DOI:
10.1039/D2NR01673G
(Paper)
Nanoscale, 2022,
14, 9474-9484
Copper metal organic framework as natural oxidase mimic for effective killing of Gram-negative and Gram-positive bacteria†
Received
26th March 2022
, Accepted 2nd June 2022
First published on 8th June 2022
Abstract
Nanozymes have been widely studied as substitutes for natural enzymes. However, the delicacy of their structures and their unclear catalytic sites make it difficult to maintain their structural robustness and catalytic durability. By mimicking active catalytic sites of natural enzymes and combining them with distinct channels of metal organic frameworks (MOFs), an active copper mimetic oxidase enzyme (Cu-MOF) was designed and synthesized with good structure and clear catalytic sites for improvement in catalytic activity. The Cu-MOFs showed excellent oxidase-like activity with a low Km of 1.09 mM and exogenous ROS generation capacity. The Cu-MOFs exhibited antibacterial efficacy at a low concentration of 12.5 μg mL−1 by an oxidative stress response. These Cu-MOFs with their simple design and effective oxidase mimicking show attractive application prospects in the field of antibacterial and enzyme catalysis.
1 Introduction
Nanozymes have gradually become the preferred substitutes for natural enzymes due to their low cost, stress resistance, high-throughput preparation and ability to overcome the limitations of natural enzymes.1–3 Until now, many nanozymes have been reported, such as metal oxides mimicking peroxidase,4,5 and metal–organic frameworks (MOFs) mimicking peroxidase.6 Benefitting from applications of nanozymes, significant advances have been achieved in catalysis science, biosensors, therapeutic applications, environmental protection and antibacterial agents.7 Although impressive progress has been made so far, it is still a challenge to fully realize the potential of nanozymes in this field.8 Most artificial enzymes, such as Fe3O4, have been based on peroxidase-like activity and unstable H2O2 as a sacrificial oxidant.9,10 The effect of localized field enhancement on electron transfer induced by surface plasmon resonance (SPR) of noble metal nanoparticles and its composites makes them an excellent candidate for nanozymes.11,12 However, the previously reported metal oxides and noble metal nanomaterials still show peroxidase-like activity in simulated oxidases,13,14 and their inferior enzyme activity and high preparation cost restrict the practical application of these materials. However, the widespread presence of H2O2 is severely restrictive, and the extra addition of easily decomposable, volatile and highly toxic H2O2 severely restricts the promotion of nanoenzymes. Compared with H2O2, O2 naturally dissolved in solution is highly stable and mild, so oxidase nanozymes have attracted extensive attention due to their oxidation mechanism by directly activating O2 to yield reactive oxygen species (ROS), avoiding the participation of H2O2,15,16 and making them green catalysts.17
In recent years, some nanozymes have been reported to imitate the structure of natural enzymes, which provide a possible solution for the design and synthesis of new artificial oxidases.18,19 In an oxygen-activating natural oxidase, a transition metal plays a wide variety of roles of a natural enzyme on the basis of its generally accessible redox couple and bioavailability,20 such as in hemocyanin,21 tyrosinases,22 dopamine β-monooxygenase (DβM)23 and catechol oxidase (CatO).24 Among many proteases, reactive copper is an important kind of catalytically active center, which can catalyze dehydrogenation.25,26 The electron transfer of an active copper center overcomes the spin-forbidden-ness of O2 binding and controls electron transfer to direct catalysis, performing either two-electron electrophilic aromatic substitution or one-electron H-atom abstraction.27,28 Therefore, by imitating a copper catalytic site, it provides a feasible idea for the rational design of new and improved biomimetic catalysts.29,30 However, the macroscopically rough preparation process of artificial oxidases leads to the loss of nanoscale fine structure, which makes it difficult to imitate the natural enzyme's inherent catalytic structure such as catalytically activity centers as well as substrate binding sites.31,32 Finding an artificial oxidase with a fine secondary structure and cost advantages is urgent.33
MOFs, a kind of multifunctional porous coordination polymer,34 are composed of organic bridging molecules (ligands) and metal ions or metal clusters.35–37 The internal structure and surface physicochemical properties of MOFs can be controlled by the selection of metal ion centers or organic ligands.38,39 In addition, the size and shape of a MOF crystal can be reasonably adjusted by the selection of temperature, solvent and reaction time in the synthesis process.40,41 The diversity of metallic nodes, bridging ligands, and a wide array of coordination interactions in all possible directions make MOFs direct surrogates for artificial oxidases,42 where the well-defined tailorable cavities and channels can offer a hydrophobic coordination environment similar to that of natural enzymes.43 Until now, there have been few submissions about MOFs applied to artificial oxidases.44 Through the pre-structural design and regulation of the preparation process, the catalytic activity and substrate selectivity of a nanozyme can be reasonably adjusted; therefore, MOF-based artificial nanomaterials are expected to improve the performance of nanozymes.
Herein, we have designed and synthesized an active copper artificial oxidase based on Cu-MOFs which can catalyze the oxidation of 3,3′,5,5′-tetramethylbenzidine (TMB) with the absence of additional H2O2. 3-Amino-5-mercapto-1,2,4-triazole (AMTA)45 not only has potential biological activity (treating viral as well as bacterial infection) due to nitrogen and sulfur heterocyclic systems but also has potential as a multifunctional ligand in coordination chemistry which can bind strongly to Cu2+ ions via N–Cu coordination to afford a catalytic center. As a result, AMTA was selected as a ligand in this catalytic system. By incorporation of the triazole unit in the structure of Cu-MOFs, the generation efficiency of reactive oxygen species (ROS) was effectively improved.46 Cu-MOFs show excellent oxidase-like activity at a very low concentration; furthermore, the oxidase-like activity and exogenous ROS generation were used in research against E. coli and S. aureus bacterial strains. The antibacterial results showed that Cu-MOFs have stronger antibacterial activity. To the best of our knowledge, this is the first time that Cu-MOFs alone have shown good oxidase activity in the absence of a second noble metal component (Scheme 1).
