DOI:
10.1039/D4RA01646G
(Paper)
RSC Adv., 2024,
14, 11388-11399
Investigation of bioactive components responsible for the antibacterial and anti-biofilm activities of Caroxylon volkensii by LC-QTOF-MS/MS analysis and molecular docking†
Received
3rd March 2024
, Accepted 28th March 2024
First published on 9th April 2024
Abstract
Caroxylon volkensii is a wild desert plant of the family Amaranthaceae. This study represents the first report of the metabolomic profiling of C. volkensii by liquid chromatography quadrupole-time-of-flight tandem mass spectrometry (LC-QTOF-MS/MS). The dereplication study of its secondary metabolites led to the characterization of 66 known compounds. These compounds include catecholamines, tyramine derivatives, phenolic acids, triterpenoids, flavonoids, and others. A new tyramine derivative, alongside other known compounds, was reported for the first time in the Amaranthaceae family. The new derivative and the first-reported compounds were putatively identified through MS/MS fragmentation data. Given the notorious taxonomical challenges within the genus Salsola, to which C. volkensii previously belonged, our study could offer a valuable insight into its chemical fingerprint and phylogenetic relationship to different Salsola species. The antibacterial potential of C. volkensii methanolic extract (CVM) against Pseudomonas aeruginosa was screened. The minimum inhibitory concentration (MIC) of CVM ranged from 32 to 256 μg mL−1. The anti-quorum sensing potential of CVM resulted in a decrease in the percentage of strong and moderate biofilm-forming isolates from 47.83% to 17.39%. It revealed a concentration-dependent inhibitory activity on violacein formation by Chromobacterium violaceum. Moreover, CVM exhibited an in vivo protective potential against the killing capacity of P. aeruginosa isolates. A molecular docking study revealed that the quorum-sensing inhibitory effect of CVM can be attributed to the binding of tyramine conjugates, ethyl-p-digallate, and isorhamnetin to the transcriptional global activator LasR.
1. Introduction
Since the first use of antibiotics, they have revolutionized the treatment of bacterial infections.1 Nevertheless, this important achievement is increasingly failing, owing to the dissemination of bacterial resistance to almost all classes of antibiotics, constituting a great worldwide health challenge. The number of patients with untreatable bacterial infections is increasing and consequently, the mortality rate of these infections is unfortunately high.2 Deaths brought on by bacterial infections are estimated to be the second most common cause of death worldwide.3 This most serious situation dictates the necessity of finding novel approaches to combat the bacterial resistance.1
Quorum sensing (QS) is a communication mechanism employed by bacterial cells to control the expression of virulence genes based on changes in their population density.4 It is one of the major mechanisms used by bacteria for biofilm formation, adaptive antibiotic resistance, increasing virulence, and overcoming immune responses.5–7 Quorum sensing inhibition is among the novel therapeutic approaches used for controlling antibiotic-resistant bacteria.6,8,9
Plants have been employed since ancient times in traditional medicine for addressing infectious diseases before the era of antibiotics.10,11 In recent times, there has been a renewed interest in exploring plants for the discovery of antimicrobials. This is attributed to their capacity to generate numerous bioactive secondary metabolites that possess the potential to combat bacterial infections and boost the human immune system.12–14
Caroxylon volkensii (Schweinf. & Asch.) Akhani & Roalson is an annual herb belonging to the family Amaranthaceae. According to the International Plant Names Index (IPNI), the plant was mentioned for the first time under the name of Salsola volkensii (Schweinf. & Asch.) in Mémoires de l'Institut Égyptien Collation 2: 130 (1887). It had previously been classified as a member of the Old-World Salsola (family Chenopodiaceae), which encompassed a large number of desert plants.15 The taxonomic classification of this plant was revised by Akhani et al. (2007), who transferred it to the genus Caroxylon.16 The plant was also suggested by Feodorova (2015) to be segregated into the genus Nitrosalsola, based on its anatomical and molecular phylogenetic data.17 Hence it has two synonyms, C. volkensii (Schweinf. & Asch.) Akhani & Roalson and Nitrosalsola volkensii (Schweinf. & Asch.) Theodorova and the basionym of Salsola volkensii Asch. & Schweinf. It was described in the flora of Egypt as shrubs or subshrubs having small, alternate, sessile leaves with solitary flowers forming dense or loose spikes at the leaf axils and showing 5-segmented perianth.18 It is also recognized by its characteristic odor of decaying fish when bruised.18 It grows in the desert and sandy plains of Egypt, Saudi Arabia, Palestine, and Iraq.18 The limited native land of the plant and its occurrence in desert and arid environments have so far restricted the research studies on its biological activities and phytochemical components. Only one research study has described the hepatoprotective effects of its alcoholic extract against CCl4-induced hepatotoxicity in Sprague Dewaly rats.19 Various plant species within the Old-World Salsola genus have been documented for their ability to exhibit antimicrobial effects against diverse pathogens.15 Hence, in the present research, we investigated the antibacterial properties of C. volkensii and clarified its capabilities in inhibiting biofilm formation and disrupting quorum sensing in clinical isolates of Pseudomonas aeruginosa.
Dereplication by Liquid Chromatography coupled to Quadrupole Time-of-Flight Mass Spectrometry (LC-QTOF-MS/MS) is a powerful analytical approach used in natural product research and metabolomics. It combines the separation capabilities of liquid chromatography with the high-resolution and accurate-mass measurement capabilities of quadrupole time-of-flight mass spectrometry. It involves rapid identification of previously characterized compounds, even from complex mixtures of natural products. Employing dereplication strategies minimizes redundant efforts of purification and re-identification of known compounds and prioritizes the isolation of novel chemical entities.20 The dereplication strategy using LC-QTOF-MS/MS has been extensively used for rapid identification of natural products in plants, bacteria, and fungi.20–22
In the current study, we investigated the phytochemical content of C. volkensii for the first time, using a dereplication process by LC-QTOF-MS/MS analysis. From a taxonomical perspective, it is worth mentioning that the genus Salsola, to which C. volkensii was belonging, is among the most contentious plant genera. The taxonomy of Salsola species is characterized by an ongoing debate and confusion within the scientific community.15 This underscores the importance of our study in understanding the taxonomic classification of C. volkensii and its taxonomic relationship to other Salsola species and to Amaranthaceae plants through the analysis of its metabolomic profile. We also performed a docking study of the major identified compounds against the LasR enzyme, which plays a role in controlling the expression of numerous genes associated with biofilm formation, pathogenicity, and virulence.
2. Materials and methods
2.1. Plant material
Aerial parts of Caroxylon volkensii (Schweinf. & Asch.) Akhani & Roalson (Fig. 1) were collected from Aswan in March 2017 and were identified by Dr Yassin Al-Sodany, professor of Plant Ecology, in the Botany Department within the Faculty of Science at Kafrelsheikh University.
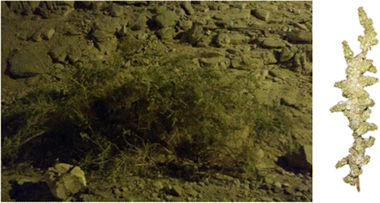 |
| Fig. 1 Caroxylon volkensii (Schweinf. & Asch.) Akhani & Roalson plant photographs. | |
2.2. Chemicals
Methanol and acetonitrile, as organic solvents of HPLC grade, were purchased from Fisher Chemicals (Fair Lawn, NJ, USA). Formic acid (HPLC-grade) was acquired from Sigma Chemical Co. (Saint-Louis, MO, USA). Deionized water was obtained using a GenPure purification system (Thermo Fisher Scientific, Waltham, MA, USA).
2.3. Preparation of the plant extract
50 g of the dried powdered plant material was extracted with methanol (250 mL) assisted by sonication for 30 min at room temperature. The extraction process was repeated three times. The supernatant was filtered and dried using a rotary evaporator at 45 °C, giving 6.5 g of total dry crude extract. A portion of 1 mg of the extracted sample was dissolved in 1 mL of methanol and subsequently filtered using 0.45 μm polyvinylidene difluoride (PVDF) syringe filters for utilization in LC-MS/MS analysis.
