DOI:
10.1039/D1NA00034A
(Paper)
Nanoscale Adv., 2021,
3, 3770-3779
Nickel–cobalt oxalate as an efficient non-precious electrocatalyst for an improved alkaline oxygen evolution reaction†
Received
13th January 2021
, Accepted 27th April 2021
First published on 27th April 2021
Abstract
The quest for developing next-generation non-precious electrocatalysts has risen in recent times. Herein, we have designed and developed a low cost electrocatalyst by a ligand-assisted synthetic strategy in an aqueous medium. An oxalate ligand-assisted non-oxide electrocatalyst was developed by a simple wet-chemical technique for alkaline water oxidation application. The synthetic parameters for the preparation of nickel–cobalt oxalate (Ni2.5Co5C2O4) were optimized, such as the metal precursor (Ni/Co) ratio, oxalic acid amount, reaction temperature, and time. Microstructural analysis revealed a mesoporous block-like architecture for nickel–cobalt oxalate (Ni2.5Co5C2O4). The required overpotential of Ni2.5Co5C2O4 for the alkaline oxygen evolution reaction (OER) was found to be 330 mV for achieving 10 mA cmgeo−2, which is superior to that of NiC2O4, CoC2O4, NiCo2O4 and the state-of-the-art RuO2. The splendid performance of Ni2.5Co5C2O4 was further verified by its low charge transfer resistance, impressive stability performance, and 87% faradaic efficiency in alkaline medium (pH = 14). The improved electrochemical activity was further attributed to double layer capacitance (Cdl), which indefinitely divulged the inferiority of NiCo2O4 compared to Ni2.5Co5C2O4 for the alkaline oxygen evolution reaction (OER). The obtained proton reaction order (ρRHE) was about 0.80, thus indicating the proton decoupled electron transfer (PDET) mechanism for OER in alkaline medium. Post-catalytic investigation revealed the formation of a flake-like porous nanostructure, indicating distinct transformation in morphology during the alkaline OER process. Further, XPS analysis demonstrated complete oxidation of Ni2+ and Co2+ centres into Ni3+ and Co3+, respectively under high oxidation potential, thereby indicating active site formation throughout the microstructural network. Additionally, from BET-normalised LSV investigation, the intrinsic activity of Ni2.5Co5C2O4 was also found to be higher than that of NiCo2O4. Finally, Ni2.5Co5C2O4 delivered a TOF value of around 3.28 × 10−3 s−1, which is 5.56 fold that of NiCo2O4 for the alkaline OER process. This report highlights the unique benefit of Ni2.5Co5C2O4 over NiCo2O4 for the alkaline OER. The structure–catalytic property relationship was further elucidated using density functional theory (DFT) study. To the best of our knowledge, nickel–cobalt oxalate (Ni2.5Co5C2O4) was introduced for the first time as a non-precious non-oxide electrocatalyst for alkaline OER application.
1. Introduction
Water splitting is becoming the most promising approach for hydrogen fuel production and energy storage.1–5 The requirement of a large overpotential for four electron trajectories all through the oxygen evolution reaction (OER) restricts the application in bulk mode.6,7 The ideal category of catalyst for OER is still RuO2 in alkaline and IrO2 in acidic solution.8 However, the cost of scalable design and availability in nature limit the use of such precious elements in day to day industrial applications. Non-precious transition metal based electrocatalysts particularly oxide and hydroxide have attracted great interest particularly owing to their simple synthetic strategy, extraordinary OER activity, and cheap market price.9,10 The improved alkaline OER performance can be attributed to the corrosion resistant physiochemical properties of the working electrode leading to substantial stability in alkaline medium.11 However, non-precious non-oxide transition metal based electrocatalysts are prone to quick in situ electrochemical active surface transformation, thereby rendering rapid kinetics, a low overpotential barrier and high stability. The performance of this catalytic system originates from the presence of microstructural defects, porosity and pore architecture, conductivity and oxidation (chemical) states of active elements.12–15 For example, non-oxide electrocatalysts based on cobalt (Co) are well-known for their metallic nature, which encourages their wide practical application.16–18 There are quite a few literature studies on non-precious non-oxide electrocatalysts for alkaline OER application, such as Co4N, (Co0.7Fe0.3)2B, (CoxFe1−x)2P and Co0.85Se/Co9Se8.19–22 Thus, non-precious non-oxide electrocatalysts have attracted great attention for the alkaline OER.
A ligand assisted soft-chemical strategy has been employed for the design of electrocatalysts in recent years. Feng et al. demonstrated an ionic liquid assisted microwave irradiation technique for the preparation of nickel–cobalt fluoride for water electrolysis.23 Next, hierarchical hydrous cobalt phosphate micro-flower was employed as an efficient electrocatalyst for the alkaline OER.24 Recently, our group has discussed the superior OER electrocatalytic activity of NiCo2OxS4−x over NiCo2O4.25 On the other hand, transition metal based oxalate is renowned as a carbon sink, suggesting it as a more sustainable way to design energy storage materials. Numerous bio- and artificial-synthesis routes have already been reported for the synthesis of oxalate anion (C2O42−) from CO2.26 Therefore, utilization of oxalate-based materials is an impactful way from the clean and green synthesis viewpoint. To our knowledge, there are only few reports on oxalate based materials for OER applications. For instance, cobalt oxalate has shown promising activity as an electrocatalyst for alkaline OER applications.27,28 Liu et al. reported the most convenient and widely used solvothermal synthetic procedure for the preparation of CoC2O4–2H2O with a tuneable rod and 3-D polyhedron like morphology. Post-catalytic transformation into CoOOH species resulted in active sites for the alkaline OER with 436 and 492 mV overpotential (for 10 mA cmgeo−2) for the micro-rod and 3-D polyhedron respectively.29 However, the high overpotential and sluggish kinetics of the catalyst are identified as major limitations for practical implementation in large scale production. To date, no such reports were found for nickel–cobalt oxalate, which might be an excellent electrocatalyst for alkaline water oxidation. In addition, fabrication of non-precious non-oxide transition metal based electrocatalysts that meet the requirement of 10 mA cmgeo−2 current density, thereby reaching a benchmark of 12% solar-to-hydrogen efficiency, is a challenging task.
Herein, we have demonstrated an oxalic acid assisted non-precious non-oxide transition metal (nickel and cobalt) based electrocatalyst for the alkaline OER. Nickel–cobalt oxalate was primarily synthesized by a wet chemical technique, followed by annealing to form nickel–cobalt oxide. The preparation technique was optimised in terms of Ni/Co ratio, oxalic acid amount, temperature and processing time. The overpotential requirement for the block-like Ni2.5Co5C2O4 was found to be 330 mV for reaching the benchmark of 10 mA cmgeo−2. The improved performance of the catalyst was further verified by low charge transfer resistance, long term stability and 87% faradic efficiency. Interestingly, the control studies and the change in double layer capacitance (Cdl) because of electrochemical preconditions clearly suggest that the alkaline OER catalytic performance of Ni2.5Co5C2O4 is superior to that of its high temperature oxide counterpart i.e. NiCo2O4. The structure–catalytic property relationship was further elucidated by density functional theory (DFT) study which was performed to get insight into the improved electrochemical performance of Ni2.5Co5C2O4 compared to the CoC2O4, NiC2O4 and NiCo2O4, and atomic interpretation was also proposed from computed results. In addition, electrocatalytic measurements in electrolyte solutions with different pH values were performed to understand the mechanistic details of Ni2.5Co5C2O4 for the alkaline OER. This investigation exclusively unlocks a strategy to fabricate oxalate ligand assisted non-precious nickel–cobalt based bimetallic electrocatalysts for water oxidation application in alkaline medium.
