesulfonimidate (LiTFSI) and FK209 (tris[2-(1H-pyrazol-1-yl)-4-tert-butylpyridine)cobalt(III) tris(bis(trifluoromethylsulfonyl)imide)], as well as 4-tertbutylpyridine (tBP) which mainly improves morphology (depicted in Fig. 4a).102
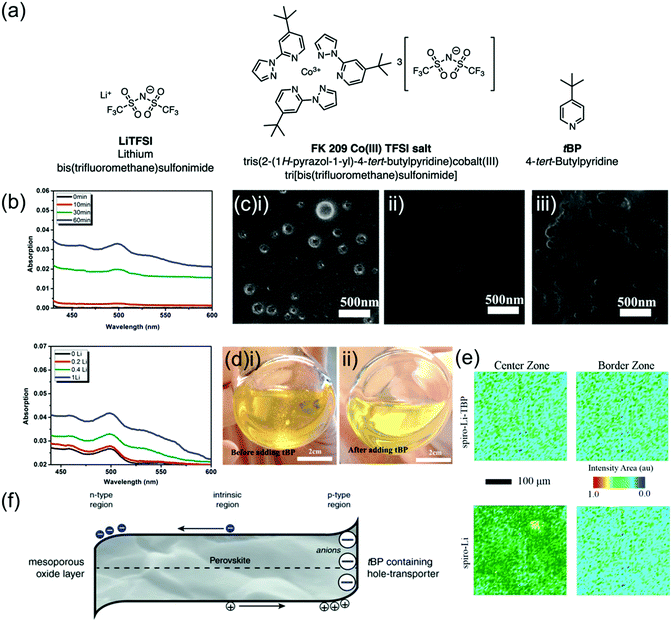 |
| Fig. 4 Effects of LiTFSI and tBP on spiro-OMeTAD. (a) Chemical structures of conventional spiro-OMeTAD dopants. Reprinted with permission.102 Copyright 2019, Royal Society of Chemistry. (b) UV-vis absorption spectra in the 450–600 nm range of LiTFSI-doped spiro-OMeTAD in chlorobenzene with either 0.4 LiTFSI/spiro-OMeTAD molar ratio at various illumination times or 1 h illumination time with various molar ratios. Growing absorption peak corresponds to the oxidized spiro-OMeTAD+ species. Reprinted with permission.101 Copyright 2015, American Chemical Society. (c) Scanning electron microscopy (SEM) images of freshly prepared spiro-OMeTAD thin film (i) without tBP, showing pinholes, (ii) with tBP, showing no pinholes, (iii) with tBP after overnight vacuum treatment, showing re-formed voids and pinholes. Reprinted with permission.117 Copyright 2016, American Chemical Society. (d) Photographs of spiro-OMeTAD solution doped with LiTFSI (i) without and (ii) with tBP. Reprinted with permission.117 Copyright 2016, American Chemical Society. (e) Fourier transform infrared spectroscopy microscopy images mapping the LiTFSI signal intensity in spiro-LiTFSI and spiro-LiTFSI-tBP. Reprinted with permission.81 Copyright 2016, American Chemical Society. (f) Diagram of perovskite region in a PSC with proposed n-doped region next to ETL and p-doped region next to tBP-containing HTL. Reprinted with permission.115 Copyright 2017 Wiley. | |
The simultaneous use of LiTFSI and tBP in spiro-OMeTAD has been found to improve its conductivity by two orders of magnitude from 2.5 × 10−7 S cm−1 to 2 × 10−5 S cm−1,103 and the addition of FK209 further improved conductivity causing a decrease in series resistance from 94.7 Ω to 65.8 Ω.79 Since these additives are generally present in large concentrations (Table 1), dopants can have significant effects not only on conductivity but also on energetics, recombination, thin film morphology and stability within a PSC.
Table 1 Spiro-OMeTAD doping ratios in recent high-efficiency PSCs
Ref. |
Spiro-OMeTAD : tBP : LiTFSI : FK209 (molar ratio) |
26
|
1 : 3.3 : 0.53 : 0.098 |
41
|
1 : 3.5 : 0.53 : 0 |
40
|
1 : 1.8 : 0.28 : 0.017 |
104
|
1 : 3.7 : 0.57 : 0.034 |
105
|
1 : 3.30 : 0.50 : 0.03 |
LiTFSI.
LiTFSI was introduced as a dopant in solid state dye sensitized solar cells by Snaith et al. in 2006.103 It p-dopes spiro-OMeTAD by increasing the concentration of oxidized spiro-OMeTAD+, moving the Fermi level of the HTL closer to its HOMO.106 In PSCs, the oxidation of spiro-OMeTAD by LiTFSI is suggested to proceed by two separate spectrum-dependent oxidation mechanisms, where spiro-OMeTAD is either oxidized directly by oxygen or by an intermediate oxidation of the perovskite depending on the wavelength of light that drives the reaction (Fig. 4b).101 The product spiro-OMeTAD+TFSI− is weakly bound due to the highly delocalized charge on TFSI−, which means that the barrier to charge hopping is reduced, improving conductivity.107 Hawash et al. also show that the concentration of spiro-OMeTAD+ in a LiTFSI-doped film increased over time upon exposure to air.108 Additionally, exposure to high humidity has been suggested to irreversibly distribute LiTFSI more evenly throughout the film resulting in a significant conductivity improvement.109 24 hours of aging LiTFSI-doped spiro-OMeTAD in ambient conditions increased both mobility and conductivity by more than 2 orders of magnitude, which was ascribed to irreversible LiTFSI redistribution by water vapour and reversible oxygen doping by extended oxygen exposure.109 However, the mechanism by which these factors specifically increase mobility rather than hole concentration remains unclear. Although low concentrations of spiro-OMeTAD+ may be created in PSCs by exposure to only light, oxygen or LiTFSI, all three factors are necessary to create significant concentrations of persistent spiro-OMeTAD+. Increases in device efficiency after light-soaking treatments, in which devices are exposed to intense illumination for 15–30 min before testing, can also be attributed to an increase in spiro-OMeTAD+ in the HTL.92,101 To obtain high efficiency devices, it is customary to age doped spiro-OMeTAD in a low humidity ambient environment overnight or even up to multiple weeks.105 Cho et al. found that this aging improved Voc and FF in devices both by increasing conductivity and lowering the HOMO of spiro-OMeTAD, and by reducing recombination in the perovskite.110 However, this was only observed in the presence of both oxygen and moisture. The necessity for light irradiation101 and the effects of tBP de-doping111 may suggest that it is unlikely that the concentration of spiro-OMeTAD+ is significantly increased in the dark. Hence, this phenomenon deserves further investigation.
tBP.
High boiling point (197 °C)81 liquid tBP is mainly said to improve the morphology and uniformity of LiTFSI-doped spiro-OMeTAD films in PSCs.81,111,112 It increases the polarity of the spiro-OMeTAD precursor which improves wetting on the perovskite.113 Spiro-OMeTAD films doped with LiTFSI tend to form pinholes immediately after deposition (Fig. 4ci), which are detrimental to device stability as they allow the diffusion of degrading ions, water and oxygen into the perovskite.108tBP is thought to generate a semi-liquid environment in the deposited film which permits solid-state diffusion,111 enabling it to fill pinholes which re-form when tBP is removed by evaporation as shown in Fig. 4c.112 Wang et al. furthermore suggest that the addition of tBP to a liquid spiro-OMeTAD precursor in chlorobenzene removes the phase separation induced by the addition of LiTFSI, which is dissolved in acetonitrile, as shown in Fig. 4d.114 This improved mixing translates to the deposited film, where the addition of tBP greatly improves the distribution of LiTFSI throughout the film as demonstrated in Fig. 4e.81 This improvement was shown to be due to the formation of LiTFSI-tBP complexes.114 In addition to this, there are indications that tBP improves the hole collection efficiency at the perovskite-HTL interface by p-doping the region of perovskite adjacent to the HTL, resulting in upward band bending as shown in Fig. 4f.115 Noel et al. also showed that direct treatment with pyridine was able to passivate perovskite surfaces to reduce recombination,116 which may indicate that tBP has a passivating effect.
Co(III) complexes.
Co(III) complexes are a more recently popularized but less well-understood class of p-dopants used in spiro-OMeTAD. Burschka et al.118 and Noh et al.79 simultaneously introduced FK209, which when used alongside LiTFSI and tBP, increased conductivity by ∼7× as compared to a LiTFSI- and tBP-doped spiro-OMeTAD film.79 Interestingly, FK209 was shown to oxidize spiro-OMeTAD significantly even under dark conditions, which LiTFSI does not.
Alternative dopants.
The remaining room for performance improvement, as well as the degrading properties of commonly used spiro-OMeTAD dopants, have prompted a search for alternative dopants and additives.102 These have included a range of metal complexes (often containing the TSFI− ion),119–122 pyridine derivatives,119,123 and different Cu(II) complexes.120 Particularly promising are dopants which are able to oxidize spiro-OMeTAD without any exposure to oxygen such as spiro-OMeTAD(TFSI)2,91 Zn(TFSI)2,124 and vanadic oxide (V2O5).125 Oxygen has been shown to participate in perovskite degradation, and the ability to encapsulate devices that were never exposed to air directly in nitrogen makes encapsulation much more effective, especially for particularly air-sensitive tin-containing perovskites.126
Despite the variety of alternative dopants and additives explored, LiTFSI, tBP and FK209 remain a favourite combination amongst high efficiency PSCs.40,41,104 Since it has been shown that additives are rarely straightforward modifiers, but rather interact strongly with multiple components of the spiro-OMeTAD HTL and the PSC, a better understanding of the doping mechanisms outlined here can aid the design of intelligent doping schemes and more stable PSCs.
3.3 Stability limitations of spiro-OMeTAD
Thermal degradation.
Whilst the electronic properties of doped spiro-OMeTAD enable high-efficiency devices in a research environment, its stability represents a major barrier. Although spiro-OMeTAD has a 245 °C melting point,85 even pristine spiro-OMeTAD was shown to be vulnerable to crystallization upon thermal stressing at 100 °C, which disturbed contact at its interfaces.85 Thermal stability is worsened by the addition of tBP, which was found to decrease the stability of the amorphous spiro-OMeTAD phase,85,111 as well as by p-dopants, since oxidized spiro-OMeTAD+ has a significantly lower glass transition temperature.127 The performance of PSCs using doped spiro-OMeTAD HTLs has been shown to degrade rapidly at temperatures as low as 85 °C, whilst most perovskites and other PSC components have been shown to be thermally stable in this range.128 Mesquita et al. observed that only part of this degradation was irreversible as shown in Fig. 5a.129 As pristine spiro-OMeTAD was much less affected by thermal stressing, the permanent degradation was ascribed to dopant evaporation. tBP has directly been shown to evaporate from a doped spiro-OMeTAD film at 85 °C,130 causing device performance to rapidly degrade80,130 through the formation of pinholes,112 although this could be partially prevented by the presence of a metal electrode.131 Additionally, the spontaneous degradation of spiro-OMeTAD+ as well as the de-doping of spiro-OMeTAD+ by tBP were shown to occur at much higher rates at high temperatures (100 °C).127
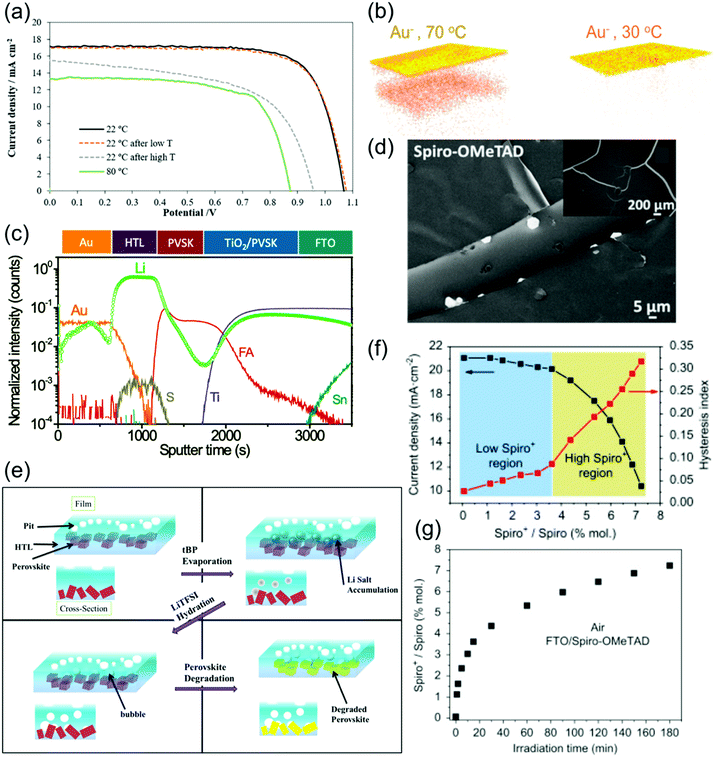 |
| Fig. 5 Stability of spiro-OMeTAD and degradation of spiro-OMeTAD-based PSCs. (a) I–V curves of PSCs employing LiTFSI- and tBP-doped spiro-OMeTAD operating at various temperatures. Reprinted with permission.129 Copyright 2019, Wiley-VCH. (b) Time-of-flight secondary ion mass spectroscopy (ToF-SIMS) reconstructed maps of Au− ions traced using depth profiling after aging at 70 °C or 30 °C in nitrogen under illumination for 15 hours. Reprinted with permission.135 Copyright 2016, American Chemical Society. (c) ToF-SIMS elemental depth profile of PSCs utilizing LiTFSI-doped spiro-OMeTAD, showing a broad distribution of Li over various layer. Reprinted with permission.138 Copyright 2017, Royal Society of Chemistry. (d) SEM image of spiro-OMeTAD-coated MAPbI3 films after 24 h exposure to a high humidity (98 ± 2% RH) environment. Reprinted with permission.72 Copyright 2015, American Chemical Society. (e) Schematic depiction of the various degradation pathways in LiTFSI- and tBP-doped spiro-OMeTAD HTLs upon aging, as well as the interactions between these various mechanisms. Reprinted with permission.112 Copyright 2016, American Chemical Society. (f) Evolution of current density and hysteresis index with spiro-OMeTAD+ concentration. (g) Evolution of spiro-OMeTAD+ concentration in spiro-OMeTAD film on FTO under 1 sun illumination. Both reprinted with permission.92 Copyright 2016, Elsevier. | |
Interface degradation & electrode migration.
Lee et al. showed that adhesion at the spiro-OMeTAD/perovskite interface is poor. This is due to weak interactions as well as a hardening of the film by an accumulation of additives at this interface.132 The addition of a very thin polyethylenimine (PEI) interlayer between the perovskite and spiro-OMeTAD was shown to improve adhesion as well as device stability.133 Additionally, Wei et al. observed photodegradation of the chemical contact between spiro-OMeTAD and Au at the interface under continuous illumination.134
Another stability problem is the migration of metal ions stemming from the electrode through the spiro-OMeTAD HTL into the perovskite, which for Au electrodes occurs at temperatures as low as 70 °C under illumination (Fig. 5b).135 The presence of Au+ inside the perovskite is suggested to degrade PSC performance by producing both shunts, which reduce FF, and inducing deep trap states, which reduce Jsc and Voc. Spiro-OMeTAD, which easily allows ionic diffusion, is found to be a very poor barrier for Au migration. This effect occurs even when PSCs are aged at room temperature.136 In other architectures, a bilayer Cu–Ag electrode has been used to inhibit electrode diffusion and improve stability.137
Li+ diffusion.
Unoxidized Li+ ions in the spiro-OMeTAD layer stemming from LiTFSI doping have been shown to migrate all the way through the device into the perovskite layer (Fig. 5c). As Li+ is small and has a high diffusion tendency, it can diffuse even faster than the intrinsic perovskite ions.138 Interestingly, upon the application of varying LiTFSI concentrations, the variation in spiro-OMeTAD resistivity was significantly different from the variation in device series resistance. This suggests that LiTFSI affects not only the electrical properties of spiro-OMeTAD but also those of other device components. This does not necessarily have to be a negative effect, as time-resolved photoluminescence measurements show that the accumulation of Li+ in TiO2 actually facilitates electron injection in the ETL.138 Dawson et al. showed that Li+ ions can also participate in spontaneous and exothermic perovskite decomposition, producing LiPbX2 or LiX (where X is a halide),139 but the extent of these processes and their effect on PSC performance remain unclear.
tBP-assisted spiro-OMeTAD+ and perovskite degradation.
Kasparavicius et al. observed tBP to degrade the conductivity of spiro-OMeTAD,127 which likely occurs as it decreased the concentration of spiro-OMeTAD+ in both solutions and films over time and even faster at elevated temperatures.111,140 It was suggested that tBP actively reduces spiro-OMeTAD+ through the formation of an oxidized tBP+ ion which is stabilized by TFSI− before reacting further with another molecule of spiro-OMeTAD+.127 This is problematic for PSC stability as it means that the concentration of spiro-OMeTAD+, and hence HTL conductivity, is highly dependent on temperature, illumination, aging, and tBP concentration.
tBP has also been shown to degrade perovskites, which is proposed to occur by reaction with PbI2, a perovskite degradation product, to drive the perovskite decomposition reaction forward.123,141 This degradation was shown to be a slow process contingent on the diffusion of tBP into the perovskite, although increasing the concentration of tBP in a spiro-OMeTAD HTL significantly increased the rate of perovskite degradation.141 The inclusion of a montmorillonite buffer layer between the perovskite and spiro-OMeTAD films was shown to successfully delay perovskite corrosion, and even reduced charge recombination in the devices.141
Moisture degradation.
The hygroscopicity of LiTFSI is a well-known stability concern for PSCs with doped spiro-OMeTAD HTLs.142,143 The rate of degradation of a perovskite film with spiro-OMeTAD deposited on top was found to increase exponentially with higher LiTFSI concentrations, indicating that the hygroscopicity of LiTFSI is directly responsible for the acceleration of perovskite degradation.72 However, Leijtens et al. showed that even when doped with an alternative non-hygroscopic dopant, spiro-OMeTAD incorporated in PSCs still allows a significant amount of moisture-related perovskite degradation during aging in humid ambient conditions at 85 °C.87 SEM images revealed that spiro-OMeTAD cracks and breaks into individual pieces on top of a perovskite layer during high humidity degradation (Fig. 5d),72 suggested to be due to the mechanical stress brought about by volume changes of the perovskite at the beginning of its degradation process, which spiro-OMeTAD cannot mechanically bear.
LiTFSI and tBP co-degradation.
