DOI:
10.1039/D4BM00005F
(Review Article)
Biomater. Sci., 2024,
12, 3321-3334
Artificial keloid skin models: understanding the pathophysiological mechanisms and application in therapeutic studies
Received
2nd January 2024
, Accepted 12th May 2024
First published on 18th May 2024
Abstract
Keloid is a type of scar formed by the overexpression of extracellular matrix substances from fibroblasts following inflammation after trauma. The existing keloid treatment methods include drug injection, surgical intervention, light exposure, cryotherapy, etc. However, these methods have limitations such as recurrence, low treatment efficacy, and side effects. Consequently, studies are being conducted on the treatment of keloids from the perspective of inflammatory mechanisms. In this study, keloid models are created to understand inflammatory mechanisms and explore treatment methods to address them. While previous studies have used animal models with gene mutations, chemical treatments, and keloid tissue transplantation, there are limitations in fully reproducing the characteristics of keloids unique to humans, and ethical issues related to animal welfare pose additional challenges. Consequently, studies are underway to create in vitro artificial skin models to simulate keloid disease and apply them to the development of treatments for skin diseases. In particular, herein, scaffold technologies that implement three-dimensional (3D) full-thickness keloid models are introduced to enhance mechanical properties as well as biological properties of tissues, such as cell proliferation, differentiation, and cellular interactions. It is anticipated that applying these technologies to the production of artificial skin for keloid simulation could contribute to the development of inflammatory keloid treatment techniques in the future.
1 Introduction
Keloid is a scar that is harder and thicker than other scars, induced by the overexpression and deposition of extra-cellular matrix components (ECMs) such as collagen resulting from the overproliferation of myofibroblasts through the differentiation of fibroblasts.1 It has been known to be caused by infection at the wound region after injury.2 During wound recovery, inflammatory programs influence fibroblasts directly or indirectly through various inflammatory cells or cytokines such as toll-like receptors (TLR); pro-inflammatory factors, such as interleukin-6 (IL-6), IL-8, and Monocyte Chemoattractant Protein-1 (MCP-1); anti-inflammatory factors such as IL-10; and inflammatory cells such as macrophages, mast cells, lymphocytes, and neutrophils.1 These fibroblasts, influenced by inflammatory mechanisms, are differentiated into myofibroblasts, inducing an excessive expression and secretion of ECMs (Fig. 1). Consequently, the resulting scars become abnormally large and hard, particularly along the border of the wound.1,3 Therefore, the keloid scar is larger and thinner than healthy scars, and it contains a higher amount of moisture and water-soluble collagen, influencing collagen synthesis and degradation of collagen cross-linking, than those in healthy skin, indicating over-synthesis of collagen on the keloid scar.1,4–7
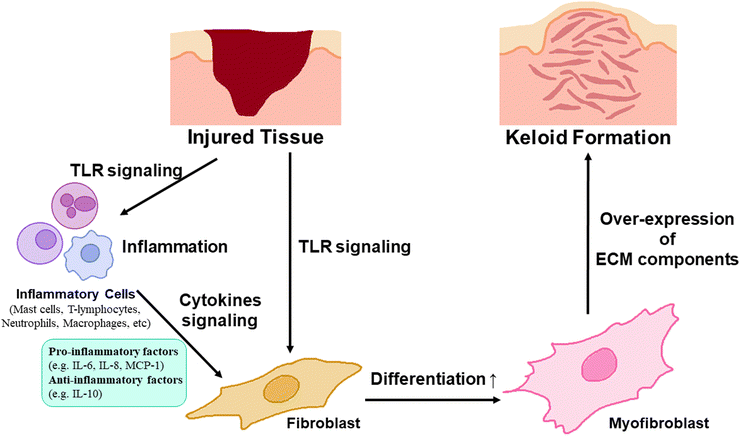 |
| Fig. 1 Inflammatory Mechanisms of Keloid Disease Onset. After injury at the skin tissue, infection occurs at the wound region and inflammation program is processed through various paths such as stimulating Toll-like receptors (TLRs) expressed on inflammatory cells (mast cells, T-lymphocytes, neutrophils, macrophages, etc.) or fibroblast. It activates fibroblast by inducing differentiation to myofibroblast through directly TLR signalling to fibroblast or cytokine signaling of up-regulated inflammatory cytokines such as pro-inflammatory factors (IL-6, IL-8, MCP-1, etc.) or anti-inflammatory factors (IL-10, etc.) from inflammatory cells. Differentiated myofibroblast promotes excessive expression of ECM components, deposition of them and further formation of keloid scars. | |
Therefore, keloid scars are enlarged, harder, and darker than normal scars, accompanied by an aesthetic problem, itching, and movement discomfort in the keloid scar region.8,9 To resolve these issues, various therapeutic methods including surgery, radiation therapy, steroid injection, pressure therapy, cryotherapy, and laser therapy have been used. These methods were applied both independently and in combination as follows.10
First, steroids are commonly administered through intralesional injections. Corticosteroids are primarily used for keloid prevention or treatment. It has been reported to suppress inflammation and has a therapeutic effect on 50–100% of keloid scars.11 It is known to exert therapeutic effects on more than 80% of keloid scars, accompanied by other methods such as surgical excision. However, possible adverse side effects include hypopigmentation, skin and subcutaneous fat atrophy, telangiectasias, necrosis, ulcerations, and Cushingoid habitus.12 To minimize these adverse factors, attempts have been made to adjust steroid dosage depending on the scar area.13
Also, silicone gel sheeting on scars has been reported to improve scar color and softness in existing scars but causes side effects such as itching and skin rashes.14
In order to solve the limitation of the therapeutic effects of corticosteroid injection or the silicone gel method, surgical excision has also been used as a second-line treatment to remove keloid scars.15 It has been reported to reduce the recurrence rate to <50% after other treatments, such as steroid administration or silicone gel treatment.8 However, stimulating the region of excision can promote collagen synthesis, resulting in additional collagen deposition and scar enlargement. Therefore, intramarginal excision should be performed to prevent collagen stimulation.16,17
For relieving some features of keloid, such as pigmentation or thickness, especially shown as side effects of the above treatment methods, pressure earrings are used to suppress keloid tissues after excision by continuously applying pressure. It has been known to notably relieve vascularity, pigmentation and thickness by controlling molecular-biological modulators, such as Matrix metalloproteinase-28 (MMP-28),18,19 a regulator protein for keloid formation,20 or IL-1β, accompanied by dermal apoptosis.21 However, it has been reported that this method requires at least 12 hours per day for several months for effective keloid scar suppression.22,23 Thus, the method of intermittently applying pressure has been investigated to offer convenience to patients using pressure-earring therapy by repeating the application-resting cycles.24
Meanwhile, to selectively treat the targeted components of scar tissues, laser-scar interactions, such as hemoglobin, water, melanin, and collagen, have been used.25 Pulsed–dye laser (PDL) and intense pulsed light (IPL) are used for targeting hemoglobin and treating erythema, pruritus, hyperpigmentation, and dyschromia.26–29 CO2 laser systems are commonly used for drug delivery because they influence the dermis through microchannels.30 Laser treatment has excellent therapeutic effects, primarily on keloids; however, it has high recurrence rates.31 Therefore, a combination of multiple lasers or superficial cryotherapy is required for the effective therapeutic effects of laser treatment.32
In cryotherapy, liquid nitrogen is applied to destroy pathological cells in the dermis, resulting in the destruction of keloid scar tissue.33,34 In particular, intralesional cryosurgery allows the needle to be inserted into the dermis and the application of liquid nitrogen directly to the keloid tissue.33 Improved penetration of the dermis occurs compared with previous cryosurgery methods such as contact cryosurgery/spray cryosurgery.35,36 Additionally, this method significantly reduces scar volume, deformity, hardness, darkness, tenderness, itching, and aesthetic discomfort.37 Despite these advantages, the intralesional cryosurgery method has been reported to cause mild local edema and epidermolysis.33,36
In addition, radiotherapy has been used to inhibit angiogenesis, reduce fibroblast proliferation, and reduce collagen deposition.38 In particular, radiotherapy following excision has shown good efficiency rates; however, it has severe adverse effects, such as malignant changes or carcinogenesis.31 Therefore, the dose and size of the radiation field should be controlled to reduce the dose to any non-target tissues and prevent undesired side effects, such as carcinoma.39
In this way, conventional keloid treatment methods focus on inflammation relief and tissue interaction, such as inhibiting cell proliferation, inhibiting fibrous material accumulation, removing keloid scars, or exerting effects on scar hardness, fibrous material, and color alleviation. Nevertheless, despite these efforts, there are limitations and side effects associated with each method, including pigmentary changes, necrosis, ulcerations, rashes, concurrent surgical interventions, keloid expansion due to wound site extension, prolonged wearing of devices, recurrence, and carcinogenesis. Therefore, to address these challenges, an inflammatory approach to keloid occurrence and treatment is necessary.