2. Results and discussion
2.1 Structural characterization
The synthesis of nickel oxalate (NiC2O4), cobalt oxalate (CoC2O4) and nickel–cobalt oxalate (Ni2.5Co5C2O4) samples was performed by a one-pot method using metal nitrate salts and oxalic acid in aqueous media. For comparative study, nickel cobalt oxide (NiCo2O4) was prepared by calcination of nickel–cobalt oxalate (Ni2.5Co5C2O4) at 350 °C for 2 h (see the details in the Experimental and Characterization section, ESI, Table S1†). The elemental phase was examined by the X-ray diffraction (XRD) method. As shown in Fig. 1a, the peak position resembles with the appearance of cobalt oxalate hydrate (CoC2O4–2H2O; ICDD no. #25-0250) and nickel oxalate hydrate (NiC2O4–2H2O; ICDD no. #01-0299). The XRD pattern of Ni2.5Co5C2O4 is quite identical to that of phase-pure cobalt oxalate hydrate, thereby indicating the existence of substituted nickel at some portion of cobalt sites throughout the mixed-metal oxalate crystal framework.30 Furthermore, the XRD pattern of a physical mixture comprising CoC2O4 and NiC2O4 was examined, revealing doublet peaks at 35.1° and 35.59° that can be labelled as CoC2O4 and NiC2O4 respectively. However, Ni2.5Co5C2O4 shows a single broad peak around 34.9°, manifesting the (022) plane of CoC2O4. The resemblance of XRD patterns between Ni2.5Co5C2O4 and CoC2O4 further supports the existence of substituted nickel at some portion of cobalt sites throughout the mixed-metal oxalate crystal framework. In addition, the (022) peak of Ni2.5Co5C2O4 shifts to a lower 2θ value w.r.t CoC2O4, revealing [022] oriented unit cell expansion due to the presence of the nickel component within the composite network (see Fig. S1, ESI†).31
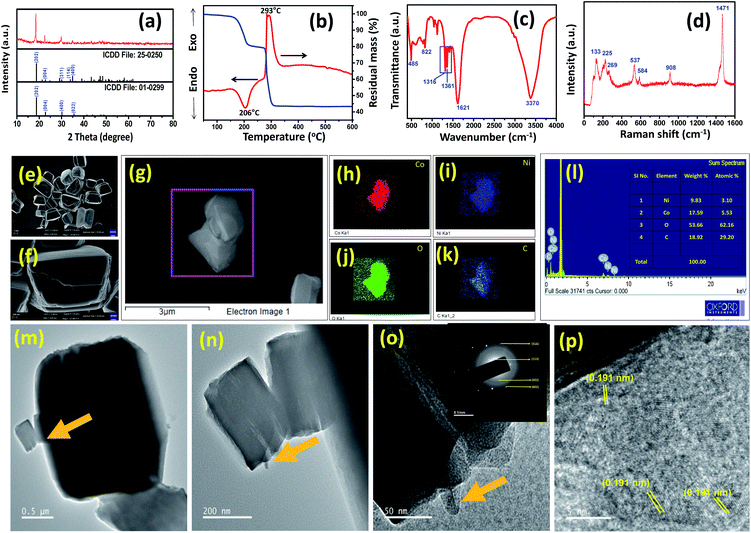 |
| Fig. 1 (a) PXRD with the standard patterns of NiC2O4–2H2O (ICDD no. 01-0299) and CoC2O4–2H2O (ICDD no. 25-0250), (b) DTA-TG, (c) FTIR and (d) Raman pattern of the as-prepared nickel–cobalt oxalate (Ni2.5Co5C2O4) sample. Morphological study including (e and f) FESEM images, (g–k) elemental mapping, (l) EDS study, (m–o) TEM images (the inset showing SAED) and (p) HRTEM image of the as-prepared nickel–cobalt oxalate (Ni2.5Co5C2O4) sample. | |
Thermal stability of the as-prepared sample was corroborated by DTA-TG analysis. Fig. 1b shows an endothermic peak around 205 °C, indicating the removal of lattice water, resulting in 19% weight loss. The exothermic peak around 294 °C represents the decomposition of oxalate ligand with a 57% weight loss compared to the as-prepared mixed oxalate sample.32 No further weight loss is observed in the 300 to 600 °C temperature range. Thus, for the preparation of the NiCo2O4 sample, the as-prepared Ni2.5Co5C2O4 was calcined at 350 °C. Fourier-transform infrared (FTIR) spectroscopy study was carried out for further structural characterization of the sample (Fig. 1c). The FTIR peak positioned around 485 cm−1 is assigned to metal–oxygen vibration mode. The C–O vibration mode is obtained around 1316 (symmetric) and 1361 cm−1 (asymmetric) along with a strong vibrational band at 1621 cm−1, which is a signature characteristic peak for carbonyl stretching vibrational mode, confirming the presence of the oxalate moiety in the as-prepared sample.29 The broad vibrational band around 3370 cm−1 is attributed to water molecules, while 822 cm−1 is to asymmetric O–C–O vibrational mode. Raman measurements were also performed to obtain more structural information (Fig. 1d). The intense peak around 912 cm−1 is attributed to C–C stretching mode, which is IR-silent. The presence of C–C–O bending mode is concluded from the observed 532 cm−1 peak. In addition, appearance of peaks around 225 and 266 cm−1 is ascribed to M–O stretching and O–M–O ring-bending modes respectively, where M stands for the metal centre (cobalt and nickel).16,33 The XPS technique was employed for the analysis of chemical state and probable elemental composition of the as-prepared sample (see Fig. S2, ESI†). The C 1s spectrum exhibits peaks around 286.48, 287.69 and 290.3 eV particularly for C–O, C
O and C–F respectively (see Fig. S2a, ESI†). The appearance of 290.3 eV binding energy could originate from Nafion residue which is used as a binder.34,35 As shown in Fig. S2b (ESI†), peaks at 782.93 and 798.87 eV can be designated as Co 2p3/2 and Co 2p1/2, respectively with a clear separation of 15.94 eV, confirming the presence of Co2+.36 The satellite signals around 786.79 and 803.86 eV further support the same (see Fig. S2b, ESI†). The presence of Ni2+ can be suspected from the binding energy peaks around 857.71 and 875.35 eV assigned to Ni 2p3/2 and Ni 2p1/2 respectively, along with satellite peaks at 862.61 and 881.14 eV (see Fig. S2c, ESI†).37 The narrow scan of the O 1s peak is deconvoluted into two peaks at 533.62 and 534.65 eV representing C–O and lattice water molecules, respectively (see Fig. S2d, ESI†).38 Inductively coupled plasma-atomic emission spectroscopy (ICP-AES) was also employed to get quantitative information regarding the elemental composition of Ni2.5Co5C2O4. The Ni/Co ratio is found to be 1
:
2.018 (see Table S2, ESI†).