Wang et al. proposed a full degradation mechanism for PSCs considering the effects of both LiTFSI and tBP in a spiro-OMeTAD HTL, shown in Fig. 5e.112 In the first ∼200 hours of storage in the dark, tBP evaporates, causing the slow aggregation of LiTFSI which becomes hydrated over the next ∼300 hours. This leaves ‘bubbles’ of water in the HTL which eventually find their way into the perovskite, so that after 1000 hours device performance is severely degraded. This theory seems to be confirmed further by the observation that spiro-OMeTAD and FAPbI3 perovskite are stable under ambient thermal stressing on their own, but show signs of degradation when interfaced.144 Devices using FK209 in conjunction with LiTFSI and tBP were also found to be less stable than those just using LiTFSI and tBP, although it is not clear why.145
Exceedingly high spiro-OMeTAD+ concentration.
Whilst a small concentration of spiro-OMeTAD+ is highly beneficial for conductivity, Sanchez & Mas-Marza found that a spiro-OMeTAD+ concentration beyond 4 mol% in the HTL increasingly decreases PSC efficiency in the form of Jsc reduction (Fig. 5f).92 Performance degradation due to overoxidation of spiro-OMeTAD is problematic as the concentration of spiro-OMeTAD+ continues to increase under full irradiation for more than 3 hours as shown in Fig. 5g, such that device performance quickly degrades under operating conditions.92Voc also decreases beyond a critical concentration of spiro-OMeTAD+, which was found to be at least partially associated with a higher charge recombination rate.101
In summary, spiro-OMeTAD in its pristine form is already vulnerable to thermal degradation in the operational thermal range, and its commonly used dopants LiTFSI, tBP and FK209 and their byproducts can be hygroscopic,72 cause morphological issues,81 evaporate,129 migrate through the PSC,138 and/or participate in undesirable side reactions.111,127 Spiro-OMeTAD was also found to be a very poor barrier for the migration of ions stemming from dopants, electrodes or other PSC components. Additionally, minor variations in dopant concentration59 as well as environmental conditions such as illumination time and humidity can significantly affect the conductivity of spiro-OMeTAD, worsening reproducibility. As a result of these stability issues, PSCs that use spiro-OMeTAD as a HTL tend to have short lifetimes, especially when unencapsulated.48 Despite these shortcomings, spiro-OMeTAD has persisted throughout the years as a favorite HTL for high efficiency PSCs.25,26,32,40,41,104
4. PTAA
4.1 Pristine PTAA properties
The other HTL regularly used in high efficiency PSCs is PTAA.59 Until recent years, PTAA did not specifically refer to the trimethyl-substituted poly(bis(4-phenyl)(2,4,6-trimethylphenyl)amine but rather described a wide range of polytriphenylamines, a class of organic polymer semiconductors which was popularized in the group of J. Verez for applications in organic field-effect transistors (OFETs).146–148 PTAA was first applied as a HTL in PSCs simultaneously by Heo et al.43 and Noh et al.149 in 2013 where it reached an efficiency of 12%, outperforming both a range of other semiconducting polymers and spiro-OMeTAD when all HTLs were doped with LiTFSI and tBP.43 PTAA was found to interact more strongly with perovskite at the interface than other hole-conducting polymers, possibly improving hole transfer. PTAA has remained a popular HTL choice for PSCs, and has been used in many high-efficiency devices since its introduction.24,44,73,150
The enduring popularity of PTAA as a HTL in PSCs stems, similarly to spiro-OMeTAD, from its thermally stable amorphous morphology, good solubility in a wide range of organic solvents, relatively good hole mobility and good energetic match with most perovskites. Pristine PTAA has repeatedly been found to be completely amorphous, with no glass transition or melting phase feature observed in DSC analysis up to 300 °C,151,152 and is able to form especially smooth films.152
Ko et al. measured the hole mobility of pristine PTAA films with Mw varying from 10–50 kDa to lie between 3–4 × 10−5 cm2 V−1 s−1,152 and observed a slight decrease in hole mobility with increasing Mw (Fig. 6a). This was ascribed to a higher degree of both positional disorder and energetic disorder in higher Mw PTAA, as impurities may be preferentially embedded in longer polymer chains.153 One occasionally also sees hole mobilities around 10−3–10−2 cm2 V−1 s−1 cited for PTAA154 when using OFET techniques, but these vary with layer thickness and contact used, and cannot determine general mobility as accurately as space-charge-limited current (SCLC) or ToF measurements. The conductivity of pristine PTAA was determined to be 5.98 × 10−7 S cm−1.155 Endres et al. showed that p-doped PTAA contains a significant number of sub-gap states below the LUMO, shown to belong to PTAA itself and not the dopant, which may act as traps and aid recombination in the HTL.156
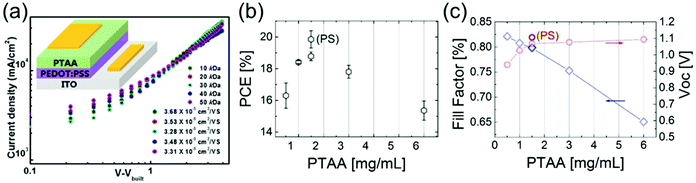 |
| Fig. 6 Impact of PTAA thickness on PSC performance. (a) Hole mobilities determined using SCLC measurements of pristine PTAA films with different Mw ranging from 10–50 kDa, demonstrating decreasing mobility with increasing Mw. Reprinted with permission.152 Copyright 2018, American Chemical Society. (b) Trend in PCE in p–i–n PSCs using various thicknesses of PTAA HTL.69 (c) Trends in FF and Voc in p–i–n PSCs using various thicknesses of PTAA HTL. Both reprinted with permission.69 Copyright 2017, Royal Society of Chemistry. | |
Various values have been measured for the HOMO of undoped PTAA, ranging from −4.91 eV27 using ultraviolet photoelectron spectroscopy to −5.2 eV67 using photoelectron spectroscopy in air (PESA), with the average value found in the literature determined to be −5.19 eV by Westbrook et al.63 The offset between the HOMO of PTAA and the perovskite VB, which again generally lies between −5.2 eV and −5.9 eV82,83 can hence be very small or can be on the order of 100s of meV, so the extent of voltage losses due to hole transport at the PTAA/perovskite interface would depend strongly on the perovskite used.
Hole transfer from the perovskite to PTAA has been found to occur on a 103 ps timescale, which is relatively slow compared to other HTLs such as spiro-OMeTAD, for which hole transfer occurs 4 orders of magnitude faster.157 However, Khadka et al. found PTAA to have low rates of interfacial recombination compared to other popular HTL materials, which was attributed partly to a better interface quality and passivation of defect states in the perovskite at the interface.67
HTL thickness is an important parameter in PSCs, as the transit time of holes through the HTL affects both the speed of charge extraction and recombination in the device. When very thin (8 nm) layers of undoped PTAA are used in p–i–n PSCs, higher-order nonradiative recombination losses, which make up half of the total FF losses, were shown to be nearly eliminated.69 However, PTAA HTLs in n–i–p architectures are normally somewhat thicker, ranging from 30–40 nm, which is still much thinner than spiro-OMeTAD HTLs.44,150 As complete coverage of the perovskite by PTAA is necessary in order to avoid shunts created by contact between the perovskite and the electrode material (Fig. 6b), thinner PTAA HTLs result in worse performances. Even in devices with very thin PTAA layers, the FF is still shown to be limited by HTL thickness (Fig. 6c), indicating room for further conductivity improvements.69
4.2 PTAA doping
Similarly to spiro-OMeTAD, PTAA is conventionally p-doped in order to increase its conductivity, most often using LiTFSI and tBP. Endres et al. confirmed that PTAA can be successfully p-doped by observing a lowering of the Fermi level of PTAA towards the HOMO upon addition of a p-dopant.156 Interestingly, the optimal concentrations of dopants for PTAA-based PSCs (shown in Table 2) are about 4 times lower than the conventional concentrations of LiTFSI and tBP added to spiro-OMeTAD, which could indicate a higher doping efficiency or, less straightforwardly, a lower ideal concentration of holes in PTAA for optimal device performance. Co(III) complexes are not normally used to dope PTAA.158
Table 2 PTAA doping ratios in recent high-efficiency PSCs
Ref. |
PTAA monomer : tBP : LiTFSI (molar ratio) |
24, 44 and 150
|
1 : 0.74 : 0.12 |
159
|
1 : 0.70 : 0.12 |
105
|
1 : 0.39 : 0.08 |
LiTFSI and tBP.
Although the doping mechanisms and effects of LiTFSI and tBP in PTAA-based PSCs are still somewhat unexplored, in some ways they are likely to echo their effects in devices employing spiro-OMeTAD. Additionally, the tendency of Lewis acids to p-dope Lewis base polymers by forming complexes with them160 suggests that LiTFSI may form charge-transfer complexes with the lone electron pair on the nitrogen in PTAA.161 Indeed, Kim et al. observed a new UV-vis absorbance peak arising at 500 nm after doping PTAA with LiTFSI and tBP (Fig. 7a), which they ascribed to the formation of oxidized PTAA+, but PTAA was shown to be oxidized only under illumination.159 It seems likely that tBP performs a similar function in PTAA as it has been observed to have in spiro-OMeTAD, where it forms complexes with LiTFSI to improve its distribution through the film and to avoid the negative effects of dopant aggregation on film morphology.81,112
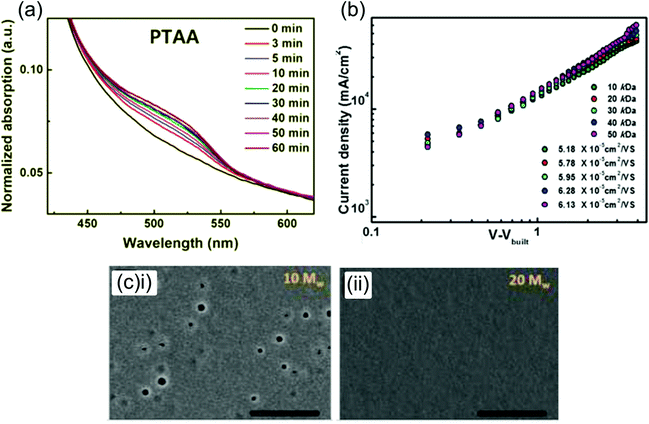 |
| Fig. 7 Effect of doping and Mw on PTAA-based PSCs. (a) Evolution of p-doped PTAA UV-vis absorption peak in LiTFSI- and tBP-doped PTAA films under illumination. Reprinted with permission.159 Copyright 2018, Wiley-VCH. (b) Charge mobilities for LiTFSI- and tBP-doped PTAA films of various Mw ranging from 10 kDa to 50 kDa, showing an increase in mobility with Mw. (c) High resolution scanning electron microscopy (HR-SEM) images showing pinholes in a LiTFSI- and tBP-doped 10 kDa PTAA film, and a lack of pinholes in a 20 kDa film. Both reprinted with permission.152 Copyright 2018, American Chemical Society. | |
The conductivity of LiTFSI- and tBP-doped PTAA was determined to be 3.4 × 10−5 S cm−1,162 around 2 orders of magnitude higher than the conductivity of pristine PTAA measured by other groups.155 Interestingly, the mobility of various Mw PTAA (10–50 kDa) increased from ∼3–4 × 10−5 a to ∼5–6 × 10−5 cm2 V−1 s−1 upon optimized doping with LiTFSI and tBP (Fig. 7a),152 and the mobility of very high Mw doped PTAA (210 kDa) increased even more dramatically from 7.47 × 10−5 cm2 V−1 s−1 to 4.28 × 10−4 cm2 V−1 s−1.27 This may indicate that LiTFSI and tBP don’t only p-dope the polymer but also affect the positional and/or electronic disorder in the film.163 Additionally, LiTFSI- and tBP-doping of PTAA has consistently been found to lower its HOMO,27,152,164 which may reduce voltage losses. Interestingly, PESA measurements made by Watson et al. showed that the addition of LiTFSI to a pristine PTAA film greatly lowers its HOMO from −5.16 eV to −5.44 eV, but the subsequent addition of tBP increases the HOMO again to −5.17 eV.165 The authors suggest that this may be due to the inhibition of p-doping by tBP.
Effect of Mw on LiTFSI- and tBP-doped PTAA.
Interestingly, LiTFSI- and tBP-doped PTAA films showed a significant increase in mobility (Fig. 7b) as well as device performance with increasing Mw.152,166 This trend is opposite to the detrimental effect of increasing Mw on the mobility of undoped PTAA. Ko et al. argued that the addition of LiTFSI turns PTAA from an amorphous into a slightly paracrystalline film,152 in which polymer chains can connect pi-stacked regions without loss of conjugation if they are long enough and can so greatly improve charge transport.163 However, this claim was justified with HR-SEM images (Fig. 7c),152 in which pi-stacking domains are not actually visible. Measurements on LiTFSI- and tBP-doped PTAA films of similar and higher Mw carried out by Nia et al. actually found no signal indicating any crystalline PTAA phases, in-plane order or indeed any effect on morphology.166 Instead, Nia et al. attributed the efficiency improvements observed using high Mw doped PTAA mainly to its reduction of recombination at the perovskite interface and faster hole transfer when compared to lower Mw PTAA.166 Electrochemical impedance spectroscopy measurements confirmed that higher Mw PTAA HTLs are better able to suppress charge recombination at the PTAA/perovskite interface.152 Interestingly, this differs from other semiconducting polymer HTLs such as poly(3-hexylthiophene-2,5-diyl) (P3HT), for which no such relationship between Mw and recombination is observed.167
BCF.
An alternative pathway to PTAA p-doping are Lewis acids, which may either form charge-transfer complexes with PTAA or completely ionize it. Tris-(pentafluorophenyl)borane (BCF, sometimes also referred to as TPFB), which was first applied to PTAA by Luo et al.,164 p-dopes PTAA by forming a frustrated Lewis acid–base adduct155 or by charge transfer to form free PTAA+ and BCF− radicals as shown in Fig. 8a.164 Usually 5–10% BCF is used, and when PTAA doped with 5 wt% BCF was compared to LiTFSI- and tBP-doped PTAA, hole mobility increased slightly from 4.28 × 10−4 cm2 V−1 s−1 to 5.72 × 10−4 cm2 V−1 s−1 and the HOMO was lowered from −5.14 eV to −5.2 eV.164 Additionally, higher efficiency, faster charge extraction, higher recombination resistance (Fig. 8b) and better stability when stored was observed in devices. This could be due to faster charge extraction or a lower concentration of trap states inside the HTL, but BCF may also have a passivating effect on perovskite defects at the interface. It is interesting to note that on several occasions, BCF seems to be the dopant of choice when particularly high concentrations of PTAA of 30 mg ml−1 are used (which will result in a relatively thick HTL).168,169 Ye et al. showed that the performance of PSCs employing BCF-doped PTAA can be further enhanced through a 5 min light soaking treatment at 1 sun illumination (Fig. 8c), which can facilitate electron transfer to BCF and so irreversibly improve conductivity.155 The concentration of holes in the film was found to continue increasing for light soaking treatments up to 30 min, but PSC performance began to deteriorate after 5 min, indicating that an overabundance of free holes in the film may increase the density of traps or scattering centres to the extent that these begin to dominate PSC performance.
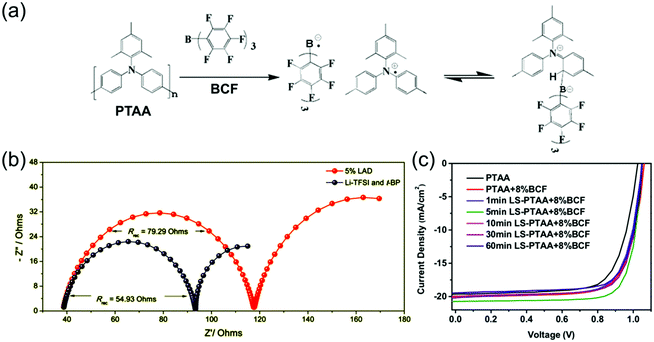 |
| Fig. 8 (a) Doping mechanism by adduct formation and charge transfer of BCF with PTAA. Reprinted with permission.155 Copyright 2017, American Chemical Society. (b) Nyquist plots of PSCs employing either LiTFSI- and tBP-doped or BCF-doped (here labelled LAD) PTAA HTLs, measured at a bias of 0.8 V under simulated AM 1.5 illumination, demonstrating larger recombination resistance in BCF-doped devices. Reprinted with permission.164 Copyright 2018, Royal Society of Chemistry. (c) J–V curves of PSCs utilizing PTAA HTLs doped with 8% BCF and exposed to various lengths of light-soaking treatments, demonstrating optimal performance after 5 min of illumination. Reprinted with permission.155 Copyright 2017, American Chemical Society. | |
Other alternative PTAA dopants.
PTAA has been doped by multiple groups with well-established p-dopant 2,3,5,6-tetrafluoro-7,7,8,8-tetracyanoquinodimethane (F4-TCNQ),170 which has an electron affinity of 5.2 eV that enables it to form charge-transfer complexes with PTAA.160 The addition of 1 wt% F4-TCNQ to PTAA (in p–i–n PSCs) was shown to cause a significant drop in the resistance of the PTAA films, but higher concentrations increased resistance which is likely due to dopant aggregation.171
Not all alternative dopants that have been added to PTAA function as p-dopants. Watson et al. used 1,3,5,7-tetrakis-(p-formylphenyl)-adamantane (TPBA), a UV-activated crosslinker, as an additive to PTAA in addition to LiTFSI and tBP in order to promote better mechanical stability, fracture resistance, and adhesion to the perovskite.165 It was able to increase fracture resistance by 221%, improving stability significantly whilst minimally affecting the electronic properties of the PTAA film.
Alternative PTAA dopants have hence shown significant potential in replacing LiTFSI and tBP and so improving device stability, although more testing under full operational conditions is needed to confirm that they don’t introduce new destabilizing effects. It is not yet clear which properties of these Lewis acids, other than their electron affinity, makes them function well as stable dopants. It would be particularly interesting to investigate whether these dopants form more charge-transfer complexes or free radical ion pairs, and what effects this has on both mobility and ion migration-related stability issues. It is also interesting that the use of different PTAA dopants results in different charge transfer rates and recombination resistances in the device,164 as it suggests that a dopant's chemical structure may affect its propensity to act as a recombination center.
4.3 Stability limitations of PTAA
Thermal degradation.