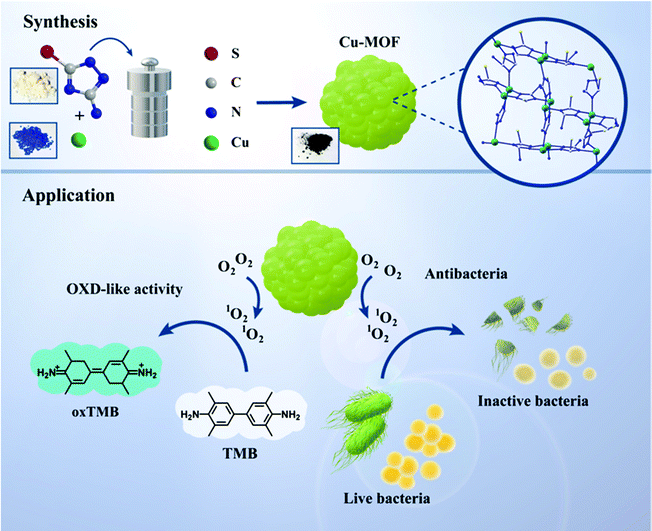 |
| Scheme 1 Schematic illustration of the synthesis and oxidase-like activity of Cu-MOFs with their antibacterial presentation. | |
2 Experimental section
2.1 Reagents
Copper(II) nitrate hydrate (Cu(NO3)2·2.5H2O) was obtained from Sigma-Aldrich Co., Ltd. AMTA and N,N-dimethylformamide (DMF) were purchased from Shanghai Aladdin Biochemical Technology Co., Ltd. Anhydrous ethanol (C2H5OH), sodium borohydride (NaBH4, 96.0%) were of analytical grade (AR) and purchased from China Medicine (Group) Shanghai Chemical Reagent Corp. All chemical reagents were used as received without further purification. Deionized water (18.4 MΩ cm) was used for all experiments and was obtained from a Milli-Q system (Millipore, Bedford, MA).
2.2 Instruments
The morphology and size of the sample were visualized using a scanning electron microscope (SEM; Nova Nano SEM 450, American). The crystallinity and the purity of the particles were determined by X-ray diffraction (XRD; D8A25, Bruker, Germany) using Cu Kα radiation (λ = 1.54051 and 1.54433 Å) over the 2θ range of 3°–80°. Transmission electron microscope (TEM) images were taken with a JEOL JEM 2100F at an accelerating voltage of 200 kV. The JEOL JEM 2100F is also equipped with an energy dispersive X-ray spectrometer (EDX) system, allowing the elemental analysis of the samples. The copper ions were measured by atomic absorption spectroscopy (AA-6300 double-beam flame spectrophotometer; Shimadzu, Europe) and inductively coupled plasma mass spectrometry (ICP-MS; Thermo Fisher, Shanghai). Fourier transform infrared (FT-IR) spectra were collected on a JASCO FT-IR 480 Plus spectrophotometer. UV-visible spectra were recorded on a Shimadzu UV2501 spectrophotometer. Zeta potential (Zeta; Malvern, UK) was measured to detect the positive and negative charges of the sample solution. Dynamic light scattering (DLS; Malvern, UK) was utilized to detect the particle size distribution. Cyclic voltammetry (CV) was performed with an electrochemical workstation (CHI660C) and a typical three-electrode system.
2.3 Preparation of the Cu-MOFs
Cu(NO3)2·2.5H2O (697.8 mg, 3 mmol) and AMTA (522.6 mg, 4.5 mmol) were placed in 100 mL of a DMF/C2H5OH solvent mixture (DMF
:
C2H5OH = 1
:
1) and heated for 12 h at 120 °C. After centrifugation, the sediment was rinsed repeatedly with C2H5OH and DMF and heated for 24 h at 120 °C in a vacuum. The obtained product was named Cu-MOFs.
2.4 Oxidase-like activity of Cu-MOFs
The oxidase-like activity of Cu-MOFs was evaluated by using TMB as a substrate molecule for a colorimetric assay. Typically, 2.5 mM TMB and 0.1 mg mL−1 Cu-MOFs were mixed with acetate buffer solution (pH = 4), followed by incubation at 40 °C with constant oscillation for 10 min in a thermostatic mixed-metal bath. Finally, the absorbance of oxidized TMB at 652 nm was measured using a UV-vis spectrophotometer. As a control group, the oxidase activities of (Cu(NO3)2·2.5H2O) and AMTA were investigated using the same experimental procedure.
ABTS was also used to investigate the enzyme-like activity of Cu-MOFs. 2.5 mM ABTS was added to 0.1 mg mL−1 Cu-MOFs with acetate buffer solution (pH = 4), followed by incubation at 40 °C with constant oscillation for 10 min in a thermostatic mixed-metal bath. The absorbance of oxidized ABTS at 430 nm was recorded on a UV-vis spectrophotometer.
2.5 Kinetic and mechanism analysis
The kinetics of TMB catalysis was monitored in a time-scan mode with the absorption spectra at 652 nm. Cu-MOFs (0.5 mg) and different concentrations of TMB (0.05, 0.1, 0.25, 0.5, 0.75, 1.0 mM) were mixed with acetate buffer solution (pH = 4). After that, the reaction was monitored immediately. The relevant kinetic parameters were calculated according to the Michaelis–Menten equation: |  | (1) |
where ν is the initial velocity, νmax is the maximal reaction velocity, Km is the Michaelis constant, and [S] is the substrate concentration.
To explore the mode of action for Cu-MOFs oxidase-like enzyme activity, active species-scavenging experiments were conducted. A scavenger of singlet oxygen (1O2) or hydroxyl radical (˙OH) was added to a mixed solution of Cu-MOFs and TMB, followed by incubation for 10 min. Finally, the absorbance spectrum of oxidized TMB was recorded.