2.2 Morphological characterization
Microstructural analysis was performed by FESEM and TEM investigation. The displayed FESEM image presents block shaped nanostructures of Ni2.5Co5C2O4 (Fig. 1e and f). Close inspection reveals a smooth surface along with a clear boundary, thereby indicating sturdy close-packed interaction among the crystallites. Elemental distribution was further determined by energy-dispersive X-ray spectroscopy (EDS) and mapping investigation (Fig. 1g–l). It shows uniform spreading of Ni and Co throughout the framework with a Ni/Co ratio of about 1
:
2. TEM study also shows a block-like morphology, which is formed from self-assembly of porous sheet-like nanostructures (Fig. 1m–o). The selected area electron diffraction (SAED) image indeed shows crystalline features of the sample which is further confirmed by 0.191 nm interplanar distance, indicating the (602) plane from HRTEM analysis (Fig. 1o and p). To study the morphological evolution, time dependent synthesis was carried out at different time intervals from 15 min to 60 min (see Fig. S3, ESI†). However, no specific change in morphology was observed from 15 min to 2 h. As a control experiment, phase pure CoC2O4, NiC2O4 and NiCo2O4 samples were prepared and subjected to microstructural analysis. Fig. S4a–c (ESI†) shows the nano-rod like architecture of CoC2O4 with a smooth surface. The TEM image presents a controllable 1-D microstructure with 150–250 nm diameter (see Fig. S5a–c, ESI†). The HRTEM image indicates 0.296 nm lattice fringes for the (400) plane (see Fig. S3d, ESI†). NiC2O4 reveals an irregular sheet-like architecture with a packed-surface, thus further indicating that nano-block units self-assembled to grow such an architecture (see Fig. S6a–c, ESI†). TEM investigation demonstrates the formation of sheet-like nanostructures with 0.390 nm interplanar spacing of the (004) plane (see Fig. S7a–d, ESI†). For comparison, NiCo2O4 was synthesized by high-temperature thermal treatment of the as-prepared Ni2.5Co5C2O4. The XRD pattern confirms the formation of phase pure NiCo2O4 and absence of any impurity phase such as NiO and Co3O4 (see Fig. S8a, ESI†). FESEM study reveals a block-like nano-porous network, thus indicating the formation of porosity due to thermal decomposition of oxalate during the high temperature treatment (see Fig. S8b and c, ESI†). EDS and elemental mapping indicate the distribution of Ni and Co throughout the framework with a Ni/Co ratio of about 1
:
2, which is further supported by ICP-AES analysis (see Fig. S8d–j, Table S3, ESI†). The TEM image indicates a micro-sheet like framework with 0.234 and 0.245 nm lattice fringes for (222) and (311) planes respectively (see Fig. S9a–d, ESI†). Close inspection shows that small nanoparticles assembled together to form a micro-sheet like architecture, leading to uniform distribution of interparticle porosity.
2.3 Electrochemical study
To investigate the electrochemical activity, working electrodes were prepared with different loading amounts of Ni2.5Co5C2O4 by the drop-casting technique. As shown in Fig. 2a, the best performance is obtained for 4 mg cmgeo−2 catalyst loading using carbon paper as the substrate. For the benchmark value of 10 mA cmgeo−2, Ni2.5Co5C2O4 consumes 330 mV overpotential for 4 mg cm−2 catalyst loading (see Table S4, ESI†).39 However, the catalytic activity of the carbon paper supported electrocatalyst was also compared to that obtained with glassy carbon as the substrate. As displayed in Fig. S10,† the glassy carbon drop-cast electrocatalyst showed lower mass activity at 1.7 V vs. RHE, showing the contribution from the porous network of carbon paper that could lead to better electrolyte diffusion for improved electrocatalytic performance of the Ni2.5Co5C2O4 sample. However, a comparative study was also carried out with this optimized loading of 4 mg cmgeo−2 for carbon paper supported reference samples such as CoC2O4, NiC2O4 and standard RuO2. To investigate the electrochemical features, the CV technique was used with correction for uncompensated solution resistance (Ru) for all the electrocatalysts (Fig. S11†). The overpotential to reach the benchmark of 10 mA cmgeo−2 is 370, 540 and 350 mV for CoC2O4, NiC2O4 and standard RuO2, respectively (Fig. 2b). Again, the OER activity of Ni2.5Co5C2O4 is superior compared to that of NiCo2O4, which requires 410 mV overpotential for reaching 10 mA cmgeo−2. The Tafel plot was recorded to study the OER kinetics of electrocatalysts. Fig. 2c shows a lower value of the Tafel slope for Ni2.5Co5C2O4 (81 mV dec−1) compared to control samples, such as CoC2O4 (90 mV dec−1), NiC2O4 (229 mV dec−1) and NiCo2O4 (121 mV dec−1), suggesting the relatively rapid kinetics for Ni2.5Co5C2O4 compared to others.35 However, the state-of-the-art RuO2 electrocatalyst exhibited 79 mV dec−1 Tafel slope, which is the best among all the samples (see Table S5, ESI†). Composition optimization was performed with the variation of Ni/Co amount, and the best activity was observed for a Ni/Co ratio of about 2.5/5, which is indexed as Ni2.5Co5C2O4 (see Fig. 2d, S12a and Table S6, ESI†). Thereafter, the process parameter for the synthesis of the Ni2.5Co5C2O4 catalyst was further optimized, such as the amount of oxalic acid, reaction temperature and time. The best performance was obtained with 12 mmol oxalic acid, 80 °C reaction temperature and 2 hours reaction time (see Fig. S12b–d and Tables S7–S9, ESI†). In addition, the OER mechanism for Ni2.5Co5C2O4 was investigated with the variation of pH of electrolytes (12, 12.5, 13, 13.5 and 14) under alkaline conditions (Fig. 2e). Proton reaction order is considered as the most convenient parameter to study the mechanistic pathway and estimated from the following equation:
where ρRHE refers to the proton reaction order on the RHE scale.40 The result suggests that the proton reaction order (ρRHE) for the Ni2.5Co5C2O4 is 0.80, thus proposing proton decoupled electron transfer (PDET) trajectory for the alkaline OER process (Fig. 2f). The deprotonation pathway is presumed to proceed as follows: OOH(ads) + OH− → OO(ads) + H+ + e− and the decoupling assisted deprotonation mechanism is most consistent with it.41
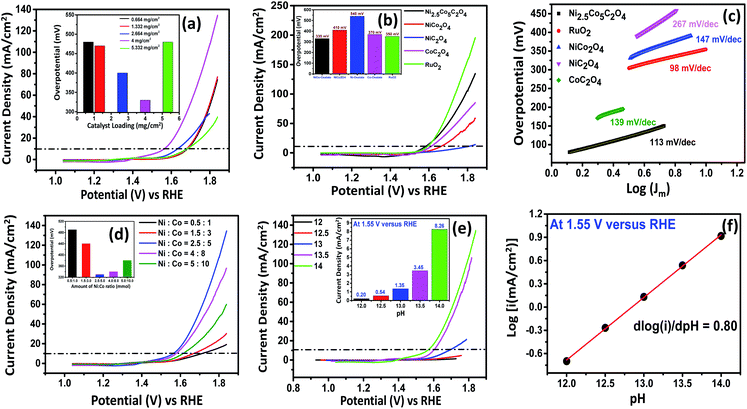 |