Pristine PTAA has a high thermal stability, with no melting phase feature observed in DSC analysis up to 300 °C152 and no optically visible crystallization in films after thermal stressing.144 Saliba et al. used PTAA in combination with a quadruple-cation perovskite to produce PSCs that are able to recover an impressive ∼90% of their original efficiency after 500 hours of thermal stressing at 85 °C in nitrogen.32 Mesquita et al. found that only 8.2% of the deterioration in device efficiency of encapsulated PSCs utilizing PTAA upon thermal stressing at 85 °C was irreversible (Fig. 9a),129 which was attributed to the evaporation of dopants tBP and LiTFSI due to the observation of pinhole formation. Yang et al. furthermore proposed that when the thermal expansion coefficients of neighboring layers in PSCs are mismatched, thermal stressing can result in cracking.172 Thermal stressing indeed resulted in cracks in the perovskite in PTAA-based PSCs, accompanied by a 60% efficiency loss.
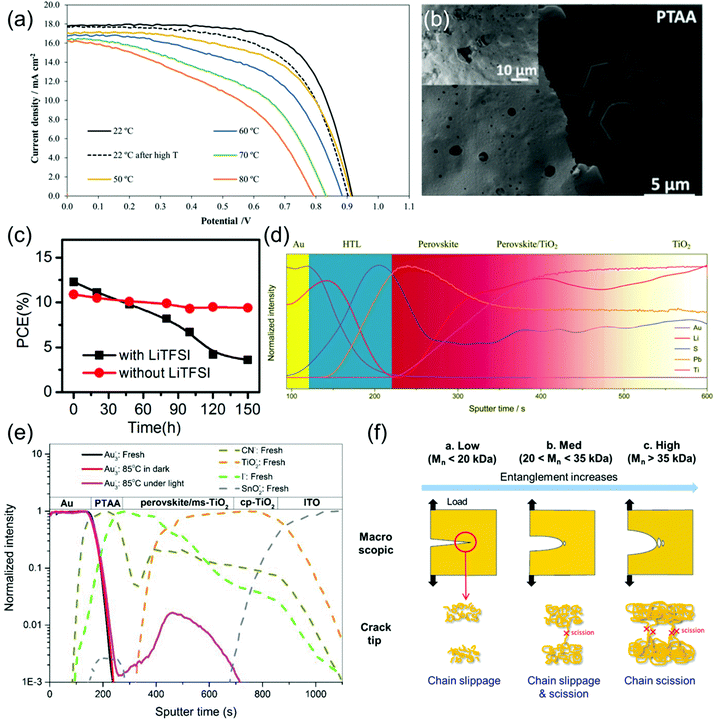 |
| Fig. 9 Stability of PTAA and degradation of PTAA-based PSCs. (a) J–V curves of a PSC utilizing a PTAA HTL operating at various temperatures, as well as at RT after thermal stressing (black dotted line). Reprinted with permission.129 Copyright 2019, Wiley-VCH. (b) SEM image of PTAA coated MAPbI3 films after 24 h exposure to a high humidity (98 ± 2% RH) environment. Reprinted with permission.72 Copyright 2015, American Chemical Society. (c) Stability of PSCs during storage in an ambient atmosphere with 50–60% humidity employing PTAA HTLs with and without the addition of LiTFSI and tBP dopants. Reprinted with permission.174 Copyright 2014, World Scientific. (d) SIMS depth profile showing distribution of Li throughout a PSC containing a LiTFSI- and tBP-doped HTL. Reprinted with permission.164 Copyright 2018 Royal Society of Chemistry. (e) ToF-SIMS analysis of a PSC using a fresh PTAA HTL, one that has been thermally aged, and one that has been thermally aged under illumination, demonstrating Au migration into the perovskite only in the device that had been thermally aged under illumination. Reprinted with permission.175 Copyright 2018, The Institute of Electrical and Electronics Engineers. (f) Diagram illustrating the effect of Mw on crack formation in PTAA. Reprinted with permission.158 Copyright 2017 American Chemical Society. | |
Moisture degradation.
At 98% humidity, a layer of PTAA was shown to slow down the degradation of MAPbI3 significantly, and SEM images (Fig. 9b) revealed large pinholes and gaps but no cracking in the PTAA layer after moisture degradation.72 Interestingly, PTAA doped with LiTFSI slowed down degradation even more than undoped PTAA – it was proposed that hydrophilic polymer HTLs may be able to ‘trap’ water in the HTL and slow its migration into the perovskite. Habisreutinger et al. found that when exposure to air is combined with thermal stressing at 80 °C, doped PTAA-covered perovskite degrades almost entirely within 24 hours, even faster than perovskite covered with spiro-OMeTAD.173 Accordingly, PSCs employing PTAA as HTLs show a fast decrease in efficiency under increasing temperatures in air. However, Bi et al. reported that the addition of LiTFSI to PTAA in PSCs significantly increases the rate of degradation of entire devices when stored in a 50–60% humidity environment as shown in Fig. 9c.174 Luo et al. observed perovskite degradation in aged devices upon storage in air employing LiTFSI and tBP doping but not in devices using alternative dopant BCF.164 Additionally, SEM images of aged PTAA films doped with LiTFSI and tBP showed crystallization, aggregation and pinholes, whilst those doped with BCF remained smooth and homogenous. This indicates that much of the moisture instability of PTAA-based HTLs can be remedied through the use of alternative dopant schemes.
Dopant effects & diffusion.
As explored in previous sections, Li+ ion and tBP migration are likely to negatively affect device stability in HTLs. Luo et al. use SIMS analysis to show that in PSCs using LiTFSI- and tBP-doped PTAA HTLs, Li+ ions migrate through the cell to accumulate at the TiO2/perovskite interface as shown in Fig. 9d.164 Li+ can in this way affect the electrical properties of other PSC layers and possibly degrade the perovskite,139 but may also facilitate electron injection in the ETL.138 Additionally, it is likely that all of the processes that tBP has been suggested to participate in within spiro-OMeTAD, such as complexation with LiTFSI,114 degradation of the perovskite,123,141 and de-doping of oxidized HTL material,111,127 can occur in a similar fashion in PTAA. However, the interaction strength between PTAA or PTAA+ and tBP could affect the extent to which these processes take place.
Au electrode migration.
Migration of Au+ from the electrode into the perovskite layer is detrimental to device efficiency as it can produce shunts between the perovskite and the electrode and because Au+ ions act as deep traps, increasing recombination rates in the device.135 Duong et al. found that after aging at elevated temperatures of 85 °C, Au does in fact migrate through PTAA HTLs into the perovskite (Fig. 9e), but only under light irradiation.175 PSCs using PTAA HTLs and Au electrodes aged at 85 °C over 16 hours under illumination indeed show a significant deterioration in efficiency, but the replacement of Au with indium zinc oxide and a MoOx interlayer significantly improved the thermal stability of the device.
Mechanical stability.
The stability of PSCs can also be compromised when PTAA cohesion or adhesion to the perovskite fails, as good morphological contact is needed to ensure efficient charge transfer. Lee et al. found that a higher Mw results in a higher crack onset strain and better cohesion within PTAA, as the larger degree of entanglement enables the deflection of cracks as demonstrated in Fig. 9f.158 They also found that cracks are more easily formed in PTAA in humid environments. Interestingly, it is possible that a larger Mw PTAA polymer would be better able to accommodate the mechanical stress provided by perovskite volume changes during its degradation as identified by Yang et al.,72 and improved cell stability under humidity. Furthermore, Watson et al. found that the addition of LiTFSI also reduced the adhesion of PTAA to the perovskite layer, and that this adhesion could be improved by the addition of the cross-linker TPBA.165
Reproducibility.
A well-known barrier to reproducibility in polymeric materials is batch-to-batch variability. PTAA can have significant variation in both polymer chain length distributions and trace metal impurity content between batches, which can significantly affect their electronic and to some extent also morphological properties.176 Variations in these parameters are not usually completely reported by the producer and are costly to control precisely.
In summary, although the amorphous morphology of PTAA is stable up to high temperatures, it is relatively vulnerable to allowing moisture ingress into the perovskite and somewhat prone to mechanical failure and delamination at interfaces. Additionally, the migration, evaporation and aggregation of LiTFSI and tBP causes many of the same stability issues as in spiro-OMeTAD, and PTAA is not shown to be a good barrier against ionic diffusion. Hence, the exploration of alternative dopant schemes as well as the use of larger Mw PTAA to improve mechanical stability will provide a pathway to better stability.
5. Comparing spiro-OMeTAD & PTAA
5.1 Energetics
Measuring the HOMO, LUMO and QFLS of semiconducting materials is rarely straightforward, as exposure to oxygen, moisture or light can affect doping levels and chemical reactivity and hence change a material's energetics in difficult to predict ways.38 Especially at interfaces, chemical interaction between HTLs and neighbouring materials can generate interfacial dipoles or band bending,58 significantly affecting charge transfer and recombination.38,60
The difficulty of these interfacial measurements makes them rare, but the HOMO of both spiro-OMeTAD (undoped)82 and PTAA (doped and undoped)156 has been measured at an interface with a perovskite, although a different perovskite composition was used in both cases (Fig. 10a and b). Both HTLs showed similar HOMO offsets with the perovskite and were also suggested to show downward band bending towards the perovskite interface, potentially due to the formation of a surface dipole. This is interesting as downward band bending is theoretically detrimental to charge extraction and recombination kinetics as it directs holes back towards the perovskite interface.
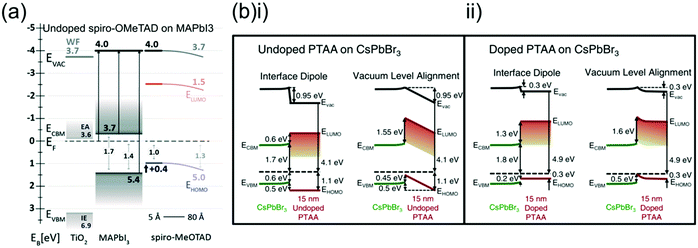 |
| Fig. 10 Comparison of energetics at the HTL/perovskite interface between spiro-OMeTAD and PTAA. (a) Experimentally determined energy level diagram of spiro-OMeTAD deposited on MAPbI3. Reprinted with permission.82 Copyright 2014, Royal Society of Chemistry. (b) Experimentally determined energy level diagram of (i) pristine and (ii) LiTFSI- and tBP-doped PTAA deposited on CsPbBr3, showing two possible scenarios which explain the vacuum level changes either with the presence of an interfacial or with band bending in the PTAA layer. Reprinted with permission.156 Copyright 2017, AIP Publishing. | |
However, this band bending was suggested to be much smaller in doped PTAA, which clearly shows a more beneficial energetic alignment with the perovskite (Fig. 10bii) with only a very small VB-HOMO offset.156 The band structure at the perovskite interface of doped spiro-OMeTAD has not been directly investigated, but tBP- and LiTFSI-doping of spiro-OMeTAD resulted in a 0.8 eV downwards shift of the Fermi level.106 Although this seems to be beneficial for device functionality it is difficult to predict how this affects electronic alignment, as HTL doping may also affect the energetics of the perovskite at the interface.
For PSCs, the alignment of the electronic band structure of HTLs with that of the perovskite absorber/emitter greatly influences device efficiency and specifically Voc. Offsets between the HTL HOMO and perovskite VB or between their Fermi levels, which should be minimized,38,62,63 affect both charge transfer losses and recombination kinetics at the perovskite/HTL interface. Overall, it is suggested that both spiro-OMeTAD and PTAA incur extra Voc losses in PSCs through the energetic offsets between their HOMO and the perovskite VB. However, it is difficult to define either spiro-OMeTAD or PTAA as having ‘better electronic alignment’ with certain perovskites, as their energetics depend heavily on the perovskite used, on doping concentrations and environmental interactions.109 It should hence become common practice to measure interfacial energetic alignments directly when tracking the impact of a novel HTL in a specific PSC architecture, but these measurements are currently not widely performed due to their difficulty.
5.2 Mobility & conductivity
The mobility and conductivity of organic semiconductors may vary with different deposition techniques, chemical purity levels, and exposure to light, oxygen and moisture – hence significant variations in measured values for the same material may be observed. Table 3 summarizes literature values for the mobility and conductivity of spiro-OMeTAD and PTAA with different doping regimes. Overall, pristine spiro-OMeTAD is suggested to have a slightly higher mobility, but similar conductivity to PTAA, and upon optimized doping conductivities continue to be very similar. The large improvements observed in both the mobility and conductivity of LiTFSI- and tBP-doped spiro-OMeTAD upon aging in air109 are very interesting and further highlight the impact of environmental conditions on the functionality of spiro-OMeTAD and PTAA.
Table 3 Mobility and conductivity values from literature for spiro-OMeTAD and PTAA films with various doping regimes
Material |
Mobility (cm2 V−1 s−1) |
Conductivity (S cm−1) |
Spiro-OMeTAD (pristine) |
2 × 10−4 86 |
∼10−7–10−8 103 |
∼4.7 × 10−5 109 |
Spiro-OMeTAD (LiTFSI- and tBP-doped) |
|
2 × 10−5 87,103 |
4 × 10−5 87 |
∼3.5 × 10−5 101 |
1.5 × 10−2 109 (aged 24 h in air) |
∼10−9 109 |
|
∼10−5 109 (aged 24 h in air) |
PTAA (pristine, high Mw) |
∼3–4 × 10−5 152 |
5.98 × 10−7 155 |
7.47 × 10−5 164 |
PTAA (LiTFSI- and tBP-doped) |
∼5–6 × 10−5 152 |
3.4 × 10−5 162 |
4.28 × 10−4 164 |
PTAA (BCF doped) |
5.72 × 10−4 164 |
2.12 × 10−5 155 |
When applied as HTLs in PSCs, the conductivity of both spiro-OMeTAD and PTAA directly limit the series resistance and FF of the device as they are orders of magnitude lower than the conductivity of perovskites.61 Device efficiency can hence be improved by increasing either the mobility or the hole density in spiro-OMeTAD and PTAA, where mobility improvements are preferable as an increase in hole density can also increase the rate of non-radiative recombination in the HTL and especially at the perovskite/HTL interface.58,61
Another strategy to decrease series resistance in PSCs is to reduce HTL thickness such that holes have to travel shorter distances, which also reduces their chance of recombination in the HTL. However, if a HTL is too thin this can allow contact between the perovskite and the electrode, which can cause shunts and increase recombination in the device.68,69,93,94 This trend holds true for both spiro-OMeTAD and PTAA, although the optimal thickness for spiro-OMeTAD at ∼20093,94–370 nm68 is much thicker than that for PTAA, which lies around 30–40 nm.44,150 This suggests that the morphology of PTAA is more successful at preventing contact between the perovskite and electrode, potentially due to its polymeric nature.
5.3 Doping mechanisms & effects
When applied in n–i–p devices, spiro-OMeTAD and PTAA are conventionally doped with Li-TFSI, tBP, and other p-dopants, often at high molar ratios.114 LiTFSI p-dopes the HTL by stabilizing radical cations created through the oxidation of the hole transport material,107 which improves its conductivity103 and can also affect mobility.89tBP is said to improve the morphology and distribution of other dopants through the HTL.81,112 However, LiTFSI is hydrophilic and draws water into the device173 which accelerates perovskite degradation, whilst tBP evaporates easily112 and corrodes the perovskite.141 Removing ionically doped HTLs from n–i–p PSCs has hence been shown to result in significant improvements in operating stability,104,177 but at the cost of lower device efficiencies.102
A direct comparison of the effects of most popular dopant LiTFSI on spiro-OMeTAD and PTAA is lacking. However, non-ionic p-dopant BCF was shown to dope spiro-OMeTAD by ground-state integer charge transfer178 whilst it dopes PTAA through the formation of charge-transfer complexes in the form of frustrated Lewis acid–base adducts.155 These mechanisms do not generally apply to spiro-OMeTAD and PTAA doped with other dopants – for example, it has been suggested that spiro-OMeTAD forms charge-transfer complexes with p-dopant F4-TCNQ,179 and that PTAA is oxidized by LiTFSI through integer charge transfer.159 Whether organic semiconductors are doped by integer charge transfer or charge-transfer complex formation depends on the strength of the Coulomb interaction between the positive charge carrier on the semiconductor and the dopant anion, and hence doping mechanisms differ for each semiconductor-dopant pair.178
Doping mechanisms in spiro-OMeTAD and PTAA are additionally complicated by the involvement of illumination as well as oxygen and moisture, which are often found to boost doping efficiency. It is well-established that exposure to both light and oxygen is necessary to build up a significant concentration of spiro-OMeTAD+ in LiTFSI-doped spiro-OMeTAD,101 and although the doping mechanism of PTAA by LiTFSI is less clear, it is also suggested to require light exposure.159 Although the doping mechanisms of PTAA are much less well-examined, for LiTFSI-doped spiro-OMeTAD multiple complex doping and de-doping mechanisms have been shown to exist. Hence, the large extent to which the conductivity of doped spiro-OMeTAD and PTAA is influenced by exposure to light, oxygen and moisture can be problematic for the reproducibility and stability of these materials.
LiTFSI p-doping seems to increase the conductivity of both spiro-OMeTAD and PTAA by 2–3 orders of magnitude (Table 3), although this was not a direct comparison and different dopant concentrations were used. However, a direct comparison of BCF p-doping has been found to increase the conductivity of PTAA significantly more than that of spiro-OMeTAD, suggesting different doping interactions. It remains somewhat unclear what effects doping can have on mobility. Whilst Abate et al. argued that the ion pairs created by ionic p-dopants cannot improve mobility in terms of the screening of traps and reduction of electronic disorder,89 Hawash et al. observe very significant mobility improvements of more than 2 orders of magnitude when spiro-OMeTAD is doped with LiTFSI and tBP.109 This could potentially be due to the rearrangement of spiro-OMeTAD into locally more crystalline regions facilitated by tBP, as proposed by Lamberti et al.111 The mobility of PTAA has also been shown to be improved by about an order of magnitude by both LiTFSI and BCF doping.164 It is possible that the influence of structural disorder on PTAA is somewhat different from its influence on spiro-OMeTAD. However, the general mechanism of whether and how dopants can affect mobility in these organic semiconductors remains unclear.
5.4 Doped HTLs in PSCs
Doping spiro-OMeTAD and PTAA to improve conductivity seems essential for PSC functionality when HTL thickness is on the order of tens-hundreds of nms. For applications in PSCs, the dopant concentrations generally used in both spiro-OMeTAD and PTAA are much higher than those in inorganic semiconductors. Especially since the moles of tBP added often approaches or exceeds the moles of the semiconductor. This means that dopants strongly affect material properties. Notably, the ideal molar concentration of LiTFSI and tBP is about 4 times higher in spiro-OMeTAD than it is in PTAA, and Bi et al. found that the effect of LiTFSI-doping of PTAA on PSC devices is much smaller and reaches an optimal point at a much lower concentration (Fig. 11a).174 This suggests that the ideal compromise between conductivity and the adverse effects of dopants on morphology, recombination and possibly mobility is reached at lower LiTFSI concentrations in PTAA as compared to spiro-OMeTAD. This is perhaps partially because thinner layers of PTAA are generally used, which decreases the dependence of device efficiency on conductivity.69 However, this drastic difference in dopant concentration seems specific to LiTFSI, as ideal dopant concentrations for both BCF (5%178 for spiro-OMeTAD compared to 5%164 or 8%155 for PTAA) and F4-TCNQ (1.5%179 for spiro-OMeTAD compared to 1%171 for PTAA) are much more similar for spiro-OMeTAD and PTAA.