To understand the inflammatory pathway of keloids and explore therapeutic alternatives to overcome the limitations of previous keloid therapeutic techniques, a keloid model based on a pathological approach for keloids has been investigated.40 In this review, the current status and future perspectives of the development of an artificial keloid skin model are introduced, and the model mimics the native keloid complex structures by inducing inflammatory factors in animal equivalent models, developing keloid-specific fibrosis by promoting the differentiation of fibroblasts into myofibroblasts through inflammatory activation for ECM overexpression and accumulation,41,42 and transplanting/implanting cells and tissues derived from keloids or artificial scaffolds mimicking keloid tissue structure into animal models,43,44 and further in vitro establishing of layered skin structures with promising scaffold engineering techniques, and finely describing the microenvironment and tissue structure specific to humans, crucial for cell–cell communication, cell proliferation, migration, and differentiation, and possibility of clinical applications for resolving the limitation of animal equivalent models, such as animal ethics and cost issues (Fig. 2).45–51
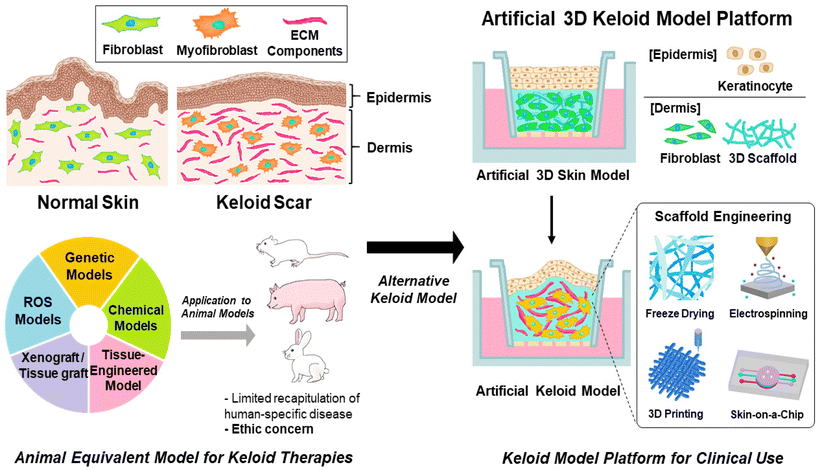 |
| Fig. 2 The keloid-specific skin model developments based on pathological approaches for understanding the inflammatory pathway of keloid and development of clinical applications. (Left) Animal equivalent keloid-induced models mimicking native keloid structure through genetic-mutated, ROS-induced, chemical-induced, and humanized approaches such as xenograft/tissue graft transplantation or artificially engineered tissue implantation. There have been limitations such as completely duplicating human specific properties of keloid for these models, and ethical issues for using animal experiment. (Right) Alternative artificial 3-dimensional (3D) keloid models for recapulating human-specific keloid complex structures. Artificial 3D keloid model consists of full thickness skin layers: epidermis layer and dermis layer. The fabrication of the complex structure of dermis layer has been developed through various scaffold engineering strategies such as freeze drying, electrospinning, 3D printing, skin-on-a chip, etc. | |
2 Animal model equivalents
In clinical approaches to keloid research, the construction of artificial keloids has been explored using several techniques such as genetic mutation, chemical treatment, and implantation of keloid tissue in animal models (Fig. 3).41–44
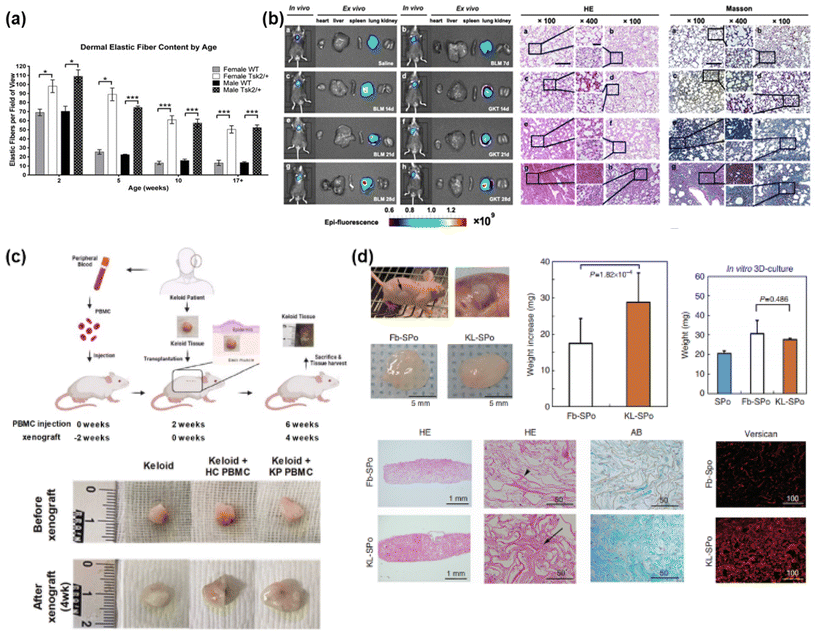 |
| Fig. 3 Construction of keloid/fibrosis models through animal model equivalents. (a) Genetic mutation models. Tight skin 2 (Tsk2), as the main inflammatory gene for fibrosis, has been used for genetic mutation, expressing fibrosis in animal models. Reprinted with permission from ref. 41; Copyright 2014, Elsevier. (b) Chemical-induced models (reactive oxygen species (ROS) through bleomycin-induced fibrosis). Bleomycin has been treated into animal model for promoting fibrosis through expressing pro-inflammatory cytokines and inflammatory cells, and inducing ROS, which have been known to induce fibrosis. Reprinted with permission from ref. 42; Copyright 2021 American Chemical Society. (c) Direct tissue xenograft models. Human keloid skin tissue has been transplanted into immune-deficient animal model, and maintained for several weeks to months through cell–cell interaction in both epithelial layers and dermal layers, and further angiogenesis and collagen production in comparable animal model. Reprinted with permission from ref. 43; Copyright 2023, Springer Nature. This article is licensed under a Creative Commons Attribution 4.0 International License. (d) Engineered tissue-implanted Models. Artificially engineered keloid tissue has been fabricated through 3D scaffold technique using human-derived fibroblast, and implanted into immunocompromised animal model, promoting ECM component deposition. Reprinted with permission from ref. 44; Copyright 2020, MDPI. | |
2.1 Genetic models
Through the mutation of inflammatory genes for the expression of fibrosis, a phenomenon of keloids, a genetic animal model has been introduced and studied (Fig. 3(a)).41 Genetic mutations are applied to construct genetic animal models for keloids by inducing inflammatory ECM overexpression and accumulation through inflammatory gene mutations that trigger fibrosis. The main genes for fibrosis are tight skin 1 and 2 (Tsk1/2), which are used for a genetic mutation in mice as a common animal model.52,53 Then, the Tsk1-positive mouse group duplicated the fibrillin-1 gene on mouse chromosome 2.52 In addition, in the Tsk2-positive mice administered ethylnitrosourea as a mutagenic agent, gain-of-function missense mutations were expressed in Collagen Type III Alpha 1 Chain (Col3a1) on chromosome 1.53,54 The resulting fibrosis of both Tsk mutation models showed tight scars, thickened dermis, and abnormal dermal structural components, with approximately 5–6 weeks of wound recovery, a delayed period compared with 2–3 weeks in the wild-type groups, and granulation tissue on the surrounding wound edge.41,55 However, neither model completely duplicates specific fibrotic diseases such as keloids.40
2.2 Trauma-induced animal models
Keloids are trauma-induced scars; therefore, trauma-induced animal models have been investigated using fibroproliferative scarring through excision and wound healing in pigs,56–58 rabbits59–62 and horses.63,64 However, it is yet to be shown that this model, using other animal models, does not completely duplicate the native keloid-specific characteristics in humans, with an insufficient degree of disfigurement and contractures,57,58 thickened, haphazardly arranged collagenous bundles,56,62,65 despite the proliferation of fibroblasts and minimal vascularity in the dermis layer, as well as increased amounts of randomly arranged collagen.63
2.3 Chemical treatment models
2.3.1. Bleomycin.
Bleomycin is a glycopeptide antibiotic used for anticancer therapy and is known to cause fibrosis in various diseases through up-regulating inflammatory cytokines and reactive oxygen species (ROS), promoting inflammatory myofibroblast differentiation and fibrosis. Therefore, it has been used as a chemical model for fibrosis or keloid (Fig. 3(b)).42,66–69 Bleomycin has been widely used to induce fibrosis in various organs through injection into animal models, expressing not only many pro-inflammatory cytokines, such as IL-1β, IL-4, IL-6, and C–X–C motif chemokine ligand 2 (CXCL2), in the skin in mice but also many inflammatory cells, including cluster of differentiation 45+ (CD45+) leukocytes, F4/80+ macrophages, CD3+ T lymphocytes, mast cells, and α-smooth muscle actin (αSMA) positive myofibroblasts with higher numbers in the skin of bleomycin-treated animals.67,68,70 The resulting collagen deposition was shown to be 2–3 fold higher in dermal thickness and hydroxyproline content than in the control group.67,68,70,71 Despite of visible fibrosis, many other factors related to abnormal scarring, such as hypoxia, genetic susceptibility, and trauma, were not observed in this model. Additionally, the resulting fibrosis was self-limiting for less than 6 weeks after the cessation of bleomycin treatment.66 In addition, the model does not completely reflect the pathogenic characteristics of any disease.66,67,71
2.3.2. Reactive oxygen species model.
Reactive oxygen species (ROS)-inducing substances, such as hypochlorous acid (HOCl), hydrogen peroxide (H2O2), peroxynitrites (ONOO−), and superoxide anions (O2−), have been known to induce fibrosis from fibroblasts in dermal tissues.72–74 Induced ROS are known to promote fibroblast proliferation by elevating the circulating concentrations of anti-DNA topoisomerase 1 and anti-centromere protein-B (anti-CENP-B) antibodies. Meanwhile, transforming growth factor-β (TGF-β) has not been shown to have a significant role in the ROS model because its expression has not been detected.72 The fibrotic pathways in this model are independent of classical fibrotic signaling cascades. Therefore, a pathological keloid approach should be investigated.40