| Fig. 2 (a) iR-corrected LSV scan for different loading amounts for Ni2.5Co5C2O4, (b) iR-corrected LSV scan for different electrocatalysts [the inset showing the overpotential value to reach the benchmark of 10 mA cmgeo−2], (c) Tafel slope analysis of different electrocatalysts, (d) iR-corrected LSV scan for different Ni/Co ratios, (e) LSV scan and (f) linear fit of Ni2.5Co5C2O4 with the variation of pH. | |
Fig. S13† presents the magnified CV data for both Ni2.5Co5C2O4 and NiCo2O4 samples. From the as-displayed oxidation and reduction peaks, higher current density and peak area are noted for the Ni2.5Co5C2O4 compared to NiCo2O4. This also indicates that improved geometric OER performance may be attributed to the presence of more number of accessible active sites in the alkaline medium for the Ni2.5Co5C2O4 sample.42 Further, the requirement of 10 mA cm−2 was achieved at 1.56 V vs. RHE for Ni2.5Co5C2O4, whereas NiCo2O4 delivered 1.64 V vs. RHE for the same. It unequivocally implies the higher geometrical OER activity of Ni2.5Co5C2O4 than NiCo2O4. To gather further support, double layer capacitance (Cdl) was calculated that was indefinitely assumed as a non-destructive parameter for the electrochemical active surface area (ECSA) calculations.43 The Cdl value for Ni2.5Co5C2O4 is calculated to be 1.31 mF cm−2, whereas NiCo2O4 shows 2.84 mF cm−2 just before precondition (Fig. 3a and d). However, the Cdl value is significantly increased up to 10.61 mF cm−2 after electrochemical precondition for Ni2.5Co5C2O4, while the corresponding value for NiCo2O4 remains nearly the same at 2.93 mF cm−2 (Fig. 3b and e). Thus development of a higher number of accessible active sites is observed during electrochemical precondition for Ni2.5Co5C2O4 compared to the NiCo2O4 sample (Fig. 3c and f).44 This also suggests more ECSA and thus more surface coverage (θ) that provides a higher value of current (j) according to the following expression:
where the current (
j) value is directly proportional to surface coverage (
θ) involving *OOH or *OH sites, [OH
−] concentration and exponential factor which actually depends on the adsorbed surface of intermediate species for the alkaline OER.
40 The change in
Cdl is also estimated for CoC
2O
4 and NiC
2O
4 samples that further supports the better electrochemical performance of CoC
2O
4 compared to NiC
2O
4 for the alkaline OER (see Fig. S14 and S15, ESI
†).
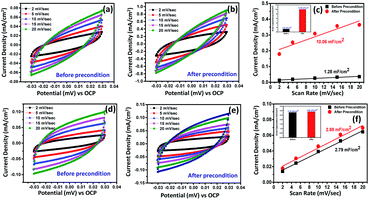 |
| Fig. 3 CV curves recorded at different scan rates to estimate the double layer capacitance (Cdl) for (a and b) Ni2.5Co5C2O4 and (d and e) NiCo2O4. Linear fit for the estimation of double layer capacitance (Cdl) from the DLC current vs. scan rate plot for (c) Ni2.5Co5C2O4 and (f) NiCo2O4. | |
To further study the electrochemical properties, electrochemical impedance spectroscopy (EIS) was employed to evaluate the charge transfer resistance (Rct) and transport kinetics. As expected, Ni2.5Co5C2O4 delivers a lower Rct value of about 6.34 ohm in comparison with NiCo2O4 (26.14 ohm), thereby indicating the occurrence of rapid charge transport phenomena for Ni2.5Co5C2O4 (Fig. 4a).45 Likewise, NiC2O4 shows a higher Rct value than CoC2O4, justifying the higher conducting behaviour of CoC2O4 in alkaline medium (see Fig. S16, ESI†). To study the intrinsic activity of the drop-cast catalyst, BET normalized electrochemical performance was already reported as the most convenient analytical technique by Shao-Horn and co-workers.46 As shown in Fig. 4b, the nitrogen adsorption–desorption isotherm displays a type-IV isotherm and H-3 hysteresis, reflecting a mesoporous network and slit-like pore geometry respectively.47 The BET surface area is found to be 25 and 49 m2 g−1 for Ni2.5Co5C2O4 and NiCo2O4 respectively, which could be attributed to thermal decomposition of oxalate ligand during calcination treatment at high-temperature. Fig. 4c presents the comparative survey of intrinsic alkaline OER performance for both the samples. From the BET normalized LSV plot, Ni2.5Co5C2O4 shows a current density of about 0.052 mA cmBET−2, which is 5.7 fold greater than that of NiCo2O4 at 1.7 V vs. RHE. In addition, the geometrical performance of Ni2.5Co5C2O4 is also superior to that of NiCo2O4 for the OER in alkaline medium (Fig. 4d). This result indicates the superiority of Ni2.5Co5C2O4 over its widely used high temperature calcined product of NiCo2O4 from both intrinsic and geometrical aspects for the OER under alkaline conditions.48 To evaluate the applicability for long-term usage, the stability test of the Ni2.5Co5C2O4 catalyst was performed for 24 hours by the chronoamperometry technique (Fig. 4e). Alkaline OER performance is relatively stable up to the initial 12 hours of the experiment; thereafter, the performance slightly decreased with time. After the stability test, the Ni2.5Co5C2O4 sample achieves 10 mA cmgeo−2 with around 350 mV overpotential, which is roughly 20 mV more than that of the as-prepared electrocatalyst (inset of Fig. 4e). Interestingly, the Ni/Co ratio is found to be 1
:
2.38, indicating mechanical inertness of the electrocatalyst even after the long-term 24 h stability test at pH = 14.
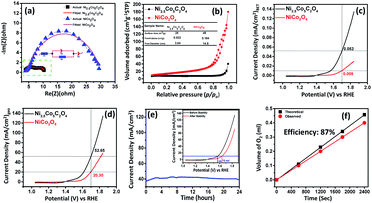 |
| Fig. 4 (a) Nyquist plots of different electrocatalysts, (b) N2 adsorption–desorption isotherm of Ni2.5Co5C2O4 and NiCo2O4 samples, catalytic performance in terms of (c) BET surface area normalised LSV plot and (d) geometrical area normalised LSV plot for Ni2.5Co5C2O4 and NiCo2O4 samples, (e) stability study of the best catalyst Ni2.5Co5C2O4 [the inset showing the overpotential value to reach the benchmark of 10 mA cmgeo−2], and (f) analysis of faradaic efficiency for the Ni2.5Co5C2O4. | |
Finally, faradaic efficiency was studied and the obtained value for Ni2.5Co5C2O4 is 87% (Fig. 4f). It indeed denotes sufficiently high activity of the electrocatalyst for alkaline water oxidation reaction. Turnover frequency (TOF) is one of the essential parameters to examine the intrinsic performance of the electrocatalyst. The TOF is expressed as follows:
where
F,
n and
j denote the Faraday constant (96
![[thin space (1/6-em)]](https://www.rsc.org/images/entities/char_2009.gif)
485C mol
−1), moles of the catalytically active species and geometrical area (SA
geo) normalized current density (mA cm
−2)
geo.