2.6 Verification of antibacterial ability
To examine the antibacterial ability of Cu-MOFs against Gram bacteria, two strains were used as representatives of different types of Gram bacteria. E. coli was used as an example of Gram-negative bacteria, and S. aureus was used as the representative of Gram-positive bacteria. All the bacterial strains were stored at 4 °C and reactivated by incubating the bacteria in 100 mL of medium at 37 °C with 150 rpm shaking overnight until the density of the bacteria reached 108 colony forming units (CFU) per mL.
To assess the sterilization ability of Cu-MOFs, comparative experiments were conducted. By setting the concentration of Cu-MOFs at 0, 0.25, 0.5, 12.5, 25, and 50 μg mL−1, bacterial solutions and antibacterial material solutions were mixed at a volume ratio of 1
:
1, followed by spreading of the mixture on an agar plate and incubating overnight. The antibacterial ability of Cu2+ and AMTA was investigated by the same experimental procedure. The structural stability of Cu-MOFs before and after co-culture with bacteria was determined by measuring the content of Cu2+ in the supernatant.
2.7 Live/dead fluorescence assay
Gram-negative bacteria (E. coli) and Gram-positive bacteria (S. aureus) suspensions were centrifuged at 2000 rpm and washed with PBS three times, and finally resuspended in PBS. Cu-MOFs (0.5 μg) were added into 1 mL of E. coli and S. aureus suspensions, respectively, and then incubated at 37 °C for 12 h. After that, the suspensions were pelleted at 2000 rpm to obtain bacteria and resuspended in PBS. SYTO-9 and propidium iodide (PI) were added to the treated bacteria and stained in the dark with for 0.5 h. Fluorescence images were achieved by using a fluorescence inverted microscope.
3 Results and discussion
3.1 Characterization of Cu-MOFs
The morphology of the prepared Cu-MOFs was characterized by SEM. Fig. 1(A) shows that the Cu-MOFs have a good spherical structure. TEM images were collected to characterize the obtained Cu-MOFs, as revealed in Fig. 1(B), where the overall nanoparticle size is estimated to about 300 nm, and the clear edge frame structure of Cu-MOFs shows that the nanoparticles have become compact, which is conducive to an improvement in the stability of the Cu-MOFs. The fast Fourier transform (FFT) pattern of the Cu-MOFs is shown in the inset to Fig. 1(C) with a lattice spacing of ∼0.206 nm (Fig. S1†), which is evidence of the crystal structure of the Cu-MOFs. It can be inferred that the formation of a good spherical structure is based on the process of crystallization and the Ostwald ripening process.47 First, Cu2+ unite with the AMTA ligands to form amorphous solid spherical particles. After a further solvothermal reaction, the amorphous solid spherical particles tend to crystallize under conditions of continuous high pressure and high heat, and self-assembly to gradually form larger spherical crystals.48 Elemental mapping by EDX (Fig. 1(D)) revealed that the Cu-MOFs consist of homogeneously distributed Cu, C, N, S elements. The crystal structure of the Cu-MOFs was further characterized by XRD. After coordination between AMTA and Cu2+, the characteristic peaks belonging to AMTA disappear. Due to the incomplete crystallization effect, the pure Cu-MOFs did not show sharp and obvious diffraction peaks, and exhibited characteristic diffraction peaks (2θ = 30°) in agreement with previous reports.49 The FTIR spectra of AMTA and Cu-MOFs are shown in Fig. 1(F). For pure AMTA, the band at 3381 cm−1 is assigned to ν(N–H) of the ligand, and disappeared in Cu-MOFs; this observation demonstrates that the amino group coordinated with Cu. The inconspicuous new peaks of Cu-MOFs at 3323 cm−1 also proved the effect of the coordination process on AMTA. A band occurs at 1655 cm−1 assigned to NH2 bending vibration. As the nitrogen atom on the imidazole ring is coordinated with a copper ion, similar atoms outside the ring can be retained in Cu-MOFs. Similarly, the triazole ring stretching band occurring at 1496 cm−1 is also retained. The C–N stretching vibration could be evidenced by the band at 1134 cm−1.
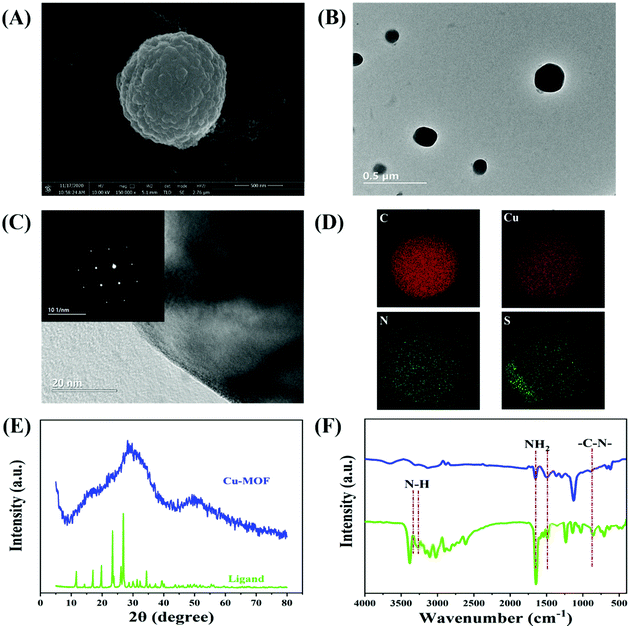 |
| Fig. 1 SEM images of uniform Cu-MOF nanoparticles (A). TEM image of Cu-MOF nanoparticles at low magnification (B). TEM image extracted from a part of a Cu-MOF nanoparticle; inset is the corresponding FFT pattern of Cu-MOF nanoparticles (C). EDS mapping of Cu-MOF nanoparticles (D). XRD and FTIR spectral analysis of bridging ligand, Cu-MOFs (E) and (F). | |
The thermal stability of the structure for the prepared Cu-MOFs was explored by thermogravimetric analysis. The individual ligands have an obvious thermally stable platform (0–280 °C). Above 280 °C, the ligands will sublime. The quality of the prepared Cu-MOFs is reduced due to the volatilization of crystal water at 0–150 °C. Above 150 °C, the weight loss can be attributed to degradation of the framework structure, and this leads to the oxidation of some Cu. Although the quality of Cu-MOFs is slowly reduced, they still have a mass retention rate of more than 70% at 400 °C, indicating their relatively excellent thermal stability (Fig. 2(A)). Due to the mild application environment (nanozyme catalysis, antibacterial), the thermal stability of Cu-MOFs guarantees their excellent stability in the operating temperature range. As a typical porous material, the porous structure brings about a larger accessible surface, which is conducive to the adsorption of dissolved oxygen and the generation of ROS, and the pores of Cu-MOFs provide active sites similar to those of natural enzymes, which help increase enzyme activity. As shown in Fig. 2(B), the N2 adsorption–desorption isotherm of Cu-MOFs belongs to the type IV isotherm, corresponding to typical mesoporous materials. The BET surface area was calculated as 212.793 m2 g−1, and the pore size distributions for Cu-MOFs are shown in the inset to Fig. 2(B).