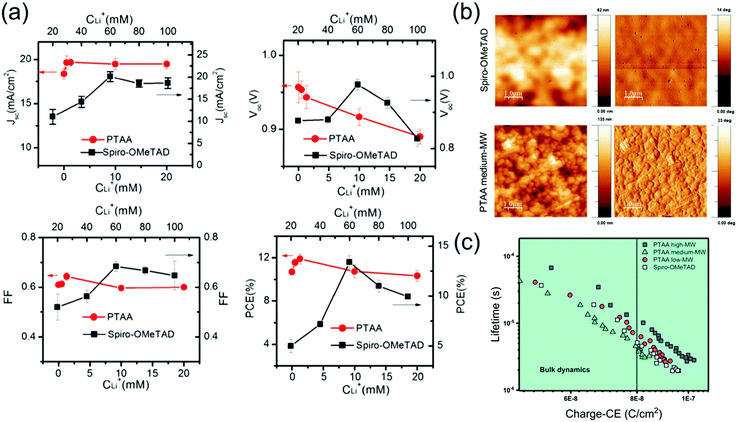 |
| Fig. 11 (a) Photovoltaic parameters of PSCs employing spiro-OMeTAD or PTAA HTLs doped with different concentrations of LiTFSI. Reprinted with permission.174 Copyright 2014, World Scientific Publishing. (b) Atomic force microscopy images of spiro-OMeTAD and PTAA films, showing a significantly rougher surface for PTAA. (c) Plot of charge extraction (CE) against carrier lifetime, demonstrating same recombination mechanisms for each HTL, but slower recombination kinetics for higher Mw PTAA. Both reprinted with permission.166 Copyright 2020, American Chemical Society. | |
Additionally, PSCs employing doped spiro-OMeTAD are conventionally aged in ambient or low humidity conditions for one or more days before electrode deposition in order to improve Voc and FF, whilst devices employing PTAA typically do not requiring aging.105 Although there is evidence that the electronic quality of perovskites can improve with ambient storage,180 Cho et al. describe this efficiency enhancement to also be due to improved conductivity and lower HOMO of spiro-OMeTAD after ambient storage.110 It is possible that PTAA is not usually aged because similar processes do not occur in PTAA, or because PTAA-based PSCs are merely less sensitive to doping efficiency due to lower dopant concentrations and thicknesses. Therefore, PSCs employing PTAA offer the advantage of high efficiency without ageing, which is particularly useful if any of the underlying layers are particularly sensitive to ambient or low humidity conditions.
5.5 Charge transfer & recombination dynamics at perovskite interfaces
Spiro-OMeTAD and PTAA are extremely popular as HTLs for various types of perovskite-based electronic devices, so their charge transfer and recombination dynamics at the interface formed with perovskite materials are highly relevant. In studies on PSCs, hole transfer from perovskite to HTL is found to occur 4 orders of magnitude faster for spiro-OMeTAD as compared to PTAA HTLs,157 and although hole transfer is found to occur on a slightly faster timescale for high Mw PTAA, it is still significantly slower than spiro-OMeTAD.166 As this comparatively slower hole transfer is common between PTAA and other polymeric HTLs, it has been ascribed to worse morphological contact between PTAA and the perovskite (Fig. 11b),166 which requires charges to travel longer paths through the perovskite until they can be transferred.157
When comparing the recombination kinetics in full devices employing spiro-OMeTAD and various Mw PTAA HTLs, Nia et al. showed that although the same type of recombination dominated regardless of the HTL used, the rate of recombination of devices employing spiro-OMeTAD HTLs lies between that of devices employing 10 kDa and 30 kDa PTAA HTLs, with the slowest recombination observed using 115 kDa PTAA (see Fig. 11c).166 Interfacial recombination can be related to many different factors, from accumulation of charges at the interface to HTL/perovskite interactions to trap density in either the HTL or the perovskite. This makes it difficult to ascribe differences in recombination kinetics to specific molecular features of spiro-OMeTAD or PTAA. However, the direct measurement of interfacial recombination currents in full devices will be essential to understanding the effect of both new HTLs and new processing techniques on Voc in PSCs. Overall, holes seem to transfer to spiro-OMeTAD faster, but high Mw PTAA HTLs seem to result in lower recombination in PSCs.
5.6 Performance in PSCs
Both spiro-OMeTAD and PTAA have proven essential to the development of PSCs, with the first four record efficiency devices employing either spiro-OMeTAD or PTAA HTLs.22,24,150,181Table 4 summarizes device data found in the literature in which PSCs employing spiro-OMeTAD and PTAA HTLs were directly compared. The most recent and highest efficiency devices fabricated by Nia et al.,166 which are proposed to best reflect the dominant loss pathways in state-of-the-art PSCs, suggest that spiro-OMeTAD and very high Mw PTAA (both doped with LiTFSI and tBP) are able to produce similar device efficiencies. However, this very high Mw PTAA (∼115 kDa) thus far is rarely used in PSCs, as Mw around ∼15–30 kDa are more common, which were shown to perform worse than spiro-OMeTAD in PSCs. This difference in efficiency, which seems to have become more pronounced as PSC architectures have become more advanced and other loss pathways have been eliminated, may explain why spiro-OMeTAD seems to have become more popular than PTAA in record efficiency devices in very recent years.
Table 4 Device parameters from literature directly comparing spiro-OMeTAD and PTAA HTLs
Authors |
Material |
Device architecture |
PCE (%) |
V
oc
|
J
sc
|
FF |
Heo et al.43 |
Spiro-OMeTAD
|
FTO/mp-TiO2/MAPbI3/spiro-OMeTAD (LiTFSI- and tBP-doped)/Au |
8.4
|
0.855 |
16.7 |
58.8 |
Heo et al.43 |
PTAA
|
FTO/mp-TiO2/MAPbI3/PTAA (LiTFSI- and tBP-doped)/Au |
12.0
|
0.997 |
16.5 |
72.7 |
Bi et al.174 |
Spiro-OMeTAD
|
FTO/bl-TiO2/mp-TiO2/MAPbI3/spiro-OMeTAD (LiTFSI- and tBP-doped)/Au |
13.9
|
0.99 |
21.3 |
0.66 |
Bi et al.174 |
PTAA
|
FTO/bl-TiO2/mp-TiO2/MAPbI3/PTAA (LiTFSI- and tBP-doped)/Au |
12.3
|
0.94 |
20.0 |
0.66 |
Nia et al.166 |
Spiro-OMeTAD
|
FTO/c-TiO2/mp-TiO2 (LiTFSI-doped)/FA1−x−yMAxCsy)Pb(I1−xBrx)3/spiro-OMeTAD (LiTFSI- and tBP-doped)/Au |
18.13
|
1.06 |
22.67 |
0.76 |
Nia et al.166 |
PTAA (10 kDa)
|
FTO/c-TiO2/mp-TiO2 (LiTFSI-doped)/FA1−x−yMAxCsy)Pb(I1−xBrx)3/PTAA (LiTFSI- and tBP-doped)/Au |
15.05
|
1.01 |
21.88 |
0.68 |
Nia et al.166 |
PTAA (115 kDa)
|
FTO/c-TiO2/mp-TiO2 (LiTFSI-doped)/FA1−x−yMAxCsy)Pb(I1−xBrx)3/PTAA (LiTFSI- and tBP-doped)/Au |
18.43
|
1.05 |
22.73 |
0.78 |
Overall, this investigation into the efficiency of spiro-OMeTAD and PTAA as HTLs in PSCs demonstrates that although HTL conductivity and HOMO (measured in vacuum) are often treated as the only important parameters, many other HTL properties also strongly influence PSC efficiency. Energetic alignment and band bending as a result of interactions at the perovskite/HTL interface, charge transfer and recombination dynamics, interface morphology, and optimal HTL thickness all significantly affect device functionality. It hence remains difficult to pinpoint which exact molecular properties of spiro-OMeTAD and PTAA support or undermine their performance as HTLs, as all of these parameters can be affected by molecular structure in complex ways. However, this does emphasize the importance of carrying out energy level and recombination kinetics measurements on novel HTLs in complete devices in order to accurately understand their properties and performance.
5.7 Stability
Thermal stability.
Although PTAA has a higher glass transition and melting temperature than spiro-OMeTAD, this should not necessarily be the parameter by which thermal stability of organic semiconductors in electronic devices should be measured, as both dopants and impurities can strongly affect glass transition temperatures.85 Additionally, thermal degradation in the form of thermally activated ion migration from dopants138 or the electrode,135 or morphological degradation at the HTL/perovskite interface,144 can also occur at temperatures far below the melting point. Kim et al. found that LiTFSI- and tBP-doped PTAA is more stably amorphous than doped spiro-OMeTAD upon thermal stressing at both 65 °C and 85 °C (Fig. 12a),144 but pinhole formation was observed in both doped spiro-OMeTAD and PTAA films upon thermal stressing and ascribed to tBP evaporation.129 This highlights the importance of the further development of alternative doping schemes or dopant-free HTLs for improved thermal stability.
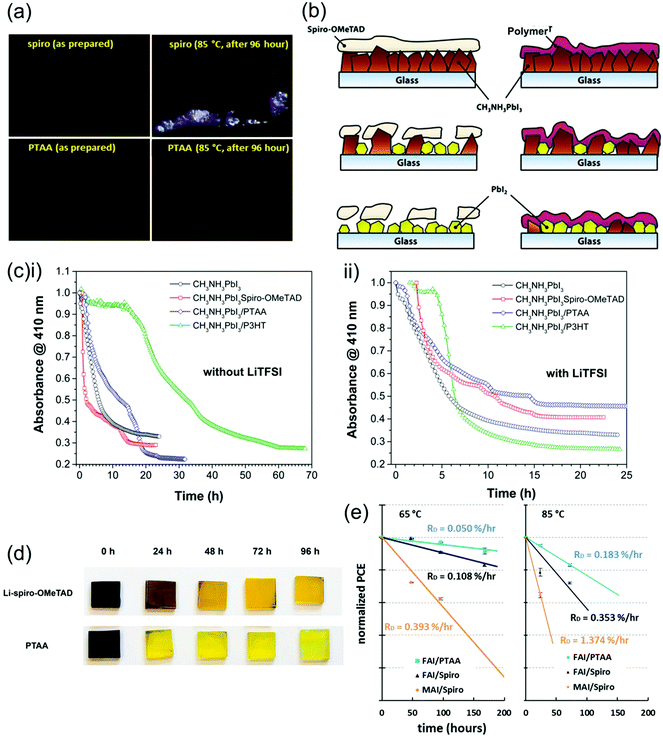 |
| Fig. 12 Comparison between the stability and effect on PSC degradation of spiro-OMeTAD and PTAA. (a) Optical microscope images of spiro-OMeTAD and PTAA thin films upon thermal stressing, showing crystallization in the spiro-OMeTAD film. Reprinted with permission.144 Copyright 2017, Elsevier. (b) Diagram portraying the different effects high humidity environments have on spiro-OMeTAD and polymer HTLs and resulting impact on perovskite degradation. (c) Decrease in absorbance of MAPbI3 films indicating degradation over time in high humidity (98 ± 2% RH) environments covered with different HTLs (i) without and (ii) with LiTFSI dopants. Both reprinted with permission.72 Copyright 2015, American Chemical Society. (d) Color change of MAPbI3 films from black to yellow indicating degradation over time during thermal stressing at 80 °C in air covered with different HTLs. Reprinted with permission.173 Copyright 2014, American Chemical Society. (e) Efficiency deterioration of PSCs employing different perovskite and spiro-OMeTAD or PTAA HTLs under operating conditions, demonstrating superior stability of PTAA-based PSCs. Reprinted with permission.144 Copyright 2017, Elsevier. | |
Moisture stability.
As HTLs provide the main barrier between the perovskite layer and the environment, their response to moisture strongly affects PSC stability. Spiro-OMeTAD has been shown to crack upon exposure to high humidity environments, allowing water to directly degrade the perovskite, whilst PTAA only forms small holes, enabling better coverage and slower degradation of the perovskite as displayed in Fig. 12b.72 This results in better moisture stability of perovskite films covered in PTAA as compared to those covered in spiro-OMeTAD (Fig. 12ci), especially when the HTLs are doped (Fig. 12cii).72 The more pronounced effect of moisture on doped HTLs is likely due to the hygroscopicity of LiTFSI, present in a much higher concentration in spiro-OMeTAD.
When their exposure to air is combined with thermal stressing at 80 °C, PTAA-covered perovskite degrades almost entirely within 24 hours, even faster than perovskite covered with spiro-OMeTAD (Fig. 12d).173 Accordingly, PSCs employing PTAA as HTLs show a slightly faster decrease in efficiency under increasing temperatures in air than those using spiro-OMeTAD. It is interesting that the use of PTAA promotes the degradation of PSCs more than spiro-OMeTAD at high temperature in air, as it is both more thermally stable in nitrogen and more stable under high humidity conditions at room temperature as explained above. This suggests that the combination of heat and moisture may enable a unique degradation mechanism to occur in PTAA.
Operational stability in PSCs.
Under operation, PSCs are subjected to a combination of thermal, moisture, illumination, and electrical stresses which cause both reversible and irreversible device degradation. HTLs and their effect on PSC stability must hence ultimately be evaluated under complete operating conditions. Kim et al. showed that perovskite, spiro-OMeTAD and PTAA thin films are stable in isolation under thermal stressing and only degrade when interfaced,144 suggesting that efficiency degradation can be mainly ascribed to deterioration of the perovskite/HTL interface as well as degradation reactions stemming specifically from contact of the perovskite with the HTL.111,127 Under operating conditions at 85 °C, PSCs employing PTAA HTLs were shown to be significantly more stable than those with spiro-OMeTAD HTLs (Fig. 12e).144 Mesquita et al. revealed that whilst both spiro-OMeTAD and PTAA showed significant losses in efficiency when operating under high temperatures (80 °C).129 However, only 8.2% of this efficiency loss was irreversible after PTAA-based devices returned to room temperature post-thermal stressing, whilst in spiro-OMeTAD based devices 21.6% of efficiency was irreversibly lost. These irreversible efficiency losses was attributed to the evaporation of tBP and LiTFSI in both HTLs, and the higher stability of PTAA was hence ascribed to its lower dopant concentration.129 However, the more stably amorphous morphology of PTAA, or a stronger interaction strength between PTAA and dopants which could reduce the rate of dopant evaporation, could also play a role. Finally, the thinner layer of PTAA used in these devices could also mean that a reduction in HTL conductivity due to dopant evaporation affects device efficiency less strongly.
Overall, this research makes it clear that HTLs strongly affect PSC stability. Specifically, the mechanical qualities of spiro-OMeTAD and PTAA turn out to be centrally important to protecting the perovskite under thermal and moisture stressing. Additionally, many of these stability problems could be linked to the presence of LiTFSI and tBP, which are generally caused by either the dopants themselves or by the byproducts of HTL oxidation, both of which can be hygroscopic,173 cause morphological issues,81 evaporate,129 migrate through the PSC,138 and/or participate in undesirable side reactions.111,127 A significant portion of the instability of PSCs can hence be ascribed to the fact that spiro-OMeTAD and PTAA are most often doped in n–i–p devices.
6. Directions for the next generation of HTLs
6.1 Cost, abundance & reproducibility
It should not be forgotten that the cost, natural abundance and reproducibility of HTLs are essential to their viability for commercialization. Unfortunately, both spiro-OMeTAD and PTAA are still prohibitively expensive, with spiro-OMeTAD carrying a usage cost of 23.96 $ per ml and PTAA carrying a usage cost of 19.80 $ per ml.182 Spiro-OMeTAD requires a multi-step synthesis process which utilizes costly materials such as Pd catalysts, particularly for the precursor containing the spiro core.183 Similarly, the cost of PTAA is driven up by the degree of purification required after polymer synthesis.84 Additionally, dopants such as LiTSFI can get quite expensive even when used in relatively small amounts. The synthetic cost of both materials unfortunately makes them much less favourable for commercial application, and no significantly cheaper synthetic routes have been discovered thus far.
Additionally, both spiro-OMeTAD and PTAA suffer from reproducibility issues. The mobility of these layers can depend strongly on both purity and deposition process (see Sections 3.1 and 4.1), and it is especially difficult to control the batch-to-batch consistency of polymers such as PTAA. When the most popular doping scheme of LiTFSI and tBP is used, complex doping and de-doping processes exist that are strongly influenced by environmental factors such as illumination, humidity and oxygen exposure. Hence, HTL conductivity may vary significantly both between lab environments and within PSCs over time. Ideally, HTLs should also be synthesized and deposited by non-toxic, environmentally friendly processes. Currently the use of chlorobenzene in the deposition of spiro-OMeTAD has been cited as a major possible (eco)toxicity impact.184
Even the most efficient HTL will ultimately not help bring PSCs towards commercialization if considerations of cost are ignored. This makes the discovery of alternative HTLs that can deliver the same functionality as doped spiro-OMeTAD and PTAA at a lower material cost essential for the successful commercialization of PSCs. Hence, the future development of materials should aim to produce a HTL that can be produced at low-cost with high purity, stable morphology and chemical composition, and ideally undoped or containing dopants that are insensitive to standard changes in environmental conditions.