49 The amount of active elemental species was calculated from ICP-AES study (see Tables S2 and S3, ESI
†). The TOF value is found to be 3.28 × 10
−3 s
−1 for Ni
2.5Co
5C
2O
4, whereas the value for NiCo
2O
4 is 5.89 × 10
−4 s
−1 at 1.63 V
vs. RHE, indicating 5.56 fold increased intrinsic superiority of Ni
2.5Co
5C
2O
4 over NiCo
2O
4, which is also previously supported by BET normalised LSV investigation. Furthermore, the electrochemical activity of Ni
2.5Co
5C
2O
4 is almost found to be comparable with that of earlier reported non-precious electrocatalysts and standard RuO
2 for the alkaline OER (see Table S10, ESI
†).
19–24,29,50–53
2.4 Post-catalytic analysis
To understand the post-catalytic transformation, the carbon paper supported catalyst was subjected to composition and microstructural analysis. As evident from Fig. S17 (ESI†), no characteristic XRD pattern of the post-catalytic sample is observed except the sharp conspicuous diffraction signal which is assigned to the peaks from carbon paper. However, XPS measurements clearly show the structural features and elemental composition of the post-catalytic sample (see Fig. S18, ESI†). The C 1s pattern shows the appearance of two new signals around 291.77 and 294.76 eV, indicating the existence of a K+ adsorbed microstructural framework for the post-catalytic sample (see Fig. S18a, ESI†).37 From the Co 2p narrow scan, the peaks around 780.25 and 796.15 eV could corroborate the existence of Co3+ in the post-catalytic network (see Fig. S18b, ESI†).36 Further, the presence of satellite peaks around 785.33 and 802.35 eV confirms the same.54 Likewise, distinct XPS signals around 855.95 and 874.1 eV along with satellite peaks around 861.54 and 880.01 eV confirm the presence of Ni3+ (see Fig. S18c, ESI†).37 However, the O 1s spectrum shows peaks around 530.01, 531.08 and 534.69 eV particularly for lattice oxygen, hydroxyl groups (adsorbed) and lattice water molecules respectively (see Fig. S18d, ESI†). Thus, composition analysis clearly shows that almost all Ni2+ and Co2+ oxidizes into Ni3+ and Co3+ respectively under high oxidation potential gradient during the OER process. Furthermore, inductively coupled plasma-atomic emission spectroscopy (ICP-AES) study reveals a Ni/Co ratio of about 1
:
2.15 for the post-catalyst sample, thereby confirming the absence of any such metal ion leaching during the OER for Ni2.5Co5C2O4 that further confirms the mechanical stability of the working electrode in alkaline medium. The morphology of the post-OER sample was investigated by FESEM and TEM. The FESEM image shows flake-like assembly, which confirms structural transformation from a block to flake-like nanostructure under alkaline OER conditions (see Fig. S19a–d, ESI†). In addition, TEM investigation displays microstructural aggregation throughout the mesoporous network of the post-catalytic sample with lattice fringes of 0.220 nm representing the (006) plane of CoOOH (ICDD no. 014-0673).48 EDS and elemental mapping further reveal the uniform spreading of Ni, Co, C and O for the post-catalytic sample (see Fig. S19e–j, ESI†). For further validation, Raman measurements were performed to examine the in situ transformation of the catalyst during electrochemical precondition treatment. The Raman signals appear at 186, 470, 510 and 666 cm−1 for Ag, Eg, F2g and A1g respectively, confirming the formation of oxyhydroxide species from nickel cobalt oxalate (see Fig. S20, ESI†).20,29
2.5 Computational study
To get better insight into the structure–catalytic property relationship of the designed materials, we have performed density functional theory (DFT) study based on first-principles calculations using the Vienna Ab Initio Simulation Package (VASP).55 As revealed from PXRD analysis, the highest intensity planes namely (311) for NiCo2O4 and (202) for NiC2O4, CoC2O4 and Ni2.5Co5C2O4 are considered to be the active planes for the electrochemical OER and all the DFT studies were performed on NiCo2O4 (311), NiC2O4 (202), CoC2O4 (202) and Ni2.5Co5C2O4 (202) surfaces. The NiCo2O4 (311) surface contains surface exposed Ni2+ and Co3+ ions which are bi and tri-coordinated respectively while both NiC2O4 (202) and CoC2O4 (202) surfaces consist of tri-coordinated surface exposed Ni2+ and Co2+ respectively (see Fig. S21a–c, ESI†). On the contrary, the Ni2.5Co5C2O4 (202) surface contains both surface exposed Ni2+ and Co2+ (see Fig. S21d, ESI†). However, all of the oxalates have bulk tetra and penta-coordinated Ni2+ and Co2+ ions (see Fig. S21, ESI†). The M–OH bond strength for the metal-based electrocatalysts is considered to be the key descriptor for OER activity.56–60 The weaker the M–OH bond strength, the higher will be the activity. The more stable OH adsorption site for the NiCo2O4 (311) surface is Co3+ with a binding energy (B. E.) of −0.65 eV while those for NiC2O4 and CoC2O4 (202) surfaces are −1.15 and −0.46 eV respectively (see Fig. 5a–c, S22 and Table S11, ESI†). On the other hand, tri-coordinated surface Ni2+ ion is the most stable site for OH adsorption with a B.E. of −0.23 eV (see Fig. S23 and Table S11, ESI†). Hence, the M–OH bond strength follows the order Ni2.5Co5C2O4 (202) < CoC2O4 (202) < NiCo2O4 (311) < NiC2O4 (202). In addition, Bader charge analysis61,62 exhibits a charge transfer of 0.28|e| from adsorbed OH (OH*) to the Ni2.5Co5C2O4 (202) catalyst surface, indicating relatively weaker charge transfer interaction compared to CoC2O4 (0.4|e|), NiCo2O4 (0.65|e|) and NiC2O4 (0.84|e|) surfaces. The charge density difference (CDD) plot further confirms the charge transfer from OH* to different surfaces (Fig. S24†). Therefore, with relatively weaker M–OH bonding interaction, the Ni2.5Co5C2O4 (202) catalyst surface is superior to the other surfaces and consequently the order of OER activity is found to be NiC2O4 (202) < NiCo2O4 (311) < CoC2O4 (202) < Ni2.5Co5C2O4 (202), which very nicely corroborates the experimental findings (vide infra). The bonding interaction between adsorbed OH and interacting Ni in OH*–Ni2.5Co5C2O4 (202) can be further confirmed from the density of states (DOS) plot (asterisk indicates the contribution from O-2p) as shown in Fig. 5d. The generation of the O-2p state can be clearly visualized near −5.95 eV (as marked with a black box) in the DOS plot for OH*–Ni2.5Co5C2O4 (202) (Fig. 5e). Besides, projected density of states (PDOS) analysis further confirms the bonding interaction between O-2pz and Ni-dxy orbitals which indicates that hybridization mainly occurs between these orbitals (Fig. 5f).