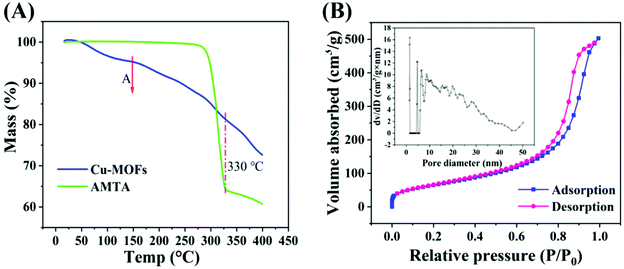 |
| Fig. 2 (A) TGA curve of Cu-MOFs (blue), AMTA (green). (B) N2 adsorption–desorption isotherm; inset is the pore size distribution. | |
The surface chemical composition and oxidation state of an enzyme have a significant effect on the catalytic ability of the enzyme. CV curves of bare electrodes and Cu-MOF-modified electrodes are compared in Fig. 3(A). There are two characteristic peaks at 0.11 and 0.38 V in 0.5 M H2SO4 under 50 mV s−1 conditions, corresponding to the reduction peaks of Cu2+. The presence of Cu in the composites as well as the oxidation state were confirmed using high-resolution X-ray photoelectron spectroscopy (XPS). Fig. 3(B) reveals the spectra of Cu-MOFs. The peaks of Cu 2p, N 1s, and S 2p appeared in the spectra. Fig. 3(C) shows the core-level and shakeup satellite (sat.) lines of Cu 2p.50 The Cu 2p3/2 and 2p1/2 core levels are located at binding energies of 932.7 and 955.2 eV, and are close to the data for Cu2+, which shows the divalent nature of the Cu atoms, which are thus expected to be effective active sites during catalysis. Several strong satellite peaks (at 963.4, 943.4 eV) were easy to observe. Fig. 3(D) shows two peaks of N 1s at 400.5 and 402.1 eV. Pyrrole nitrogen (N–H) at 402.1 eV was significantly lower than C
N at 400.5 eV, confirming the formation of Cu-MOFs.
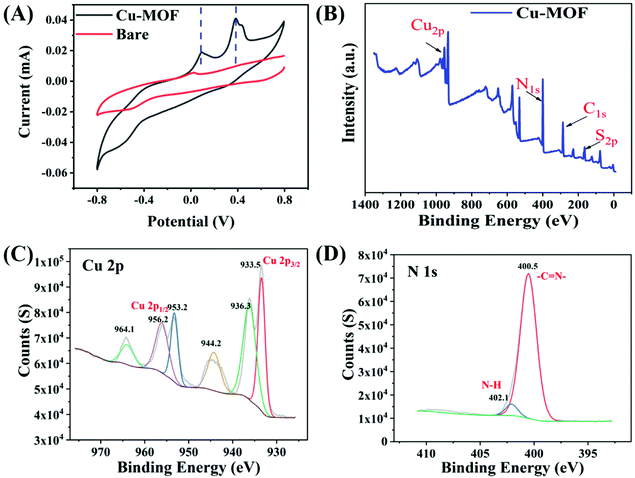 |
| Fig. 3 CV curves of the Cu-MOFs in 0.5 M H2SO4 at 50 mV s−1 (A). XPS survey scan of Cu-MOFs (B). Cu 2p (C) and N 1s (D) XPS spectrum of Cu-MOFs. | |
3.2 Oxidase-like activity of Cu-MOFs
TMB and ABTS, as well-established chromogenic substrate molecules, were utilized to investigate the oxidase-like activity of Cu-MOFs without the help of additional H2O2.51 In the model reaction of catalytic oxidation of TMB (Fig. 4(A)) and ABTS (Fig. 4(B)), they are able to show a clear color change from colorless to blue or green. Fig. 4(C) presents the results of the oxidase activity analysis of Cu-MOFs. ROS generated by Cu-MOFs can catalyze TMB to oxidase TMB (blue color) with maximal absorbance at 652 nm, but the TMB or Cu-MOFs only groups show no color change or absorption at 652 nm. Similarly, oxidized ABTS shows a green color under the catalysis of Cu-MOFs and has a maximal absorbance at 430 nm in Fig. 4(D). For comparison, the single Cu-MOFs or ABTS groups show no color change and the absorbance at 430 nm is unprecedented. Cu(NO3)2·2.5H2O and AMTA alone do not show any enzymatic activity (Fig. S4†). These results clearly verify the strong oxidase-like activity of Cu-MOFs.
