DOI:
10.1039/C4RA15152F
(Paper)
RSC Adv., 2015,
5, 17405-17412
Ruthenium complexes as inhibitors of human islet amyloid polypeptide aggregation, an effect that prevents beta cell apoptosis†
Received
25th December 2014
, Accepted 19th January 2015
First published on 20th January 2015
Abstract
Human islet amyloid polypeptide (hIAPP) aggregation is essential in the loss of insulin-producing pancreatic beta cells in type 2 diabetes mellitus (T2DM). Recent studies have identified hIAPP fibril as a therapeutic target of T2DM. Metal complexes could covalently bind to the intracellular peptides to regulate their biological functions. In the present study, ruthenium (Ru) complexes NAMI-A (1) [Ru(bpy)3](ClO4)2 (2) (bpy = 2,2′-dipyridyl), [Ru(pip)3](ClO4)2 (3) (pip = 2-phenylimidazo[4,5-f]-[1,10]phenanthroline) and [Ru(phtpy)(phen)Cl]ClO4 (4) (phtpy = 2,6-bis(2-pyridyl)-4-phenylpyridine, phen = 1,10-phenanthroline) were selected to investigate their influence on hIAPP fibrillation in vitro. The results of thioflavin T (ThT) fluorescence assay showed that Ru complexes effectively inhibited the formation of hIAPP fibril. AFM images and TEM images further validated that the hIAPP fibrillation was disaggregated by the Ru complexes and then formed nanoscale particles, which tends to be a time-dependent process. Moreover, Ru complexes demonstrated a protective effect towards hIAPP-caused cell damage by restraining ROS generation and blocking cell apoptosis. In addition, it has been found that Ru complexes can also disaggregate hIAPP fibrils effectively inside the cells, and that the effects were proportional to the lipophilicity of the Ru complexes. Taken together, this study provides a strategy for designing Ru complexes for treating T2DM by targeting hIAPP.
Introduction
Cancer amyloid-related diseases, such as Alzheimer's disease (AD) with amyloid-β peptide (Aβ), spongiform encephalopathies with prion protein (PrP) and type 2 diabetes mellitus (T2DM) with islet amyloid polypeptide (IAPP), as well as other degenerative diseases, are characterized by protein conformational change and amyloid fibril formation.1–3 Human islet amyloid polypeptide (hIAPP, also known as human amylin) is a 37-amino-acid peptide synthesized in the pancreatic beta cells and co-secreted along with insulin in response to beta cell secretagogues, which has been demonstrated to easily aggregate and form amyloid fibril deposits, inducing the dysfunction and death of pancreatic β-cells in the pathology of T2DM.4–8 Whereas, the misfolding and amyloid fibril formation of hIAPP, which undergo the conformational transition of a random coil into the β-sheet, are cytotoxic in a disease state.9,10 Evidence has suggested that the toxicity of hIAPP fibril formation is very likely to be correlated with membrane disruption.11,12 The oxidative stress and ion-permeable channels are thought to be the toxicity mechanisms,13–15 but this argument is controversial. Thus, the exact mechanism of toxicity induced by hIAPP aggregation remains elusive.
Considering the toxicity of hIAPP aggregates, a variety of inhibitors towards hIAPP aggregation, such as peptide-based inhibitors, coordination compounds and small molecule inhibitors,16–18 is developed. It is reported that several metal ions could effectively inhibit hIAPP fibril formation.19 Ward et al. discovered Cu(II) prevents hIAPP from forming the β-sheet conformers through the destabilisation of the intramolecular disulphide bridge, Al(III) and Zn(II) significantly increase the formation of hIAPP fibrils, and Fe(III) appears to have the least influence upon the hIAPP aggregation.20 In addition, the metal complexes that could covalently bind to the corresponding peptide would be potential drugs for inhibiting amyloid fibril formation.21 Lee et al. designed a Co(III) complex as peptide-cleavage agent to cleave the oligomers of hIAPP and inhibit the apoptosis of INS-1 cells induced by hIAPP even in the presence of polymeric aggregate of hIAPP.22 Similarly, a cleavage agent of Cu(II) cyclen obtained an estimated 8.3 mol% cleavage yield of β-sheet conformers.17 Vyas et al. synthesized two ruthenium (Ru) polypyridyl complexes that could efficiently inhibit Aβ (1–40) aggregation and were non-toxic to human neuroblastoma cells, whose ancillary ligand plays a significant role in the Aβ inhibitory potency.23 Since Aβ and hIAPP are both inclined to form fibrous deposition, Ru complexes were also found to inhibit amylin from forming β-sheets and promote the disaggregation of the amyloidogenesis by remarkably changing the β-sheet components.24 However, little information about the interaction between Ru complexes and hIAPP is available.