2.4. LC-MS/MS analysis
The methanol extract of C. volkensii (CVM) was analyzed on an HPLC-MS system consisting of a 1260 Infinity II Flexible HPLC coupled to a 6530 Q-TOF-MS (Agilent Technologies, USA). An injection of 8 μL from the processed crude extract was introduced into a Zorbax RP-18 column provided by Agilent Technologies (150 mm × 3 mm, 2.7 μm), maintained at a temperature of 40 °C, with a flow rate set at 0.5 mL min−1. The mobile phase system comprised a combination of solvent A (0.1% formic acid) and a gradual gradient increase from 0 to 100% of solvent B (acetonitrile + 0.1% formic acid) over a duration of 120 min. Mass spectra were acquired in both positive and negative electrospray ionization (ESI) modes, employing a capillary voltage of 4000 V. The recorded spectra encompassed the m/z values range from 100 to 1500. Parameters such as the capillary temperature and drying gas flow were adjusted to 320 °C and 10 L min−1, respectively. The collision energy and the nebulization pressure were adjusted to 18–45 eV and 40 psi respectively. The LC-MS/MS data acquired was transformed using Proteowizard msconvert, followed by processing and analysis through MZmine.
2.5. Biological study
2.5.1. In vitro antibacterial activity and susceptibility to Caroxylon volkensii. The utilized culture media and chemicals employed were sourced from Oxoid in the UK and Merck in the USA. A collection of 23 clinical isolates of P. aeruginosa was acquired from the culture repository at the Department of Pharmaceutical Microbiology, Faculty of Pharmacy, Tanta University, Egypt. The reference isolate utilized was P. aeruginosa ATCC 27853.In vitro testing of antibacterial susceptibility to CVM was conducted using the agar well diffusion method.23 Following the inoculation of Muller-Hinton agar (MHA) plates with bacterial suspensions, wells were created and loaded with CVM, ciprofloxacin (used as a positive control), and dimethyl sulfoxide (DMSO) at a concentration of 10% (employed as a negative control). Subsequently, the plates underwent an overnight incubation at 37 °C. CVM demonstrated antibacterial activity by generating zones of inhibition around the wells. The determination of minimum inhibitory concentrations (MICs) was carried out using the broth microdilution method as previously outlined.24
2.5.2. Anti-biofilm and anti-quorum sensing activities of Caroxylon volkensii. Initially, the ability of the examined isolates to form biofilms was assessed through the crystal violet assay as previously reported.25 The optical density (OD) at 490 nm was measured using an ELISA reader (Sunrise Tecan, Austria). The tested isolates were categorized into four groups based on their OD values: Non-biofilm producer (NBP) for OD values between the cut-off (ODc) and twice ODc, Weak biofilm producer (WBP) for OD values between twice ODc and four times ODc, Moderate biofilm producer (MBP) for OD values between four times ODc and six times ODc, Strong biofilm producer (SBP) for OD values greater than six times ODc. The cut-off OD (ODc) was determined as the mean OD plus three times the standard deviation (SD) of the negative control. After grouping the bacterial isolates into four groups (strong, moderate, weak, and non-biofilm forming), they were treated with CVM (0.5 MIC). Subsequently, the assessment of biofilm-forming ability was repeated to evaluate the impact of CVM. The quorum quenching potential (quorum inhibitory action) of CVM was elucidated as previously described.26 In brief, various concentrations of CVM were created through a serial two-fold dilution method, commencing from 1 μg mL−1 and progressing up to 1024 μg mL−1. The prepared concentrations were then added to Chromobacterium violaceum (ATCC 12472) suspension and left at 30 °C. After overnight incubation, the mixtures were centrifuged and DMSO (500 mL) was added to the formed pellets. Vigorous vortexing was carried out to extract the violacein pigment (purple), subsequently quantified by measuring the optical density (OD) at 585 nm using an ELISA reader (Sunrise Tecan, Austria). The control group involved a C. violaceum suspension without the presence of CVM.
2.5.3. In vivo protection assay. Thirty male albino mice were provided by the animal facility of Cairo University, Egypt. The mice, weighing between 22 g and 26 g, were provided with standard pellet meals and access to filtered water. Furthermore, the mice were housed in a controlled environment with a temperature set at 25 °C and subjected to a 12 hour light and dark cycle.27 Following a 7 day acclimation period for the mice, the in vivo experiment was conducted in accordance with the established guidelines for laboratory animal care and services. The study received ethical approval with the designated number TP/RE/06-22P-014 from the Research Ethical Committee of the Faculty of Pharmacy at Tanta University, Egypt.A survival assay was utilized to evaluate the protective efficacy of CVM against P. aeruginosa infection.1 Briefly, P. aeruginosa bacteria in phosphate-buffered saline (1 × 108 CFU mL−1) with and without treatment with CVM (0.5 MIC) were injected via an intraperitoneal route into the mice. The mice were randomly divided into three groups, each consisting of 10 mice: group I (non-infected control group), group II (infected with P. aeruginosa but untreated with CVM), and group III (infected with P. aeruginosa and treated with CVM). The survival of the mice was observed over a period of 14 days.
The assays were carried out in triplicate and the results are presented as mean ± standard deviation (SD). Statistical significance was evaluated using two-way ANOVA with a significance level set at p < 0.05. To assess the survival of mice, Kaplan–Meier survival curves were employed. The analysis was conducted using Graph Pad Prism software (Version 8, GraphPad Software Inc., USA).
2.5.4. Docking study. The procedure was carried out utilizing AutoDock Vina 1.2.3 (ref. 28 and 29) following the details outlined in the ESI.†
3. Results and discussion
3.1. LC-MS/MS analysis
Liquid chromatography quadrupole-time-of-flight tandem mass spectrometry (LC-QTOF-MS/MS) analysis was used for the identification of the secondary metabolites present in the methanolic extract of C. volkensii (CVM). The plant sample was analyzed in both negative and positive ESI modes to identify the highest-possible number of secondary metabolites. The total ion chromatograms (TIC) obtained from both modes are presented in Fig. S1–S3.†
The dereplication study of the plant secondary metabolites against the Dictionary of Natural Products (DNP) and METLIN databases led to the identification of 67 compounds belonging to diverse chemical classes. The identification of these compounds relied on the concordance of their ions in positive and/or negative ESI modes with literature data and reviewing the biosynthetic abilities of other Salsola species. Furthermore, the structures of some of these compounds were putatively verified through MS/MS fragmentation data. The molecular formula, mode of ionization, retention time, mass error (≤10 ppm), peak area, MS/MS fragmentation data, as well as bibliographic references of each phytochemical are presented in Table S1.† The identified compounds are categorized into six chemical classes, which will be discussed in detail.