6.2 State of the art in novel HTLs
Despite the enduring dominant popularity of spiro-OMeTAD and PTAA, significant progress has been made over the past few years in designing novel HTLs (for a thorough exploration of these, see detailed reviews by Urieta-Mora et al.185 and Kim et al.186). Many novel small molecule HTLs have been developed through gradual chemical tuning of existing HTLs. In PSCs, these new materials are often more stable than spiro-OMeTAD, but only occasionally result in better PCEs. A foremost example is TQ2, a quinoxaline-based molecule in which the conventional pi-spacer core is replaced with an acceptor group. When used in PSCs, TQ2 is able to produce slightly higher PCEs than spiro-OMeTAD at a much lower synthetic cost.187 Another popular alternative is 9-(2-ethylhexyl)-N,N,N,N-tetrakis(4-methoxyphenyl)-9H-carbazole-2,7-diamine (also referred to as EH44), which is significantly more hydrophobic than spiro-OMeTAD and when doped with Li-free dopants, results in PSCs that retain 94% of their efficiency after 1000 hours continuous operation in ambient conditions.177
Chemical modification of polymers has also been successfully used to synthesize alternative HTLs. Single fluorination of the PTAA side chain has been shown to downshift the HOMO of this HTL to create poly(bis(4-phenyl)(2-fluoro-4,6-dimethylphenyl)amine (also referred to as 1F-PTAA), which was shown to significantly outperform PTAA in PSCs in terms of PCE.159 Sidechain modification can be performed on any polymer to improve its suitability as a HTL – for example, Zhang et al. introduced diethylene glycol groups on the sidechain of carbazole- and benzothiadiazole-based copolymer to optimize its interactions at the perovskite interface for better hole transfer and mobility.188 Older popular semiconducting polymers, such as the very high mobility P3HT, can also be used as HTLs in PSCs. However, P3HT shows high recombination rates at the perovskite interface, hence a passivation layer is required for it to function efficiently as a HTL.182
The past few years have also seen the synthesis of entirely new classes of HTLs. Cross-linkable polymers were successfully employed as a dopant-free and particularly stable HTLs by Wang et al., but efficiencies don’t yet compete with spiro-OMeTAD and PTAA.189 Similarly, Hwang et al. were able to blend together a small molecule organic semiconductor and a conjugated polymer to create a stable HTL with strong adhesion at the perovskite interface, but this did not enable a competitive PCE.190 Bilayer HTLs are another novel strategy in which materials with non-ideal HOMO, LUMO or interfacial interactions can complement each other. Particularly promising examples include NiOx/spiro-OMeTAD191 and carbon nanotubes/spiro-OMeTAD,192 but this strategy opens up far more opportunities for material exploration. Additionally, inorganic HTLs have long been proposed as a cheaper, more stable alternative to organic HTLs, but are difficult to deposit on top of perovskites as their solvent often degrades perovskites. However, some materials such as copper thiocyanate76 and copper phthalocyanine193 have been successfully used in PSCs with efficiencies exceeding 20% and good thermal stabilities through the development of new deposition methods. However, inorganic HTLs have overall only been moderately successful in PSCs despite their seemingly suitable properties as isolated materials such as good chemical stability, high conductivity, and suitable energetics. This may indicate some unfavourable interactions at the HTL/perovskite interface, which are potentially exacerbated by deposition challenges.
In addition to this, a new type of HTL made from a monolayer of self-assembled molecules (SAMs) was very recently introduced in p–i–n PSCs by Magomedov et al.194 This new HTL is unique in its ability to form a very thin (<2 nm) layer whilst still maintaining a uniform and complete barrier between the electrode and the perovskite, which enables very high (∼80%) fill factors without doping. Additionally, the two sides of the SAM can be independently tuned to ensure both good surface coverage and a good energetic match with the perovskite. Although development has only just begun, perhaps it is this shift towards a new type of HTL that that will enable the next significant improvement in PSC efficiency. However, SAMs currently cannot be successfully deposited onto perovskites and hence are not suitable for n–i–p architectures.
It is worth noting that none of these alternative HTLs have yet risen to the same level of popularity as spiro-OMeTAD and PTAA in n–i–p PSCs. This may partially be ascribed to the fact that groups working on PSCs may not always wish to spend lots of time optimizing the HTLs they use and hence prefer well-established options – however, it also suggests that results obtained with novel HTLs may not always be reproducible.
6.3 Future HTL development
It is clear that doped spiro-OMeTAD and PTAA cannot achieve the required stability or low enough cost for commercialization, especially with current doping schemes. We believe that the development of novel HTLs should be carried out along four areas of focus: (a) understanding and tuneability of interactions at the perovskite interface, (b) characterization within the full PSC device stack, (c) development of alternative dopants or dopant-free materials, and (d) focus on material cost.
In order to further improve stability and efficiency, it will be critical to understand the environment created by chemical interaction between materials at the perovskite/HTL interface.195 Considering the ongoing development of complex perovskite compositions with differing energetics, it is clear that any broadly successful HTL must also be tuneable to interact favourably with a range of different perovskite materials. Thus far, it is clear that molecular tuning of both small molecule and polymer HTL materials can be very successful at improving stability in PSCs, as well as adjusting energetics, hole mobility and morphology. However, it remains difficult to predict how these properties will affect how charges behave at the interface in terms of transfer kinetics and recombination, which critically affects PCE.
Because of this, it is essential to characterize both the electronic properties and stability of any new materials within the full PSC device stack, where they have had the chance to interact with any neighbouring materials. Measurements on stand-alone thin films of organic semiconductors can be severely different to its actual properties within the device, as both interactions between the HTL and the perovskite and environmental factors can strongly influence fundamental HTL properties. Similarly, device stability measurements should ultimately be carried out at realistic operating conditions under elevated temperatures, humid environments, and at the maximum power point.
Spiro-OMeTAD and PTAA measurements show that HTL doping can aid charge extraction in PSCs by improving energetic alignment with the perovskite, increasing mobility and conductivity in the HTL, and increasing the voltage drop through the perovskite layer, aiding charge extraction.61 However, the increase in the concentration of holes in the HTL caused by doping may also increase recombination at the interface,58 and the presence of dopant molecules may decrease mobility89 or cause morphological problems.81 Accordingly, alternative dopants often result in much better device stabilities,119,121,123,164 and may in some cases provide a more facile path to developing more stable HTLs. This makes an understanding of doping mechanisms, effects and efficiencies centrally important to the further development of HTLs. The development of alternative dopants may go a long way in improving the stability of spiro-OMeTAD, PTAA and other existing HTLs. However, materials that are completely dopant-free are expected to have much better reproducibility and stability, so this should be the ultimate focus of novel HTL development.
Finally, novel HTLs need to be significantly cheaper than spiro-OMeTAD and PTAA. Currently, novel small molecule HTLs are often produced by making small chemical modifications such as side chain replacements to spiro-OMeTAD. However, it is unlikely that small chemical modifications will significantly change the synthetic routes by which these molecules can be produced, and hence their production is expected to be similarly expensive. This may indicate that a stricter focus on cost is necessary within the field when judging new HTLs, and in fact candidates with lower synthetic costs187 deserve more credit for fulfilling this requirement.
7. Summary & outlook
Over the past decade, research into how spiro-OMeTAD and PTAA function so well as HTLs has enabled a much deeper understanding of both the properties a HTL requires and the best ways to accurately measure these properties. Applying these lessons is critical to pushing PSCs towards their theoretical efficiency limits and better stabilities. So, which is the better HTL – spiro-OMeTAD or PTAA? Despite the seeming decrease in popularity of PTAA in record-efficiency n–i–p devices in recent years, direct device comparisons have given conflicting answers, so there is no straightforward choice. Both spiro-OMeTAD and PTAA have properties that inhibit their optimal functioning as HTLs, which include sub-optimal conductivity and significant recombination at the perovskite/HTL interface. Stability studies do indicate that PTAA has better thermal and mechanical stability, possibly due to the lower concentration of dopants generally used, leading to longer PSC lifetimes. However, both spiro-OMeTAD and PTAA suffer from a host of stability issues linked to dopant hydrophilicity, evaporation, migration, aggregation, and reactivity, making the development of alternative dopants a promising pathway to improving PSC stability.
Additionally, despite their enduring popularity in research environments spiro-OMeTAD and PTAA remain prohibitively expensive for commercial PSCs. This, along with the potential for further efficiency and stability improvements, continues to motivate the search for new HTLs, which should be informed by the wealth of knowledge about spiro-OMeTAD and PTAA HTLs generated over the past decade. This review has revealed that these materials are successful not only due to their amorphous nature, energetics and conductivity, but the chemical interactions at the perovskite interface, the resulting charge transfer characteristics, and complex dopant interactions also play a significant role. Additionally, it highlights that material properties such as energy levels, conductivity, and stability should ideally be measured and compared within complete devices. It also shows that a significant amount of the stability problems associated with n–i–p PSCs can be traced back to the dopants used in spiro-OMeTAD and PTAA. Ultimately, the commercialization of PSCs will necessitate cheaper and more reproducible materials.
All of this research is equally applicable to the many other contexts in which spiro-OMeTAD and PTAA are frequently used, including various types of LEDs, DSSCs, OFETs and even more novel applications in devices such as photodetectors. The deep understanding of the properties of these organic semiconductors that has been developed over the past decade, presented in this review, enables researchers to make predictions about how their will behave in a variety of electronic applications.
The research examined in this review indicates that interfacial recombination at the HTL interface represents a bottleneck for further efficiency improvements in PSCs, and stability has long been established as a limiting factor on further PSC development. Within n–i–p PSCs, the HTL is uniquely positioned to address both of these problems. The development of new HTLs should be extended beyond its current focus on conductivity and HOMO improvements, and rather become more informed by an improved understanding of the various ways in which HTL interactions with the perovskite, dopants, and the environment affect device efficiency and stability. The great strides that have been made in understanding the fundamental properties of spiro-OMeTAD and PTAA over the past decade will be essential to developing the next generation of HTL materials.
Acronyms
BCF | Tris-(pentafluorophenyl)borane |
CB | Conduction band |
Cs | Cesium |
c-Si | Crystalline silicon |
CTL | Charge transport layer |
DSSC | Dye-sensitized solar cell |
ETL | Electron transport layer |
F4-TCNQ | 2,3,5,6-Tetrafluoro-7,7,8,8-tetracyanoquinodimethane |
FA | Formamidinium |
FK209 | Tris[2-(1H-pyrazol-1-yl)-(4-tert-butylpyridine)cobalt(III) tris(bis(trifluoromethylsulfonyl)imide)] |
FF | Fill factor |
HOMO | Highest occupied molecular orbital |
HTL | Hole transport layer |
J
sc
| Short-circuit current density |
LED | Light emitting diode |
LiTFSI | Lithium bistrifluoromethanesulfonimidate |
LUMO | Lowest unoccupied molecular orbital |
MA | Methylammonium |
OFET | Organic field-effect transistor |
P3HT | Poly(3-hexylthiophene-2,5-diyl) |
Pb | Lead |
PCE | Power conversion efficiency |
PESA | Photoelectron spectroscopy in air |
PSC | Perovskite solar cell |
PTAA | Poly[bis(4-phenyl)(2,4,6-trimethylphenyl)amine] |
PV | Photovoltaic |
QFLS | Quasi Fermi level splitting |
SEM | Scanning electron microscopy |
SIMS | Secondary ion mass spectrometry |
Sn | Tin |
Spiro-OMeTAD | 2,2′,7,7′-Tetrakis(N,N-p-dimethoxyphenylamino)-9,9′-spirobifluorene |
tBP | 4-tert-Butylpyridine |
ToF | Time-of-flight |
TPBA | 1,3,5,7-Tetrakis-(p-formylphenyl)-adamantane |
VB | Valence band |
V
oc
| Open-circuit voltage |
Conflicts of interest
There are no conflicts of interest to declare.
Acknowledgements
S. A. H. acknowledges support from the EPSRC (grant numbers EP/R020574/1 and EP/R023581/1) and the UKRI Global Challenge Research Fund project SUNRISE (grant number EP/P032591/1). T. J. M. thanks the Royal Commission for the Exhibition of 1851 for their financial support through a Research Fellowship.
References
- European Union, World Energy Technology Outlook – 2050, 2012.
-
IPCC, Global warming of 1.5 °C. An IPCC Special Report on the impacts of global warming of 1.5 °C above pre-industrial levels and related global greenhouse gas emission pathways, in the context of strengthening the global response to the threat of climate change, sustainable development, and efforts to eradicate poverty, ed. V. Masson-Delmotte, P. Zhai, H. O. Pörtner, D. Roberts, J. Skea, P.R. Shukla, A. Pirani, W., Moufouma-Okia, C. Péan, R. Pidcock, S. Connors, J. B. R. Matthews, Y. Chen, X. Zhou, M. I. Gomis, E. Lonnoy, T. Maycock, M. Tignor and T. Waterfield, 2018 Search PubMed
.
- C. F. Shih, T. Zhang, J. Li and C. Bai, Powering the Future with Liquid Sunshine, Joule, 2018, 2, 1925–1949 CrossRef CAS
.
- A. Polman, M. Knight, E. C. Garnett, B. Ehrler and W. C. Sinke, Photovoltaic materials: Present efficiencies and future challenges, Science, 2016, 352, aad4424 CrossRef
.
- J. Yan and B. R. Saunders, Third-generation solar cells: a review and comparison of polymer:fullerene, hybrid polymer and perovskite solar cells, RSC Adv., 2014, 4, 43286–43314 RSC
.
- W. E. I. Sha, X. Ren, L. Chen and W. C. H. Choy, The efficiency limit of CH3NH3PbI3 perovskite solar cells, Appl. Phys. Lett., 2015, 106, 221104 CrossRef
.
- M. Saliba, J.-P. Correa-Baena, M. Grätzel, A. Hagfeldt and A. Abate, Perovskite Solar Cells: From the Atomic Level to Film Quality and Device Performance, Angew. Chem., Int. Ed., 2018, 57, 2554–2569 CrossRef CAS PubMed
.
- A. D. Vos, Detailed balance limit of the efficiency of tandem solar cells, J. Phys. Appl. Phys., 1980, 13, 839–846 CrossRef
.
- A. Kojima, K. Teshima, Y. Shirai and T. Miyasaka, Organometal Halide Perovskites as Visible-Light Sensitizers for Photovoltaic Cells, J. Am. Chem. Soc., 2009, 131, 6050–6051 CrossRef CAS PubMed
.
- B. O’Regan and M. Gratzel, A low-cost, high-efficiency solar cell based on dye-sensitized colloidal Ti02 films, Nature, 1991, 353, 737–740 CrossRef
.
- H.-S. Kim, C.-R. Lee, J.-H. Im, K.-B. Lee, T. Moehl, A. Marchioro, S.-J. Moon, R. Humphry-Baker, J.-H. Yum, J. E. Moser, M. Grätzel and N.-G. Park, Lead Iodide Perovskite Sensitized All-Solid-State Submicron Thin Film Mesoscopic Solar Cell with Efficiency Exceeding 9%, Sci. Rep., 2012, 2, 591 CrossRef PubMed
.
- Q. Lin, A. Armin, R. C. R. Nagiri, P. L. Burn and P. Meredith, Electro-optics of perovskite solar cells, Nat. Photonics, 2015, 9, 106–112 CrossRef CAS
.
- N.-G. Park, Perovskite solar cells: an emerging photovoltaic technology, Mater. Today, 2015, 18, 65–72 CrossRef CAS
.
- M. M. Lee, J. Teuscher, T. Miyasaka, T. N. Murakami and H. J. Snaith, Efficient Hybrid Solar Cells Based on Meso-Superstructured Organometal Halide Perovskites, Science, 2012, 338, 643–647 CrossRef CAS
.
- L. Etgar, P. Gao, Z. Xue, Q. Peng, A. K. Chandiran, B. Liu, Md. K. Nazeeruddin and M. Grätzel, Mesoscopic CH3NH3PbI3/TiO2 Heterojunction Solar Cells, J. Am. Chem. Soc., 2012, 134, 17396–17399 CrossRef CAS
.
- S. D. Stranks, G. E. Eperon, G. Grancini, C. Menelaou, M. J. P. Alcocer, T. Leijtens, L. M. Herz, A. Petrozza and H. J. Snaith, Electron-Hole Diffusion Lengths Exceeding 1 Micrometer in an Organometal Trihalide Perovskite Absorber, Science, 2013, 342, 341–344 CrossRef CAS PubMed
.
- G. Xing, N. Mathews, S. Sun, S. S. Lim, Y. M. Lam, M. Gratzel, S. Mhaisalkar and T. C. Sum, Long-Range Balanced Electron- and Hole-Transport Lengths in Organic-Inorganic CH3NH3PbI3, Science, 2013, 342, 344–347 CrossRef CAS PubMed
.
- C. S. Ponseca, T. J. Savenije, M. Abdellah, K. Zheng, A. Yartsev, T. Pascher, T. Harlang, P. Chabera, T. Pullerits, A. Stepanov, J.-P. Wolf and V. Sundström, Organometal Halide Perovskite Solar Cell Materials Rationalized: Ultrafast Charge Generation, High and Microsecond-Long Balanced Mobilities, and Slow Recombination, J. Am. Chem. Soc., 2014, 136, 5189–5192 CrossRef CAS PubMed
.
- J. M. Ball, M. M. Lee, A. Hey and H. J. Snaith, Low-temperature processed meso-superstructured to thin-film perovskite solar cells, Energy Environ. Sci., 2013, 6, 1739 RSC
.
- M. Liu, M. B. Johnston and H. J. Snaith, Efficient planar heterojunction perovskite solar cells by vapour deposition, Nature, 2013, 501, 395–398 CrossRef CAS PubMed
.
- J.-Y. Jeng, Y.-F. Chiang, M.-H. Lee, S.-R. Peng, T.-F. Guo, P. Chen and T.-C. Wen, CH3NH3PbI3 Perovskite/Fullerene Planar-Heterojunction Hybrid Solar Cells, Adv. Mater., 2013, 25, 3727–3732 CrossRef CAS
.
- J. Burschka, N. Pellet, S.-J. Moon, R. Humphry-Baker, P. Gao, M. K. Nazeeruddin and M. Grätzel, Sequential deposition as a route to high-performance perovskite-sensitized solar cells, Nature, 2013, 499, 316–319 CrossRef CAS
.
- Z. Xiao, Q. Dong, C. Bi, Y. Shao, Y. Yuan and J. Huang, Solvent Annealing of Perovskite-Induced Crystal Growth for Photovoltaic-Device Efficiency Enhancement, Adv. Mater., 2014, 26, 6503–6509 CrossRef CAS PubMed
.
- N. J. Jeon, J. H. Noh, W. S. Yang, Y. C. Kim, S. Ryu, J. Seo and S. I. Seok, Compositional engineering of perovskite materials for high-performance solar cells, Nature, 2015, 517, 476–480 CrossRef CAS PubMed
.
- M. Saliba, T. Matsui, J.-Y. Seo, K. Domanski, J.-P. Correa-Baena, M. K. Nazeeruddin, S. M. Zakeeruddin, W. Tress, A. Abate, A. Hagfeldt and M. Grätzel, Cesium-containing triple cation perovskite solar cells: improved stability, reproducibility and high efficiency, Energy Environ. Sci., 2016, 9, 1989–1997 RSC
.
- M. Abdi-Jalebi, Z. Andaji-Garmaroudi, S. Cacovich, C. Stavrakas, B. Philippe, J. M. Richter, M. Alsari, E. P. Booker, E. M. Hutter, A. J. Pearson, S. Lilliu, T. J. Savenije, H. Rensmo, G. Divitini, C. Ducati, R. H. Friend and S. D. Stranks, Maximizing and stabilizing luminescence from halide perovskites with potassium passivation, Nature, 2018, 555, 497–501 CrossRef CAS PubMed
.