Ru complexes offer several advantages such as feasible synthesis, inertness to substitution, stability in biological environments, expansions of functional groups by variation in surrounding ligands and inherent photophysical properties.25,26 Until now, several Ru complexes have been proposed as anti-cancer drug leads. However, studies also reported the toxicity of Ru complexes against different cell lines.27,28 Therefore, the rational design of biologically active Ru complexes with low toxicity and hIAPP-inhibitory effects has kindled great interest of scientists from the fields of chemistry and medicine. In the present study, a series of Ru complexes, including NAMI-A (1) [Ru(bpy)3](ClO4)2 (2), [Ru(pip)3](ClO4)2 (3) and [Ru(phtpy)(phen)Cl]ClO4 (4) were synthesized and selected to investigate their interaction with hIAPP. The results of both extra-cellular and in vitro cell studies provided strong evidence of the inhibition of Ru complexes on the amyloid fibrillation of hIAPP, and thus prevent beta cell apoptosis, which demonstrates that Ru complexes could bind hIAPP and alter its physicochemical properties to inhibit cellular toxicity. Taken together, this study provides a strategy for designing Ru complexes for treating T2DM by targeting hIAPP.
Experimental sections
Materials and chemicals
Human islet amyloid polypeptide (hIAPP), RuC13·3H2O, NaClO4, ligands 2,2′-bipyridine (bpy), 2-phenylimidazo[4,5-f][1,10]phenanthroline (pip), 2,6-bis(2-pyridyl)-4-phenylpyridine (phtpy) and 1,10-phenanthroline (phen) were purchased commercially and used without further purification. Thioflavin T (ThT) was purchased from Shanghai Godo Industrial Co., Ltd. H2DCF-DA, dihydroethidium (DHE), bicinchoninic acid (BCA) protein assay kit, substrates for caspase-3 (Ac-DEVD-AMC), caspase-8 (Ac-IETD-AMC) and caspase-9 (Ac-LEHD-AMC) were purchased from Sigma-Aldrich.
Synthesis of the Ru complexes
In the present study, complexes 1, 2, 3 and 4 were synthesized and characterized as previously reported with slight modification.28–30
Tyrosine intrinsic fluorescence assay
10 μM hIAPP solution was incubated alone or in the presence of 5 μM complexes 1, 2, 3 and 4 for 48 h at 37 °C. Two groups were carried out, one group was incubated in normal room light and the other group was incubated in a dark room. Different concentrations of complex 3 were incubated 10 μM hIAPP for 48 h at 37 °C. Samples were placed in a four-sided quartz fluorescence cuvette and data were recorded at indicated time. Fluorescence spectra were collected using an F-4500 FL spectrophotometer (Hitachi, Japan). Ex = 280 nm (slit width = 5 nm) and emission (slit width = 10 nm) were monitored over the range of 290–400 nm.
Thioflavin T (ThT) fluorescence assay
10 μM hIAPP was incubated with or without 5 μM complexes 1, 2, 3 and 4 solution in PBS buffer (phosphate buffered saline, containing NaCl 137 mM, KCl 2.7 mM, Na2HPO4 10 mM, KH2PO4 2 mM, pH 7.4) for 48 h at 37 °C. 40 μl incubated solution and 160 μl ThT (50 μM) were added to a 96-well black microplate. Fluorescence measurements were observed using a spectrofluorometer (Spectra Max M5, Bio-Tek) at 37 °C. 440 nm and 482 nm were set as the excitation and emission wavelengths, respectively.
Particle size analysis
The particle size was measured by the Zetasizer Nano ZS particle analyzer (Malvern Instruments Limited).31 Briefly, 10 μM hIAPP in the absence and presence of 5 μM complexes 1, 2, 3 and 4 solution was incubated in PBS for 48 h at 37 °C. 1 ml mixed solution was extracted for measurement.
Particle size analysis by AFM and TEM
hIAPP samples were characterized by AFM and TEM.28 In brief, 10 μM hIAPP was incubated with or without 5 μM complexes 1, 2, 3 and 4 solution for 48 h at 37 °C. 10 μl incubated solution was obtained by a Bioscope Catalyst Nanoscope-V (Veeco Instruments, USA) to get ATM images. The TEM images were visualized using a Hitachi (H-7650) transmission electron microscope operating at 80 kV.
Cell culture and MTT assay
INS-1 rat insulinoma cell line was purchased from American Type Culture Collection (ATCC, Manassas, VA, USA). The cells were incubated in RPMI-1640 medium supplemented with 10% FBS, 10 mmol l−1 HEPES, 50 mmol l−1 mercaptoethanol, 2 mmol l−1 l-glutamine, 1 mmol l−1 sodium pyruvate, 100 U per ml penicillin and 100 mg ml−1 streptomycin at 37 °C in a CO2 incubator (95% relative humidity, 5% CO2). Cell viability was measured using an MTT assay as described previously.32,33 6 × 104 INS-1 cells per well were seeded in 96-well tissue culture plates at 37 °C for 24 h. Different concentrations of hIAPP and Ru complexes were added for another 48 h. Then, MTT solution was added to each well and incubated at 37 °C for 4 h. The absorbance of the cells at 570 nm was determined by a microplate reader (Spectra Max M5). All the experiments were carried out at least three times, and the data are presented as averages of three independent experiments, mean ± standard deviations.
Caspase activity assay
Caspase activity was detected through fluorescence intensity using a specific caspase-3, -8, and -9 substrates. Briefly, after treatment with 20 μM hIAPP alone or with 5 μM Ru complexes, the cell pellets were harvested and then suspended in cell lysis buffer for 2 h. Then the cell proteins were obtained after centrifugation at 12
000g for 20 min. After that, the cell lysates were placed in 96-well plates with caspase-3, -8, and -9 substrates to determine the fluorescence intensity with a microplate reader (Spectra Max M5, Bio-Tek) with ex/em wavelengths set at 380/460 nm.