3.2. Alkaloids and nitrogenous compounds
The dereplication study led to the characterization of 30 nitrogenous compounds of various classes (Table S1† and Fig. 2). Salsoline (1.2), an isoquinoline alkaloid previously found in different Salsola species,30–34 was identified in our study. Additionally, we report here the presence of its O-demethylated analog, salsolinol (1.1), in the family Amaranthaceae for the first time. Its identification was supported by the MS/MS fragmentation data (Fig. S4†). Another methylated isoquinoline derivative was tentatively identified based on the correspondence of its molecular ion peak in the positive mode to salsolidine (1.3.1) or N-methylisosalsolidine (1.3.2) that were reported previously in other Salsola species.34 Compound 1.4 with [M − H]− at m/z 218.0816 was tentatively characterized as a pyrrolo[2,1-a]isoquinoline derivative that could be either salsoline A (1.4.1)35–38 or salsoline B (1.4.2).39 Compound 1.5 was identified as a terrestric acid that had previously been isolated from S. collina.37
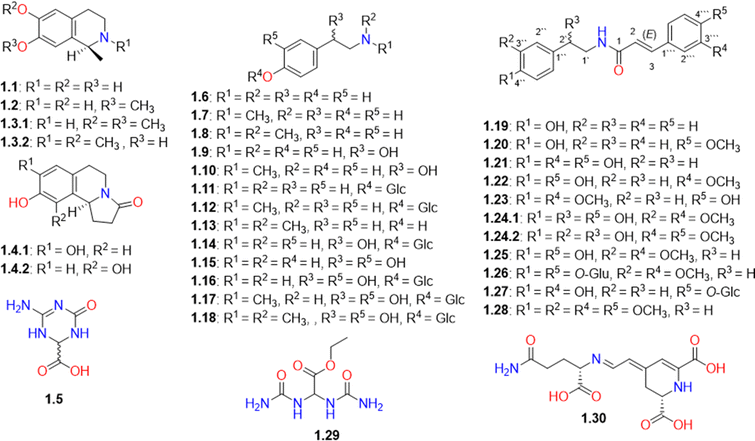 |
| Fig. 2 Structures of alkaloids and other nitrogen-containing compounds (1.1-1.30) identified in Caroxylon volkensii. | |
CVM was found to be rich in N-methyltyramine (1.7), which showed the highest peak intensity among the metabolites detected in the positive ESI mode (Fig. S1†). Its identification was putatively verified through MS/MS fragmentation data (Fig. S6†) which agree with the human metabolome MS/MS spectra with the ID number HMDB0003633.40 Other tyramine derivatives include catecholamines, such as dopamine/octopamine, synephrine, norepinephrine, and their glucosylated analogs (1.6-1.18) were also detected (Table S1† and Fig. 2). The MS/MS spectra of some of these compounds are presented in the ESI file (Fig. S5–S10†).
In this study, catecholamine derivatives were reported in Salsola species for the first time. While tyramine and other catecholamines were previously reported in Spinacia oleracea belonging to the Amaranthaceae plant family.41,42 It is worth noting that catecholamines were previously reported to be synthesized in many plants as a response to stress conditions.42,43 They were suggested to be involved in growth regulation, carbohydrate metabolism, nitrogen detoxification, and protection against pathogens.42 The presence of catecholamines in C. volkensii may be attributed to its growth in a stressful environment under arid conditions. We are suggesting that catecholamines could be the constituents responsible for the traditional use of other Salsola species as antihypertensive agents.15
Another group of nitrogenous compounds that are formed by the conjugation of tyramine analogs with phenolic acids was also detected. This group is represented by moupinamide (N-trans-feruloyltyramine, 1.22), which was reported in several Salsola species.15,44 Other derivatives such as cinnamoyl (1.19), 4-methoxy-cinnamoyl (1.20), and caffeoyl (1.21) tyramine conjugates were also detected (Table S1† and Fig. 2). Another related derivative with a molecular ion peak [M + H]+ at m/z 328.1534 was characterized as (E)-3-(4-hydroxy-3-methoxyphenyl)-N-(4-methoxyphenethyl)acrylamide (1.23), which was reported to be naturally occurring in Sinomenium acutum and Zanthoxylum piperitum.45,46 However, this is the first report of identification of this compound in the family Amaranthaceae, which is supported by MS/MS fragmentation data (Fig. S11†).
Compound 1.24 with [M − H]− at m/z 358.1287 was tentatively characterized as 2′-hydroxy- 3′′-methyoxymoupinamide (1.24.1) or N-(3′,4′-dimethoxy-cinnamoyl)-norepinephrine (1.24.2) that were previously detected in different Salsola species.37,38,47 N-Trans-feruloyl-3-O-methyldopamine (1.25) and rubemamine (E)-N-(3,4-dimethoxyphenethyl)-3-(3,4-dimethoxyphenyl)acrylamide (1.28) previously identified in family Amaranthaceae37,48 were also detected. While aristomanoside (1.26), a di-glucoside derivative of 1.25 previously reported in Aristolochia manshuriensis,49 was detected herein for the first time from the Amaranthaceae family.
Furthermore, we detected the m/z values for several new moupinamide analogs that could be biosynthetically accessible through hydroxylation, methylation, or glycosylation of the identified analogs. However, we couldn't find the MS/MS fragmentation data that support their identification. Fortunately, we got enough MS/MS fragmentation spectra (Fig. S13 and S14†) that could support the identification of only one new analog identified as 4′′′-O-β-D-glucopyranosyl caffeoyltyramine (1.27).
In this research, a urea derivative, namely ethyl 2,2-diureidoacetate (syn. allantoic acid ethyl ester, 1.29) was detected from a natural source for the first time. Its identification in CVM was supported by the MS/MS fragmentation spectrum presented in Fig. S15.† In addition, compound 1.30, with a molecular ion peak [M + H]+ at m/z 340.1131897, was tentatively identified as vulgaxanthin I that had been previously identified in Beta vulgaris, a plant belonging to the family Amaranthaceae.50
3.3. Phenolic acids and simple phenols
The current LC-MS study revealed the presence of several phenolic compounds in the methanolic extract of C. volkensin (Table S1† and Fig. 3). These compounds included cinnamic acid (2.1), ferulic acid (2.2), rosmarinic acid (2.3), vanillic acid glucoside (2.5), and resorcinol (2.6), which had been identified previously in different plants belonging to family Amaranthaceae. Two phenolic compounds (2.4 and 2.7) were observed in relatively high concentrations in CVM as indicated by their high peak intensities in the TIC (negative mode, Fig. S2†). Compound 2.7 was identified as ethyl-p-digallate previously isolated from Mangifera indica.51 This study represents the first instance of identifying this compound within the plant family Amaranthaceae. Its identification was supported by the MS/MS fragmentation data presented in Fig. S17,† while compound 2.4 was identified as the sulfonic acid derivative, tichocarpol A, which had previously been isolated from Tichocarpus crinitus algae.52 This study represents the first report on the occurrence of this compound in higher plants. For this reason, it is important to highlight that the identification process of this compound extends beyond mere concordance of its ion in negative ESI mode with existing literature and the close correspondence of its experimental molecular weight with the theoretical value, showcasing a remarkably low mass error of just 2.56 ppm. Our identification is further bolstered by the ESI-MS/MS spectrum (Fig. S16†), which reveals molecular ion fragments that support our identification. It is noteworthy that Caroxylon volkensii belongs to the Amaranthaceae family, which encompasses former Chenopodiaceae members, including halophytes and Salsola species recognized for their abundant mineral content, including sulfur.53,54 Other sulfur-containing compounds had been previously reported in Salsola species.44,55 Additionally, halophytes share a high salt tolerance similar to algae,56,57 whereas members of the Chenopodiaceae family are recognized as the most salt-tolerant higher plants.58
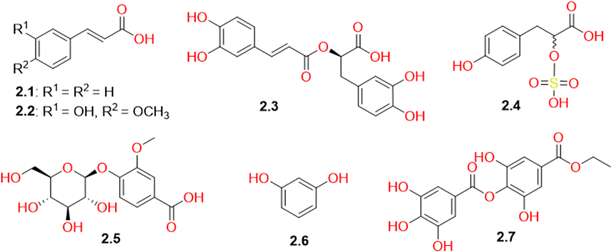 |
| Fig. 3 Structures of phenolic acids and simple phenols (2.1-2.7) identified in Caroxylon volkensii. | |
3.4. Triterpenoids and their derivatives
CVM was found to be rich in triterpenes (Table S1† and Fig. 4). Only one triterpene of the ursane nucleus, named 2α,3β,23,24-tetrahydroxyurs-12-en-28-oic acid (3.1), was detected, while triterpenes of the oleanane skeleton were found to be more predominant. They included free oleanolic acid (3.2) as well as its combination derivatives with different sugar units of variable length. Among these derivatives, salsoloside C (3.4) and salsoloside E (3.5) were present in high concentrations in CVM, as indicated by their high peak intensities in the TIC (negative mode, Fig. S3†). They had previously been isolated from different Salsola species.59–62 Compound 3.7, with a molecular ion peak [M − H]− at m/z 955.492, also appeared in a high concentration. It was tentatively identified as β-D-glucopyranosiduronic acid, (3β)-28-(β-D-glucopyranosyloxy)-28-oxoolean-12-en-3-yl 3-O-β-D-galactopyranosyl (3.7.1) or β-D-glucopyranosiduronic acid, (2β,3β)-28-(β-D-glucopyranosyloxy)-2-hydroxy-28-oxoolean-12-en-3-yl 3-O-(6-deoxy-α-L-mannopyranosyl) (3.7.2), previously reported in the plant family Amaranthaceae.63,64
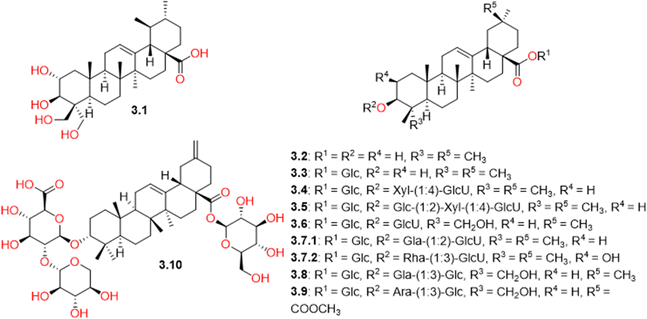 |
| Fig. 4 Structures of triterpenoids (3.1-3.10) identified in Caroxylon volkensii. | |
3.5. Flavonoids
Several types of flavonoids were detected in CVM. They can be subcategorized into flavones, isoflavones, flavanols, and isoflavanes. The identified flavones were isorhamnetin (4.1) and its glycosidic analogues, including isorhamnetin-3-O-β-D-glucopyranoside (4.2), narcissoside (4.3), isorhamnetin-3-O-β-D-apiofuranosyl(1→2)-O-[α-L-rhamnopyranosyl(1→6)]-β-D-glucopyranoside (4.4), and chrysoeriol-7-O-β-D-glucopyranoside (4.5). Few isoflavones, compounds 4.6-4.8, were tentatively identified (Table S1† and Fig. 5), based on the consistency of their ion peaks to known isoflavones isolated from Salsola somalensis.65,66 However, one flavanol, catechin (4.9), and one isoflavane, salisoflavan (4.10), which had previously been reported in S. imbricata,67,68 were also detected.