- D. Luo, W. Yang, Z. Wang, A. Sadhanala, Q. Hu, R. Su, R. Shivanna, G. F. Trindade, J. F. Watts, Z. Xu, T. Liu, K. Chen, F. Ye, P. Wu, L. Zhao, J. Wu, Y. Tu, Y. Zhang, X. Yang, W. Zhang, R. H. Friend, Q. Gong, H. J. Snaith and R. Zhu, Enhanced photovoltage for inverted planar heterojunction perovskite solar cells, Science, 2018, 360, 1442–1446 CrossRef CAS PubMed
.
- M. Batmunkh, K. Vimalanathan, C. Wu, A. S. R. Bati, L. Yu, S. A. Tawfik, M. J. Ford, T. J. Macdonald, C. L. Raston, S. Priya, C. T. Gibson and J. G. Shapter, Efficient Production of Phosphorene Nanosheets via Shear Stress Mediated Exfoliation for Low-Temperature Perovskite Solar Cells, Small Methods, 2019, 3, 1800521 CrossRef
.
- T. J. Macdonald, M. Batmunkh, C. Lin, J. Kim, D. D. Tune, F. Ambroz, X. Li, S. Xu, C. Sol, I. Papakonstantinou, M. A. McLachlan, I. P. Parkin, J. G. Shapter and J. R. Durrant, Origin of Performance Enhancement in TiO2 -Carbon Nanotube Composite Perovskite Solar Cells, Small Methods, 2019, 3, 1900164 CrossRef CAS
.
- C. Lin, J. Lee, J. Kim, T. J. Macdonald, J. Ngiam, B. Xu, M. Daboczi, W. Xu, S. Pont, B. Park, H. Kang, J. Kim, D. J. Payne, K. Lee, J. R. Durrant and M. A. McLachlan, Origin of Open-Circuit Voltage Enhancements in Planar Perovskite Solar Cells Induced by Addition of Bulky Organic Cations, Adv. Funct. Mater., 2020, 30, 1906763 CrossRef CAS
.
- A. S. R. Bati, M. Hao, T. J. Macdonald, M. Batmunkh, Y. Yamauchi, L. Wang and J. G. Shapter, 1D-2D Synergistic MXene-Nanotubes Hybrids for Efficient Perovskite Solar Cells, Small, 2021, 2101925 CrossRef CAS
.
- M. Saliba, T. Matsui, K. Domanski, J.-Y. Seo, A. Ummadisingu, S. M. Zakeeruddin, J.-P. Correa-Baena, W. R. Tress, A. Abate, A. Hagfeldt and M. Gratzel, Incorporation of rubidium cations into perovskite solar cells improves photovoltaic performance, Science, 2016, 354, 206–209 CrossRef CAS
.
- N. Aristidou, I. Sanchez-Molina, T. Chotchuangchutchaval, M. Brown, L. Martinez, T. Rath and S. A. Haque, The Role of Oxygen in the Degradation of Methylammonium Lead Trihalide Perovskite Photoactive Layers, Angew. Chem., Int. Ed., 2015, 54, 8208–8212 CrossRef CAS PubMed
.
- N. Aristidou, C. Eames, I. Sanchez-Molina, X. Bu, J. Kosco, M. S. Islam and S. A. Haque, Fast oxygen diffusion and iodide defects mediate oxygen-induced degradation of perovskite solar cells, Nat. Commun., 2017, 8, 15218 CrossRef PubMed
.
- D. Bryant, N. Aristidou, S. Pont, I. Sanchez-Molina, T. Chotchunangatchaval, S. Wheeler, J. R. Durrant and S. A. Haque, Light and oxygen induced degradation limits the operational stability of methylammonium lead triiodide perovskite solar cells, Energy Environ. Sci., 2016, 9, 1655–1660 RSC
.
- F. T. F. O’Mahony, Y. H. Lee, C. Jellett, S. Dmitrov, D. T. J. Bryant, J. R. Durrant, B. C. O’Regan, M. Graetzel, M. K. Nazeeruddin and S. A. Haque, Improved environmental stability of organic lead trihalide perovskite-based photoactive-layers in the presence of mesoporous TiO2, J. Mater. Chem. A, 2015, 3, 7219–7223 RSC
.
- S. Pont, D. Bryant, C.-T. Lin, N. Aristidou, S. Wheeler, X. Ma, R. Godin, S. A. Haque and J. R. Durrant, Tuning CH3NH3 Pb(I1−xBrx)3 perovskite oxygen stability in thin films and solar cells, J. Mater. Chem. A, 2017, 5, 9553–9560 RSC
.
- C. M. Wolff, P. Caprioglio, M. Stolterfoht and D. Neher, Nonradiative Recombination in Perovskite Solar Cells: The Role of Interfaces, Adv. Mater., 2019, 31, 1902762 CrossRef CAS PubMed
.
- J. Jeong, M. Kim, J. Seo, H. Lu, P. Ahlawat, A. Mishra, Y. Yang, M. A. Hope, F. T. Eickemeyer, M. Kim, Y. J. Yoon, I. W. Choi, B. P. Darwich, S. J. Choi, Y. Jo, J. H. Lee, B. Walker, S. M. Zakeeruddin, L. Emsley, U. Rothlisberger, A. Hagfeldt, D. S. Kim, M. Grätzel and J. Y. Kim, Pseudo-halide anion engineering for α-FAPbI3 perovskite solar cells, Nature, 2021, 592, 381–385 CrossRef CAS PubMed
.
- J. J. Yoo, S. Wieghold, M. C. Sponseller, M. R. Chua, S. N. Bertram, N. T. P. Hartono, J. S. Tresback, E. C. Hansen, J.-P. Correa-Baena, V. Bulović, T. Buonassisi, S. S. Shin and M. G. Bawendi, An interface stabilized perovskite solar cell with high stabilized efficiency and low voltage loss, Energy Environ. Sci., 2019, 12, 2192–2199 RSC
.
- Q. Jiang, Y. Zhao, X. Zhang, X. Yang, Y. Chen, Z. Chu, Q. Ye, X. Li, Z. Yin and J. You, Surface passivation of perovskite film for efficient solar cells, Nat. Photonics, 2019, 13, 460–466 CrossRef CAS
.
- U. Bach, D. Lupo, P. Comte, J. E. Moser, F. Weissörtel, J. Salbeck, H. Spreitzer and M. Grätzel, Solid-state dye-sensitized mesoporous TiO2 solar cells with high photon-to-electron conversion efficiencies, Nature, 1998, 395, 583–585 CrossRef CAS
.
- J. H. Heo, S. H. Im, J. H. Noh, T. N. Mandal, C.-S. Lim, J. A. Chang, Y. H. Lee, H. Kim, A. Sarkar, Md. K. Nazeeruddin, M. Grätzel and S. I. Seok, Efficient inorganic–organic hybrid heterojunction solar cells containing perovskite compound and polymeric hole conductors, Nat. Photonics, 2013, 7, 486–491 CrossRef CAS
.
- W. S. Yang, B.-W. Park, E. H. Jung, N. J. Jeon, Y. C. Kim, D. U. Lee, S. S. Shin, J. Seo, E. K. Kim, J. H. Noh and S. I. Seok, Iodide management in formamidinium-lead-halide–based perovskite layers for efficient solar cells, Science, 2017, 356, 1376–1379 CrossRef CAS PubMed
.
- H. Li, K. Fu, P. P. Boix, L. H. Wong, A. Hagfeldt, M. Grätzel, S. G. Mhaisalkar and A. C. Grimsdale, Hole-Transporting Small Molecules Based on Thiophene Cores for High Efficiency Perovskite Solar Cells, ChemSusChem, 2014, 7, 3420–3425 CrossRef CAS
.
- Y. Liu, Q. Chen, H.-S. Duan, H. Zhou, Y. (Michael) Yang, H. Chen, S. Luo, T.-B. Song, L. Dou, Z. Hong and Y. Yang, A dopant-free organic hole transport material for efficient planar heterojunction perovskite solar cells, J. Mater. Chem. A, 2015, 3, 11940–11947 RSC
.
- M. Saliba, S. Orlandi, T. Matsui, S. Aghazada, M. Cavazzini, J.-P. Correa-Baena, P. Gao, R. Scopelliti, E. Mosconi, K.-H. Dahmen, F. De Angelis, A. Abate, A. Hagfeldt, G. Pozzi, M. Graetzel and M. K. Nazeeruddin, A molecularly engineered hole-transporting material for efficient perovskite solar cells, Nat. Energy, 2016, 1, 15017 CrossRef CAS
.
- Z. H. Bakr, Q. Wali, A. Fakharuddin, L. Schmidt-Mende, T. M. Brown and R. Jose, Advances in hole transport materials engineering for stable and efficient perovskite solar cells, Nano Energy, 2017, 34, 271–305 CrossRef CAS
.
- O. A. Jaramillo-Quintero, R. S. Sanchez, M. Rincon and I. Mora-Sero, Bright Visible-Infrared Light Emitting Diodes Based on Hybrid Halide Perovskite with Spiro-OMeTAD as a Hole-Injecting Layer, J. Phys. Chem. Lett., 2015, 6, 1883–1890 CrossRef CAS PubMed
.
- Y.-S. Liu, S. Guo, J. Feng, Y.-F. Liu, Y.-G. Bi, D. Yin, X.-L. Zhang and H.-B. Sun, Enhanced efficiency of all-inorganic perovskite light-emitting diodes by using F4-TCNQ-doped PTAA as a hole-transport layer, Opt. Lett., 2019, 44, 4817–4820 CrossRef CAS
.
- N. Zhang, G. Tian, X. Wu, W. Qin, M. Liu, Y. Li, H. Rui, L. Li, Y. Hua and S. Yin, Low driving voltage and enhanced performance of solution-processed blue flexible organic light-emitting diode with PTAA/AgNWs/PTAA composite hole injection layers, J. Phys. Appl. Phys., 2019, 52, 315102 CrossRef CAS
.
- C.-Y. Hsu, Y.-C. Chen, R. Y.-Y. Lin, K.-C. Ho and J. T. Lin, Solid-state dye-sensitized solar cells based on spirofluorene (spiro-OMeTAD) and arylamines as hole transporting materials, Phys. Chem. Chem. Phys., 2012, 14, 14099 RSC
.
- J. Veres, S. Ogier, G. Lloyd and D. de Leeuw, Gate Insulators in Organic Field-Effect Transistors, Chem. Mater., 2004, 16, 4543–4555 CrossRef CAS
.
- J. Li, S. Yuan, G. Tang, G. Li, D. Liu, J. Li, X. Hu, Y. Liu, J. Li, Z. Yang, S. F. Liu, Z. Liu, F. Gao and F. Yan, High-Performance, Self-Powered Photodetectors Based on Perovskite and Graphene, ACS Appl. Mater. Interfaces, 2017, 9, 42779–42787 CrossRef CAS
.
- A. Bera, A. Das Mahapatra, S. Mondal and D. Basak, Sb2S3/Spiro-OMeTAD Inorganic–Organic Hybrid p–n Junction Diode for High Performance Self-Powered Photodetector, ACS Appl. Mater. Interfaces, 2016, 8, 34506–34512 CrossRef CAS
.
- V. Sarritzu, N. Sestu, D. Marongiu, X. Chang, S. Masi, A. Rizzo, S. Colella, F. Quochi, M. Saba, A. Mura and G. Bongiovanni, Optical determination of Shockley-Read-Hall and interface recombination currents in hybrid perovskites, Sci. Rep., 2017, 7, 44629 CrossRef CAS PubMed
.
- M. Stolterfoht, P. Caprioglio, C. Wolff, J. Márquez Prieto, J. Nordmann, S. Zhang, D. Rothhardt, U. Hörmann, Y. Amir, A. Redinger, L. Kegelmann, F. Zu, S. Albrecht, N. Koch, T. Kirchartz, M. Saliba, T. Unold and D. Neher, The impact of energy alignment and interfacial recombination on the internal and external open-circuit voltage of perovskite solar cells, Energy Environ. Sci., 2019, 12, 2778–2788 RSC
.
- I. Gelmetti, N. Montcada, A. Pérez-Rodríguez, E. Barrena, C. Ocal, I. García-Benito, A. Molina-Ontoria, N. Martín, A. Vidal-Ferran and E. Palomares, Energy Alignment and Recombination in Perovskite Solar Cells: Weighted Influence on the Open Circuit Voltage, Energy Environ. Sci., 2019, 12, 1309–1316 RSC
.
- Z. Hawash, L. K. Ono and Y. Qi, Recent Advances in Spiro-MeOTAD Hole Transport Material and Its Applications in Organic-Inorganic Halide Perovskite Solar Cells, Adv. Mater. Interfaces, 2018, 5, 1700623 CrossRef
.
- N. E. Courtier, J. M. Cave, J. M. Foster, A. B. Walker and G. Richardson, How transport layer properties affect perovskite solar cell performance: insights from a coupled charge transport/ion migration model, Energy Environ. Sci., 2019, 12, 396–409 RSC
.
- V. M. Le Corre, M. Stolterfoht, L. Perdigón Toro, M. Feuerstein, C. Wolff, L. Gil-Escrig, H. J. Bolink, D. Neher and L. J. A. Koster, Charge Transport Layers Limiting the Efficiency of Perovskite Solar Cells: How To Optimize Conductivity, Doping, and Thickness, ACS Appl. Energy Mater., 2019, 2, 6280–6287 CrossRef CAS
.
- T. Kirchartz, High open-circuit voltages in lead-halide perovskite solar cells: experiment,
theory and open questions, Philos. Trans. R. Soc., A, 2019, 377, 20180286 CrossRef
.
- R. J. E. Westbrook, Dr I. Sanchez-Molina, Dr J. Manuel Marin-Beloqui, Dr H. Bronstein and Dr S. A. Haque, Effect of Interfacial Energetics on Charge Transfer from Lead Halide Perovskite to Organic Hole Conductors, J. Phys. Chem. C, 2018, 122, 1326–1332 CrossRef CAS
.
- J. Wang, K. Liu, L. Ma and X. Zhan, Triarylamine: Versatile Platform for Organic, Dye-Sensitized, and Perovskite Solar Cells, Chem. Rev., 2016, 116, 14675–14725 CrossRef CAS
.
- V. M. Le Corre, M. Stolterfoht, L. Perdigón Toro, M. Feuerstein, C. Wolff, L. Gil-Escrig, H. J. Bolink, D. Neher and L. J. A. Koster, Charge Transport Layers Limiting the Efficiency of Perovskite Solar Cells: How To Optimize Conductivity, Doping, and Thickness, ACS Appl. Energy Mater., 2019, 2, 6280–6287 CrossRef CAS
.
- D. Głowienka, D. Zhang, F. Di Giacomo, M. Najafi, S. Veenstra, J. Szmytkowski and Y. Galagan, Role of surface recombination in perovskite solar cells at the interface of HTL/CH3NH3PbI3, Nano Energy, 2020, 67, 104186 CrossRef
.
- D. B. Khadka, Y. Shirai, M. Yanagida, J. W. Ryan and K. Miyano, Exploring the effects of interfacial carrier transport layers on device performance and optoelectronic properties of planar perovskite solar cells, J. Mater. Chem. C, 2017, 5, 8819–8827 RSC
.
- I. Grill, M. F. Aygüler, T. Bein, P. Docampo, N. F. Hartmann, M. Handloser and A. Hartschuh, Charge Transport Limitations in Perovskite Solar Cells: The Effect of Charge Extraction Layers, ACS Appl. Mater. Interfaces, 2017, 9, 37655–37661 CrossRef CAS PubMed
.
- M. Stolterfoht, C. M. Wolff, Y. Amir, A. Paulke, L. Perdigon, P. Caprioglio and D. Neher, Approaching the Fill Factor Shockley Queisser Limit in Stable, Dopant-Free Triple Cation Perovskite Solar Cells, Energy Environ. Sci., 2017, 10, 1530 RSC
.
- Y. Da, Y. Xuan and Q. Li, Quantifying energy losses in planar perovskite solar cells, Sol. Energy Mater. Sol. Cells, 2018, 174, 206–213 CrossRef CAS
.
- N.-G. Park, M. Grätzel, T. Miyasaka, K. Zhu and K. Emery, Towards stable and commercially available perovskite solar cells, Nat. Energy, 2016, 1, 16152 CrossRef CAS
.
- J. Yang, B. D. Siempelkamp, D. Liu and T. L. Kelly, Investigation of CH3NH3PbI3 Degradation Rates and Mechanisms in Controlled Humidity Environments Using in Situ Techniques, ACS Nano, 2015, 9, 1955–1963 CrossRef CAS
.
- S. Ryu, J. H. Noh, N. J. Jeon, Y. Chan Kim, W. S. Yang, J. Seo and S. I. Seok, Voltage output of efficient perovskite solar cells with high open-circuit voltage and fill factor, Energy Environ. Sci., 2014, 7, 2614–2618 RSC
.
- J. H. Heo, D. H. Song and S. H. Im, Planar CH3NH3 PbBr3 Hybrid Solar Cells with 10.4% Power Conversion Efficiency, Fabricated by Controlled Crystallization in the Spin-Coating Process, Adv. Mater., 2014, 26, 8179–8183 CrossRef CAS PubMed
.
- J. A. Christians, R. C. M. Fung and P. V. Kamat, An Inorganic Hole Conductor for Organo-Lead Halide Perovskite Solar Cells. Improved Hole Conductivity with Copper Iodide, J. Am. Chem. Soc., 2014, 136, 758–764 CrossRef CAS PubMed
.
- N. Arora, M. I. Dar, A. Hinderhofer, N. Pellet, F. Schreiber, S. M. Zakeeruddin and M. Grätzel, Perovskite solar cells with CuSCN hole extraction layers yield stabilized efficiencies greater than 20%, Science, 2017, 358, 768–771 CrossRef CAS PubMed
.
- F. E. Goodson, S. I. Hauck and J. F. Hartwig, Palladium-Catalyzed Synthesis of Pure, Regiodefined Polymeric Triarylamines, J. Am. Chem. Soc., 1999, 121, 7527–7539 CrossRef CAS
.
- J. Salbeck, N. Yu, J. Bauer, F. Weissörtel and H. Bestgen, Low molecular organic glasses for blue electroluminescence, Synth. Met., 1997, 91, 209–215 CrossRef CAS
.