2.4 Humanized animal model equivalents
2.4.1. Direct xenograft/tissue graft.
This method involves the transplantation of human keloid skin tissues into animal models. To construct a keloid-specific fibrosis model based on pathological aspects, human keloid tissue is transplanted into immuno-deficient animal models to establish a graft model for keloid with a comparable environment (Fig. 3(c)).43 Animal models have been used in immune-deficient states to prevent immune rejection responses between implanted human tissues and animal models during tissue grafting.75,76 It has the following advantages: a comparable animal model for the specific human tissue in vivo environment, preservation of complex human cell–cell interactions in both the epidermal and dermal layers, and continuous growth of cells such as fibroblasts in a 3-dimensional organotypic environment.40 The viability of keloid xenografts can be maintained for several weeks to months after implantation or transplantation based on angiogenesis and collagen production in the dermal layer.76–78
However, for several months post-xenograft, most groups lost more than 50% of their weight, and ECM production decreased.76,78,79 Moreover, although an immune-deficient model was used, the immune system was not completely absent, including functioning natural killer cells with humoral adaptive immune systems. Additionally, graft rejection can occur through the remaining immune cells or antigenicity of the skin tissue. This can influence the viability of xenografts and limit the effectiveness of the model. Universal acceptance of the graft model as a model for keloid pathology is limited.78,80
2.4.2. Tissue-engineered models.
It was constructed by implanting an artificially engineered keloid skin tissue into an animal model. The introduction of scaffold engineering allows for the refinement of the microstructure and cellular interactions of keloid tissue for the construction of a keloid-specific fibrosis model (Fig. 3(d)).44 During the preparation of artificial keloid tissue, fibroblasts are isolated from human keloid tissue and cultured in 3D scaffolding materials as in vitro models or implanted in immunocompromised mice as in vivo models.81–83 Matrigel and collagen were used as scaffolding materials. In such materials, keloid fibroblasts maintain an elevated expression of collagen I monoclonal antibody (Col-1), MMP-9, and MMP-13.84 The fibroblast-containing scaffolds maintained their weight post-implantation and histologically demonstrated increased collagen deposition, mostly type I collagen, within the scaffold matrix, with significant neovascularization in the host animal.82 Despite of various animal equivalent keloid models, the challenge of the complete duplication of human-specific properties in keloids remains. Additionally, most animal tests have limitations such as high cost, time-consuming processes, ethical concerns, and immune rejection.50,51 To resolve these issues, a non-animal keloid model was investigated.
3 Non-animal keloid model
3.1 2D (mono- and co-culture) models
A two-dimensional (2D) keloid model typically consists of a separated single layer of keloid-derived fibroblasts or keratinocytes through mono- or co-cellular culture (Fig. 4(a)). The advantages of 2D models are their simple process, high reproducibility and cost-effectiveness because the culture period is short.85 However, cells grown in 2D monolayers cannot capture the relevant complexity of in vivo-environment interactions between an individual cell, its immediate neighbors, and the ECM, which are responsible for the control of cell behavior.86 Therefore, 2D culture models have limitations in achieving realistic tissue representations owing to insufficient cellular communication and cell signaling in native whole skin.61,87–89
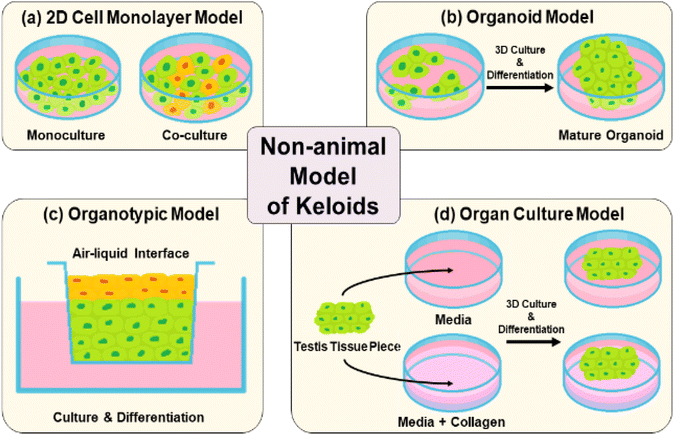 |
| Fig. 4 Non-animal keloid models. (a) Cell monolayer structures through mono cellular cultures or co-cellular cultures using keloid-derived fibroblasts or keratinocytes. (b) Organoid culture models through multicellular aggregation cultures using keloid-derived biopsies. (c) Organotypic models with full-thickness skin structures, consisting of stratified epidermal layers and dermal layers with biological complexity. (d) Organ culture models by embedding harvested keloid tissues through biopsy in medium with/without collagen matrix for culture and differentiation of both epidermal and dermal layers. | |
3.2 3D (organoid and organotypic) culture models
Organoid culture models are prepared by the formation of multicellular aggregates through cultured keloid-derived biopsies in suspension (Fig. 4(b)).90,91 They have the advantages of medium-throughput testing for keloid therapies and simple culture conditions.92 In contrast, organotypic models, which are full-thickness skin structures, consist of stratified layers of epidermal and dermal-derived cells (Fig. 4(c)). They show relative biological complexity, longevity in culture, and similarities in stratification and cell components to human skin. However, short-term use is a major problem. In organotypic culture models, multiple skin cell types are absent during multicellular interplay. They usually lack structural complexity and mimic the human dermis.75,77,79,93–95
3.3 Organ-culture
Organ culture is performed by harvesting tissue from a keloid patient through biopsy, followed by embedding in a collagen matrix and culturing in a medium for the growth of epidermal and dermal layers of the skin (Fig. 4(d)).96 This model has a long-term pathological state, as well as therapeutic effects.96,97 However, it also has limitations, such as complex and relatively expensive medium components, which are essential for organ culture growth.67,68,71,98 Owing to the drawbacks of previous non-animal models, the overall duplication of native keloid skin structure and time-cost-effective alternatives have been further explored.
4 Artificial keloid skin model platform
4.1 3D multi-layered keloid models
Artificial 3D keloid skin models, with full-thickness multi-layered structures, are introduced to overcome the limitations of the previous keloid models related to multicellular interplay as well as structural complexity. By mimicking the actual skin structure, these models allow in vitro studies, thereby avoiding ethical concerns related to animal use. Additionally, they contribute to time and cost savings in research. For these models, various types of cells, such as keloid fibroblasts, keratinocytes, and ECM scaffold components have been employed. Dermal cells, such as keloid fibroblast cells, are seeded with a scaffold material in the lower layer to form a 3D structure.99 The epidermal layers were seeded on the top for cultivation and differentiation to corneal layers at the air–liquid phase (Fig. 5(a)).100,101 This method achieves a multilayered structure, allowing bidirectional growth with cell–cell and cell–ECM communication and interaction. This enabled the activation of growth factors. Owing to its similarity to the actual skin tissue structure, this model is applicable to therapeutic efficacy experiments.45,46
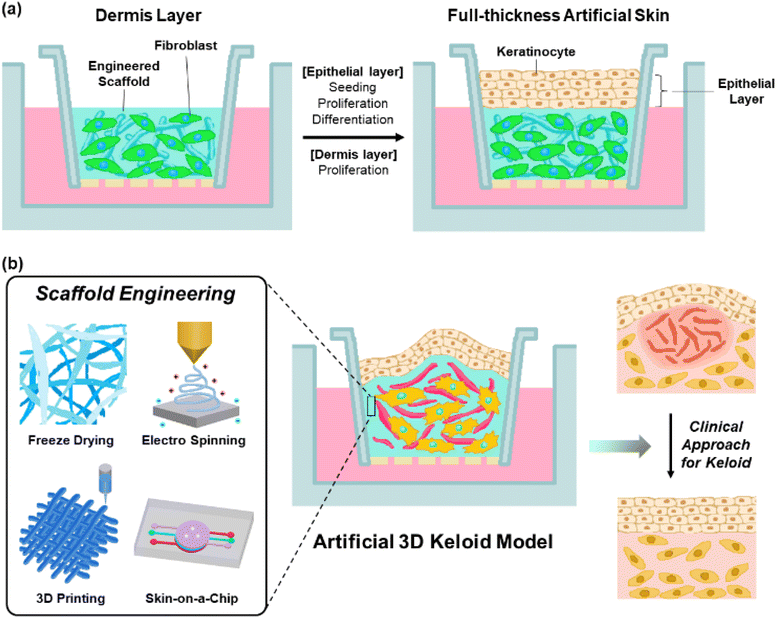 |
| Fig. 5
In vitro artificial full-thickness keloid skin model platform with full-thickness 3D structures. (a) Construction of artificial 3D skin models with multi-layer structure consisting of various cells such as keloid-derived fibroblast and keratinocyte, and scaffolds for 3D keloid model, with cell–cell and cell–ECM communication and interaction. (b) Promising scaffold engineering for complete native keloid-skin model/pathological mechanism-mimic 3D keloid structure models (freeze-drying, 3D printing, electrospinning and skin-on-a-chip), and application of skin model for clinical approaches for keloid. Scaffold engineering would be important for cell adhesion and growth through controlling pore sizes, and duplicating the native matrix complex structure. | |
Scaffold materials, including biocompatible materials such as gelatin, hyaluronic acid, and collagen, allow the dermal layer to provide elastic and tensile properties to the skin structure through the formation of collagen and elastic fiber networks,102–104 support the cells in tissue structures,47 and control tissue segregation and intracellular communication through signaling processes involving growth factors and/or enzymes.48,105 These functions are essential for cell proliferation and differentiation.49 These materials possess biodegradability, low immunogenicity, and high water retention.106 When creating scaffold structures using these materials, adjusting the pore size promotes cell regeneration and facilitates the formation of intricate structures.107,108 Additionally, the precise control of pore size enhances cell attachment and proliferation over a large surface area.109 Especially, collagen and elastin are commonly used as scaffold materials for keloid skin models.110–113 Collagen, the most common connective tissue material, promotes cell adhesion, constructs tissue architecture, and activates platelets,114 with excellent biodegradability, high tensile strength in cartilage and tendons, mechanical strength, low antigenicity, cell-binding capability, and stratified squamous epithelium.115,116 Meanwhile, elastin influences the physical and biological characteristics of tissue functions by exhibiting low stiffness, high extensibility, and effective elastic properties.117–119
4.2 Future perspectives of scaffold engineering for complete 3D keloid structure model