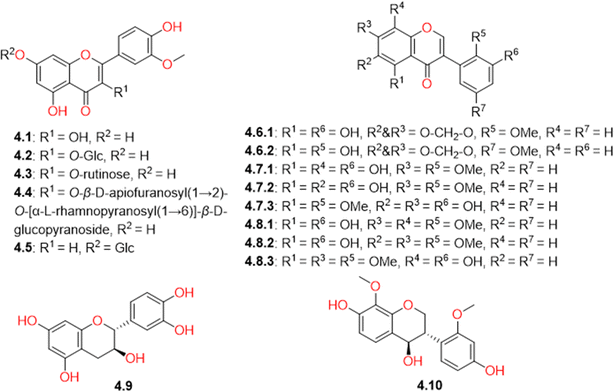 |
| Fig. 5 Structures of flavonoids (4.1-4.10) identified in Caroxylon volkensii. | |
3.6. Steroids
Two steroids (Table S1† and Fig. 6) were detected in CVM. The first steroid is the common campesterol (5.1), which had previously been isolated from S. collina.69 The second is amasterol (5.2), an ecdysteroid isolated before from the roots of Amaranthus viridis, was reported to exhibit a growth inhibitory activity against lettuce seedlings and to possess antifungal activity against the pathogen Helminthosporium oryzi.70
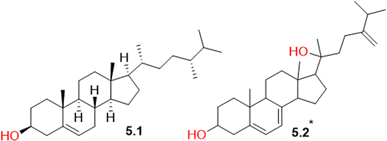 |
| Fig. 6 Structures of steroids (5.1 and 5.2) identified in Caroxylon volkensii. *Stereochemistry is undefined. | |
3.7. Miscellaneous compounds
Other secondary metabolites biosynthesized from mixed pathways, including the malonate, isoprenoid, and shikimate pathways, were detected in CVM and categorized as miscellaneous compounds (Table S1† and Fig. 7). They included the fatty acid component, 9,12,13-trihydroxyoctadeca-10(E),15(Z)-dienoic acid (6.1), which had previously been isolated from Salsola tetrandra.71 Plastoquinone 3 (6.2), a chloroplast component involved in photosynthesis, was also detected. It had previously been isolated from the plant Spinacia oleracea, belonging to the family Amaranthaceae.72 Also, N-cyclohexanecarbonylpentadecylamine (6.3), previously detected in Senna siamea pods73 and Polyporus umbellatus,74 was identified for the first time in the family Amaranthaceae. Its identification was supported by the MS/MS fragmentation spectrum (Fig. S18†) as evident from the pseudo molecular ion peak [M + H]+ corresponding to palmitamide at m/z 256.26349. This compound showed high peak intensity in the TIC (positive mode) of the CVM (Fig. S1†). Compound 6.3 had previously shown a selective inhibition of the acidamidase hydrolyzing N-acylethanolamines.75 Several isoprenoid derivatives (6.4-6.8) were also identified in CVM. They included the sesquiterpene glucoside, amarantholidoside IV (6.4), which had previously been isolated from Amaranthus retroflexus,76 in addition to two monoterpenes, viz. carvone (6.5) and chenopanone (6.6), and two sesquiterpenes, namely chenopodiol (6.7) and 6,11-dihydroxy-3-eudesmen-2-one (6.8).
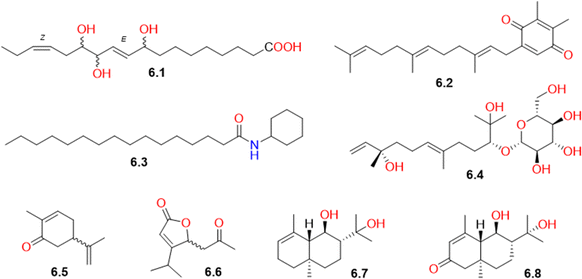 |
| Fig. 7 Structures of biosynthetically miscellaneous compounds (6.1-6.8) identified in Caroxylon volkensii. | |
3.8. Biological activity
Pseudomonas aeruginosa plays a significant role in causing severe and life-threatening infections, particularly in individuals with compromised immune systems, such as those with cancer or AIDS. Numerous isolates of P. aeruginosa exhibit the ability to form biofilms, rendering them more resistant to existing antibiotics.77 Thus, it is crucial to explore alternative approaches to the conventional use of antibiotics. Plants serve as a natural reservoir of numerous bioactive compounds with a wide range of biological activities. Researchers worldwide have extensively explored the antibacterial properties of plant extracts against isolates of P. aeruginosa.26,77–80 Herein, we present the antibacterial, anti-biofilm, and anti-quorum sensing potentials of CVM against P. aeruginosa isolates.
3.9. In vitro antibacterial activity
The preliminary screening of the antibacterial activity of CVM was conducted using the agar well diffusion method. Remarkably, it demonstrated the presence of an inhibition zone around the wells, signifying its antibacterial potential against the tested isolates. MIC values were established through the broth microdilution method, revealing that the MIC values of CVM against P. aeruginosa isolates varied from 32 to 256 μg mL−1, as detailed in Table 1.