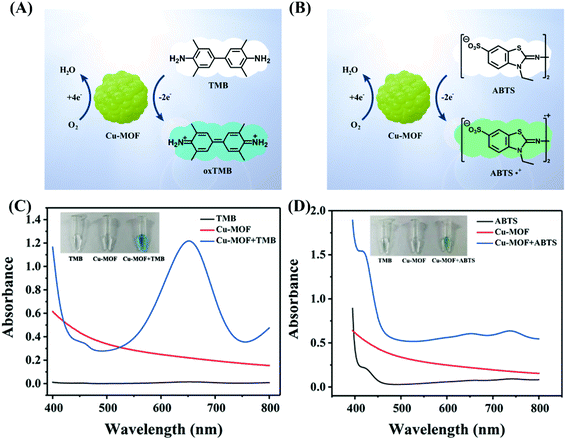 |
| Fig. 4 Schematic illustration of the oxidase-like activity of Cu-MOFs for TMB (A) and ABTS (B). The UV-vis spectra of TMB (C) and ABTS (D) in the absence and presence of Cu-MOFs. Inserts: the color change of the corresponding solution. | |
The catalytic activity of a natural enzyme is affected by its reaction conditions. Fig. S2† shows the effect of reaction conditions on the oxidase-like activity of prepared Cu-MOFs, like the pH, temperature, and concentration of Cu-MOFs. Fig. S2(A)† shows the absorption at 652 nm of the Cu-MOFs + TMB reaction systems under different pH from 3 to 8 and the optimum pH was found to be 4.0. The Cu-MOFs presented better catalytic activity over a wide temperature range (4–60 °C), which is shown in Fig. S2(B).† The catalytic effect was enhanced with increasing Cu-MOFs concentration (Fig. S2(C)).† When the concentration of Cu-MOFs was 0.5 mg mL−1, the absorbance reached its optimum. Therefore, the optimum catalytic pH and Cu-MOFs concentration are nearly 4 and 0.5 mg mL−1, respectively. To investigate the stability over time of Cu-MOFs in aqueous medium, the Cu-MOF dispersion was stored at room temperature and the oxidase activity was tested for 4 weeks continuously. As shown in Fig. S3,† there were no remarkable changes in the oxidized TMB intensity after 28 days, indicating the satisfactory long-term stability of the Cu-MOFs.
3.3 Kinetic and mechanism analysis
To verify the catalytic mechanism, a kinetic study was conducted by steady-state kinetics experiments. According to time-dependent absorbance at 652 nm with different concentrations of TMB in the presence of 0.5 mg mL−1 (Fig. 5(A)), the kinetics plot was obtained and is shown in Fig. 5(B), which followed Michaelis–Menten kinetics.52Vmax and Km were calculated from the kinetics plot as 17.06 μM s−1 and 1.09 mM, respectively. The Vmax value represents the catalytic activity of Cu-MOFs, and the high value indicates stronger catalytic activity. Compared with other nanozymes listed in Table S1,† Cu-MOFs exhibited a higher catalytic activity and affinity, which may be associated with the copper metal center and larger specific surface area of Cu-MOFs.53
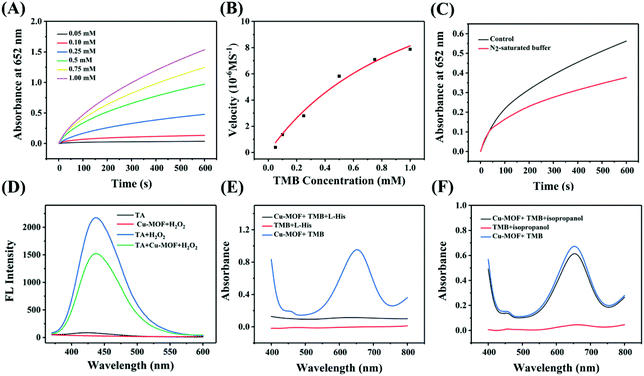 |
| Fig. 5 Time-dependent absorbance at 652 nm with different concentrations of TMB in the presence of 0.5 mg mL−1 Cu-MOFs (A). Michaelis–Menten kinetic assay for TMB (B). UV-vis absorption spectra of TMB in air-saturated and N2-saturated buffer with Cu-MOFs (C). The fluorescence spectra of the TA assay for the detection of hydroxyl radicals (˙OH) (D). Absorbance at 652 nm of TMB to estimate the nature of the intermediates for the oxidase-like activity of Cu-MOFs (0.5 mg mL−1) in the presence of various quenchers (E and F). | |
As oxidase nanozymes, Cu-MOFs were assumed to have an oxidase-type mechanism by activating O2 to yield ROS. Fig. 5(C) shows the effect of O2 on oxidase-like activity. Compared to the absorbance of control groups, the absorbance of TMB catalyzed by Cu-MOFs was significantly inhibited in N2-saturated buffer, indicating that O2 plays an important role in this reaction. To understand the catalysis mechanism of Cu-MOFs between oxidase and peroxidase-like activity, a fluorescent assay with terephthalic acid (TA) was conducted to investigate the possibility of peroxidase-like activity. It is well known that peroxidase-like nanozymes can catalyze H2O2 to generate ˙OH which converts non-fluorescent TA to highly fluorescent 2-hydroxy terephthalic acid (TAOH). In Fig. 5(D), the TA itself and Cu-MOFs/H2O2 have no fluorescence peak at 435 nm, while a distinct fluorescence peak can be observed in H2O2/TA due to the self-decomposition of H2O2. After the addition of Cu-MOFs, there is no clear enhancement in the emission peak, and this strongly indicates that Cu-MOFs did not depend on peroxidase-like activity at the same time.
To figure out the model of action for the oxidase-like activity of Cu-MOFs, an ROS-scavenging experiment was performed. L-Histidine and isopropanol were added into Cu-MOFs + TMB reaction systems, as quenchers of single oxygen (1O2) and hydroxyl radicals (˙OH), respectively. As shown in Fig. 5(E and F), L-histidine effectively suppressed the oxidation of TMB, but the absorbance of the group with isopropanol showed no significant difference from the control, indicating that the formation of 1O2 is the prominent reason for the oxidase-like activity of Cu-MOF instead of ˙OH. The result further verified the oxidation mechanism of Cu-MOFs by activating O2 to produce 1O2.