To create an artificial keloid skin that faithfully mimics the actual keloid skin structure, inflammatory mechanisms as well as microenvironment, composed of various types of cells such as fibroblast, myofibroblast, keloid-derived stem cells, macrophages, mast cells and neutrophils,120 with multiple activated signaling pathways, such as TGF-β/Smad, YAP/TAZ, α1β1 or αvβ3, it is particularly crucial to reproduce cell proliferation, differentiation, migration, collagen synthesis, expression of profibrotic genes, keloid-relative receptor expression and cell–cell communication.121–125 In controlling these aspects, matrix scaffolds play a significant role in contributing to cell attachment, support, and behaviour. Therefore, by applying scaffold formation and structure refinement technologies, adjusting pore sizes, mimicking the actual matrix structure, and optimizing physiological conditions within the body, it is possible to advance technologies that not only replicate the structure but also reproduce the functions of keloid skin. Additionally, tensile properties of the scaffold have a significant role in controlling cell proliferation and ECM component synthesis by regulating the expression of keloid-relative proteins such as integrin αvβ3 and α2β1.125,126 Recent studies have reported various techniques for constructing artificial skin models, such as electrospinning, 3D printing, and microfluidic systems, as skin-on-a-chip systems, realizing the intricate structure of human skin (Fig. 5(b), left).127
The freeze-drying technique is known to control pore size at the freezing rate, freezing temperature and pH environment through solvent lyophilization of the frozen polymer solution under high vacuum and low-temperature conditions.128 Therefore, it would be suitable for a wide range of biomedical applications, primarily because it allows for exquisitely mimicking actual keloid tissue by reproducing the proliferation and differentiation processes of cells.50 3D printing is a technology that reproduces multilayered skin structures by layer-by-layer deposition of ECM material scaffolds and constituent cells.129,130 This technique not only provides precision and reproducibility but also enables precise control of internal and outer scaffold structures,131,132 such as the shape, arrangement, size, and structure of pores.133 Therefore, cell proliferation, differentiation, migration, and ECM substance expression are promoted,134 which would be crucial for replicating the characteristic fibrosis as well as the pathological mechanism in keloid disease. Electrospinning is known to reproduce the resulting fiber structure, similar to the actual ECM fibers, using a strong electric field to draw out a polymer solution into thin, continuous fiber forms. It is applied to cell interactions and tissue fabrication135 controlled by variables such as polymer properties (e.g., viscosity and concentration), electrical conductivity, surface tension, solvent, electric field strength, solution injection rate, temperature, and humidity.136 It allows for the resulting electrospun nanofiber network to enable the achievement of morphological similarity to the native ECM structure with an optimized surface-to-volume ratio and porosity,137,138 allowing for improving cell adhesion and proliferation.139 These advantages of electrospinning would be applied to mimic the interaction between keloid constituent cells and ECM, further extending to replicate the structure and function of tissues by emulating the actual keloid ECM structure. Microfluidic systems, such as the skin-on-a-chip technique, are known to mimic complex skin tissue structures and microenvironments by allowing for dynamic cultivation of internal cells to mimic the physiological characteristics of the tissue.140 Additionally, it imparts physical and chemical stimuli to the tissues within the chip, allowing control over micro-environmental factors such as flow, force, and chemical gradients,141–146 influencing cell differentiation, cell interactions, and cell morphology.147 Meanwhile, the application of porous materials that separate microchannels has led to research on tissue barrier functions and tissue interactions,144,148 with each layer separated by porous membranes,149,150 which would be suitable for realistic keloid models.
3D skin model platform technology developed through scaffold engineering would hold significance in mimicking pathological paths based on multiple cellular signal pathways through optimizing porous structures as well as tensile properties of artificially engineered scaffolds and reproducing dermal and epidermal architectures of keloid tissues. Its application in replicating keloid skin structures can be extended to foundational clinical research for keloid.
4.3 Limitation and future perspective of clinical approach for 3D keloid model
Artificial skin models have been widely applied in basic clinical research to develop therapeutic systems for various skin diseases or wounds (Fig. 5(b), right).151,152 With increasing regulations on animal experiments in drug and treatment research, there is growing interest in the development of artificial skin models that mimic the actual skin structure. Thus, research would be conducted on the development of an artificial keloid model that mimics the actual keloid structure for application in the treatment of keloid. However, there has been limited research and therapeutic development using 3D artificial skin models for keloid treatment. Therefore, there is a need for the development of a 3D full-thickness artificial skin platform specifically tailored for keloid studies.
5 Conclusions
Research efforts are underway to artificially develop keloid skin models based on pathophysiological mechanisms for the development of keloid treatment methods. These studies have progressed in terms of reproducing the actual skin structure and function, adhering to animal ethics, and optimizing production time and cost efficiency. In particular, a 3D full-thickness artificial keloid model can be produced in vitro without the need for animal experiments, and its ability to replicate the epidermis, structure, and ECM-based cellular interactions of real skin makes it fascinating. Additionally, engineering studies on scaffolds would be conducted to optimize cell growth, differentiation, and communication through the refinement of the ECM network structures as well as multiple cellular signalling pathways induced by the tensile property of the scaffold. Therefore, the development of an artificial keloid structure that closely mimics the actual skin structure is anticipated, and further applications in the development of treatments for keloid are promising. However, for keloids, more research is needed to mimic the inflammatory processes and unique skin structures over an extended period. Consequently, investigations into keloid treatment using skin disease mimic models are expected to continue in the future.
Author contributions
Jung Y. conceptualized and supervised this work and contributed to writing – original draft, writing – review & editing, funding acquisition and project administration; Kwon S. conceptualized this work, and contributed to writing – original draft, writing – review & editing, investigation, data curation and visualization; Lee J. conceptualized this work, and contributed to writing – original draft, writing – review & editing, investigation, data curation and visualization; Yoo J. conceptualized this work, and contributed to writing – original draft, writing – review & editing, investigation, data curation and visualization. All authors have read and agreed to the published version of the manuscript.
Abbreviations
αSMA | Alpha-smooth muscle actin |
CENP | Centromere protein |
CD | Cluster of differentiation |
Col-1 | Collagen I monoclonal antibody |
Col3a1 | Collagen type III alpha 1 chain |
CXCL | C–X–C motif chemokine ligand |
ECM | Extra-cellular matrix component |
H2O2 | Hydrogen peroxide |
HOCl | Hypochlorous acid |
IPL | Intense pulsed light |
IL | Interleukin |
MMP | Matrix metalloproteinase |
MCP | Monocyte chemoattractant protein |
ONOO− | Peroxynitrites |
PDL | Pulsed-dye laser |
ROS | Reactive oxygen species |
O2− | Superoxide anions |
3D | Three-dimensional |
Tsk1/2 | Tight skin 1 and 2 |
TLR | Toll-like receptors |
TGF | Transforming growth factor |
2D | Two-dimensional |
Conflicts of interest
There are no conflicts to declare.
Acknowledgements
This research was supported by the National Research Foundation of Korea (NRF) grant funded by the Korea government (MSIT) (No. 2021R1A2C2004634), the Korean Fund for Regenerative Medicine (KFRM) grant funded by the Korea government (the Ministry of Science and ICT, the Ministry of Health & Welfare) (21A0504L1-12).
References
- Z. C. Wang, W. Y. Zhao, Y. Cao, Y. Q. Liu, Q. Sun and P. Shi,
et al., The Roles of Inflammation in Keloid and Hypertrophic Scars, Front. Immunol., 2020, 11, 603187 CrossRef CAS PubMed.
- S. T. O'Sullivan, M. O'Shaughnessy and T. P. O'Connor, Aetiology and management of hypertrophic scars and keloids, Ann. R. Coll. Surg. Engl., 1996, 78(3 (Pt 1)), 168–175 Search PubMed.
- G. P. Wittenberg, B. G. Fabian, J. L. Bogomilsky, L. R. Schultz, E. J. Rudner and M. L. Chaffins,
et al., Prospective, single-blind, randomized, controlled study to assess the efficacy of the 585 nm flashlamp-pumped pulsed-dye laser and silicone gel sheeting in hypertrophic scar treatment, Arch. Dermatol., 1999, 135(9), 1049–1055 CAS.
- F. B. Niessen, P. H. M. Spauwen, P. H. Robinson, V. Fidler and M. Kon, The use of silicone occlusive sheeting (Sil-K) and silicone occlusive gel (epiderm) in the prevention of hypertrophic scar formation, Plast. Reconstr. Surg., 1998, 102(6), 1962–1972 CrossRef CAS PubMed.
-
M. H. Beers, R. Berkow, R. M. Bogin, A. J. Fletcher, B. A. Nace and D. E. Moy, et al., The Merck manual of diagnosis and therapy, Whitehouse Station, NJ, Merck, 1999, Available from: https://www.merck.com/pubs/mmanual/ Search PubMed.
- L. O'Brien and D. J. Jones, Silicone gel sheeting for preventing and treating hypertrophic and keloid scars, Cochrane Database Syst. Rev., 2013, 2013(9), CD003826 Search PubMed.
- D. A. Ladin, W. L. Garner and D. J. Smith Jr., Excessive scarring as a consequence of healing, Wound Repair Regen., 1995, 3(1), 6–14 CrossRef CAS PubMed.
- T. A. Mustoe, R. D. Cooter, M. H. Gold, F. D. Hobbs, A. A. Ramelet and P. G. Shakespeare,
et al., International clinical recommendations on scar management, Plast. Reconstr. Surg., 2002, 110(2), 560–571 CrossRef PubMed.