Table 1 Values of the minimum inhibitory concentrations (MICs) of Caroxylon volkensii against the tested isolates
Isolate code |
MIC (μg mL−1) |
Isolate code |
MIC (μg mL−1) |
P1 |
32 |
P13 |
128 |
P2 |
32 |
P14 |
256 |
P3 |
256 |
P15 |
128 |
P4 |
64 |
P16 |
32 |
P5 |
128 |
P17 |
32 |
P6 |
32 |
P18 |
64 |
P7 |
32 |
P19 |
256 |
P8 |
128 |
P20 |
128 |
P9 |
256 |
P21 |
128 |
P10 |
32 |
P22 |
64 |
P11 |
64 |
P23 |
32 |
P12 |
64 |
3.10. Anti-biofilm and anti-quorum sensing activities
Bacterial virulence quenching is a crucial tactic that has proven to be highly effective in reducing the dissemination of bacterial resistance.81 This strategy is able to reduce the pathogenesis of the pathogenic bacteria, thus helping the host immune system to eradicate bacteria, and avoid the development of resistance. Besides, there is an important advantage to such an approach as it does not affect the normal flora of the host.82,83 Biofilm is a pivotal target for many antivirulence compounds as biofilm eradication can affect disease progression.80 Thus, we investigated the impact of CVM on the biofilm forming ability of the tested isolates using the crystal violet assay as shown in Table 2. CVM demonstrated the ability to hinder biofilm formation in the tested isolates, leading to a reduction in the percentage of isolates classified as strong and moderate biofilm formers from 47.83% to 17.39%.
Table 2 Impact of Caroxylon volkensii on the biofilm forming ability of P. aeruginosa isolates
Biofilm formationa |
Before treatment |
After treatment |
NBP: non-biofilm producer (ODc < OD < 2 ODc), WBP: weak biofilm producer (2 ODc < OD < 4 ODc), MBP: moderate biofilm producer (4 ODc < OD < 6 ODc), SBP: strong biofilm producer (6 ODc < OD). |
Non-biofilm producer (NBP) |
8 |
11 |
Weak biofilm producer (WBP) |
4 |
8 |
Moderate biofilm producer (MBP) |
6 |
3 |
Strong biofilm producer (SBP) |
5 |
1 |
Moreover, the quorum quenching potential of CVM was elucidated on Chromobacterium violaceum standard isolate. C. violaceum is frequently used in microbiology labs as a bacterial model possessing a QS system. The impact of potential quorum quenching compounds is examined by monitoring the production of its violacein pigment. This pigment has a violet color and its production is regulated by the QS system of C. violaceum.84 Interestingly, CVM revealed a concentration-dependent inhibitory activity on violacein production as shown in Fig. 8, and this finding can be correlated with its anti-biofilm activity.
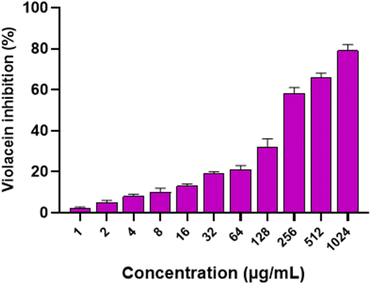 |
| Fig. 8 Bar chart illustrating the concentration-dependent inhibitory impact of Caroxylon volkensii on the production of violacein pigment by C. violaceum (ATCC 12472). | |
3.11. In vivo antibacterial activity
We elucidated the in vivo protective effect of CVM to assess its antibacterial activity in vivo. Interestingly, CVM significantly (p < 0.05) diminished the killing ability of the treated isolates in comparison with the non-treated ones. The survival curve for the three examined groups was graphed, as depicted in Fig. 9. In group I, all mice survived throughout the entire experiment. In group II, three mice perished after 2 days, another three after 5 days, and the remainder after 8 days. Group III experienced the demise of only one mouse after 6 days, with the remaining mice in group III surviving until the 14th day.
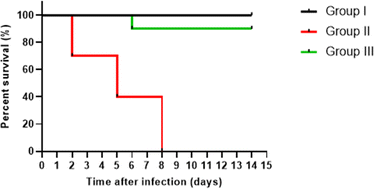 |
| Fig. 9 Kaplan–Meier survival curve of the three groups; group I (non-infected control group, black), group II (infected with P. aeruginosa but untreated with CVM, red), and group III (infected with P. aeruginosa and treated with CVM, green). | |
3.12. Docking study
Molecular docking is a popular computational tool in drug discovery. It is most helpful for estimating the interaction of the ligands with target proteins.85,86 Las is a well-studied quorum system in P. aeruginosa. This system orchestrates the biofilm formation in a sophisticated way to ensure the infection establishment in the host.87 LasR is a transcriptional global activator that regulates the expression of several genes associated with biofilm formation, pathogenicity, and virulence.88–90 LasR must interact with its inducer, the 3-oxo-C12 derivative of acylhomoserine lactone (AHL), synthesized by Acyl-homoserine-lactone synthase (LasI) in order to be activated.91 In the present investigation, we conducted a molecular docking experiment using AutoDock Vina software to investigate the potential secondary metabolites responsible for the anti-quorum sensing activity of CVM through binding to the LasR enzyme. For the docking study, 18 compounds, identified as the main components in CVM by exhibiting the highest peak intensities in the TIC (Fig. S1–S3†) and the highest peak areas as presented in Table S1,† were selected. The peak areas of all the identified compounds, presented in Table S1,† were arranged in descending order, and the first 18 compounds with the highest peak areas were selected for the docking study. Among the tested compounds, tyramine derivatives showed great binding affinity towards LasR (Table 3). Moupinamide (1.22) showed the highest docking score (−11.1 kcal mol−1), with a better binding affinity than the co-crystallized ligand, AHL (−8.723 kcal mol−1). Visualization of the molecular model of binding of moupinamide (1.22) and other tyramine derivatives, compounds 1.23 and 1.25, showed H-bonding with Arg-61, which is important for LasR interaction with its autoinducer.92,93 They also exhibited H-bonding with Tyr-93 and Leu-125 and π–π stacking with the Trp-88 amino acid residue in the ligand-binding domain (LBD) of LasR (Fig. 10 and S20†). Our predicted anti-quorum sensing activity for the identified tyramine derivatives was in accordance with the findings reported by David et al. (2018), which demonstrated tyramine derivatives as quorum sensing inhibitors against Agrobacterium tumefaciens.94 Ethyl-p-digallate (2.7) and isorhamnetin (4.1) also showed remarkable docking scores of −9.75 and −9.9 kcal mol−1, respectively, towards LasR (Table 3). Ethyl-p-digallate (2.7) revealed H-bonding with Arg-61, Tyr-93, Thr-75, and π–π stacking with Trp-88 (Fig. S20†), while isorhamnetin (4.1) showed H-bonding with Arg-61, Trp-60, Thr-115, and π–π stacking with Tyr-64 (Fig. S20†). This result is supported by our previous finding of the anti-quorum sensing activity of gallic acid and the flavonoid compound, catechin.95
Table 3 Docking scores of Caroxylon volkensii secondary metabolites against the transcriptional activator receptor (LasR) using AutoDock Vina 1.2.3
Compound no. |
Peak area |
Name |
Docking score |
1.1 |
2.69 × 107 |
Salsolinol |
−7.439 |
1.2 |
3.06 × 107 |
Salsoline |
−7.605 |
1.7 |
1.46 × 109 |
N-Methyltyramine |
−7.467 |
1.8 |
4.47 × 108 |
Hordenine |
−7.548 |
1.11 |
1.92 × 107 |