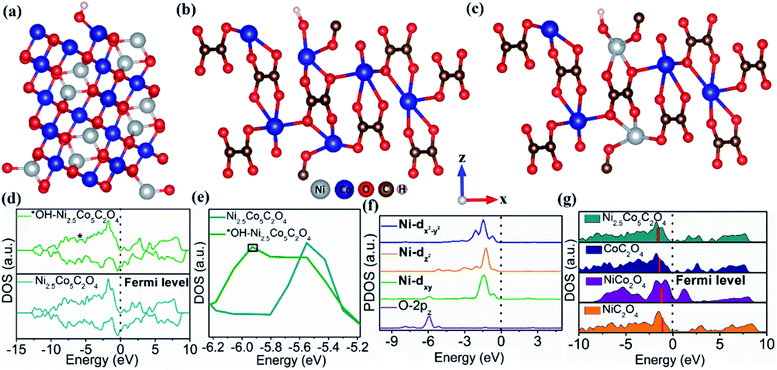 |
| Fig. 5 Optimized structure of OH* adsorbed (a) NiCo2O4 (311), (b) CoC2O4 (202), and (c) Ni2.5Co5C2O4 (202) surfaces, (d) DOS plots for Ni2.5Co5C2O4 (202) and OH*–Ni2.5Co5C2O4 (202) surfaces (asterisk indicates the contribution from O-2p), (e) magnified DOS (the O-2p state is situated at around −5.95 eV as marked by a black box in the OH*–Ni2.5Co5C2O4 (202) DOS), (f) projected density of states (PDOS) for O-2pz, Ni-dxy, Ni-dz2 and Ni-dx2–y2 of OH*–Ni2.5Co5C2O4 (202), and (g) DOS plots for different catalyst surfaces. The thick red bar indicates the position of the d-band centre (Ed) on catalyst surfaces. | |
From the above discussion, it can be concluded that the adsorption of OH is weaker on the Ni2.5Co5C2O4 (202) catalyst surface and hence exhibiting better OER activity and this conclusion can be further justified by a d-band model which correlates the d-band centre (DBC) with the adsorption energies of the intermediates (here OH).63–65 The positions of DBCs are −1.50, −1.37, −1.26 and −1.07 eV respectively for Ni2.5Co5C2O4 (202), CoC2O4 (202), NiCo2O4 (311) and NiC2O4 (202) surfaces (Fig. 5g) which vividly shows that the DBC gradually shifts towards the Fermi level from Ni2.5Co5C2O4 to the NiC2O4 (202) surface. The closer the DBC to the Fermi level, the stronger will be the adsorption of OH and hence deterioration of OER activity. This finding overwhelmingly supports our conclusion that the Ni2.5Co5C2O4 catalyst is the best surface for the OER with weaker OH adsorption ability while NiC2O4 is the least active with stronger OH binding ability. The present study highlights the benefit of nickel–cobalt oxalate over nickel–cobalt oxide for the alkaline OER process. We also believe that the use of oxalate ligand could be a promising strategy for the fabrication of new-generation electrocatalysts for electrochemical water oxidation in alkaline medium.
3. Conclusions
In summary, nickel–cobalt oxalate (Ni2.5Co5C2O4) based block-like nanostructures were prepared by a wet-chemical technique in an aqueous medium and further treated by calcination at 350 °C for the synthesis of nickel cobalt oxide (NiCo2O4). The preparation method was optimized in terms of Ni/Co ratio, oxalic acid amount, reaction temperature and time. The working electrode fabrication was performed on carbon paper as the substrate surface maintaining an optimum loading of the electrocatalyst. Next, Ni2.5Co5C2O4 achieves 10 mA cmgeo−2 with 330 mV overpotential, whereas NiCo2O4 requires 410 mV for the same. The comparative study suggests the best electrochemical catalytic efficiency for Ni2.5Co5C2O4, and that can be attributed to lower charge transfer resistance, more changes in double layer capacitance during precondition in alkaline medium, and high surface coverage. DFT study reveals that comparatively weaker OH adsorption as well as favourable d-band centre (DBC) position makes the Ni2.5Co5C2O4 catalyst the best surface for the OER compared to individual CoC2O4 and NiC2O4 as well as well studied NiCo2O4. This report thoroughly compares the activity of Ni2.5Co5C2O4 and NiCo2O4 for the alkaline OER process and highlights the benefit of Ni2.5Co5C2O4 over NiCo2O4. The prudent choice of the oxalate based ligand-assisted synthetic approach could be used for other transition metals to execute bulk-scale alkaline water oxidation for a clean ecosystem and sustainable living.
Conflicts of interest
There are no conflicts to declare.
Acknowledgements
The author Sourav Ghosh would like to acknowledge the Department of Science and Technology (DST), India, for providing National Postdoctoral Fellowship (PDF/2017/001728/ES). RJ thanks IACS and DST. For their fellowships, HRI, GT, and HVSRMK thank UGC, RGNF UGC and DST-Inspire, respectively. The authors also acknowledge IISER Kolkata for instrumental facilities (including “TEM, DST-FIST facility, IISER, Kolkata, India” for providing electron microscopy), “DST and SAIF, IIT Bombay” for ICP-AES analysis and IACS for XPS facility. R. J. thanks IACS and A. D. acknowledges DIA-SERB for funding computational resources. Dr Sourav Ghosh also acknowledges Technical Research Centre project (AI/1/64/SNB/2014) of S. N. Bose National Centre for Basic Sciences, Kolkata, India, for financial support. Dr Sourav Ghosh also thanks Dr Subhra Jana, Dr Mohua Chakraborty, Dr Saptarshi Pal, Ayan Mondal and Ruma Ghosh for helpful suggestions.
References
- A. Sivanantham, P. Ganesan, A. Vinu and S. Shanmugam, Surface Activation and Reconstruction of Non-Oxide-Based Catalysts Through In Situ Electrochemical Tuning for Oxygen Evolution Reactions in Alkaline Media, ACS Catal., 2020, 10, 463 CrossRef CAS.
- D. Li, J. Sun, R. Ma and J. Wei, High-efficient and Low-cost H2 Production by Solar-driven Photo-thermo-reforming of Methanol with CuO Catalyst, ES Energy Environ., 2020, 9, 82 CAS.
- P. Yang, H. Zhao, Y. Yang, P. Zhao, X. Zhao and L. Yang, Fabrication of N, P-Codoped Mo2C/Carbon Nanofibers Via Electrospinning as Electrocatalyst for Hydrogen Evolution Reaction, ES Mater. Manuf., 2020, 7, 34 CAS.
- Y. Lv, L. Zhu, H. Xu, L. Yang, Z. Liu, D. Cheng, X. Cao, J. Yun and D. Cao, Core/Shell Template-Derived Co, N-Doped Carbon Bifunctional Electrocatalysts for Rechargeable Zn–Air Battery, Eng. Sci., 2019, 7, 26 Search PubMed.
- Z. Cai, P. Wang, J. Yang and X. Wang, Update on Recent Designing Strategies of Transition Metal-Based Layered Double Hydroxides Bifunctional Electrocatalysts, ES Energy Environ., 2019, 5, 22 Search PubMed.
- J. Suntivich, K. J. May, H. A. Gasteiger, J. B. Goodenough and Y. A. Shao-Horn, Perovskite Oxide Optimized for Oxygen Evolution Catalysis from Molecular Orbital Principles, Science, 2011, 334, 1383 CrossRef CAS PubMed.