- A. P. Trace, C. W. Enos, A. Mantel and V. M. Harvey, Keloids and Hypertrophic Scars: A Spectrum of Clinical Challenges, Am. J. Clin. Dermatol., 2016, 17(3), 201–223 CrossRef PubMed.
- J. J. Shaffer, S. C. Taylor and F. Cook-Bolden, Keloidal scars: A review with a critical look at therapeutic options, J. Am. Acad. Dermatol., 2002, 46(2), S63–S97 CrossRef PubMed.
- F. B. Niessen, P. H. Spauwen, J. Schalkwijk and M. Kon, On the nature of hypertrophic scars and keloids: a review, Plast. Reconstr. Surg., 1999, 104(5), 1435–1458 CrossRef CAS PubMed.
- C. Roques and L. Teot, The use of corticosteroids to treat keloids: a review, Int. J. Lower Extremity Wounds, 2008, 7(3), 137–145 CrossRef PubMed.
- M. A. Darzi, N. A. Chowdri, S. K. Kaul and M. Khan, Evaluation of Various Methods of Treating Keloids and Hypertrophic Scars - a 10-Year Follow-up-Study, Br. J. Plast. Surg., 1992, 45(5), 374–379 CrossRef CAS PubMed.
- L. O'Brien and A. Pandit, Silicon gel sheeting for preventing and treating hypertrophic and keloid scars, Cochrane Database Syst. Rev., 2006,(1), CD003826 Search PubMed.
- G. Juckett and H. Hartman-Adams, Management of Keloids and Hypertrophic Scars, Am. Fam. Physician, 2009, 80(3), 253–260 Search PubMed.
- N. Jumper, R. Paus and A. Bayat, Functional histopathology of keloid disease, Histol. Histopathol., 2015, 30(9), 1033–1057 CAS.
- P. Donkor, Head and neck keloid: treatment by core excision and delayed intralesional injection of steroid, J. Oral Maxillofac. Surg., 2007, 65(7), 1292–1296 CrossRef PubMed.
- C. B. Thompson, Apoptosis in the Pathogenesis and Treatment of Disease, Science, 1995, 267(5203), 1456–1462 CrossRef CAS PubMed.
- Y. Kawamoto, Y. Nakajima and E. Kuranaga, Apoptosis in Cellular Society: Communication between Apoptotic Cells and Their Neighbors, Int. J. Mol. Sci., 2016, 17(12), 2144 CrossRef PubMed.
- F. Reno, M. Sabbatini, M. Stella, G. Magliacani and M. Cannas, Effect of in vitro mechanical compression on Epilysin (matrix metalloproteinase-28) expression in hypertrophic scars, Wound Repair Regen., 2005, 13(3), 255–261 CrossRef PubMed.
- F. Reno, M. Sabbatini, F. Lombardi, M. Stella, C. Pezzuto and G. Magliacani,
et al., In vitro mechanical compression induces apoptosis and regulates cytokines release in hypertrophic scars, Wound Repair Regen., 2003, 11(5), 331–336 CrossRef PubMed.
- T. H. Park, S. W. Seo, J. K. Kim and C. H. Chang, Outcomes of surgical excision with pressure therapy using magnets and identification of risk factors for recurrent keloids, Plast. Reconstr. Surg., 2011, 128(2), 431–439 CrossRef CAS PubMed.
- V. Tanaydin, J. Beugels, A. Piatkowski, C. Colla, E. van den Kerckhove and G. C. Hugenholtz,
et al., Efficacy of custom-made pressure clips for ear keloid treatment after surgical excision, J. Plast. Reconstr. Aesthet. Surg., 2016, 69(1), 115–121 CrossRef CAS PubMed.
- D. Jun, D. Shin, H. Choi and M. Lee, Clinical efficacy of intermittent magnetic pressure therapy for ear keloid treatment after excision, Arch. Craniofac. Surg., 2019, 20(6), 354–360 CrossRef PubMed.
- X. Fu, J. Dong, S. Wang, M. Yan and M. Yao, Advances in the treatment of traumatic scars with laser, intense pulsed light, radiofrequency, and ultrasound, Burns Trauma, 2019, 7, 1 Search PubMed.
- S. R. Tan and W. D. Tope, Pulsed dye laser treatment of rosacea improves erythema, symptomatology, and quality of life, J. Am. Acad. Dermatol., 2004, 51(4), 592–599 CrossRef PubMed.
- R. R. Anderson, M. B. Donelan, C. Hivnor, E. Greeson, E. V. Ross and P. R. Shumaker,
et al., Laser treatment of traumatic scars with an emphasis on ablative fractional laser resurfacing: consensus report, JAMA Dermatol., 2014, 150(2), 187–193 CrossRef PubMed.
- A. C. Issler-Fisher, J. S. Waibel and M. B. Donelan, Laser Modulation of Hypertrophic Scars: Technique and Practice, Clin. Plast. Surg., 2017, 44(4), 757–766 CrossRef PubMed.
- R. E. Eilers Jr., E. V. Ross, J. L. Cohen and A. E. Ortiz, A Combination Approach to Surgical Scars, Dermatol. Surg., 2016, 42(Suppl 2), S150–S156 CrossRef PubMed.
- B. Behera, R. Kumari, D. M. Thappa and M. Malathi, Therapeutic Efficacy of Intralesional Steroid With Carbon Dioxide Laser Versus With Cryotherapy in Treatment of Keloids: A Randomized Controlled Trial, Dermatol. Surg., 2016, 42(10), 1188–1198 CrossRef CAS PubMed.
- G. G. Gauglitz, H. C. Korting, T. Pavicic, T. Ruzicka and M. G. Jeschke, Hypertrophic scarring and keloids: pathomechanisms and current and emerging treatment strategies, Mol. Med., 2011, 17(1–2), 113–125 CAS.
- J. Kim, Y. I. Lee, J. H. Lee, S. H. Oh, S. E. Lee and Y. K. Kim, Successful Treatment of Post-operative Keloid with Combined Cryotherapy and Ablative Fractional CO2 Laser, Med. Lasers, 2020, 9(1), 58–61 CrossRef.
- Y. Har-Shai, E. Dujovny, E. Rohde and C. C. Zouboulis, Effect of skin surface temperature on skin pigmentation during contact and intralesional cryosurgery of keloids (vol 21, pg 191, 2007), J. Eur. Acad. Dermatol. Venereol., 2007, 21(2), 292 CrossRef.
- A. H. Weshahy, Intralesional cryosurgery. A new technique using cryoneedles, J. Dermatol. Surg. Oncol., 1993, 19(2), 123–126 CrossRef CAS PubMed.
- L. Rusciani, A. Paradisi, C. Alfano, S. Chiummariello and A. Rusciani, Cryotherapy in the treatment of keloids, J. Drugs Dermatol., 2006, 5(7), 591–595 CAS.
- Y. Har-Shai, M. Amar and E. Sabo, Intralesional cryotherapy for enhancing the involution of hypertrophic scars and keloids, Plast. Reconstr. Surg., 2003, 111(6), 1841–1852 CrossRef PubMed.
- Y. Har-Shai, W. Brown, D. Labbe, A. Dompmartin, I. Goldine and T. Gil,
et al., Intralesional cryosurgery for the treatment of hypertrophic scars and keloids following aesthetic surgery: the results of a prospective observational study, Int. J. Lower Extremity Wounds, 2008, 7(3), 169–175 CrossRef PubMed.
- H. Willers and K. D. Held, Introduction to clinical radiation biology, Hematol./Oncol. Clin. North Am., 2006, 20(1), 1–24 CrossRef PubMed.
- S. Dzul, H. Jaenisch, C. Nagle, M. Joiner and S. Miller, Radiation induced mucoepidermoid carcinoma of the parotid gland following post-operative radiotherapy to the earlobe for keloid prophylaxis, Ear Nose Throat J., 2022, 1455613221099998 Search PubMed.
- J. Marttala, J. P. Andrews, J. Rosenbloom and J. Uitto, Keloids: Animal models and pathologic equivalents to study tissue fibrosis, Matrix Biol., 2016, 51, 47–54 CrossRef CAS PubMed.
- K. B. Long, C. M. Artlett and E. P. Blankenhorn, Tight skin 2 mice exhibit a novel time line of events leading to increased extracellular matrix deposition and dermal fibrosis, Matrix Biol., 2014, 38, 91–100 CrossRef CAS PubMed.
- X. Y. Song, S. Bai, N. He, R. Wang, Y. L. Xing and C. J. Lv,
et al., Real-Time Evaluation of Hydrogen Peroxide Injuries in Pulmonary Fibrosis Mice Models with a Mitochondria-Targeted Near-Infrared Fluorescent Probe, ACS Sens., 2021, 6(3), 1228–1239 CrossRef CAS PubMed.
- A. R. Lee, S. Y. Lee, J. W. Choi, I. G. Um, H. S. Na and J. H. Lee,
et al., Establishment of a humanized mouse model of keloid diseases following the migration of patient immune cells to the lesion: Patient-derived keloid xenograft (PDKX) model, Exp. Mol. Med., 2023, 55(8), 1713–1719 CrossRef CAS PubMed.
- K. Kim, H. M. Jeon, K. C. Choi and G. Y. Sung, Testing the Effectiveness of Curcuma longa Leaf Extract on a Skin Equivalent Using a Pumpless Skin-on-a-Chip Model, Int. J. Mol. Sci., 2020, 21(11), 3898 CrossRef CAS PubMed.
- D. M. Titmarsh, V. Nurcombe, C. Cheung and S. M. Cool, Vascular Cells and Tissue Constructs Derived from Human Pluripotent Stem Cells for Toxicological Screening, Stem Cells Dev., 2019, 28(20), 1347–1364 CrossRef CAS PubMed.