Tyramine-O-β-D-glucoside |
−8.204 |
1.22 |
3.33 × 107 |
Moupinamide (N-trans-feruloyltyramine) |
−11.100 |
1.23 |
2.27 × 107 |
(E)-3-(4-Hydroxy-3-methoxyphenyl)-N-(4-methoxyphenethyl)acrylamide |
−9.565 |
1.25 |
1.39 × 107 |
N-trans-feruloyl 3-O-methyldopamine |
−9.762 |
1.29 |
5.23 × 107 |
Allantoic acid ethyl ester |
−6.673 |
2.4 |
6.46 × 107 |
Tichocarpol A |
−8.437 |
2.7 |
2.36 × 107 |
Ethyl-p-digallate |
−9.755 |
3.4 |
4.78 × 107 |
Salsoloside C |
−8.450 |
3.5 |
2.32 × 107 |
Salsoloside E |
−8.052 |
4.1 |
1.72 × 107 |
Isorhamnetin |
−9.923 |
4.3 |
4.71 × 107 |
Isorhamnetin-3-O-rutinoside (narcissin) |
−7.169 |
6.1 |
1.38 × 107 |
9,12,13-Trihydroxyoctadeca-10(E),15(Z)-dienoic acid |
−8.101 |
6.2 |
2.19 × 108 |
Plastoquinone 3 |
−7.180 |
6.3 |
1.17 × 109 |
N-Cyclohexanecarbonylpentadecylamine |
−6.845 |
Co-crystallized ligand |
— |
3-Oxo-C12 acylhomoserine lactone (AHL) |
−8.723 |
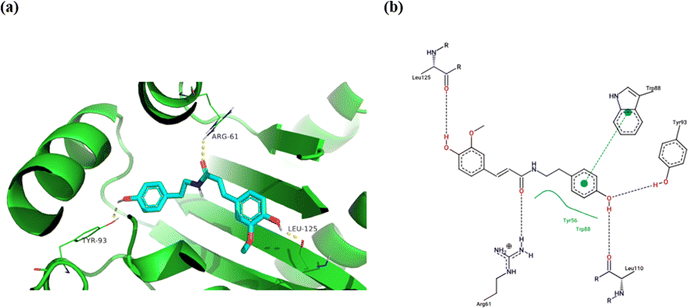 |
| Fig. 10 Molecular binding of moupinamide (1.22) to the transcriptional activator receptor (LasR, PDB code: 2UV0); (a) 3D molecular binding model, (b) 2D molecular binding model demonstrating LasR amino acid residues involved in the interaction. | |
It is important to highlight that tyramine conjugates, which are predicted to be responsible for the anti-quorum sensing potential of CVM, are reported to be present in numerous plant genera.96 They are documented to contribute to the growth, development, and defence mechanisms of several plants.96 The activity of the enzyme responsible for the biosynthesis of moupinamide (feruloyltyramine, 1.22) was reported to be increased by 5–8 fold after the infection of Nicotiana tabacum with the tobacco mosaic virus.97 In recent years, there has been growing interest in tyramine conjugates with phenolic acids, extending from their role in plants to their biological activities and applications in the food industry.46,96,98 They have been documented to exhibit antioxidant, anti-cancer, anti-inflammatory, anti-diabetic, and neuroprotective activities.96 Moreover, N-trans-feruloyltyramine has been reported to exert anti-bacterial activity against Staphylococcus aureus with a MIC value of 7.8 mg mL−1, in comparison to the positive control, tetracyline (125 μg mL−1),99 which supports the findings of our docking study. However, additional, in-depth studies are necessary to validate this finding. Many researchers have studied the mass production of tyramine conjugates using both chemical synthesis and bioengineering approaches.96 The maximum yield (96.3%) for producing N-trans-feruloyltyramine (1.22) from ferulic acid and tyramine hydrochloride was attained through a green one-step lipase-catalyzed synthesis.96,98 The high production yield of tyramine conjugates encourages further exploration of their antimicrobial activity to be used as potential novel alternatives to traditionally used antibiotics.
4. Conclusion
In the current study, the bioactive components of C. volkensii have been investigated for the first time, using LC-QTOF-MS/MS analysis. This study has revealed the great chemo-diversity of the plant. It was found to be rich in catecholamines, tyramine derivatives, phenolic acids, triterpenoids, and flavonoids. The obtained results can be most helpful for understanding the potential medicinal applications of C. volkensii and its phylogenetic relationship to other plant species in the Old-World Salsola and the family Amaranthaceae. Our study has shown that C. volkensii could be a potential therapeutic candidate for P. aeruginosa infections owing to its anti-biofilm and quorum-quenching potential. Through a molecular docking study, tyramine conjugates, ethyl-p-digallate (2.7), and isorhamnetin (4.1) were predicted to be responsible for the anti-quorum sensing inhibitory activity of CVM through binding to the transcriptional global activator, LasR. They can be considered as novel alternatives or adjuncts to the traditionally utilized antibiotics, which suffer from the spread of resistance. Therefore, additional in vitro and in vivo in-depth studies are necessary to validate these findings. Moreover, further future studies are suggested to elucidate their anti-quorum sensing activity against other bacterial species.
Author contributions
Conceptualization, M. H. E., F. M. A. B., E. E.; methodology, M. H. E., U. R. A., A. A. K., E. E.; investigation, M. H. E., U. R. A., F. M. A. B., G. B., A. A. K., E. E.; data curation, M. H. E., U. R. A.; writing original draft, M. H. E., E. E.; revising and editing the manuscript, M. H. E., U. R. A., F. M. A. B., G. B., A. A. K., E. E.
Conflicts of interest
There are no conflicts to declare.
Notes and references
- M. T. Khayat, H. A. Abbas, T. S. Ibrahim, A. N. Khayyat, M. Alharbi, K. M. Darwish, S. S. Elhady, E.-S. Khafagy, M. K. Safo and W. A. Hegazy, Biomedicines, 2022, 10, 1169 CrossRef CAS PubMed.
- M. Almukainzi, T. A. El-Masry, W. A. Negm, E. Elekhnawy, A. Saleh, A. E. Sayed, M. A. Khattab and D. H. Abdelkader, Int. J. Nanomed., 2022, 17, 1203 CrossRef CAS PubMed.
- K. S. Ikuta, L. R. Swetschinski, G. R. Aguilar, F. Sharara, T. Mestrovic, A. P. Gray, N. D. Weaver, E. E. Wool, C. Han and A. G. Hayoon, Lancet, 2022, 400, 2221–2248 CrossRef PubMed.
- M. B. Miller and B. L. Bassler, Annu. Rev. Microbiol., 2001, 55, 165–199 CrossRef CAS PubMed.
- D. Reynolds and M. Kollef, Drugs, 2021, 81, 2117–2131 CrossRef CAS PubMed.
- Z. Pang, R. Raudonis, B. R. Glick, T.-J. Lin and Z. Cheng, Biotechnol. Adv., 2019, 37, 177–192 CrossRef CAS PubMed.
- A. Kariminik, M. Baseri-Salehi and B. Kheirkhah, Immunol. Lett., 2017, 190, 1–6 CrossRef CAS PubMed.
- M. Shaaban, A. Elgaml and E.-S. E. Habib, Microb. Pathog., 2019, 127, 138–143 CrossRef CAS PubMed.
- R. S. Smith and B. H. Iglewski, J. Clin. Invest., 2003, 112, 1460–1465 CrossRef CAS PubMed.
- L. M. Castronovo, A. Vassallo, A. Mengoni, E. Miceli, P. Bogani, F. Firenzuoli, R. Fani and V. Maggini, Pathogens, 2021, 10, 106 CrossRef CAS PubMed.
- R. Perumal Samy and P. Gopalakrishnakone, Evidence-Based Complementary Altern. Med., 2010, 7, 283–294 CrossRef PubMed.
- J.-L. Rios and M. C. Recio, J. Ethnopharmacol., 2005, 100, 80–84 CrossRef CAS PubMed.
- H. M. A. AlSheikh, I. Sultan, V. Kumar, I. A. Rather, H. Al-Sheikh, A. Tasleem Jan and Q. M. R. Haq, Antibiotics, 2020, 9, 480 CrossRef PubMed.
- B. Khameneh, M. Iranshahy, V. Soheili and B. S. Fazly Bazzaz, Antimicrob. Resist. Infect. Control, 2019, 8, 1–28 CrossRef PubMed.
- M. H. ElNaggar, W. M. Eldehna, M. A. Abourehab and F. M. Abdel Bar, J. Enzyme Inhib. Med. Chem., 2022, 37, 2036–2062 CrossRef CAS PubMed.
- H. Akhani, G. Edwards and E. H. Roalson, Int. J. Plant Sci., 2007, 168, 931–956 CrossRef CAS.
- T. Feodorova, Ukr. Bot. Zh., 2015, 72, 442–445 CrossRef.
- L. Boulos, Flora of Egypt, Al Hadara Publishing, Cairo, Egypt, 1999 Search PubMed.