Measurement of ROS generation
Intracellular ROS accumulation was evaluated by an H2DCF-DA assay and DHE assay according to an existing protocol.34,35 Briefly, INS-1 cells at a density of 1 × 106 cells per ml were seeded in 96-well plates, hIAPP (10 μM) with or without different Ru complexes (5 μM) was added, and were incubated for 60 min. Subsequently, the cells were incubated with H2DCF-DA (10 μM) or DHE (10 μM) at 37 °C for 30 min. The ROS level was then examined by fluorescence intensity using a microplate reader (Spectra Max M5) with ex/em wavelengths set at 488/525 nm for DCF and 300/600 nm for the DHE probe.
Detection of intracellular hIAPP aggregation
The intracellular hIAPP aggregate detection was performed inside INS-1 cells. Briefly, cells were grown on cover glasses in 6-well plates pretreated with 10 μM hIAPP and 5 μM Ru complexes for 48 h at room temperature. Subsequently, 4.0% paraformaldehyde was added, followed by incubation for 10 min at 37 °C. Then the cells were cultured with 50 μM ThT and DAPI (1 μg mg−1) for 30 min, washed twice with PBS and observed under a fluorescence microscope (Axiophot, Zeiss).
Measurement of lipophilicity of Ru complexes
The lipophilicity of the Ru complexes was measured using the “shake-flask” method as previously described.36 The concentrations of the Ru complexes in each phase were determined by UV-Vis analysis. The results were calculated by log
P = lg([solute]octanol/[solute]water).
Interaction between hIAPP and Ru complexes
Briefly, 10 μM of hIAPP was incubated with 5 μM of Ru complex 3 for 48 h at 37 °C. Subsequently, the mass spectra were obtained on an ABI4000 Q TRAP liquid chromatography-mass spectrometer (ABI, USA).
Statistics analysis
All experiments were carried out at least in triplicate, and the data are expressed as mean ± standard deviation. Differences between the control and the experimental groups were analyzed by two-tailed Student's t test. The one-way analysis of variance (ANOVA) was used in multiple group comparisons. Statistical analysis was performed using the SPSS statistical program version 13 (SPSS Inc., Chicago, IL). Significant difference between treatment and control groups is indicated at * P < 0.05, ** P < 0.01 level.
Results and discussion
Ru complexes inhibit hIAPP fibrillation in vitro
As Ru polypyridyl complexes could efficiently inhibit Aβ (1–40) aggregation and demonstrated negligible toxicity to human neuroblastoma cells,23 Ru complexes may show several advantages in inhibiting amyloid fibril formation. Herein, we selected different Ru complexes 1, 2, 3 and 4 to investigate their effect on the fibrillation of hIAPP (Fig. 1). The change in tyrosine fluorescence intensity was used widely to examine the interaction between the polypeptide and the Ru complexes. The tyrosine fluorescence of hIAPP would be quenched when a complex binds to the tyrosine. Many studies have examined the change in the tyrosine fluorescence intensity to confirm the interaction between hIAPP and Cu(II).20,37,38 Therefore, in this study, the tyrosine intrinsic fluorescence assay was chosen to detect the interaction between Ru complexes and hIAPP. As a result, after hIAPP co-incubated with complexes 1, 2, 3 and 4, the tyrosine intrinsic fluorescence was quenched in the darkroom group (Fig. S1†) as well as in the normal light group (Fig. 2A). These results indicate that the fluorescence quenching effects were not attributed to the photo-induced cross-linking of the peptide with the Ru complexes, but should be due to the interaction between Ru complexes and hIAPP. Furthermore, the fluorescence intensity was proportional to the concentration of 3, which also confirmed the interaction between Ru complexes and hIAPP. Then, for the in-depth exploration of the structural impact of Ru complexes on hIAPP, we take the thioflavin T (ThT) fluorescence assay, which is thought to be the effective method to detect amyloid fibril formation.39 The degree of amyloid fibril formation is associated with the intensity of ThT fluorescence signal, which was thought to be a proportional relationship. Through ThT assay (Fig. 2B), we found that compared with the hIAPP group, ThT fluorescence signal decreased after incubation with complexes 1, 2, 3 and 4 at pH 7.4, suggesting that Ru complexes could inhibit the formation of hIAPP fibril. Furthermore, the multimodal size distribution peaks of hIAPP after treatment with Ru complexes was revealed by the particle size analysis. The mean radius of hIAPP was more than 1000 nm after 48 h incubation, whereas the radius decreased dramatically after the addition of Ru complexes (Fig. 2C). Taking complex 3 as an example, hIAPP size obtained a minimum of less than 400 nm under the influence of complex 3. The size distribution of hIAPP with or without complex 3 is shown in Fig. 2D. The result of particle size analysis further confirmed that different Ru complexes could inhibit hIAPP aggregation in vitro.