- D. Herrmann, J. R. Conway, C. Vennin, A. Magenau, W. E. Hughes and J. P. Morton,
et al., Three-dimensional cancer models mimic cell-matrix interactions in the tumour microenvironment, Carcinogenesis, 2014, 35(8), 1671–1679 CrossRef CAS PubMed.
- J. K. Kular, S. Basu and R. I. Sharma, The extracellular matrix: Structure, composition, age-related differences, tools for analysis and applications for tissue engineering, J. Tissue Eng., 2014, 5, 1–17 Search PubMed.
- N. K. Karamanos, A. D. Theocharis, Z. Piperigkou, D. Manou, A. Passi and S. S. Skandalis,
et al., A guide to the composition and functions of the extracellular matrix, FEBS J., 2021, 288(24), 6850–6912 CrossRef CAS PubMed.
- C. A. Brohem, L. B. Cardeal, M. Tiago, M. S. Soengas, S. B. Barros and S. S. Maria-Engler, Artificial skin in perspective: concepts and applications, Pigm. Cell Melanoma Res., 2011, 24(1), 35–50 CrossRef PubMed.
- S. Ahn, H. Yoon, G. Kim, Y. Kim, S. Lee and W. Chun, Designed Three-Dimensional Collagen Scaffolds for Skin Tissue Regeneration, Tissue Eng., Part C, 2010, 16(5), 813–820 CrossRef CAS PubMed.
- R. Cheluvappa, P. Scowen and R. Eri, Ethics of animal research in human disease remediation, its institutional teaching; and alternatives to animal experimentation, Pharmacol. Res. Perspect., 2017, 5(4), e00332 CrossRef PubMed.
- R. M. Baxter, T. P. Crowell, M. E. McCrann, E. M. Frew and H. Gardner, Analysis of the tight skin (/+) mouse as a model for testing antifibrotic agents, Lab. Invest., 2005, 85(10), 1199–1209 CrossRef CAS PubMed.
- P. J. Christner, J. Peters, D. Hawkins, L.
D. Siracusa and S. A. Jimenez, The tight skin 2 mouse. An animal model of scleroderma displaying cutaneous fibrosis and mononuclear cell infiltration, Arthritis Rheum., 1995, 38(12), 1791–1798 CrossRef CAS PubMed.
- K. B. Long, Z. Li, C. M. Burgwin, S. G. Choe, V. Martyanov and S. Sassi-Gaha,
et al., The Tsk2/+ mouse fibrotic phenotype is due to a gain-of-function mutation in the PIIINP segment of the Col3a1 gene, J. Invest. Dermatol., 2015, 135(3), 718–727 CrossRef CAS PubMed.
- K. B. Long, C. M. Burgwin, R. Huneke, C. M. Artlett and E. P. Blankenhorn, Tight Skin 2 Mice Exhibit Delayed Wound Healing Caused by Increased Elastic Fibers in Fibrotic Skin, Adv. Text. Wound Care, 2014, 3(9), 573–581 CrossRef PubMed.
- A. Wells, A. Nuschke and C. C. Yates, Skin tissue repair: Matrix microenvironmental influences, Matrix Biol., 2016, 49, 25–36 CrossRef CAS PubMed.
- N. Harunari, K. Q. Zhu, R. T. Armendariz, H. Deubner, P. Muangman and G. J. Carrougher,
et al., Histology of the thick scar on the female, red Duroc pig: final similarities to human hypertrophic scar, Burns, 2006, 32(6), 669–677 CrossRef PubMed.
- K. Q. Zhu, G. J. Carrougher, N. S. Gibran, F. F. Isik and L. H. Engrav, Review of the female Duroc/Yorkshire pig model of human fibroproliferative scarring, Wound Repair Regen., 2007, 15(Suppl 1), S32–S39 Search PubMed.
- K. Q. Zhu, G. J. Carrougher, O. P. Couture, C. K. Tuggle, N. S. Gibran and L. H. Engrav, Expression of collagen genes in the cones of skin in the Duroc/Yorkshire porcine model of fibroproliferative scarring, J. Burn Care Res., 2008, 29(5), 815–827 CrossRef PubMed.
- J. Joseph and M. Dyson, Tissue replacement in the rabbit's ear, Br. J. Surg., 1966, 53(4), 372–380 CrossRef CAS PubMed.
- D. E. Morris, L. Wu, L. L. Zhao, L. Bolton, S. I. Roth and D. A. Ladin,
et al., Acute and chronic animal models for excessive dermal scarring: quantitative studies, Plast. Reconstr. Surg., 1997, 100(3), 674–681 CrossRef CAS PubMed.
- T. T. Tollefson, F. Kamangar, S. Aminpour, A. Lee, B. Durbin-Johnson and S. Tinling, Comparison of effectiveness of silicone gel sheeting with microporous paper tape in the prevention of hypertrophic scarring in a rabbit model, Arch. Facial Plast. Surg., 2012, 14(1), 45–51 CrossRef PubMed.
- C. L. Theoret, O. O. Olutoye, L. K. Parnell and J. Hicks, Equine exuberant granulation tissue and human keloids: a comparative histopathologic study, Vet. Surg., 2013, 42(7), 783–789 CrossRef PubMed.
- S. Ud-Din, S. W. Volk and A. Bayat, Regenerative healing, scar-free healing and scar formation across the species: current concepts and future perspectives, Exp. Dermatol., 2014, 23(9), 615–619 CrossRef PubMed.
- H. Wang, Z. Chen, X. J. Li, L. Ma and Y. L. Tang, Anti-inflammatory cytokine TSG-6 inhibits hypertrophic scar formation in a rabbit ear model, Eur. J. Pharmacol., 2015, 751, 42–49 CrossRef CAS PubMed.
- V. Della Latta, A. Cecchettini, S. Del Ry and M. A. Morales, Bleomycin in the setting of lung fibrosis induction: From biological mechanisms to counteractions, Pharmacol. Res., 2015, 97, 122–130 CrossRef CAS PubMed.
- T. Yamamoto, S. Takagawa, I. Katayama, K. Yamazaki, Y. Hamazaki and H. Shinkai,
et al., Animal model of sclerotic skin. I: Local injections of bleomycin induce sclerotic skin mimicking scleroderma, J. Invest. Dermatol., 1999, 112(4), 456–462 CrossRef CAS PubMed.
- M. Liang, J. Lv, L. Zou, W. Yang, Y. Xiong and X. Chen,
et al., A modified murine model of systemic sclerosis: bleomycin given by pump infusion induced skin and pulmonary inflammation and fibrosis, Lab. Invest., 2015, 95(3), 342–350 CrossRef CAS PubMed.
- T. Takahashi, Y. Asano, Y. Ichimura, T. Toyama, T. Taniguchi and S. Noda,
et al., Amelioration of tissue fibrosis by toll-like receptor 4 knockout in murine models of systemic sclerosis, Arthritis Rheumatol., 2015, 67(1), 254–265 CrossRef CAS PubMed.
- T. Yamamoto, Y. Takahashi, S. Takagawa, I. Katayama and K. Nishioka, Animal model of sclerotic skin. II. Bleomycin induced scleroderma in genetically mast cell deficient WBB6F1-W/W(V) mice, J. Rheumatol., 1999, 26(12), 2628–2634 CAS.
- A. M. Cameron, D. H. Adams, J. E. Greenwood, P. J. Anderson and A. J. Cowin, A novel murine model of hypertrophic scarring using subcutaneous infusion of bleomycin, Plast. Reconstr. Surg., 2014, 133(1), 69–78 CrossRef CAS PubMed.
- A. Servettaz, C. Goulvestre, N. Kavian, C. Nicco, P. Guilpain and C. Chereau,
et al., Selective oxidation of DNA topoisomerase 1 induces systemic sclerosis in the mouse, J. Immunol., 2009, 182(9), 5855–5864 CrossRef CAS PubMed.
- G. Bagnato, A. Bitto, G. Pizzino, N. Irrera, D. Sangari and M. Cinquegrani,
et al., Simvastatin attenuates the development of pulmonary and cutaneous fibrosis in a murine model of systemic sclerosis, Rheumatology, 2013, 52(8), 1377–1386 CrossRef CAS PubMed.
- F. Batteux, N. Kavian and A. Servettaz, New insights on chemically induced animal models of systemic sclerosis, Curr. Opin. Rheumatol., 2011, 23(6), 511–518 CrossRef CAS PubMed.
- S. A. Estrem, M. Domayer, A. E. Cram and J. Bardach, Implantation of Human Keloid into Athymic Mice, Laryngoscope, 1987, 97(10), 1214–1218 CrossRef CAS PubMed.
- C. W. Kischer, J. Pindur, M. R. Shetlar and C. L. Shetlar, Implants of Hypertrophic Scars and Keloids into the Nude (Athymic) Mouse - Viability and Morphology, J. Trauma, 1989, 29(5), 672–677 CrossRef CAS PubMed.
- C. W. Kischer, D. Sheridan and J. Pindur, Use of nude (athymic) mice for the study of hypertrophic scars and keloids: vascular continuity between mouse and implants, Anat. Rec., 1989, 225(3), 189–196 CrossRef CAS PubMed.
- D. Y. Yang, S. R. Li, J. L. Wu, Y. Q. Chen, G. Li and S. Bi,
et al., Establishment of a hypertrophic scar model by transplanting full-thickness human skin grafts onto the backs of nude mice, Plast. Reconstr. Surg., 2007, 119(1), 104–109 CrossRef CAS PubMed.