- J. H. Noh, N. J. Jeon, Y. C. Choi, Md. K. Nazeeruddin, M. Grätzel and S. I. Seok, Nanostructured TiO2/CH3NH3PbI3 heterojunction solar cells employing spiro-OMeTAD/Co-complex as hole-transporting material, J. Mater. Chem. A, 2013, 1, 11842 RSC
.
- L. K. Ono, S. R. Raga, M. Remeika, A. J. Winchester, A. Gabe and Y. Qi, Pinhole-free hole transport layers significantly improve the stability of MAPbI 3 -based perovskite solar cells under operating conditions, J. Mater. Chem. A, 2015, 3, 15451–15456 RSC
.
- E. J. Juarez-Perez, M. R. Leyden, S. Wang, L. K. Ono, Z. Hawash and Y. Qi, Role of the Dopants on the Morphological and Transport Properties of Spiro-MeOTAD Hole Transport Layer, Chem. Mater., 2016, 28, 5702–5709 CrossRef CAS
.
- P. Schulz, E. Edri, S. Kirmayer, G. Hodes, D. Cahen and A. Kahn, Interface energetics in organo-metal halide perovskite-based photovoltaic cells, Energy Environ. Sci., 2014, 7, 1377 RSC
.
- T. Zhang, J. Wu, P. Zhang, W. Ahmad, Y. Wang, M. Alqahtani, H. Chen, C. Gao, Z. D. Chen, Z. Wang and S. Li, High Speed and Stable Solution-Processed Triple Cation Perovskite Photodetectors, Adv. Opt. Mater., 2018, 6, 1701341 CrossRef
.
- V. Coropceanu, J. Cornil, D. A. da Silva Filho, Y. Olivier, R. Silbey and J.-L. Brédas, Charge Transport in Organic Semiconductors, Chem. Rev., 2007, 107, 926–952 CrossRef CAS PubMed
.
- T. Malinauskas, D. Tomkute-Luksiene, R. Sens, M. Daskeviciene, R. Send, H. Wonneberger, V. Jankauskas, I. Bruder and V. Getautis, Enhancing Thermal Stability and Lifetime of Solid-State Dye-Sensitized Solar Cells via Molecular Engineering of the Hole-Transporting Material Spiro-OMeTAD, ACS Appl. Mater. Interfaces, 2015, 7, 11107–11116 CrossRef CAS
.
- D. Poplavskyy and J. Nelson, Nondispersive hole transport in amorphous films of methoxy-spirofluorene-arylamine organic compound, J. Appl. Phys., 2003, 93, 341–346 CrossRef CAS
.
- T. Leijtens, I.-K. Ding, T. Giovenzana, J. T. Bloking, M. D. McGehee and A. Sellinger, Hole Transport Materials with Low Glass Transition Temperatures and High Solubility for Application in Solid-State Dye-Sensitized Solar Cells, ACS Nano, 2012, 6, 1455–1462 CrossRef CAS
.
- H. J. Snaith and M. Grätzel, Enhanced charge mobility in a molecular hole transporter via addition of redox inactive ionic dopant: Implication to dye-sensitized solar cells, Appl. Phys. Lett., 2006, 89, 262114 CrossRef
.
- A. Abate, D. R. Staff, D. J. Hollman, H. J. Snaith and A. B. Walker, Influence of ionizing dopants on charge transport in organic semiconductors, Phys. Chem. Chem. Phys., 2014, 16, 1132–1138 RSC
.
- J. A. Röhr, X. Shi, S. A. Haque, T. Kirchartz and J. Nelson, Charge Transport in Spiro-OMeTAD Investigated through Space-Charge-Limited Current Measurements, Phys. Rev. Appl., 2018, 9, 044017 CrossRef
.
- W. H. Nguyen, C. D. Bailie, E. L. Unger and M. D. McGehee, Enhancing the Hole-Conductivity of Spiro-OMeTAD without Oxygen or Lithium Salts by Using Spiro(TFSI) 2 in Perovskite and Dye-Sensitized Solar Cells, J. Am. Chem. Soc., 2014, 136, 10996–11001 CrossRef CAS PubMed
.
- R. S. Sanchez and E. Mas-Marza, Light-induced effects on Spiro-OMeTAD films and hybrid lead halide perovskite solar cells, Sol. Energy Mater. Sol. Cells, 2016, 158, 189–194 CrossRef CAS
.
- G.-W. Kim, D. V. Shinde and T. Park, Thickness of the hole transport layer in perovskite solar cells: performance versus reproducibility, RSC Adv., 2015, 5, 99356–99360 RSC
.
- N. Marinova, W. Tress, R. Humphry-Baker, M. I. Dar, V. Bojinov, S. M. Zakeeruddin, M. K. Nazeeruddin and M. Grätzel, Light Harvesting and Charge Recombination in CH3NH3PbI3 Perovskite Solar Cells Studied by Hole Transport Layer Thickness Variation, ACS Nano, 2015, 9, 4200–4209 CrossRef CAS
.
- C. S. Ponseca, E. M. Hutter, P. Piatkowski, B. Cohen, T. Pascher, A. Douhal, A. Yartsev, V. Sundström and T. J. Savenije, Mechanism of Charge Transfer and Recombination Dynamics in Organo Metal Halide Perovskites and Organic Electrodes, PCBM, and Spiro-OMeTAD: Role of Dark Carriers, J. Am. Chem. Soc., 2015, 137, 16043–16048 CrossRef CAS PubMed
.
- D. Bi, L. Yang, G. Boschloo, A. Hagfeldt and E. M. J. Johansson, Effect of Different Hole Transport Materials on Recombination in CH3NH3PbI3 Perovskite-Sensitized Mesoscopic Solar Cells, J. Phys. Chem. Lett., 2013, 4, 1532–1536 CrossRef CAS
.
- A. Torres and L. G. C. Rego, Surface Effects and Adsorption of Methoxy Anchors on Hybrid Lead Iodide Perovskites: Insights for Spiro-MeOTAD Attachment, J. Phys. Chem. C, 2014, 118, 26947–26954 CrossRef CAS
.
- M. Pfeiffer, K. Leo, X. Zhou, J. S. Huang, M. Hofmann, A. Werner and J. Blochwitz-Nimoth, Doped organic semiconductors: Physics and application in light emitting diodes, Org. Electron., 2003, 4, 89–103 CrossRef CAS
.
- U. B. Cappel, T. Daeneke and U. Bach, Oxygen-Induced Doping of Spiro-MeOTAD in Solid-State Dye-Sensitized Solar Cells and Its Impact on Device Performance, Nano Lett., 2012, 12, 4925–4931 CrossRef CAS
.
- S. Fantacci, F. De Angelis, M. K. Nazeeruddin and M. Grätzel, Electronic and Optical Properties of the Spiro-MeOTAD Hole Conductor in Its Neutral and Oxidized Forms: A DFT/TDDFT Investigation, J. Phys. Chem. C, 2011, 115, 23126–23133 CrossRef CAS
.
- S. Wang, W. Yuan and Y. S. Meng, Spectrum-Dependent Spiro-OMeTAD Oxidization Mechanism in Perovskite Solar Cells, ACS Appl. Mater. Interfaces, 2015, 7, 24791–24798 CrossRef CAS
.
- T. H. Schloemer, J. A. Christians, J. M. Luther and A. Sellinger, Doping strategies for small molecule organic hole-transport materials: impacts on perovskite solar cell performance and stability, Chem. Sci., 2019, 10, 1904–1935 RSC
.
- H. J. Snaith and M. Grätzel, Enhanced charge mobility in a molecular hole transporter via addition of redox inactive ionic dopant: Implication to dye-sensitized solar cells, Appl. Phys. Lett., 2006, 89, 262114 CrossRef
.
- H. Min, M. Kim, S.-U. Lee, H. Kim, G. Kim, K. Choi, J. H. Lee and S. I. Seok, Efficient, stable solar cells by using inherent bandgap of a-phase formamidinium lead iodide, Science, 2019, 366, 749–753 CrossRef CAS PubMed
.
- M. Saliba, J.-P. Correa-Baena, C. M. Wolff, M. Stolterfoht, N. Phung, S. Albrecht, D. Neher and A. Abate, How to Make over 20% Efficient Perovskite Solar Cells in Regular (n–i–p) and Inverted (p–i–n) Architectures, Chem. Mater., 2018, 30, 4193–4201 CrossRef CAS
.
- R. Schölin, M. H. Karlsson, S. K. Eriksson, H. Siegbahn, E. M. J. Johansson and H. Rensmo, Energy Level Shifts in Spiro-OMeTAD Molecular Thin Films When Adding Li-TFSI, J. Phys. Chem. C, 2012, 116, 26300–26305 CrossRef
.
- A. Abate, T. Leijtens, S. Pathak, J. Teuscher, R. Avolio, M. E. Errico, J. Kirkpatrik, J. M. Ball, P. Docampo, I. McPherson and H. J. Snaith, Lithium salts as “redox active” p-type dopants for organic semiconductors and their impact in solid-state dye-sensitized solar cells, Phys. Chem. Chem. Phys., 2013, 15, 2572 RSC
.
- Z. Hawash, L. K. Ono, S. R. Raga, M. V. Lee and Y. Qi, Air-Exposure Induced Dopant Redistribution and Energy Level Shifts in Spin-Coated Spiro-MeOTAD Films, Chem. Mater., 2015, 27, 562–569 CrossRef CAS
.
- Z. Hawash, L. K. Ono and Y. Qi, Moisture and Oxygen Enhance Conductivity of LiTFSI-Doped Spiro-MeOTAD Hole Transport Layer in Perovskite Solar Cells, Adv. Mater. Interfaces, 2016, 3, 1600117 CrossRef
.
- Y. Cho, H. D. Kim, J. Zheng, J. Bing, Y. Li, M. Zhang, M. A. Green, A. Wakamiya, S. Huang, H. Ohkita and A. W. Y. Ho-Baillie, Elucidating Mechanisms behind Ambient Storage-Induced Efficiency Improvements in Perovskite Solar Cells, ACS Energy Lett., 2021, 6, 925–933 CrossRef CAS
.
- F. Lamberti, T. Gatti, E. Cescon, R. Sorrentino, A. Rizzo, E. Menna, G. Meneghesso, M. Meneghetti, A. Petrozza and L. Franco, Evidence of Spiro-OMeTAD De-doping by tert-Butylpyridine Additive in Hole-Transporting Layers for Perovskite Solar Cells, Chem, 2019, 5, 1806–1817 CAS
.
- S. Wang, M. Sina, P. Parikh, T. Uekert, B. Shahbazian, A. Devaraj and Y. S. Meng, Role of 4- tert-Butylpyridine as a Hole Transport Layer Morphological Controller in Perovskite Solar Cells, Nano Lett., 2016, 16, 5594–5600 CrossRef CAS
.
- J. Krüger, R. Plass, L. Cevey, M. Piccirelli, M. Grätzel and U. Bach, High efficiency solid-state photovoltaic device due to inhibition of interface charge recombination, Appl. Phys. Lett., 2001, 79, 2085–2087 CrossRef
.
- S. Wang, Z. Huang, X. Wang, Y. Li, M. Günther, S. Valenzuela, P. Parikh, A. Cabreros, W. Xiong and Y. S. Meng, Unveiling the Role of tBP–LiTFSI Complexes in Perovskite Solar Cells, J. Am. Chem. Soc., 2018, 140, 16720–16730 CrossRef CAS
.
- S. N. Habisreutinger, N. K. Noel, H. J. Snaith and R. J. Nicholas, Investigating the Role of 4-tert Butylpyridine in Perovskite Solar Cells, Adv. Energy Mater., 2017, 7, 1601079 CrossRef
.
- N. K. Noel, A. Abate, S. D. Stranks, E. S. Parrott, V. M. Burlakov, A. Goriely and H. J. Snaith, Enhanced Photoluminescence and Solar Cell Performance via Lewis Base Passivation of Organic–Inorganic Lead Halide Perovskites, ACS Nano, 2014, 8, 9815–9821 CrossRef CAS PubMed
.
- S. Wang, M. Sina, P. Parikh, T. Uekert, B. Shahbazian, A. Devaraj and Y. S. Meng, Role of 4- tert-Butylpyridine as a Hole Transport Layer Morphological Controller in Perovskite Solar Cells, Nano Lett., 2016, 16, 5594–5600 CrossRef CAS PubMed
.
- J. Burschka, F. Kessler, M. K. Nazeeruddin and M. Grätzel, Co(III) Complexes as p-Dopants in Solid-State Dye-Sensitized Solar Cells, Chem. Mater., 2013, 25, 2986–2990 CrossRef CAS
.
- L. Caliò, M. Salado, S. Kazim and S. Ahmad, A Generic Route of Hydrophobic Doping in Hole Transporting Material to Increase Longevity of Perovskite Solar Cells, Joule, 2018, 2, 1800–1815 CrossRef
.
- J. Zhang, Q. Daniel, T. Zhang, X. Wen, B. Xu, L. Sun, U. Bach and Y.-B. Cheng, Chemical Dopant Engineering in Hole Transport Layers for Efficient Perovskite Solar Cells: Insight into the Interfacial Recombination, ACS Nano, 2018, 12, 10452–10462 CrossRef CAS
.
- T. Leijtens, T. Giovenzana, S. N. Habisreutinger, J. S. Tinkham, N. K. Noel, B. A. Kamino, G. Sadoughi, A. Sellinger and H. J. Snaith, Hydrophobic Organic Hole Transporters for Improved Moisture Resistance in Metal Halide Perovskite Solar Cells, ACS Appl. Mater. Interfaces, 2016, 8, 5981–5989 CrossRef CAS PubMed
.
- J. Zhang, T. Zhang, L. Jiang, U. Bach and Y.-B. Cheng, 4-tert-Butylpyridine Free Hole Transport
Materials for Efficient Perovskite Solar Cells: A New Strategy to Enhance the Environmental and Thermal Stability, ACS Energy Lett., 2018, 3, 1677–1682 CrossRef CAS
.
- Y. Yue, N. Salim, Y. Wu, X. Yang, A. Islam, W. Chen, J. Liu, E. Bi, F. Xie, M. Cai and L. Han, Enhanced Stability of Perovskite Solar Cells through Corrosion-Free Pyridine Derivatives in Hole-Transporting Materials, Adv. Mater., 2016, 28, 10738–10743 CrossRef CAS PubMed
.
- J.-Y. Seo, H.-S. Kim, S. Akin, M. Stojanovic, E. Simon, M. Fleischer, A. Hagfeldt, S. M. Zakeeruddin and M. Grätzel, Novel p-dopant toward highly efficient and stable perovskite solar cells, Energy Environ. Sci., 2018, 11, 2985–2992 RSC
.
- X. Wang, J. Wu, Y. Yang, X. Liu, Q. Guo, Z. Song, G. Li, Z. Lan and M. Huang, High performance and stable perovskite solar cells using vanadic oxide as a dopant for spiro-OMeTAD, J. Mater. Chem. A, 2019, 7, 13256–13264 RSC
.
- L. Lanzetta, N. Aristidou and S. A. Haque, Stability of Lead and Tin Halide Perovskites: The Link between Defects and Degradation, J. Phys. Chem. Lett., 2020, 11, 574–585 CrossRef CAS PubMed
.
- E. Kasparavicius, A. Magomedov, T. Malinauskas and V. Getautis, Long-Term Stability of the Oxidized Hole-Transporting Materials used in Perovskite Solar Cells, Chem. – Eur. J., 2018, 24, 9910–9918 CrossRef CAS PubMed
.
- X. Zhao, H.-S. Kim, J.-Y. Seo and N.-G. Park, Effect of Selective Contacts on the Thermal Stability of Perovskite Solar Cells, ACS Appl. Mater. Interfaces, 2017, 9, 7148–7153 CrossRef CAS
.
- I. Mesquita, L. Andrade and A. Mendes, Temperature Impact on Perovskite Solar Cells Under Operation, ChemSusChem, 2019, 12, 2186–2194 CrossRef CAS PubMed
.
- C. D. Bailie, E. L. Unger, S. M. Zakeeruddin, M. Grätzel and M. D. McGehee, Melt-infiltration of spiro-OMeTAD and thermal instability of solid-state dye-sensitized solar cells, Phys. Chem. Chem. Phys., 2014, 16, 4864 RSC
.
- A. K. Jena, Y. Numate, M. Ikegami and T. Miyasaka, Role of Spiro-OMeTAD in Performance Deterioration of Perovskite Solar Cells at High Temperature and Reuse of the Perovskite Films to Avoid Pb-waste, J. Mater. Chem. A, 2018, 6, 2219–2230 RSC
.
- I. Lee, J. H. Yun, H. J. Son and T.-S. Kim, Accelerated Degradation Due to Weakened Adhesion from Li-TFSI Additives in Perovskite Solar Cells, ACS Appl. Mater. Interfaces, 2017, 9, 7029–7035 CrossRef CAS PubMed
.
- J. H. Yun, I. Lee, T.-S. Kim, M. J. Ko, J. Y. Kim and H. J. Son, Synergistic enhancement and mechanism study of mechanical and moisture stability of perovskite solar cells introducing polyethylene -imine into CH3NH3PbI3/HTM interface, J. Mater. Chem. A, 2015, 3, 22176–22182 RSC
.
- D. Wei, T. Wang, J. Ji, M. Li, P. Cui, Y. Li, G. Li, J. M. Mbengue and D. Song, Photo-induced degradation of lead halide perovskite solar cells caused by the hole transport layer/metal electrode interface, J. Mater. Chem. A, 2016, 4, 1991–1998 RSC
.
- K. Domanski, J.-P. Correa-Baena, N. Mine, M. K. Nazeeruddin, A. Abate, M. Saliba, W. Tress, A. Hagfeldt and M. Grätzel, Not All That Glitters Is Gold: Metal-Migration-Induced Degradation in Perovskite Solar Cells, ACS Nano, 2016, 10, 6306–6314 CrossRef CAS PubMed
.
- S. Guarnera, A. Abate, W. Zhang, J. M. Foster, G. Richardson, A. Petrozza and H. J. Snaith, Improving the Long-Term Stability of Perovskite Solar Cells with a Porous Al 2 O 3 Buffer Layer, J. Phys. Chem. Lett., 2015, 6, 432–437 CrossRef CAS
.
- C.-T. Lin, J. Ngiam, B. Xu, Y.-H. Chang, T. Du, T. J. Macdonald, J. R. Durrant and M. A. McLachlan, Enhancing the operational stability of unencapsulated perovskite solar cells through Cu–Ag bilayer electrode incorporation, J. Mater. Chem. A, 2020, 8, 8684–8691 RSC
.