3.4 Antibacterial performance of Cu-MOFs
ROS is able to destroy the bacterial membrane to inhibit bacterial growth through oxidative stress. Based on their outstanding oxidase-like activity, Cu-MOFs can induce O2 to produce 1O2; therefore the antibacterial ability was studied. An antibacterial experiment was conducted to evaluate the antibacterial efficacy of Cu-MOFs against E. coli and S. aureus. Fig. 6(A) shows the relationship between antibacterial performance against E. coli and S. aureus with the concentration of Cu-MOFs from 0 to 50 μg mL−1. With the increasing concentration of Cu-MOFs, the antibacterial rate increased from 0% to 100%, which indicated that Cu-MOFs with higher concentration had better antibacterial properties. When the concentration of Cu-MOFs reached 25 μg mL−1, the antibacterial ratio could reach 99.9%. Compared with E. coli, the antibacterial efficacy against S. aureus of Cu-MOFs is more obvious, owing to E. coli having a multilayer outer membrane and strong drug resistance. The above results indicated that Cu-MOFs displayed good broad-spectrum antibacterial ability at low concentration. 12.5 μg mL−1 of Cu-MOFs was selected to verify the time-dependent antibacterial properties against E. coli and S. aureus in the time range of 0 to 40 minutes; the results are shown in Fig. S5.† The antibacterial rate increased with increasing incubation time with Cu-MOFs. When the incubation time reached 40 min, the antibacterial ratio could reach 99.9%, which confirms that Cu-MOFs have satisfactory antibacterial ability against E. coli and S. aureus within 40 min.
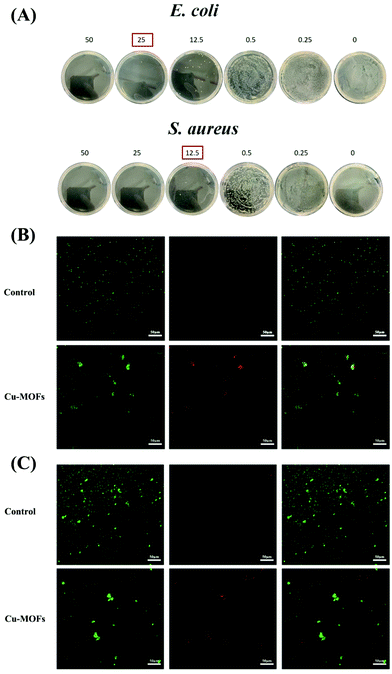 |
| Fig. 6 Antibacterial performance against E. coli and S. aureus (A) of Cu-MOFs with concentrations from 0 to 50 μg mL−1. (B and C) Fluorescence images of E. coli (B) and S. aureus (C) that had undergone Cu-MOFs treatment. Live bacteria (green) and dead bacteria (red). | |
To further assess the effect of the Cu-MOFs on bacterial cell viability and morphology, a live/dead staining experiment was conducted and is revealed in Fig. 6(B) and (C). SYTO-9 (green channel), as a cell-permeant nucleic acid stain, stains living E. coli and S. aureus cells. PI (red channel) can enter bacterial cells through broken membranes to stain dead E. coli and S. aureus cells. In control images of bacteria alone without added Cu-MOFs, a bright green (live) signal with high contrast was observed in E. coli and S. aureus cells. When the bacteria cells were treated with 0.167 mg mL−1 Cu-MOFs, the emission in the red channel showed higher contrast in E. coli and S. aureus cells, suggesting that bacterial cells in the image had been effectively killed by Cu-MOFs. As mentioned above, this illustrates the excellent antibacterial properties of Cu-MOF due to an oxidative stress response, also revealing the antibacterial mechanism of Cu-MOFs that may be caused by the destruction of the cell membrane.
Cu2+ has a certain antibacterial effect. In order to verify that the antibacterial effect of the prepared Cu-MOFs came from its excellent oxidase activity rather than Cu2+ or AMTA, the structural stability of Cu-MOFs was explored before and after antibacterial treatment. The amount of Cu2+ in the supernatant before and after co-culture with bacteria was quantified by AAS and ICP-MS. The Cu2+ content in the supernatant before co-culture with bacteria determined according to the standard curve is 23.6 ng mL−1 (Fig. 7(A)). Compared with the supernatant before antibacterial treatment, there was no significant increase in Cu2+ (32.3 ng mL−1) after co-culture with bacteria, indicating that the Cu-MOFs had good structural stability, and framework collapse would not occur. By changing Cu2+ concentration, a dose-dependent study of antibacterial performance against E. coli and S. aureus of Cu2+ (20 and 100 ng mL−1 prepared from Cu(NO3)2) was conducted. As shown in Fig. 7(B), compared with the control group without adding Cu2+, the survival rate of the bacteria (E. coli and S. aureus) did not decrease when the Cu2+ concentration was 20 ng mL−1, and even at a very high concentration (100 ng mL−1) it showed negligible antibacterial activity, and for AMTA alone (5 mg mL−1), no obvious antibacterial activity was found. That shows that high-efficiency oxidase activity is the main reason for the excellent antibacterial effect of Cu-MOFs.
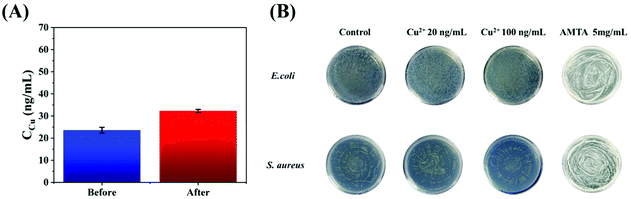 |
| Fig. 7 (A) The Cu2+ content in the supernatant before and after co-incubation with bacteria. (B) Antibacterial performance against E. coli and S. aureus in the corresponding different concentrations of Cu2+ and AMTA. | |
In order to understand the morphological effects on different kinds of bacteria in the presence of Cu-MOFs, the morphological changes in E. coli and S. aureus cells were observed by SEM. Fig. 8(A) shows that normal E. coli have a complete and smooth outer membrane. After co-incubation with Cu-MOFs (Fig. 8(B)), the ROS produced by Cu-MOFs caused serious oxidative damage to the outer membrane, and the outer membrane was seriously damaged with obvious folds. Fig. 8(C) and (D) indicated that S. aureus could hardly maintain their normal cell morphology after co-incubation with Cu-MOFs. The cytoplasm of bacteria flew out after the membrane was damaged, resulting in the death of the bacteria. Above all this showed that Cu-MOFs have a strong inhibitory effect on E. coli and S. aureus, and the oxidative damage to the bacterial membrane leads to the death of the bacteria.