- M. R. Shetlar, C. L. Shetlar, C. W. Kischer and J. Pindur, Implants of Keloid and Hypertrophic Scars into the Athymic Nude-Mouse - Changes in the Glycosaminoglycans of the Implants, Connect. Tissue Res., 1991, 26(1–2), 23–36 CrossRef CAS PubMed.
- B. F. Seo, J. Y. Lee and S. N. Jung, Models of abnormal scarring, BioMed Res. Int., 2013, 2013, 423147 Search PubMed.
- Y. Yagi, E. Muroga, M. Naitoh, Z. Isogai, S. Matsui and S. Ikehara,
et al., An ex vivo model employing keloid-derived cell-seeded collagen sponges for therapy development, J. Invest. Dermatol., 2013, 133(2), 386–393 CrossRef CAS PubMed.
- H. Wang and S. Luo, Establishment of an animal model for human keloid scars using tissue engineering method, J. Burn Care Res., 2013, 34(4), 439–446 CrossRef PubMed.
- Y. S. Lee, T. Hsu, W. C. Chiu, H. Sarkozy, D. A. Kulber and A. Choi,
et al., Keloid-derived, plasma/fibrin-based skin equivalents generate de novo dermal and epidermal pathology of keloid fibrosis in a mouse model, Wound Repair Regen., 2016, 24(2), 302–316 CrossRef PubMed.
- H. Li, Z. Nahas, F. Feng, J. H. Elisseeff and K. Boahene, Tissue engineering for in vitro analysis of matrix metalloproteinases in the pathogenesis of keloid lesions, JAMA Facial Plast. Surg., 2013, 15(6), 448–456 CrossRef PubMed.
- E. Sutterby, P. Thurgood, S. Baratchi, K. Khoshmanesh and E. Pirogova, Evaluation of in vitro human skin models for studying effects of external stressors and stimuli
and developing treatment modalities, VIEW, 2021, 3(2), 20210012 CrossRef.
- G. Mazzoleni, D. Di Lorenzo and N. Steimberg, Modelling tissues in 3D: the next future of pharmaco-toxicology and food research?, Genes Nutr., 2009, 4(1), 13–22 CrossRef CAS PubMed.
- A. M. Babar and A. H. Nagi, A New Technique for Development of Rabbit Ear Keloid Model, Pak. J. Zool., 2017, 49(1), 379–382 CrossRef.
- O. Kloeters, A. Tandara and T. A. Mustoe, Hypertrophic scar model in the rabbit ear: a reproducible model for studying scar tissue behavior with new observations on silicone gel sheeting for scar reduction, Wound Repair Regen., 2007, 15(Suppl 1), S40–S45 Search PubMed.
- L. Nabai and A. Ghahary, Hypertrophic Scarring in the Rabbit Ear: A Practical Model for Studying Dermal Fibrosis, Methods Mol. Biol., 2017, 1627, 81–89 CrossRef CAS PubMed.
- W. J. Lee, I. K. Choi, J. H. Lee, Y. O. Kim and C. O. Yun, A novel three-dimensional model system for keloid study: organotypic multicellular scar model, Wound Repair Regen., 2013, 21(1), 155–165 CrossRef PubMed.
- M. A. Lancaster and J. A. Knoblich, Organogenesis in a dish: modeling development and disease using organoid technologies, Science, 2014, 345(6194), 1247125 CrossRef PubMed.
- K. I. Votanopoulos, S. Forsythe, H. Sivakumar, A. Mazzocchi, J. Aleman and L. Miller,
et al., Model of Patient-Specific Immune-Enhanced Organoids for Immunotherapy Screening: Feasibility Study, Ann. Surg. Oncol., 2020, 27(6), 1956–1967 CrossRef PubMed.
- B. Hochman, F. C. Vilas Bôas, M. Mariano and L. M. Ferreiras, Keloid heterograft in the hamster (Mesocricetus auratus) cheek pouch, Brazil, Acta Cir. Bras., 2005, 20(3), 200–212 CrossRef PubMed.
- C. Philandrianos, D. Gonnelli, L. Andrac-Meyer, M. Bruno, G. Magalon and S. Mordon, Establishment of a keloid model by transplanting human keloid onto the backs of nude mice, Ann. Chir. Plast. Esthet., 2014, 59(4), 246–252 CrossRef CAS PubMed.
- M. Polo, Y. J. Kim, A. Kucukcelebi, P. G. Hayward, F. Ko and M. C. Robson, An in vivo model of human proliferative scar, J. Surg. Res., 1998, 74(2), 187–195 CrossRef CAS PubMed.
- R. Bagabir, F. Syed, R. Paus and A. Bayat, Long-term organ culture of keloid disease tissue, Exp. Dermatol., 2012, 21(5), 376–381 CrossRef CAS PubMed.
- G. M. Bran, U. R. Goessler, K. Hormann, F. Riedel and H. Sadick, Keloids: Current concepts of pathogenesis (Review), Int. J. Mol. Med., 2009, 24(3), 283–293 CAS.
- T. Maeda, T. Yamamoto, T. Imamura and R. Tsuboi, Impaired wound healing in bleomycin-induced murine scleroderma: a new model of wound retardation, Arch. Dermatol. Res., 2016, 308(2), 87–94 CrossRef CAS PubMed.
- Q. L. Loh and C. Choong, Three-dimensional scaffolds for tissue engineering applications: role of porosity and pore size, Tissue Eng., Part B, 2013, 19(6), 485–502 CrossRef CAS PubMed.
- M. Ahn, W. W. Cho, W. Park, J. S. Lee, M. J. Choi and Q. Gao,
et al., 3D biofabrication of diseased human skin models in vitro, Biomater. Res., 2023, 27(1), 80 CrossRef PubMed.
- E. Hofmann, A. Schwarz, J. Fink, L. P. Kamolz and P. Kotzbeck, Modelling the Complexity of Human Skin In Vitro, Biomedicines, 2023, 11(3), 794 CrossRef CAS PubMed.
- M. A. Stepp, S. Spurrmichaud, A. Tisdale, J. Elwell and I. K. Gipson, Alpha-6-Beta-4 Integrin Heterodimer Is a Component of Hemidesmosomes, Proc. Natl. Acad. Sci. U. S. A., 1990, 87(22), 8970–8974 CrossRef CAS PubMed.
- S. H. Hussain, B. Limthongkul and T. R. Humphreys, The biomechanical properties of the skin, Dermatol. Surg., 2013, 39(2), 193–203 CrossRef CAS PubMed.
- F. H. Silver, J. W. Freeman and D. DeVore, Viscoelastic properties of human skin and processed dermis, Sking Res. Technol., 2001, 7(1), 18–23 CrossRef CAS PubMed.
- J. Fares, M. Y. Fares, H. H. Khachfe, H. A. Salhab and Y. Fares, Molecular principles of metastasis: a hallmark of cancer revisited, Signal Transduction Targeted Ther., 2020, 5(1), 28 CrossRef PubMed.
- T. Biswal, Biopolymers for tissue engineering applications: A review, Mater. Today: Proc., 2021, 41, 397–402 CAS.
- I. Bruzauskaite, D. Bironaite, E. Bagdonas and E. Bernotiene, Scaffolds and cells for tissue regeneration: different scaffold pore sizes-different cell effects, Cytotechnology, 2016, 68(3), 355–369 CrossRef CAS PubMed.
- J. Liang, P. Liu, X. Yang, L. Liu, Y. Zhang and Q. Wang,
et al., Biomaterial-based scaffolds in promotion of cartilage regeneration: Recent advances and emerging applications, J. Orthop. Translat., 2023, 41, 54–62 CrossRef PubMed.
- C. M. Murphy and F. J. O'Brien, Understanding the effect of mean pore size on cell activity in collagen-glycosaminoglycan scaffolds, Cell Adhes. Migr., 2010, 4(3), 377–381 CrossRef PubMed.
- D. Suttho, S. Mankhetkorn, D. Binda, L. Pazart, P. Humbert and G. Rolin, 3D modeling of keloid scars in vitro by cell and tissue engineering, Arch. Dermatol. Res., 2017, 309(1), 55–62 CrossRef CAS PubMed.
- G. C. Limandjaja, L. J. van den Broek, M. Breetveld, T. Waaijman, S. Monstrey and E. M. de Boer,
et al., Characterization of In Vitro Reconstructed Human Normotrophic, Hypertrophic, and Keloid Scar Models, Tissue Eng., Part C, 2018, 24(4), 242–253 CrossRef CAS PubMed.
- G. C. Limandjaja, L. J. van den Broek, T. Waaijman, M. Breetveld, S. Monstrey and R. J. Scheper,
et al., Reconstructed human keloid models show heterogeneity within keloid scars, Arch. Dermatol. Res., 2018, 310(10), 815–826 CrossRef CAS PubMed.
- T. Waaijman, M. Breetveld, M. Ulrich, E. Middelkoop, R. J. Scheper and S. Gibbs, Use of a Collagen-Elastin Matrix as Transport Carrier System to Transfer Proliferating Epidermal Cells to Human Dermis In Vitro, Cell Transplant., 2010, 19(10), 1339–1348 Search PubMed.
- M. Tzaphlidou, Bone architecture: collagen structure and calcium/phosphorus maps, J. Biol. Phys., 2008, 34(1–2), 39–49 CrossRef CAS PubMed.
- C. S. Sobral, A. Gragnani, X. Cao, J. R. Morgan and L. M. Ferreira, Human keratinocytes cultured on collagen matrix used as an experimental burn model, J. Burns Wounds, 2007, 7, e6 Search PubMed.
- S. Xiong, X. Zhang, P. Lu, Y. Wu, Q. Wang and H. Sun,
et al., A Gelatin-sulfonated Silk Composite Scaffold based on 3D Printing Technology Enhances Skin Regeneration by Stimulating Epidermal Growth and Dermal Neovascularization, Sci. Rep., 2017, 7(1), 4288 CrossRef PubMed.