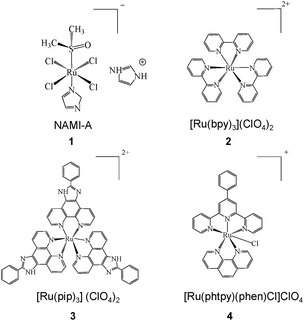 |
| Fig. 1 Chemical structures of Ru complexes used in this study. | |
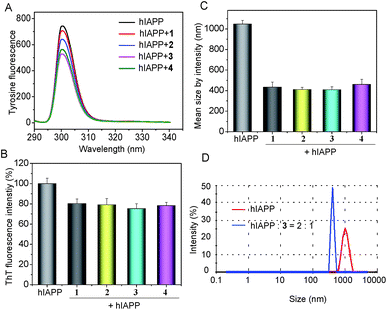 |
| Fig. 2 The influence of Ru complexes on hIAPP fibrillation. (A) The tyrosine fluorescence signal of 10 μM hIAPP after treatment with or without Ru complexes (5 μM) in normal light. (B) The intensity of ThT fluorescence signal of 10 μM hIAPP after incubation with or without 5 μM different Ru complexes for 48 h at 37 °C. The fluorescence signal was quenched after incubation with the complexes. (C) Size distribution of 10 μM hIAPP incubated alone or with different complexes for 48 h at 37 °C characterized by intensity. (D) Size distribution of 10 μM hIAPP incubated alone or with 5 μM complex 3 for 48 h at 37 °C characterized by intensity. | |
TEM images validated the changed morphology of hIAPP by complexes 1, 2, 3 and 4 visually. Herein, TEM and AFM images both showed that the fibrillation of hIAPP appeared when incubated alone for 0 h or 48 h (Fig. 3 and 4A). Whereas, when hIAPP incubated with 5 μM different Ru complexes, fibrillation was interrupted by them to form nanoscale granular particles. As showed in Fig. 3, slender and fibroid amyloid fibrils were observed evidently at the beginning. Whereas, after 24 h of incubation, the disaggregation of fibril was clearly seen, the long fibroid hIAPP have disaggregated into many shortened fibrils and there appeared some spherical particles. After 48 h of incubation, there were no visible fibrils; moreover, most of the previous shortened fibrils were disaggregated and changed into different degrees of spherical particles. Compared with other complexes, the complex 3 incubated with hIAPP presented a thorough disaggregation with the appearance of dispersive and small spherical particles, demonstrating that the fibrils were completely disaggregated into spherical particles. To investigate the complex 3 potential for fibril disaggregation, the individual AFM and TEM imaging was performed (Fig. 4). hIAPP could form fibrils during incubation alone (Fig. 4A), while nanoscale oligomers were formed when the peptides were incubated with complex 3 for 48 h (Fig. 4B). Fig. 4C demonstrated the surface morphology of line a and line b, which demonstrates the difference between smooth hIAPP fibril and granular particles. Complex 3 blocked the formation of hIAPP oligomers to form small particles, which continued to grow into nano size particles in 24 h (Fig. 4D). The inhibition of hIAPP fibrillation by Ru complexes was also revealed by a time-course study. The potential mechanism of the time-dependent disintegration of hIAPP fibril by complex 3 is proposed in Fig. 4E. These results were in accordance with tyrosine intrinsic fluorescence assay, ThT fluorescence assay and particle size analysis. It proved that amyloid fibril formation was restrained by Ru complexes, which tended to form granular particles.
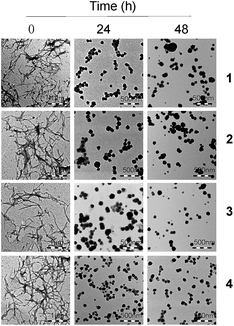 |
| Fig. 3 TEM images of time-dependent disaggregation of 10 μM hIAPP incubated with 5 μM different Ru complexes for 24 h or 48 h at 37 °C. | |
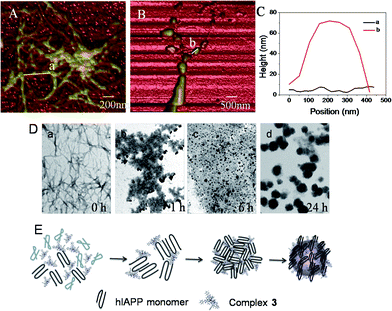 |
| Fig. 4 Complex 3 disaggregate hIAPP fibrils. AFM images of 10 μM hIAPP incubated alone (A), with 5 μM complex 3 (B) for 48 h at 37 °C. (C) Height and position comparison of the fibril and granular aggregate along with lines a and b. (D) TEM images of 10 μM hIAPP incubated (a) alone, (b–d) with 5 μM complex 3 for 1 h, 6 h and 24 h at 37 °C, respectively. (E) Proposed schematic diagram of the mechanism of the disintegration of hIAPP amyloid fibril by complex 3. | |
Furthermore, mass spectrometry was used to examine the chemical interaction between the hIAPP and the Ru complexes.
As shown in Fig. 5, sharp peaks were observed in the spectra of complex 3 and hIAPP alone. After the incubation, new peaks were observed, indicating the binding and interaction between complex 3 and hIAPP. The detailed analysis of the peaks revealed the binding sequences of the peptides with the Ru complexes.