- M. Chatti, A. M. Glushenkov, T. Gengenbach, G. P. Knowles, T. C. Mendes, A. V. Ellis, L. Spiccia, R. K. Hocking and A. N. Simonov, Highly Dispersed and Disordered Nickel–Iron Layered Hydroxides and Sulphides: Robust and High-Activity Water Oxidation Catalysts, Sustainable Energy Fuels, 2018, 2, 1561 RSC.
- Z. P. Wu, X. F. Lu, S. Q. Zang and X. W. Lou, Non-Noble–Metal-based Electrocatalysts toward the Oxygen Evolution Reaction, Adv. Funct. Mater., 2020, 30, 1910274 CrossRef CAS.
- D. Xu, M. B. Stevens, Y. Rui, G. DeLuca, S. W. Boettcher, E. Reichmanis, Y. Li, Q. Zhang and H. Wang, The Role of Cr Doping in NiFe Oxide/(oxy)hydroxide Electrocatalysts for Oxygen Evolution, Electrochim. Acta, 2018, 265, 10 CrossRef CAS.
- S. Jin, Are Metal Chalcogenides, Nitrides, and Phosphides Oxygen Evolution Catalysts or Bifunctional Catalysts?, ACS Energy Lett., 2017, 2, 1937 CrossRef CAS.
- D. Y. Chung, S. W. Jun, G. Yoon, H. Kim, J. M. Yoo, K.-S. Lee, T. Kim, H. Shin, A. K. Sinha, S. G. Kwon, K. Kang, T. Hyeon and Y.-E. Sung, Large-Scale Synthesis of Carbon–Shell-Coated FeP Nanoparticles for Robust Hydrogen Evolution Reaction Electrocatalyst, J. Am. Chem. Soc., 2017, 139, 6669 CrossRef CAS PubMed.
- N.-T. Suen, S.-F. Hung, Q. Quan, N. Zhang, Y.-J. Xu and H. M. Chen, Electrocatalysis for the Oxygen Evolution Reaction: Recent Development and Future Perspectives, Chem. Soc. Rev., 2017, 46, 337 RSC.
- C. Zhang, Y. Xie, H. Deng, C. Zhang, J. Su and J. Lin, Nitrogen Doped Coal with High Electrocatalytic Activity for Oxygen Reduction Reaction, Eng. Sci., 2019, 8, 39 Search PubMed.
- H. Wu, Y. Zhang, R. Yin, W. Zhao, X. Li and L. Qian, Magnetic negative permittivity with dielectric resonance in random Fe3O4@graphene–phenolic resin composites, Adv. Compos. Hybrid Mater., 2018, 1, 168 CrossRef CAS.
- P. Xie, Y. Liu, M. Feng, M. Niu, C. Liu, N. Wu, K. Sui, R. R. Patil, D. Pan, Z. Guo and R. Fan, Hierarchically porous Co/C nanocomposites for ultralight high-performance microwave absorption, Adv. Compos. Hybrid Mater., 2021, 4, 173 CrossRef CAS.
- J. Qi, W. Zhang and R. Cao, Aligned Cobalt-Based Co@CoOx Nanostructures for Efficient Electrocatalytic Water Oxidation, Chem. Commun., 2017, 53, 9277 RSC.
- H. Yu, L. Yang, D. Cheng and D. Cao, Zeolitic-imidazolate Framework (ZIF)@ZnCo-ZIF Core–shell Template-derived Co, N-doped Carbon Catalysts for Oxygen Reduction Reaction, Eng. Sci., 2018, 3, 54 Search PubMed.
- G. Li, C. Dang, Y. Hou, F. Dang, Y. Fan and Z. Guo, Experimental and Theoretical Characteristic of Single Atom Co–N–C Catalyst for Li–O2 Batteries, Eng. Sci., 2020, 10, 85 CAS.
- P. Chen, K. Xu, Z. Fang, Y. Tong, J. Wu, X. Lu, X. Peng, H. Ding, C. Wu and Y. Xie, Metallic Co4N Porous Nanowire Arrays Activated by Surface Oxidation as Electrocatalysts for the Oxygen Evolution Reaction, Angew. Chem., 2015, 127, 14923 CrossRef.
- S. Ghosh, G. Tudu, A. Mondal, S. Ganguli, H. R. Inta and V. Mahalingam, Inception of Co3O4 as Microstructural Support to Promote Alkaline Oxygen Evolution Reaction for Co0.85Se/Co9Se8 Network, Inorg. Chem., 2020, 59, 17326 CrossRef CAS PubMed.
- A. Mendoza-Garcia, D. Su and S. Sun, Sea Urchin-like Cobalt–Iron Phosphide as an Active Catalyst for Oxygen Evolution Reaction, Nanoscale, 2016, 8, 3244 RSC.
- S. Klemenz, J. Schuch, S. Hawel, A.-M. Zieschang, B. Kaiser, W. Jaegermann and B. Albert, Synthesis of a Highly Efficient Oxygen-Evolution Electrocatalyst by Incorporation of Iron into Nanoscale Cobalt Borides, ChemSusChem, 2018, 11, 3150 CrossRef CAS PubMed.
- Y. Xue, Y. Wang, H. Liu, X. Yu, H. Xue and L. Feng, Electrochemical Oxygen Evolution Reaction Catalyzed by a Novel Nickel–Cobalt–Fluoride Catalyst, Chem. Commun., 2018, 54, 6204 RSC.
- P. K. Katkar, S. J. Marje, S. B. Kale, A. C. Lokhande, C. D. Lokhande and U. M. Patil, Synthesis of Hydrous Cobalt Phosphate Electro-catalysts by a Facile Hydrothermal Method for Enhanced Oxygen Evolution Reaction: Effect of Urea Variation, CrystEngComm, 2019, 21, 884 RSC.
- S. Ganguli, S. Das, S. Kumari, H. R. Inta, A. K. Tiwari and V. Mahalingam, Effect of Intrinsic Properties of Anions on the Electrocatalytic Activity of NiCo2O4 and NiCo2OxS4−x Grown by Chemical Bath Deposition, ACS Omega, 2018, 3, 9066 CrossRef CAS PubMed.
- J. S. Yeoh, C. F. Armer and A. Lowe, Transition Metal Oxalates as Energy Storage Materials: A review, Mater. Today Energy, 2018, 9, 198 CrossRef.
- J. W. Kim, J. K. Lee, D. Phihusut, Y. Yi, H. J. Lee and J. Lee, Self-Organized One-Dimensional Cobalt Compound Nanostructures from CoC2O4 for Superior Oxygen Evolution Reaction, J. Phys. Chem. C, 2013, 117, 23712 CrossRef CAS.
- D. Phihusut, J. D. Ocon, B. Jeong, J. W. Kim, J. K. Lee and J. Lee, Gently Reduced Graphene Oxide Incorporated into Cobalt Oxalate Rods as Bifunctional Oxygen Electrocatalyst, Electrochim. Acta, 2014, 140, 404 CrossRef CAS.
- X. Liu, J. Jiang and L. Ai, Non-Precious Cobalt Oxalate Microstructures as Highly Efficient Electrocatalysts for Oxygen Evolution Reaction, J. Mater. Chem. A, 2015, 3, 9707 RSC.