- Z. Li, C. Xiao, Y. Yang, S. P. Harvey, D. H. Kim, J. A. Christians, M. Yang, P. Schulz, S. U. Nanayakkara, C.-S. Jiang, J. M. Luther, J. J. Berry, M. C. Beard, M. M. Al-Jassim and K. Zhu, Extrinsic ion migration in perovskite solar cells, Energy Environ. Sci., 2017, 10, 1234–1242 RSC
.
- J. A. Dawson, A. J. Naylor, C. Eames, M. Roberts, W. Zhang, H. J. Snaith, P. G. Bruce and M. S. Islam, Mechanisms of Lithium Intercalation and Conversion Processes in Organic–Inorganic Halide Perovskites, ACS Energy Lett., 2017, 2, 1818–1824 CrossRef CAS
.
- W. H. Howie, J. E. Harris, J. R. Jennings and L. M. Peter, Solid-state dye-sensitized solar cells based on spiro-MeOTAD, Sol. Energy Mater. Sol. Cells, 2007, 91, 424–426 CrossRef CAS
.
- W. Li, H. Dong, L. Wang, N. Li, X. Guo, J. Li and Y. Qiu, Montmorillonite as bifunctional buffer layer material for hybrid perovskite solar cells with protection from corrosion and retarding recombination, J. Mater. Chem. A, 2014, 2, 13587–13592 RSC
.
- H. Sarvari, X. Wang, Y. Wang, P. Zhang, S. Li, V. P. Singh and Z. Chen, Photovoltaic Performance of Lead-Iodide Perovskite Solar Cells Fabricated Under Ambient Air Conditions With HTM Solution Excluding LiTFSI, IEEE J. Photovolt., 2018, 8, 1051–1057 Search PubMed
.
- Y. Zhang, A. Kirs, F. Ambroz, C. Lin, A. S. R. Bati, I. P. Parkin, J. G. Shapter, M. Batmunkh and T. J. Macdonald, Ambient Fabrication of Organic–Inorganic Hybrid Perovskite Solar Cells, Small Methods, 2021, 5, 2000744 CrossRef CAS
.
- J. Kim, N. Park, J. S. Yun, S. Huang, M. A. Green and A. W. Y. Ho-Baillie, An effective method of predicting perovskite solar cell lifetime–Case study on planar CH3NH3PbI3 and HC(NH 2) 2PbI3 perovskite solar cells and hole transfer materials of spiro-OMeTAD and PTAA, Sol. Energy Mater. Sol. Cells, 2017, 162, 41–46 CrossRef CAS
.
- Q. Wang, Influence of Cobalt Additive in spiro-OMeTAD on Charge Recombination and Carrier Density in Perovskite Solar Cells investigated by Impedance Spectroscopy, Phys. Chem. Chem. Phys., 2018, 20, 10114–10120 RSC
.
- J. Veres, S. Ogier, S. Leeming, B. Brown and D. Cupertino, Air Stable, Amorphous Organic Films and their Applications to Solution Processable Flexible Electronics, MRS Proc., 2001, 708, BB8.7 CrossRef
.
- J. Veres, S. D. Ogier, S. W. Leeming, D. C. Cupertino and S. Mohialdin, Khaffaf, Low-k Insulators as the Choice of Dielectrics in Organic Field-Effect Transistors, Adv. Funct. Mater., 2003, 13, 199–204 CrossRef CAS
.
- L. A. Majewski, M. Grell, S. D. Ogier and J. Veres, A novel gate insulator for flexible electronics, Org. Electron., 2003, 4, 27–32 CrossRef CAS
.
- J. H. Noh, S. H. Im, J. H. Heo, T. N. Mandal and S. I. Seok, Chemical Management for Colorful, Efficient, and Stable Inorganic–Organic Hybrid Nanostructured Solar Cells, Nano Lett., 2013, 13, 1764–1769 CrossRef CAS PubMed
.
- W. S. Yang, J. H. Noh, N. J. Jeon, Y. C. Kim, S. Ryu, J. Seo and S. I. Seok, High-performance photovoltaic perovskite layers fabricated through intramolecular exchange, Science, 2015, 348, 1234–1237 CrossRef CAS PubMed
.
-
B. Souharce, PhD thesis, Bergische Universität
Wuppertal, 2008.
- Y. Ko, Y. Kim, C. Lee, Y. Kim and Y. Jun, Investigation of Hole-Transporting Poly(triarylamine) on Aggregation and Charge Transport for Hysteresisless Scalable Planar Perovskite Solar Cells, ACS Appl. Mater. Interfaces, 2018, 10, 11633–11641 CrossRef CAS PubMed
.
- S. Barard, M. Heeney, L. Chen, M. Cölle, M. Shkunov, I. McCulloch, N. Stingelin, M. Philips and T. Kreouzis, Separate charge transport pathways determined by the time of flight method in bimodal polytriarylamine, J. Appl. Phys., 2009, 105, 013701 CrossRef
.
- W. Zhang, J. Smith, R. Hamilton, M. Heeney, J. Kirkpatrick, K. Song, S. E. Watkins, T. Anthopoulos and I. McCulloch, Systematic Improvement in Charge Carrier Mobility of Air Stable Triarylamine Copolymers, J. Am. Chem. Soc., 2009, 131, 10814–10815 CrossRef CAS PubMed
.
- T. Ye, W. Chen, S. Jin, S. Hao, X. Zhang, H. Liu and D. He, Enhanced Efficiency of Planar Heterojunction Perovskite Solar Cells by a Light Soaking Treatment on Tris(pentafluorophenyl)borane-Doped Poly(triarylamine) Solution, ACS Appl. Mater. Interfaces, 2019, 11, 14004–14010 CrossRef CAS
.
- J. Endres, M. Kulbak, L. Zhao, B. P. Rand, D. Cahen, G. Hodes and A. Kahn, Electronic structure of the CsPbBr 3/polytriarylamine (PTAA) system, J. Appl. Phys., 2017, 121, 035304 CrossRef
.
- J. C. Brauer, Y. H. Lee, M. K. Nazeeruddin and N. Banerji, Charge Transfer Dynamics from Organometal Halide Perovskite to Polymeric Hole Transport Materials in Hybrid Solar Cells, J. Phys. Chem. Lett., 2015, 6, 3675–3681 CrossRef CAS PubMed
.
- I. Lee, N. Rolston, P.-L. Brunner and R. H. Dauskardt, Hole-Transport Layer Molecular Weight and Doping Effects on Perovskite Solar Cell Efficiency and Mechanical Behavior, ACS Appl. Mater. Interfaces, 2019, 11, 23757–23764 CrossRef CAS
.
- Y. Kim, E. H. Jung, G. Kim, D. Kim, B. J. Kim and J. Seo, Sequentially Fluorinated PTAA Polymers for Enhancing VOC of High-Performance Perovskite Solar Cells, Adv. Energy Mater., 2018, 8, 1801668 CrossRef
.
- P. Zalar, M. Kuik, Z. B. Henson, C. Woellner, Y. Zhang, A. Sharenko, G. C. Bazan and T.-Q. Nguyen, Increased Mobility Induced by Addition of a Lewis Acid to a Lewis Basic Conjugated Polymer, Adv. Mater., 2014, 26, 724–727 CrossRef CAS PubMed
.
- H. Méndez, G. Heimel, A. Opitz, K. Sauer, P. Barkowski, M. Oehzelt, J. Soeda, T. Okamoto, J. Takeya, J.-B. Arlin, J.-Y. Balandier, Y. Geerts, N. Koch and I. Salzmann, Doping of Organic Semiconductors: Impact of Dopant Strength and Electronic Coupling, Angew. Chem., Int. Ed., 2013, 52, 7751–7755 CrossRef PubMed
.
- J. H. Heo, H. J. Han, D. Kim, T. K. Ahn and S. H. Im, Hysteresis-less inverted CH3NH3PbI3 planar perovskite hybrid solar cells with 18.1% power conversion efficiency, Energy Environ. Sci., 2015, 8, 1602–1608 RSC
.
- R. Noriega, J. Rivnay, K. Vandewal, F. P. V. Koch, N. Stingelin, P. Smith, M. F. Toney and A. Salleo, A general relationship between disorder, aggregation and charge transport in conjugated polymers, Nat. Mater., 2013, 12, 1038–1044 CrossRef CAS PubMed
.
- J. Luo, J. Xia, H. Yang, L. Chen, Z. Wan, F. Han, H. A. Malik, X. Zhu and C. Jia, Toward high-efficiency, hysteresis-less, stable perovskite solar cells: unusual doping of a hole-transporting material using a fluorine-containing hydrophobic Lewis acid, Energy Environ. Sci., 2018, 11, 2035–2045 RSC
.
- B. L. Watson, N. Rolston, K. A. Bush, L. Taleghani and R. H. Dauskardt, Synthesis and use of a hyper-connecting cross-linking agent in the hole-transporting layer of perovskite solar cells, J. Mater. Chem. A, 2017, 5, 19267–19279 RSC
.
- N. Y. Nia, M. Méndez, B. Paci, A. Generosi, A. Di Carlo and E. Palomares, Analysis of the Efficiency Losses in Hybrid Perovskite/PTAA Solar Cells with Different Molecular Weights: Morphology versus Kinetics, ACS Appl. Energy Mater., 2020, 3, 6853–6859 CrossRef
.
- N. Y. Nia, M. Méndez, A. di Carlo and E. Palomares, Energetic disorder in perovskite/polymer solar cells and its relationship with the interfacial carrier losses, Philos. Trans. R. Soc., A, 2019, 377, 20180315 CrossRef CAS
.
- R. Wang, J. Xue, L. Meng, J.-W. Lee, Z. Zhao, P. Sun, L. Cai, T. Huang, Z. Wang, Z.-K. Wang, Y. Duan, J. L. Yang, S. Tan, Y. Yuan, Y. Huang and Y. Yang, Caffeine Improves the Performance and Thermal Stability of Perovskite Solar Cells, Joule, 2019, 3, 1464–1477 CrossRef CAS
.
- L. Meng, C. Sun, R. Wang, W. Huang, Z. Zhao, P. Sun, T. Huang, J. Xue, J.-W. Lee, C. Zhu, Y. Huang, Y. Li and Y. Yang, Tailored Phase Conversion under Conjugated Polymer Enables Thermally Stable Perovskite Solar Cells with Efficiency Exceeding 21%, J. Am. Chem. Soc., 2018, 140, 17255–17262 CrossRef CAS PubMed
.
- M. Maitrot, G. Guillaud, B. Boudjema, J. J. André and J. Simon, Molecular material-based junctions: Formation of a Schottky contact with metallophthalocyanine thin films doped by the cosublimation method, J. Appl. Phys., 1986, 60, 2396–2400 CrossRef CAS
.
- Q. Wang, C. Bi and J. Huang, Doped hole transport layer for efficiency enhancement in planar heterojunction organolead trihalide perovskite solar cells, Nano Energy, 2015, 15, 275–280 CrossRef CAS
.
- W. Yang, D. Zhong, M. Shi, S. Qu and H. Chen, Toward Highly Thermal Stable Perovskite Solar Cells by Rational Design of Interfacial Layer, iScience, 2019, 22, 534–543 CrossRef CAS PubMed
.
- S. N. Habisreutinger, T. Leijtens, G. E. Eperon, S. D. Stranks, R. J. Nicholas and H. J. Snaith, Carbon Nanotube/Polymer Composites as a Highly Stable Hole Collection Layer in Perovskite Solar Cells, Nano Lett., 2014, 14, 5561–5568 CrossRef CAS
.
- D. Bi, G. Boschloo and A. Hagfeldt, High-efficiency solid-state perovskite solar cell without lithium salt in the hole transport material, NANO, 2014, 09, 1440001 CrossRef
.
-
T. Duong, Y. Wu, H. Shen, J. Peng, N. Wu, T. White, K. Weber and K. Catchpole, in 2018 IEEE 7th World Conference on Photovoltaic Energy Conversion (WCPEC) (A Joint Conference of 45th IEEE PVSC, 28th PVSEC & 34th EU PVSEC), IEEE, Waikoloa Village, HI, 2018, pp. 3506–3508.
- H. K. H. Lee, Z. Li, I. Constantinou, F. So, S. W. Tsang and S. K. So, Batch-to-Batch Variation of Polymeric Photovoltaic Materials: its Origin and Impacts on Charge Carrier Transport and Device Performances, Adv. Energy Mater., 2014, 4, 1400768 CrossRef
.
- J. A. Christians, P. Schulz, J. S. Tinkham, T. H. Schloemer, S. P. Harvey, B. J. Tremolet de Villers, A. Sellinger, J. J. Berry and J. M. Luther, Tailored interfaces of unencapsulated perovskite solar cells for >1000 hour operational stability, Nat. Energy, 2018, 3, 68–74 CrossRef CAS
.
- T. Ye, J. Wang, W. Chen, Y. Yang and D. He, Improved Performance and Reproducibility of Perovskite Solar Cells by Well-Soluble Tris(pentafluorophenyl)borane as a p-Type Dopant, ACS Appl. Mater. Interfaces, 2017, 9, 17923–17931 CrossRef CAS PubMed
.
- J. Luo, C. Jia, Z. Wan, F. Han, B. Zhao and R. Wang, The novel dopant for hole-transporting material opens a new processing route to efficiently reduce hysteresis and improve stability of planar perovskite solar cells, J. Power Sources, 2017, 342, 886–895 CrossRef CAS
.
- S. Moghadamzadeh, I. M. Hossain, M. Jakoby, B. Abdollahi Nejand, D. Rueda-Delgado, J. A. Schwenzer, S. Gharibzadeh, T. Abzieher, M. R. Khan, A. A. Haghighirad, I. A. Howard, B. S. Richards, U. Lemmer and U. W. Paetzold, Spontaneous enhancement of the stable power conversion efficiency in perovskite solar cells, J. Mater. Chem. A, 2020, 8, 670–682 RSC
.
- N. J. Jeon, H. G. Lee, Y. C. Kim, J. Seo, J. H. Noh, J. Lee and S. I. Seok,
o-Methoxy Substituents in Spiro-OMeTAD for Efficient Inorganic–Organic Hybrid Perovskite Solar Cells, J. Am. Chem. Soc., 2014, 136, 7837–7840 CrossRef CAS PubMed
.
- E. H. Jung, N. J. Jeon, E. Y. Park, C. S. Moon, T. J. Shin, T.-Y. Yang, J. H. Noh and J. Seo, Efficient, stable and scalable perovskite solar cells using poly(3-hexylthiophene), Nature, 2019, 567, 511–515 CrossRef CAS PubMed
.
- J. Urieta-Mora, I. García-Benito, A. Molina-Ontoria and N. Martín, Hole transporting materials for perovskite solar cells: a chemical approach, Chem. Soc. Rev., 2018, 47, 8541–8571 RSC
.
- M. Monteiro Lunardi, A. Wing Yi Ho-Baillie, J. P. Alvarez-Gaitan, S. Moore and R. Corkish, A life cycle assessment of perovskite/silicon tandem solar cells: Perovskite/silicon tandem solar cell LCA, Prog. Photovoltaics, 2017, 25, 679–695 CAS
.
- J. Urieta-Mora, I. García-Benito, A. Molina-Ontoria and N. Martín, Hole transporting materials for perovskite solar cells: a chemical approach, Chem. Soc. Rev., 2018, 47, 8541–8571 RSC
.
- G. Kim, H. Choi, M. Kim, J. Lee, S. Y. Son and T. Park, Hole Transport Materials in Conventional Structural (n–i–p) Perovskite Solar Cells: From Past to the Future, Adv. Energy Mater., 2020, 10, 1903403 CrossRef CAS
.
- H. Zhang, Y. Wu, W. Zhang, E. Li, C. Shen, H. Jiang, H. Tian and W.-H. Zhu, Low cost and stable quinoxaline-based hole-transporting materials with a D–A–D molecular configuration for efficient perovskite solar cells, Chem. Sci., 2018, 9, 5919–5928 RSC
.
- L. Zhang, C. Liu, X. Wang, Y. Tian, A. K. Y. Jen and B. Xu, Side-Chain Engineering on Dopant-Free Hole-Transporting Polymers toward Highly Efficient Perovskite Solar Cells (20.19%), Adv. Funct. Mater., 2019, 29, 1904856 CrossRef
.
- L. Wang, F. Zhang, T. Liu, W. Zhang, Y. Li, B. Cai, L. He, Y. Guo, X. Yang, B. Xu, J. M. Gardner, L. Kloo and L. Sun, A crosslinked polymer as dopant-free hole-transport material for efficient n–i–p type perovskite solar cells, J. Energy Chem., 2021, 55, 211–218 CrossRef
.
- H. Hwang, S. Park, J. H. Heo, W. Kim, H. Ahn, T.-S. Kim, S. H. Im and H. J. Son, Enhancing performance and stability of perovskite solar cells using hole transport layer of small molecule and conjugated polymer blend, J. Power Sources, 2019, 418, 167–175 CrossRef CAS
.
- R. Li, P. Wang, B. Chen, X. Cui, Y. Ding, Y. Li, D. Zhang, Y. Zhao and X. Zhang, NiOx/Spiro Hole Transport Bilayers for
Stable Perovskite Solar Cells with Efficiency Exceeding 21%, ACS Energy Lett., 2020, 5, 79–86 CrossRef CAS
.
- S. N. Habisreutinger, N. K. Noel, B. W. Larson, O. G. Reid and J. L. Blackburn, Rapid Charge-Transfer Cascade through SWCNT Composites Enabling Low-Voltage Losses for Perovskite Solar Cells, ACS Energy Lett., 2019, 4, 1872–1879 CrossRef CAS
.
- T. Duong, J. Peng, D. Walter, J. Xiang, H. Shen, D. Chugh, M. Lockrey, D. Zhong, J. Li, K. Weber, T. P. White and K. R. Catchpole, Perovskite Solar Cells Employing Copper Phthalocyanine Hole-Transport Material with an Efficiency over 20% and Excellent Thermal Stability, ACS Energy Lett., 2018, 3, 2441–2448 CrossRef CAS
.
- A. Magomedov, A. Al-Ashouri, E. Kasparavičius, S. Strazdaite, G. Niaura, M. Jošt, T. Malinauskas, S. Albrecht and V. Getautis, Self-Assembled Hole Transporting Monolayer for Highly Efficient Perovskite Solar Cells, Adv. Energy Mater., 2018, 8, 1801892 CrossRef
.
- R. J. E. Westbrook, T. J. Macdonald, W. Xu, L. Lanzetta, J. M. Marin-Beloqui, T. M. Clarke and S. A. Haque, Lewis Base Passivation Mediates Charge Transfer at Perovskite Heterojunctions, J. Am. Chem. Soc., 2021, 143, 12230–12243 CrossRef CAS
.
|
This journal is © The Royal Society of Chemistry 2021 |
Click here to see how this site uses Cookies. View our privacy policy here.