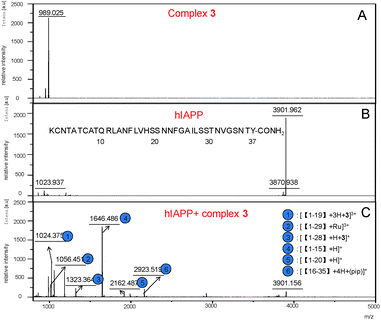 |
| Fig. 5 Interaction of the hIAPP with complex 3. MALDI-TOF-MS analysis of 5 μM complex 3 (A), 10 μM hIAPP (B) and the mixed solution of complex 3 and hIAPP (C) after incubation for 48 h at 37 °C. | |
Ru complexes inhibit hIAPP-induced cell apoptosis
Misfolded hIAPP fibrillating is known to be crucial in the pathogenesis of T2DM, leading to the death of islet beta cells.40 The Ru complexes could inhibit hIAPP fibrillation and disaggregate hIAPP amyloid fibril into nanoscale particles in PBS buffer, but whether the Ru complexes could disaggregate hIAPP fibrils in cells is indeterminable. Therefore, we select INS-1 cells as a pattern to examine whether the disaggregation still exists. Herein, the cytotoxicity of hIAPP on cultured INS-1 cells in the absence and presence of complexes 1, 2, 3 and 4 were measured by MTT assay. As shown in Fig. 6A–D, the INS-1 cells' viability was reduced after being cultured with 20 μM hIAPP alone, whereas in presence of different concentrations of Ru complexes, the reduction of cell viability was suppressed, except for the complex 1. As shown in Fig. 6A, after being co-treated with 5 μM complex 1 the hIAPP showed a higher toxicity in the INS-1 cells, which indicates that complex 1 will induce cell death at the concentration of 5 μM. On the other hand, these results indicated that most of the Ru complexes could effectively inhibit hIAPP-induced beta cell death. Take complex 3 for instance, the viability of the cells treated with hIAPP alone was only 83.2%, whereas after the hIAPP was incubated with complex 3, cell viability increased to 101.1% and 105.2% (Fig. 6C), indicating that complex 3 not only weakened the cell cytotoxicity of hIAPP fibrillation on INS-1 cells but also promoted cell growth. To characterize that hIAPP-induced INS-1 cell death was weakened by Ru complexes directly, INS-1 cells were examined by phase contrast microscopy (Fig. S4†). Compared with healthy and regularly shaped control cells, the cells after being cultured with hIAPP exhibited anomalous cellular morphology and lessened cell numbers. Whereas, after hIAPP was co-treated with Ru complexes, hIAPP-induced cell death and morphological changes were inhibited. To identify whether the Ru complexes could inhibit hIAPP-induced apoptosis, the caspase activity assay was conducted to analyze the activation of caspase-8 (Fas/TNF-mediated), caspase-9 (mitochondrial-mediated) and caspase-3 (executive caspase), which are vital components involved in extrinsic and intrinsic apoptotic signaling pathways.33 As shown in Fig. 6E, hIAPP treatment significantly enhanced the activity of caspase-8 and caspase-9, and finally triggered the activity of executive caspase-3. Interestingly, the addition of Ru complexes markedly reversed the activation of caspase-8 and caspase-9, which irreversibly blocked the activity of caspase-3 in hIAPP-treated INS-1 cells. These results indicate that Ru complexes act as effective inhibitors for hIAPP-caused cell death.
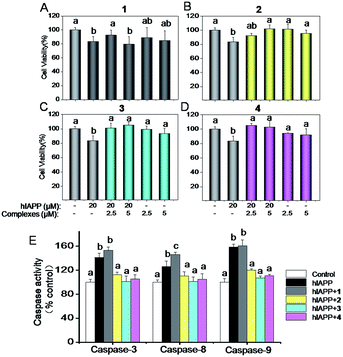 |
| Fig. 6 Ru complexes inhibit hIAPP-induced cell cytotoxicity and apoptosis. The cells were treated with 20 μM hIAPP in combination with different concentrations of complexes 1 (A), 2 (B), 3 (C) and 4 (D) at 37 °C for 48 h. (E) The caspase activities in cells treated with hIAPP were suppressed by the Ru complexes. INS-1 cells were pretreated with hIAPP (10 μM) and Ru complexes (5 μM) for 48 h as described in the Experimental section. Caspase activities were measured by synthetic fluorogenic substrate. Bars with different characters are statistically different at P < 0.05 level as analyzed by one-way ANOVA multiple comparison. | |
Ru complexes inhibit hIAPP-induced ROS generation
Existing studies demonstrated that mitochondrial dysfunction may lead to insulin secretory failure and metabolic dysregulation in T2DM.41,42 Mitochondria is thought to be the principal source of ROS, whose ROS-signaling enhances ROS production, leading to potentially significant mitochondrial and cellular injury.43,44 ROS production has been highlighted as an initiator for hIAPP-induced cell death in pancreatic beta cells.45–47 To deeply understand the biochemical and intracellular interaction between the Ru complexes and hIAPP, the intracellular ROS level was monitored by detecting the intensity of fluorescein-labeled dye (H2DCF-DA and DHE). Fig. 7 shows the DCF fluorescence intensity as an indicator of ROS generation produced during hIAPP fibril formation with or without Ru complexes. After incubation of hIAPP, the intensity of the fluorescence was almost ten times higher than the control group. However, after co-treatment with Ru complexes, ROS generation was reduced dose-dependently, implying that Ru complexes has disaggregated hIAPP amyloid fibrils, thus suppressed ROS formation. The same result was demonstrated in the DHE fluorescence assay (Fig. S5†), which indicated that the superoxide anion induced by hIAPP in INS-1 cells was effectively inhibited by Ru complexes. Among these complexes, complex 3 achieved the best effect on inhibition of ROS generation. Therefore, Ru complexes, especially complex 3, may exert a protective effect towards hIAPP-caused cell damage by restraining ROS generation.