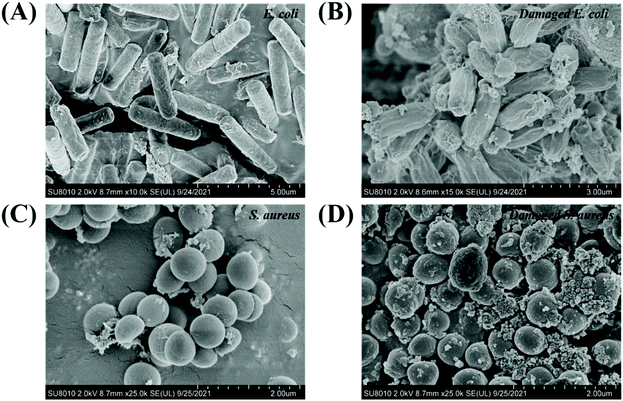 |
| Fig. 8 SEM morphological images of bacteria: normal and damaged E. coli (A and B); normal and damaged S. aureus (C and D). | |
4 Conclusion
In this work, a novel strategy was adopted to construct an oxidase-like nanozyme (Cu-MOFs) according to the blueprint of a natural enzyme. The well-defined tailorable cavities of Cu-MOFs can offer a hydrophobic coordination environment similar to that of natural enzymes. Compared with natural enzymes, Cu-MOFs with a reactive copper core have a stronger oxygen activation ability without the addition of H2O2 and higher stability. Antibacterial test results indicated that Cu-MOFs displayed good broad-spectrum antibacterial ability at low concentration, strong oxidase activity leading to wrinkle changes and functional inactivation of bacterial cell membranes. Our work reveals that oxidase Cu-MOFs show potential in catalysis and bacterial control.
Conflicts of interest
The authors declare that they have no known competing financial interests or personal relationships that could have appeared to influence the work reported in this paper.
Acknowledgements
This work was supported by the National Natural Science Foundation of China (Grant No. 61875114 and 62005156).
References
- H. Wei and E. Wang, Chem. Soc. Rev., 2013, 42, 6060–6093 RSC.
- J. Kim, J. W. Grate and W. Ping, Trends Biotechnol., 2008, 26, 639–646 CrossRef CAS PubMed.
- H. Wei, C. G. Chen, B. Y. Han and E. K. Wang, Anal. Chem., 2008, 80, 7051–7055 CrossRef CAS PubMed.
- P. Anjani, A. Nagvenkar and A. Gedanken, ACS Appl. Mater. Interfaces, 2016, 8, 22301–22308 CrossRef PubMed.
- W. He, X. Wu, J. Liu, X. Hu, K. Zhang, S. Hou, W. Zhou and S. Xie, Chem. Mater., 2010, 22, 2988–2994 CrossRef CAS.
- L. Mi, Y. Sun, L. Shi and T. Li, ACS Appl. Mater. Interfaces, 2020, 12, 7879–7887 CrossRef CAS PubMed.
- Y. M. Park, Y. S. Choi, H. R. Lee, S. H. Yun and S. J. Lee, Biosens. Bioelectron., 2020, 161, 112252 CrossRef CAS PubMed.
- A. R. Vazquez-Duhalt, Chem. Biol., 2002, 9, 555–565 CrossRef.
- R. A. Lawrence and R. F. Burk, Biochem. Biophys. Res. Commun., 2012, 425, 503–509 CrossRef CAS PubMed.
- Q. Wang, Z. Yang, X. Zhang, X. Xiao and B. Xu, Angew. Chem., Int. Ed., 2010, 46, 4285–4289 CrossRef PubMed.
- T. K. Sau, A. L. Rogach, F. J. Ckel, T. A. Klar and J. Feldmann, Adv. Mater., 2010, 22, 1805–1825 CrossRef CAS PubMed.
- K. Li, N. J. Hogan, M. J. Kale, N. J. Halas and P. Christopher, Nano Lett., 2017, 17, 3710–3717 CrossRef CAS PubMed.
- K. Abdullah, G. Sevil, A. Osman, S. Atac and G. Kursad, Neonatology, 2005, 87, 15–18 CrossRef PubMed.
- X. Jiang, X. Wang, A. Lin and H. Wei, Anal. Chem., 2021, 93, 5954–5962 CrossRef CAS PubMed.
- Z. Peng, S. Dengrong, C. Ara, W. Seunghyun and L. Seonggyu, Nat. Commun., 2019, 10, 940 CrossRef PubMed.
- A. A. Vernekar, T. Das, S. Ghosh and G. Mugesh, Chem. – Asian J., 2016, 11, 72–76 CrossRef CAS PubMed.
- M. Huo, L. Wang, Y. Chen and J. Shi, Nat. Commun., 2017, 8, 357 CrossRef PubMed.
- Q. Liu, K. Wan, Y. Shang, Z. G. Wang and B. Ding, Nat. Mater., 2021, 20, 395–402 CrossRef CAS PubMed.
- S. Y. Lee, S. Y. Lim, D. Seo, J. Y. Lee and T. D. Chung, Adv. Energy Mater., 2016, 6, 1502207 CrossRef.
- C. L. Coyle, W. G. Zumft, P. Kroneck, H. KÖRNER and W. Jakob, FEBS J., 2010, 153, 459–467 Search PubMed.