- S. M. Nofal, S. Nada, N. S. Hassan, M. A. Abdel-Alim and F. S. El-Sharabasy, Egypt. Med. JNRC, 2002, 1, 115–139 Search PubMed.
- J. Hubert, J.-M. Nuzillard and J.-H. Renault, Phytochem. Rev., 2017, 16, 55–95 CrossRef CAS.
- M. Azizah, P. Pripdeevech, T. Thongkongkaew, C. Mahidol, S. Ruchirawat and P. Kittakoop, Antibiotics, 2020, 9, 606 CrossRef CAS PubMed.
- T. Ito and M. Masubuchi, J. Antibiot., 2014, 67, 353–360 CrossRef CAS PubMed.
- B. Alotaibi, T. A. El-Masry, E. Elekhnawy, A. H. El-Kadem, A. Saleh, W. A. Negm and D. H. Abdelkader, Drug Delivery, 2022, 29, 1848–1862 CrossRef CAS PubMed.
- M. J. Alqahtani, E. Elekhnawy, W. A. Negm, S. Mahgoub and I. A. Hussein, J. Fungi, 2022, 8, 521 CrossRef CAS PubMed.
- W. A. Negm, M. El-Aasr, G. Attia, M. J. Alqahtani, R. I. Yassien, A. Abo Kamer and E. Elekhnawy, J. Fungi, 2022, 8, 426 CrossRef CAS PubMed.
- E. Elekhnawy, W. A. Negm, M. El-Aasr, A. A. Kamer, M. Alqarni, G. E.-S. Batiha, A. J. Obaidullah and H. M. Fawzy, Sci. Rep., 2022, 12, 1–15 CrossRef PubMed.
- N. G. Attallah, F. A. Mokhtar, E. Elekhnawy, S. Z. Heneidy, E. Ahmed, S. Magdeldin, W. A. Negm and A. H. El-Kadem, Pharmaceuticals, 2022, 15, 549 CrossRef CAS PubMed.
- O. Trott and A. J. Olson, J. Comput. Chem., 2010, 31, 455–461 CrossRef CAS PubMed.
- J. Eberhardt, D. Santos-Martins, A. F. Tillack and S. Forli, J. Chem. Inf. Model., 2021, 61, 3891–3898 CrossRef CAS PubMed.
- H. L. Ammon, S. M. Prasad, D. M. Barnhart, V. K. Syal, K. El-Sayed and G. M. Wassel, Acta Crystallogr., Sect. C, 1987, 43, 567–570 CrossRef.
- N. Proskurnina and A. Orekhov, Bull. Soc. Chim. Fr., Mem., 1937, 4, 1265–1271 CAS.
- A. P. Orekhov and N. Proskurnina, Khim.-Farm. Prom-st., 1934, 2, 8–10 Search PubMed.
- M. Boulaaba, F. Medini, H. Hajlaoui, K. Mkadmini, H. Falleh, R. Ksouri, H. Isoda, A. Smaoui and C. Abdelly, S. Afr. J. Bot., 2019, 123, 193–199 CrossRef CAS.
- R. Tundis, F. Menichini, F. Conforti, M. R. Loizzo, M. Bonesi, G. Statti and F. Menichini, J. Enzyme Inhib. Med. Chem., 2009, 24, 818–824 CrossRef CAS PubMed.
- Y. Zhao and X. Ding, Yaoxue Xuebao, 2004, 39, 598–600 CAS.
- U. Pässler and H.-J. Knölker, Alkaloids: Chem. Biol., 2011, 70, 79–151 Search PubMed.
- Y.-S. Jin, J.-L. Du, Y. Yang, L. Jin, Y. Song, W. Zhang and H.-S. Chen, Chem. Nat. Compd., 2011, 47, 257–260 CrossRef CAS.
- D. M. Rasheed, S. M. El Zalabani, M. A. Koheil, H. M. El-Hefnawy and M. A. Farag, Nat. Prod. Res., 2013, 27, 2320–2327 CrossRef CAS PubMed.
- Y. Xiang, Y.-B. Li, J. Zhang, P. Li and Y.-Z. Yao, Yaoxue Xuebao = Acta Pharm. Sin., 2007, 42, 618–620 CAS.
- D. S. Wishart, A. Guo, E. Oler, F. Wang, A. Anjum, H. Peters, R. Dizon, Z. Sayeeda, S. Tian, B. L. Lee, M. Berjanskii, R. Mah, M. Yamamoto, J. Jovel, C. Torres-Calzada, M. Hiebert-Giesbrecht, V. W. Lui, D. Varshavi, D. Varshavi, D. Allen, D. Arndt, N. Khetarpal, A. Sivakumaran, K. Harford, S. Sanford, K. Yee, X. Cao, Z. Budinski, J. Liigand, L. Zhang, J. Zheng, R. Mandal, N. Karu, M. Dambrova, H. B. Schiöth, R. Greiner and V. Gautam, Nucleic Acids Res., 2022, 50, D622–D631 CrossRef CAS PubMed.
- D. Ly, K. Kang, J.-Y. Choi, A. Ishihara, K. Back and S.-G. Lee, J. Med. Food, 2008, 11, 385–389 CrossRef CAS PubMed.
- A. Kulma and J. Szopa, Plant Sci., 2007, 172, 433–440 CrossRef CAS.
- L. Servillo, D. Castaldo, A. Giovane, R. Casale, N. D'Onofrio, D. Cautela and M. L. Balestrieri, J. Agric. Food Chem., 2017, 65, 892–899 CrossRef CAS PubMed.
- S. S. Murshid, D. Atoum, D. R. Abou-Hussein, H. M. Abdallah, R. H. Hareeri, H. Almukadi and R. Edrada-Ebel, Plants, 2022, 11, 714 CrossRef CAS PubMed.
- J.-J. Cheng, T.-H. Tsai and L.-C. Lin, Planta Med., 2012, 1873–1877 CAS.
- E. Frerot, N. Neirynck, I. Cayeux, Y. H.-J. Yuan and Y.-M. Yuan, J. Agric. Food Chem., 2015, 63, 7161–7168 CrossRef CAS.
- K. M. Khan, G. M. Maharvi, A. Abbaskhan, S. Hayat, M. T. H. Khan, T. Makhmoor, M. I. Choudhary, F. Shaheen and A. ur-rahman, Helv. Chim. Acta, 2003, 86, 457–464 CrossRef CAS.
- F. Cutillo, B. D'Abrosca, M. DellaGreca, C. Di Marino, A. Golino, L. Previtera and A. Zarrelli, Phytochem., 2003, 64, 1381–1387 CrossRef CAS PubMed.
- P.-L. Wu, G.-C. Su and T.-S. Wu, J. Nat. Prod., 2003, 66, 996–998 CrossRef CAS PubMed.
- I. Sadowska-Bartosz and G. Bartosz, Molecules, 2021, 26, 2520 CrossRef CAS PubMed.
- N. Shaheen, Y. Lu, P. Geng, Q. Shao and Y. Wei, J. Chromatogr. B, 2017, 1046, 211–217 CrossRef CAS PubMed.
- T. Ishii, T. Okino, M. Suzuki and Y. Machiguchi, J. Nat. Prod., 2004, 67, 1764–1766 CrossRef CAS PubMed.
- V. Altay and M. Ozturk, The Genera Salsola and Suaeda (Amaranthaceae) and Their Value as Fodder, Springer, 2020 Search PubMed.
- T. Al-Ani, I. Habib, A. Abdulaziz and N. Ouda, Plant Soil, 1971, 35, 29–36 CrossRef CAS.
- R. K. Suleiman, S. A. Umoren, W. Iali and B. El Ali, ACS Omega, 2022, 7, 20332–20338 CrossRef CAS PubMed.
- J. Rozema and H. Schat, Environ. Exp. Bot., 2013, 92, 83–95 CrossRef CAS.
- C. M. Messina, G. Renda, V. A. Laudicella, R. Trepos, M. Fauchon, C. Hellio and A. Santulli, Int. J. Mol. Sci., 2019, 20, 881 CrossRef CAS PubMed.