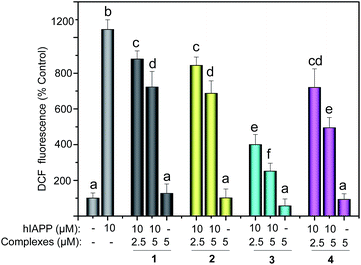 |
| Fig. 7 Ru complexes reduced ROS generation that was induced dose-dependently by hIAPP. INS-1 cells incubated hIAPP (10 μM) alone or with different Ru complexes (5 μM) at 37 °C for 60 min. The changes of ROS level were determined by DCF fluorescence intensity. The fluorescence intensity of the control that was treated without hIAPP and Ru complexes was expressed as 100%. The values represented were means ± SD from three independent experiments. Bars with different characters are statistically different at P < 0.05 level as analyzed by one-way ANOVA multiple comparison. | |
Inhibition of hIAPP fibrillation inside INS-1 cells by Ru complexes
Ru complexes showed the inhibition of hIAPP-caused cell damage; this is possibly due to the intracellular hIAPP fibrillation being interfered with by the Ru complexes. To confirm whether the fibril disaggregation was still happening in INS-1 cells, the THT fluorescence assay was carried out in INS-1 cells. As shown in Fig. 8A, a strong and evident green fluorescence was observed in cells treated with hIAPP alone; moreover, when co-incubated with Ru complexes, the ThT fluorescence intensity decreased, with only a slight green fluorescence observed. The quantitative analysis of fluorescence intensity is demonstrated in Fig. 8B. The incubation of hIAPP alone increased the fluorescence intensity to 146.7%, which declined to 123.2%, 121.5%, 114.7% and 118.4% after incubation with complexes 1, 2, 3 and 4, respectively, indicating that hIAPP fibril formation was inhibited by the complexes. Obviously, complex 3 showed a more effective inhibition. To further investigate the mechanism for this phenomenon of complex 3 showing a more effective inhibition, the lipophilicity of the complexes was investigated (Fig. 8B). Several studies have showed that the cellular uptake of Ru complexes was connected with their action of anticancer activities, which is thought to be energy-dependent and dependent on their lipophilicities.48,49 Whether the lipophilicities of the Ru complexes in our study are proportional to their inhibition of hIAPP fibril formation is not yet clear. From the result, complex 3 exhibited the highest lipophilicity (log
P = 1.27), which is consistent with its best inhibition of hIAPP fibril formation. Therefore, it is possible that high lipophilicity could enhance the cellular uptake of complex 3, which in turn further increases the interaction of the complexes with hIAPP, thus inhibiting the formation of hIAPP fibril.
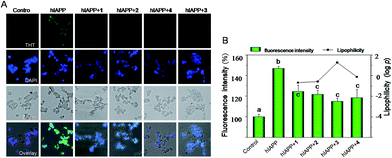 |
| Fig. 8 ThT fluorescence of hIAPP fibrillation inside INS-1 cells. (A) INS-1 cells were pretreated with 10 μM hIAPP and 5 μM Ru complexes for 48 h at 37 °C, ThT staining was used to examine the presence of intracellular hIAPP. The stained cells were visualized by a fluorescence microscope. (B) ThT fluorescence intensity reflects the quantified evaluation of hIAPP aggregation; moreover, the relationship between the ThT fluorescence and lipophilicity (log P) of the complexes was also shown. Bars with different characters are statistically different at P < 0.05 level as analyzed by one-way ANOVA multiple comparison. | |
Conclusions
Recent studies have shown that the death of islet beta cells was due to the misfolding and hIAPP fibril formation in the pathogenesis of T2DM.50,51 Thus, discovery of drugs that could inhibit the formation of amyloid fibrillation is thought to be a potentially therapeutic strategy towards diabetes. In this study, Ru complexes 1, 2, 3 and 4 were selected to investigate their influence on hIAPP fibrillation. Decreased tyrosine intrinsic fluorescence and ThT fluorescence signal proved that hIAPP fibril formation was inhibited by Ru complexes. The particle size analysis, AFM images and TEM images further indicated that the fibrillation of hIAPP was interrupted by Ru complexes to form nanoscale granular particles. This inhibition was revealed as a time-dependent process. After that, we found that Ru complexes could protect INS-1 cells from hIAPP-caused cell damage by suppressing ROS generation during hIAPP fibrillation in cells and the blocking of caspase activation and cell apoptosis. Moreover, the inhibition of hIAPP fibril formation was discovered to be proportional to the lipophilicity of the complexes. The complex with the highest lipophilicity showed the most effective inhibition. Taken together, this study provides a strategy for designing Ru complexes for treating T2DM by targeting hIAPP.