- J. Gosline, M. Lillie, E. Carrington, P. Guerette, C. Ortlepp and K. Savage, Elastic proteins: biological roles and mechanical properties, Philos. Trans. R. Soc. London, Ser. B, 2002, 357(1418), 121–132 CrossRef CAS PubMed.
- S. M. Mithieux and A. S. Weiss, Elastin, Adv. Protein Chem., 2005, 70, 437–461 CrossRef CAS PubMed.
- L. D. Muiznieks and F. W. Keeley, Molecular assembly and mechanical properties of the extracellular matrix: A fibrous protein perspective, BBA, Mol. Basis Dis., 2013, 1832(7), 866–875 CrossRef CAS PubMed.
- M. Zhang, H. Chen, H. Qian and C. Wang, Characterization of the skin keloid microenvironment, Cell Commun. Signaling, 2023, 21(1), 207 CrossRef CAS PubMed.
- E. J. Macarak, P. J. Wermuth, J. Rosenbloom and J. Uitto, Keloid disorder: Fibroblast differentiation
and gene expression profile in fibrotic skin diseases, Exp. Dermatol., 2021, 30(1), 132–145 CrossRef CAS PubMed.
- S. Chawla and S. Ghosh, Regulation of fibrotic changes by the synergistic effects of cytokines, dimensionality and matrix: Towards the development of an in vitro human dermal hypertrophic scar model, Acta Biomater., 2018, 69, 131–145 CrossRef CAS PubMed.
- J. M. Carthy, TGFbeta signaling and the control of myofibroblast differentiation: Implications for chronic inflammatory disorders, J. Cell. Physiol., 2018, 233(1), 98–106 CrossRef CAS PubMed.
- T. Panciera, L. Azzolin, M. Cordenonsi and S. Piccolo, Mechanobiology of YAP and TAZ in physiology and disease, Nat. Rev. Mol. Cell Biol., 2017, 18(12), 758–770 CrossRef CAS PubMed.
- E. Suarez, F. Syed, T. Alonso-Rasgado, P. Mandal and A. Bayat, Up-regulation of tension-related proteins in keloids: knockdown of Hsp27, alpha2beta1-integrin, and PAI-2 shows convincing reduction of extracellular matrix production, Plast. Reconstr. Surg., 2013, 131(2), 158e–173e CrossRef CAS PubMed.
- H. Song, T. Liu, W. Wang, H. Pang, Z. Zhou and Y. Lv,
et al., Tension enhances cell proliferation and collagen synthesis by upregulating expressions of integrin alphavbeta3 in human keloid-derived mesenchymal stem cells, Life Sci., 2019, 219, 272–282 CrossRef CAS PubMed.
- Y. E. Yun, Y. J. Jung, Y. J. Choi, J. S. Choi and Y. W. Cho, Artificial skin models for animal-free testing, J. Pharm. Invest., 2018, 58, 215–223 CrossRef.
- F. J. O'Brien, B. A. Harley, I. V. Yannas and L. J. Gibson, The effect of pore size on cell adhesion in collagen-GAG scaffolds, Biomaterials, 2005, 26(4), 433–441 CrossRef PubMed.
- W. Lee, J. C. Debasitis, V. K. Lee, J. H. Lee, K. Fischer and K. Edminster,
et al., Multi-layered culture of human skin fibroblasts and keratinocytes through three-dimensional freeform fabrication, Biomaterials, 2009, 30(8), 1587–1595 CrossRef CAS PubMed.
- O. J. Lee, H. W. Ju, J. H. Kim, J. M. Lee, C. S. Ki and J. H. Kim,
et al., Development of artificial dermis using 3D electrospun silk fibroin nanofiber matrix, J. Biomed. Nanotechnol., 2014, 10(7), 1294–1303 CrossRef CAS PubMed.
- L. Koch, S. Kuhn, H. Sorg, M. Gruene, S. Schlie and R. Gaebel,
et al., Laser printing of skin cells and human stem cells, Tissue Eng., Part C, 2010, 16(5), 847–854 CrossRef CAS PubMed.
- L. Koch, A. Deiwick, S. Schlie, S. Michael, M. Gruene and V. Coger,
et al., Skin tissue generation by laser cell printing, Biotechnol. Bioeng., 2012, 109(7), 1855–1863 CrossRef CAS PubMed.
- K. J. De France, F. Xu and T. Hoare, Structured Macroporous Hydrogels: Progress, Challenges, and Opportunities, Adv. Healthc. Mater., 2018, 7(1), 1700927 CrossRef PubMed.
- N. Cubo, M. Garcia, J. F. del Cañizo, D. Velasco and J. L. Jorcano, 3D bioprinting of functional human skin: production and analysis, Biofabrication, 2017, 9(1), 015006 CrossRef PubMed.
- C. E. Ayres, B. S. Jha, S. A. Sell, G. L. Bowlin and D. G. Simpson, Nanotechnology in the design of soft tissue scaffolds: innovations in structure and function, Wiley Interdiscip. Rev.: Nanomed. Nanobiotechnol., 2010, 2(1), 20–34 CAS.
- A. Haider, S. Haider and I. K. Kang, A comprehensive review summarizing the effect of electrospinning parameters and potential applications of nanofibers in biomedical and biotechnology, Arabian J. Chem., 2018, 11(8), 1165–1188 CrossRef CAS.
- J. X. Li, A. H. He, J. F. Zheng and C. C. Han, Gelatin and gelatin-hyaluronic acid nanofibrous membranes produced by electrospinning of their aqueous solutions, Biomacromolecules, 2006, 7(7), 2243–2247 CrossRef CAS PubMed.
- S. P. Miguel, R. S. Sequeira, A. F. Moreira, C. S. D. Cabral, A. G. Mendonca and P. Ferreira,
et al., An overview of electrospun membranes loaded with bioactive molecules for improving the wound healing process, Eur. J. Pharm. Biopharm., 2019, 139, 1–22 CrossRef CAS PubMed.
- S. Zhong, W. E. Teo, X. Zhu, R. W. Beuerman, S. Ramakrishna and L. Y. Yung, An aligned nanofibrous collagen scaffold by electrospinning and its effects on in vitro fibroblast culture, J. Biomed. Mater. Res., Part A, 2006, 79(3), 456–463 CrossRef PubMed.
- S. N. Bhatia and D. E. Ingber, Microfluidic organs-on-chips, Nat. Biotechnol., 2014, 32(8), 760–772 CrossRef CAS PubMed.
- J. H. Sung, M. B. Esch, J. M. Prot, C. J. Long, A. Smith and J. J. Hickman,
et al., Microfabricated mammalian organ systems and their integration into models of whole animals and humans, Lab Chip, 2013, 13(7), 1201–1212 RSC.
- J. Kieninger, A. Weltin, H. Flamm and G. A. Urban, Microsensor systems for cell metabolism - from 2D culture to organ-on-chip, Lab Chip, 2018, 18(9), 1274–1291 RSC.
- Q. Wu, J. Liu, X. Wang, L. Feng, J. Wu and X. Zhu,
et al., Organ-on-a-chip: recent breakthroughs and future prospects, Biomed. Eng. Online, 2020, 19(1), 9 CrossRef PubMed.
- H. J. Kim, D. Huh, G. Hamilton and D. E. Ingber, Human gut-on-a-chip inhabited by microbial flora that experiences intestinal peristalsis-like motions and flow, Lab Chip, 2012, 12(12), 2165–2174 RSC.
- H. J. Kim and D. E. Ingber, Gut-on-a-Chip microenvironment induces human intestinal cells to undergo villus differentiation, Integr. Biol., 2013, 5(9), 1130–1140 CrossRef CAS PubMed.
- Q. Zhang, L. Sito, M. Mao, J. He, Y. S. Zhang and X. Zhao, Current advances in skin-on-a-chip models for drug testing, Microphysiol. Syst., 2018, 2, 4 Search PubMed.
- M. H. Mohammadi, B. H. Araghi, V. Beydaghi, A. Geraili, F. Moradi and P. Jafari,
et al., Skin Diseases Modeling using Combined Tissue Engineering and Microfluidic Technologies, Adv. Healthcare Mater., 2016, 5(19), 2459–2480 CrossRef CAS PubMed.
- D. Huh, D. C. Leslie, B. D. Matthews, J. P. Fraser, S. Jurek and G. A. Hamilton,
et al., A human disease model of drug toxicity-induced pulmonary edema in a lung-on-a-chip microdevice, Sci. Transl. Med., 2012, 4(159), 159ra47 Search PubMed.
- H. J. Song, H. Y. Lim, W. Chun, K. C. Choi, J. H. Sung and G. Y. Sung, Fabrication of a pumpless, microfluidic skin chip from different collagen sources, J. Ind. Eng. Chem., 2017, 56, 375–381 CrossRef CAS.
- I. Risueno, L. Valencia, J. L. Jorcano and D. Velasco, Skin-on-a-chip models: General overview and future perspectives, APL Bioeng., 2021, 5(3), 030901 CrossRef CAS PubMed.
- F. Groeber, M. Holeiter, M. Hampel, S. Hinderer and K. Schenke-Layland, Skin tissue engineering–in vivo and in vitro applications, Adv. Drug Delivery Rev., 2011, 63(4–5), 352–366 CrossRef CAS PubMed.
- L. Semlin, M. Schafer-Korting, C. Borelli and H. C. Korting, In vitro models for human skin disease, Drug Discovery Today, 2011, 16(3–4), 132–139 CrossRef CAS PubMed.
|
This journal is © The Royal Society of Chemistry 2024 |
Click here to see how this site uses Cookies. View our privacy policy here.