- J. Gorham, Mechanisms of salt tolerance of halophytes in Halophytes and biosaline agriculture, CRC Press, 1st edn, 1995 Search PubMed.
- C. Annaev, M. Isamukhamedova and N. Abubakirov, Chem. Nat. Compd., 1983, 19, 691–695 CrossRef.
- I. E. Orhan, N. Kucukboyaci, I. Calis, J. P. Ceron-Carrasco, H. den-Haan, J. Pena-Garcia and H. Perez-Sanchez, Phytochem. Lett., 2017, 20, 373–378 CrossRef CAS.
- N. Kucukboyaci, I. Süntar and I. Calis, Rec. Nat. Prod., 2016, 10, 369–379 CAS.
- A. M. Iannuzzi, R. Moschini, M. De Leo, C. Pineschi, F. Balestri, M. Cappiello, A. Braca and A. Del-Corso, Food Biosci., 2020, 37, 100713 CrossRef CAS.
- N. Nkobole and G. Prinsloo, Molecules, 2021, 26, 795 CrossRef CAS PubMed.
- M. Junkuszew, W. Oleszek, M. Jurzysta, S. Piancente and C. Pizza, Phytochem., 1998, 49, 195–198 CrossRef CAS.
- B. M. Abegaz and Y. Woldu, Phytochem., 1991, 30, 1281–1284 CrossRef CAS.
- Y. Woldu and B. Abegaz, Phytochem., 1990, 29, 2013–2015 CrossRef CAS.
- M. Saleem, N. Akhter, M. Shaiq Ali, M. Nazir, N. Riaz, M. Moazzam, M. Arshad and A. Jabbar, Magn. Reson. Chem., 2009, 47, 263–265 CrossRef CAS PubMed.
- N. G. Shehab and E. Abu-Gharbieh, J. Evidence-Based Complementary Altern. Med., 2014, 2014, 695291 Search PubMed.
- T. I. Mayakova, V. G. Leont'eva, T. I. Zharkaya, A. A. Semenov, E. E. Kuznetsova and S. P. Chupin, Khim. Prir. Soedin., 1984, 4, 531–532 Search PubMed.
- S. Roy, A. Dutta and D. Chakraborty, Phytochem., 1982, 21, 2417–2420 CrossRef CAS.
- M. H. Oueslati, H. Ben Jannet, Z. Mighri, J. Chriaa and P. M. Abreu, J. Nat. Prod., 2006, 69, 1366–1369 CrossRef CAS.
- D. Misiti, H. W. Moore and K. Folkers, J. Am. Chem. Soc., 1965, 87, 1402–1403 CrossRef CAS.
- S. H. Patil, D. D. Kurlapkar and D. K. Gaikwad, Open Access Libr. J., 2020, 7, 1–11 Search PubMed.
- G.-K. Liu, N. Li, Y.-J. Zhang and J.-R. Wang, Microchem. J., 2019, 144, 351–360 CrossRef CAS.
- K. Tsuboi, C. Hilligsmann, S. Vandevoorde, D. M. Lambert and N. Ueda, Biochem. J., 2004, 379, 99–106 CrossRef CAS PubMed.
- A. Fiorentino, M. DellaGreca, B. D'Abrosca, A. Golino, S. Pacifico, A. Izzo and P. Monaco, Tetrahedron, 2006, 62, 8952–8958 CrossRef CAS.
- K. Alam, D. A. Al Farraj, S. Mah-e-Fatima, M. A. Yameen, M. S. Elshikh, R. M. Alkufeidy, A. E.-Z. M. Mustafa, P. Bhasme, M. K. Alshammari and N. A. Alkubaisi, J. Infect. Public Health, 2020, 13, 1734–1741 CrossRef PubMed.
- F. M. Husain, I. Ahmad, A. S. Al-Thubiani, H. H. Abulreesh, I. M. AlHazza and F. Aqil, Front. Microbiol., 2017, 8, 727 CrossRef PubMed.
- M. Mombeshora and S. Mukanganyama, BMC Complementary Altern. Med., 2019, 19, 1–12 CrossRef PubMed.
- A. Das, M. C. Das, P. Sandhu, N. Das, P. Tribedi, U. C. De, Y. Akhter and S. Bhattacharjee, RSC Adv., 2017, 7, 5497–5513 RSC.
- B. Alotaibi, W. A. Negm, E. Elekhnawy, T. A. El-Masry, M. E. Elharty, A. Saleh, D. H. Abdelkader and F. A. Mokhtar, Artif. Cells, Nanomed., Biotechnol., 2022, 50, 96–106 CrossRef CAS PubMed.
- C. A. Ford, I. M. Hurford and J. E. Cassat, Front. Microbiol., 2021, 11, 632706 CrossRef PubMed.
- N. G. Attallah, S. A. El-Sherbeni, A. H. El-Kadem, E. Elekhnawy, T. A. El-Masry, E. I. Elmongy, N. Altwaijry and W. A. Negm, Molecules, 2022, 27, 1329 CrossRef CAS PubMed.
- S. Mion, N. Carriot, J. Lopez, L. Plener, A. Ortalo-Magné, E. Chabrière, G. Culioli and D. Daudé, npj Biofilms Microbiomes, 2021, 7, 1–16 CrossRef PubMed.
- F. Stanzione, I. Giangreco and J. C. Cole, Prog. Med. Chem., 2021, 60, 273–343 CrossRef PubMed.
- A. Sethi, K. Joshi, K. Sasikala and M. Alvala, Drug Discovery Dev. New Adv., 2019, 2, 1–21 Search PubMed.
- T. Pacheco, A. É. I. Gomes, N. M. G. Siqueira, L. Assoni, M. Darrieux, H. Venter and L. F. C. Ferraz, Front. Microbiol., 2021, 12, 597735 CrossRef PubMed.
- F. F. Tuon, L. R. Dantas, P. H. Suss and V. S. Tasca Ribeiro, Pathogens, 2022, 11, 300 CrossRef CAS PubMed.
- M. V. Turkina and E. Vikström, J. Innate Immun., 2019, 11, 263–279 CrossRef CAS PubMed.
- M. J. Gambello, S. Kaye and B. H. Iglewski, Infect. Immun., 1993, 61, 1180–1184 CrossRef CAS PubMed.
- T. A. Gould, H. P. Schweizer and M. E. Churchill, Mol. Microbiol., 2004, 53, 1135–1146 CrossRef CAS PubMed.
- N. Chowdhury and A. Bagchi, Gene, 2016, 580, 80–87 CrossRef CAS PubMed.
- J. P. Gerdt, C. E. McInnis, T. L. Schell and H. E. Blackwell, Org. Biomol. Chem., 2015, 13, 1453–1462 RSC.
- S. David, A. Mandabi, S. Uzi, A. Aharoni and M. M. Meijler, ACS Chem. Biol., 2018, 13, 247–252 CrossRef CAS PubMed.
- F. M. Abdel Bar, M. A. Alossaimi, E. Elekhnawy, M. A. A. Alzeer, A. Abo Kamer, E. Moglad and M. H. ElNaggar, Molecules, 2022, 27, 7841 CrossRef CAS PubMed.
- W. Leonard, P. Zhang, D. Ying and Z. Fang, Crit. Rev. Food Sci. Nutr., 2022, 62, 1608–1625 CrossRef CAS PubMed.
- J. Negrel and C. Martin, Phytochem., 1984, 23, 2797–2801 CrossRef CAS.
- M. Basri, M. A. Alrub, E. A. Malek, S. Ainliah, A. B. Salleh and M. B. Abdul Rahman, Chem. Eng. Commun., 2014, 201, 1582–1592 CrossRef CAS.
- F. Samita, C. O. Ochieng, P. O. Owuor, L. O. A. Manguro and J. O. Midiwo, Nat. Prod. Res., 2017, 31, 529–536 CrossRef CAS PubMed.
|
This journal is © The Royal Society of Chemistry 2024 |
Click here to see how this site uses Cookies. View our privacy policy here.