Acknowledgements
This study was supported by the National High Technology Research and Development Program of China (863 Program, SS2014AA020538), the Science Foundation for Distinguished Young Scholars of Guangdong Province, the Natural Science Foundation of China and Guangdong Province, the Foundation for High-level Talents in Higher Education of Guangdong, Research Fund for the Doctoral Program of Higher Education of China (20114401110004), the China Postdoctoral Science Foundation, the Shenzhen Basic Research Grant (JCYJ20130401152508660) and the YangFan Innovative & Entrepreneurial Research Team Project (201312H05).
Notes and references
- D. Eisenberg and M. Jucker, Cell, 2012, 148, 1188–1203 CrossRef CAS PubMed.
- F. Chiti and C. M. Dobson, Annu. Rev. Biochem., 2006, 75, 333–366 CrossRef CAS PubMed.
- S. Laurent, M. R. Ejtehadi, M. Rezaei, P. G. Kehoe and M. Mahmoudi, RSC Adv., 2012, 2, 5008–5033 RSC.
- P. Westermark, C. Wernstedt, E. Wilander, D. W. Hayden, T. D. O'Brien and K. H. Johnson, Proc. Natl. Acad. Sci. U. S. A., 1987, 84, 3881–3885 CrossRef CAS.
- I. Saraogi, J. A. Hebda, J. Becerril, L. A. Estroff, A. D. Miranker and A. D. Hamilton, Angew. Chem., 2010, 122, 748–751 CrossRef.
- R. L. Hull, G. T. Westermark, P. Westermark and S. E. Kahn, J. Clin. Endocrinol. Metab., 2004, 89, 3629–3643 CrossRef CAS PubMed.
- S. E. Kahn, D. A. D'Alessio, M. W. Schwartz, W. Y. Fujimoto, J. W. Ensinck, G. J. Taborsky and D. Porte, Diabetes, 1990, 39, 634–638 CrossRef CAS.
- A. Lukinius, E. Wilander, G. Westermark, U. Engström and P. Westermark, Diabetologia, 1989, 32, 240–244 CrossRef CAS.
- E. Ahmad, A. Ahmad, S. Singh, M. Arshad, A. H. Khan and R. H. Khan, Biochimie, 2011, 93, 793–805 CrossRef CAS PubMed.
- J. R. Brender, S. Salamekh and A. Ramamoorthy, Acc. Chem. Res., 2011, 45, 454–462 CrossRef PubMed.
- J. A. Hebda and A. D. Miranker, Annu. Rev. Biophys., 2009, 38, 125–152 CrossRef CAS PubMed.
- X. Li, L. Ma, W. Zheng and T. Chen, Biomaterials, 2014, 35, 8596–8604 CrossRef CAS PubMed.
- T. A. Mirzabekov, M. C. Lin and B. L. Kagan, J. Biol. Chem., 1996, 271, 1988–1992 CrossRef CAS PubMed.
- A. Quist, I. Doudevski, H. Lin, R. Azimova, D. Ng, B. Frangione, B. Kagan, J. Ghiso and R. Lal, Proc. Natl. Acad. Sci. U. S. A., 2005, 102, 10427–10432 CrossRef CAS PubMed.
- X. Zhou, J. Tan, L. Zheng, S. Pillai, B. Li, P. Xu, B. Zhang and Y. Zhang, RSC Adv., 2012, 2, 5418–5423 RSC.
- L. A. Scrocchi, Y. Chen, S. Waschuk, F. Wang, S. Cheung, A. A. Darabie, J. McLaurin and P. E. Fraser, J. Mol. Biol., 2002, 318, 697–706 CrossRef CAS.
- K. Jeong, W. Y. Chung, Y. S. Kye and D. Kim, Bioorg. Med. Chem., 2010, 18, 2598–2601 CrossRef CAS PubMed.
- R. Mishra, B. Bulic, D. Sellin, S. Jha, H. Waldmann and R. Winter, Angew. Chem., Int. Ed. Engl., 2008, 47, 4679–4682 CrossRef CAS PubMed.
- B. Alies, C. Hureau and P. Faller, Metallomics, 2013, 5, 183–192 RSC.
- B. Ward, K. Walker and C. Exley, J. Inorg. Biochem., 2008, 102, 371–375 CrossRef CAS PubMed.
- V. B. Kenche, I. Zawisza, C. L. Masters, W. Bal, K. J. Barnham and S. C. Drew, Inorg. Chem., 2013, 52, 4303–4318 CrossRef CAS PubMed.
- T. Y. Lee, W. S. Chei, H. Ju, M. S. Lee, J. W. Lee and J. Suh, Bioorg. Med. Chem. Lett., 2012, 22, 5689–5693 CrossRef CAS PubMed.
- N. A. Vyas, S. S. Bhat, A. S. Kumbhar, U. B. Sonawane, V. Jani, R. R. Joshi, S. N. Ramteke, P. P. Kulkarni and B. Joshi, Eur. J. Med. Chem., 2014, 75, 375–381 CrossRef CAS PubMed.
- L. He, X. Wang, C. Zhao, H. Wang and W. Du, Metallomics, 2013, 5, 1599–1603 RSC.