- R. J. Quinlan, M. D. Sweeney, L. L. Leggio and H. Otten, Proc. Natl. Acad. Sci. U. S. A., 2011, 108, 15079–15084 CrossRef CAS PubMed.
- Y. A. Bae, G. B. Cai, S. H. Kim, W. M. Sohn and Y. Kong, Int. J. Parasitol., 2013, 43, 891–900 CrossRef CAS PubMed.
- P. J. Klinman, J. Biol. Chem., 2006, 281, 3013–3016 CrossRef PubMed.
- N. Fujieda, A. Hasegawa, K. I. Ishihama and S. Itoh, Chem.– Asian J., 2012, 7, 1203–1207 CrossRef CAS PubMed.
- N. A. Miti, S. J. Smith, A. Neves, L. W. Guddat, L. R. Gahan and G. Schenk, Chem. Rev., 2006, 106, 3338–3363 CrossRef PubMed.
- J. W. Ginsbach, M. T. Kieber-Emmons, R. Nomoto and A. Noguchi, Proc. Natl. Acad. Sci. U. S. A., 2012, 109, 10793–10797 CrossRef CAS PubMed.
- K. P. Jensen and U. Ryde, J. Biol. Chem., 2004, 279, 14561–14569 CrossRef CAS PubMed.
- E. I. Solomon, Inorg. Chem., 2016, 55, 6364 CrossRef CAS PubMed.
- P. Wu, F. Fan, J. Song, W. Peng and B. Wang, J. Am. Chem. Soc., 2019, 141, 19776–19789 CrossRef CAS PubMed.
- X. Feng, Y. Song, J. S. Chen, Z. Xu and W. Lin, J. Am. Chem. Soc., 2021, 143, 1107–1118 CrossRef CAS PubMed.
- J. Vogt, R. Perozzo, A. Pautsch, A. Prota and G. E. Schulz, Proteins: Struct., Funct., Bioinf., 2015, 41, 545–553 CrossRef.
- F. Fresno, R. Portela, S. Suárez and J. M. Coronado, J. Mater. Chem. A, 2014, 2, 2863–2884 RSC.
- R. R. Zhu, W. R. Wang, X. Y. Sun, H. Liu and S. L. Wang, Toxicol. in Vitro, 2010, 24, 1639–1647 CrossRef CAS PubMed.
- H. L. Li, M. M. Eddaoudi, M. O'Keeffe and O. M. Yaghi, Nature, 1999, 402, 272–279 Search PubMed.
- P. Li, S. Y. Moon, M. A. Guelta, L. Lin, D. Gómez-Gualdrón, R. Q. Snurr, S. P. Harvey, J. T. Hupp and O. K. Farha, ACS Nano, 2016, 10, 9174–9182 CrossRef CAS PubMed.
- X. Lian, Y. Fang, E. Joseph, Q. Wang, J. Li, S. Banerjee, C. Lollar, X. Wang and H. C. Zhou, Chem. Soc. Rev., 2017, 46, 3386–3401 RSC.
- C. Li, K. Wang, J. Li and Q. Zhang, Nanoscale, 2020, 12, 7870–7874 RSC.
- S. Horike, M. Dinca, K. Tamaki and J. R. Long, J. Am. Chem. Soc., 2008, 130, 5854–5855 CrossRef CAS PubMed.
- K. Wang, R. Bi, M. Huang, B. Lv, H. Wang, C. Li, H. Wu and Q. Zhang, Inorg. Chem., 2020, 59, 6808–6814 CrossRef CAS PubMed.
- Z. Yue, D. Haiyun, L. Yuan, F. Chunhuan and Y. Hu, Chem. Commun., 2019, 55, 3445–3448 RSC.
- C. Li, K. Wang, J. Li and Q. Zhang, ACS Mater. Lett., 2020, 2, 779–797 CrossRef CAS.
- J. Liu, L. Y. Ye, W. H. Xiong, T. Liu, H. Yang and J. Lei, Chem. Commun., 2021, 57, 2820–2823 RSC.
- J. Zhuang, Y. Duan, Q. Zhang, W. Gao and L. Zhang, Nano Lett., 2020, 20, 4051–4058 CrossRef CAS PubMed.
- L. Xinping, Y. Zhengqing, Z. Zhang, S. Yuhuan and R. Jinsong, ACS Nano, 2019, 13, 5222–5230 CrossRef PubMed.
- A. Association, Am. Music Ther. Assoc., 2008, 272, ED504518 Search PubMed.
- S. k. S. Bhattacharyya, R. Ali, M. Venkateswarulu and P. S. Mukherjee, J. Am. Chem. Soc., 2020, 142, 2020 Search PubMed.
- J. H. E. Cartwright, O. Piro and I. Tuval, Phys. Rev. Lett., 2007, 98, 165501 CrossRef PubMed.
- E. S. Jeong, I. H. Hwang and S. W. Han, Langmuir, 2020, 36, 10565–10576 CrossRef CAS PubMed.
- L. Pan, Q. Tao, S. Zhang, S. Wang, Z. Jian, S. Wang, Z. Wang and Z. Zhang, Sol. Energy Mater. Sol. Cells, 2012, 98, 66–70 CrossRef CAS.
- L. Ottaviano, L. Lozzi, F. Ramondo, P. Picozzi and S. Santucci, J. Electron Spectrosc. Relat. Phenom., 1999, 105, 145–154 CrossRef CAS.
- C. Wyns, L. Derycke, B. Soenen, S. Bolca, D. Deforce, M. Bracke and A. Heyerick, Talanta, 2011, 85, 197–205 CrossRef CAS PubMed.
- B. S. Razavi, B. Evgenia and K. Yakov, Front. Microbiol., 2015, 6, 1126 Search PubMed.
- T. Zhang, J. Liu, M. Fellner, C. Zhang, D. Sui and J. Hu, Sci. Adv., 2017, 3, e1700344 CrossRef PubMed.
|
This journal is © The Royal Society of Chemistry 2022 |
Click here to see how this site uses Cookies. View our privacy policy here.