- T. Chen, Y. Liu, W.-J. Zheng, J. Liu and Y.-S. Wong, Inorg. Chem., 2010, 49, 6366–6368 CrossRef CAS PubMed.
- G. Ramakrishna, D. A. Jose, D. K. Kumar, A. Das, D. K. Palit and H. N. Ghosh, J. Phys. Chem. B, 2005, 109, 15445–15453 CrossRef CAS PubMed.
- C. Tan, S. Hu, J. Liu and L. Ji, Eur. J. Med. Chem., 2011, 46, 1555–1563 CrossRef CAS PubMed.
- Z. Luo, L. Yu, F. Yang, Z. Zhao, B. Yu, H. Lai, K.-H. Wong, S.-M. Ngai, W. Zheng and T. Chen, Metallomics, 2014, 6, 1480–1490 RSC.
- L. Li, Y.-S. Wong, T. Chen, C. Fan and W. Zheng, Dalton Trans., 2012, 41, 1138–1141 RSC.
- B.-w. Jing, W.-q. Wang, M.-h. Zhang and T. Shen, Dyes Pigm., 1998, 37, 177–186 CrossRef CAS.
- W. Liu, X. Li, Y.-S. Wong, W. Zheng, Y. Zhang, W. Cao and T. Chen, ACS Nano, 2012, 6, 6578–6591 CrossRef CAS PubMed.
- T. Chen and Y.-S. Wong, Biomed. Pharmacother., 2009, 63, 105–113 CrossRef CAS PubMed.
- Y. Huang, L. He, W. Liu, C. Fan, W. Zheng, Y.-S. Wong and T. Chen, Biomaterials, 2013, 34, 7106 CrossRef CAS PubMed.
- L. He, Y. Huang, H. Zhu, G. Pang, W. Zheng, Y. S. Wong and T. Chen, Adv. Funct. Mater., 2014, 24, 2754–2763 CrossRef CAS.
- Y.-P. Yu, P. Lei, J. Hu, W.-H. Wu, Y.-F. Zhao and Y.-M. Li, Chem. Commun., 2010, 46, 6909–6911 RSC.
- V. Pierroz, T. Joshi, A. Leonidova, C. Mari, J. Schur, I. Ott, L. Spiccia, S. Ferrari and G. Gasser, J. Am. Chem. Soc., 2012, 134, 20376–20387 CrossRef CAS PubMed.
- L. Ma, X. Li, Y. Wang, W. Zheng and T. Chen, Int. J. Biochem. Cell Biol., 2014, 140, 143–152 CAS.
- Q. Ma, Y. Li, J. Du, H. Liu, K. Kanazawa, T. Nemoto, H. Nakanishi and Y. Zhao, Peptides, 2006, 27, 841–849 CrossRef CAS PubMed.
- H. LeVine III, Methods Enzymol., 1999, 309, 274–284 Search PubMed.
- F. H. Epstein, J. W. Höppener, B. Ahrén and C. J. Lips, N. Engl. J. Med., 2000, 343, 411–419 CrossRef PubMed.
- M. Bensellam, L. Van Lommel, L. Overbergh, F. Schuit and J.-C. Jonas, Diabetologia, 2009, 52, 463–476 CrossRef CAS PubMed.
- H. Mulder and C. Ling, Mol. Cell. Endocrinol., 2009, 297, 34–40 CrossRef CAS PubMed.
- Q. Xie, L. He, H. Lai, W. Zheng and T. Chen, RSC Adv., 2014, 4, 34210–34216 RSC.
- D. B. Zorov, M. Juhaszova and S. J. Sollott, Biochim. Biophys. Acta, 2006, 1757, 509–517 CrossRef CAS PubMed.
- X.-L. Li, G. Xu, T. Chen, Y.-S. Wong, H.-L. Zhao, R.-R. Fan, X.-M. Gu, P. C. Tong and J. C. Chan, Int. J. Biochem. Cell Biol., 2009, 41, 1526–1535 CrossRef CAS PubMed.
- L. Haataja, T. Gurlo, C. J. Huang and P. C. Butler, Endocr. Rev., 2008, 29, 303–316 CrossRef CAS PubMed.
- B. Konarkowska, J. F. Aitken, J. Kistler, S. Zhang and G. J. Cooper, FEBS J., 2006, 273, 3614–3624 CrossRef CAS PubMed.
- C. A. Puckett and J. K. Barton, J. Am. Chem. Soc., 2007, 129, 46–47 CrossRef CAS PubMed.
- Z. Zhao, Z. Luo, Q. Wu, W. Zheng, Y. Feng and T. Chen, Dalton Trans., 2014, 43, 17017–17028 RSC.
- J. W. Hoppener, B. Ahren and C. J. Lips, N. Engl. J. Med., 2000, 343, 411–419 CrossRef CAS PubMed.
- J. W. Hoppener and C. J. Lips, Int. J. Biochem. Cell Biol., 2006, 38, 726–736 CrossRef PubMed.
Footnotes |
† Electronic supplementary information (ESI) available. See DOI: 10.1039/c4ra15152f |
‡ These authors contributed equally to the work. |
|
This journal is © The Royal Society of Chemistry 2015 |
Click here to see how this site uses Cookies. View our privacy policy here.