- H. B. Wu, H. Pang and X. W. Lou, Facile Synthesis of Mesoporous Ni0.3Co2.7O4 Hierarchical Structures for High-Performance Supercapacitors, Energy Environ. Sci., 2013, 6, 3619 RSC.
- L. Wang, R. Zhang, Y. Jiang, H. Tian, Y. Tan, K. Zhu, Z. Yu and W. Li, Interfacial synthesis of micro-cuboid Ni0.55Co0.45C2O4 solid solution with enhanced electrochemical performance for hybrid supercapacitors, Nanoscale, 2019, 11, 13894 RSC.
- S. Ghosh, M. Roy and M. K. Naskar, A Facile Soft Chemical Synthesis of Cube-Shaped Mesoporous CuO with Microcarpet-Like Interior, Cryst. Growth Des., 2014, 14, 2977 CrossRef CAS.
- H.-J. Oh, C.-H. Jo, C.-S. Yoon, H. Yashiro, S.-J. Kim, S. Passerini, Y.-K. Sun and S.-T. Myung, Nickel Oxalate Dihydrate Nanorods Attached to Reduced Graphene Oxide Sheets as a High Capacity Anode for Rechargeable Lithium Batteries, NPG Asia Mater., 2016, 8, e270–e277 CrossRef CAS.
- M. S. Loeian, D. A. Ziolkowska, F. Khosravi, J. B. Jasinski and B. Panchapakesan, Exfoliated WS2–Nafion Composite Based Electromechanical Actuators, Sci. Rep., 2017, 7, 14599 CrossRef PubMed.
- S. Ghosh, H. R. Inta, S. Ganguli, G. Tudu, H. V. S. R. M. Koppisetti and V. Mahalingam, MoO2 as a Propitious “Pore Forming Additive” for Boosting Water Oxidation Activity of Cobalt Oxalate Microrods, J. Phys. Chem. C, 2020, 124, 20010 CrossRef CAS.
- P. W. Menezes, A. Indra, D. González-Flores, N. R. Sahraie, I. Zaharieva, M. Schwarze, P. Strasser, H. Dau and M. Driess, High-Performance Oxygen Redox Catalysis with Multifunctional Cobalt Oxide Nanochains: Morphology Dependent Activity, ACS Catal., 2015, 5, 2017 CrossRef CAS.
- S. Ganguli, S. Ghosh, S. Das and V. Mahalingam, Inception of Molybdate as a “Pore Forming Additive” to Enhance the Bifunctional Electrocatalytic Activity of Nickel and Cobalt based Mixed Hydroxides for Overall Water Splitting, Nanoscale, 2019, 11, 16896 RSC.
- Y. Wei, X. Ren, H. Ma, X. Sun, Y. Zhang, X. Kuang, T. Yan, H. Ju, D. Wu and Q. Wei, CoC2O4·2H2O Derived Co3O4 Nanorods Array: A High-Efficiency 1D Electrocatalyst for Alkaline Oxygen Evolution Reaction, Chem. Commun., 2018, 54, 1533 RSC.
- C. Wei and Z. J. Xu, The Comprehensive Understanding of 10 mA cmgeo−2 as an Evaluation Parameter for Electrochemical Water Splitting, Small Methods, 2018, 2, 1800168 CrossRef.
- C. Yang, C. Laberty-Robert, D. Batuk, G. Cibin, A. V. Chadwick, V. Pimenta, W. Yin, L. Zhang, J. Tarascon and A. Grimaud, Phosphate Ion Functionalization of Perovskite Surfaces for Enhanced Oxygen Evolution Reaction, J. Phys. Chem. Lett., 2017, 8, 3466 CrossRef CAS PubMed.
- A. Grimaud, O. Diaz-Morales, B. Han, W. T. Hong, Y. L. Lee, L. Giordano, K. A. Stoerzinger, M. T. M. Koper and Y. Shao-Horn, Activating Lattice Oxygen Redox Reactions in Metal Oxides to Catalyse Oxygen Evolution, Nat. Chem., 2017, 9, 457 CrossRef CAS PubMed.
- A. Bergmann, T. E. Jones, E. M. Moreno, D. Teschner, P. Chernev, M. Gliech, T. Reier, H. Dau and P. Strasser, Unified Structural Motifs of the Catalytically Active State of Co(Oxyhydr)-Oxides During the Electrochemical Oxygen Evolution Reaction, Nat. Catal., 2018, 1, 711 CrossRef CAS.
- Y. Yoon, B. Yan and Y. Surendranath, Suppressing Ion Transfer Enables Versatile Measurements of Electrochemical Surface Area for Intrinsic Activity Comparisons, J. Am. Chem. Soc., 2018, 140, 2397 CrossRef CAS PubMed.
- C. C. L. McCrory, S. Jung, I. M. Ferrer, S. M. Chatman, J. C. Peters and T. F. Jaramillo, Benchmarking Hydrogen Evolving Reaction and Oxygen Evolving Reaction Electrocatalysts for Solar Water Splitting Devices, J. Am. Chem. Soc., 2015, 137, 4347 CrossRef CAS PubMed.
- J. Xu, J. P. S. Sousa, N. E. Mordvinova, J. D. Costa, D. Y. Petrovykh, K. Kovnir, O. I. Lebedev and Y. V. Kolen'ko, Al-Induced In Situ Formation of Highly Active Nanostructured Water-Oxidation Electrocatalyst Based on Ni–Phosphide, ACS Catal., 2018, 8, 2595 CrossRef CAS.
- C. Wei, R. Rao, J. Peng, B. Huang, I. E. L. Stephens, M. Risch, Z. J. Xu and Y. Shao-Horn, Recommended Particles and Benchmark Activity for Hydrogen and Oxygen Electrocatalysis in Water Splitting and Fuel Cells, Adv. Mater., 2019, 1806296 CrossRef PubMed.
- S. Ghosh, R. Das and M. K. Naskar, Morphologically Tuned Aluminum Hydrous Oxides and their Calcined Products, J. Am. Ceram. Soc., 2016, 99, 2273 CrossRef CAS.
- S. Ganguli, H. V. S. R. M. Koppisetti, S. Ghosh, T. Biswas and V. Mahalingam, Paradoxical Observance of “Intrinsic” and “Geometric” Oxygen Evolution Electrocatalysis in Phase-Tuned Cobalt Oxide/Hydroxide Nanoparticles, ACS Appl. Nano Mater., 2019, 2, 7957 CrossRef CAS.
- J. Saha, D. R. Chowdhury, P. Jash and A. Paul, Cobalt Phosphonates as Precatalysts for Water Oxidation: Role of Pore Size in Catalysis, Chem.–Eur. J., 2017, 23, 12519 CrossRef CAS PubMed.
- S. M. AlShehri, J. Ahmed, T. Ahamad, P. Arunachalam, T. Ahmad and A. Khan, Bifunctional Electro-Catalytic Performances of CoWO4 Nanocubes for Water Redox Reactions (OER/ORR), RSC Adv., 2017, 7, 45615 RSC.
- J. A. Vigil, T. N. Lambert and B. T. Christensen, Cobalt Phosphide-Based Nanoparticles as Bifunctional Electrocatalysts for Alkaline Water Splitting, J. Mater. Chem. A, 2016, 4, 7549 RSC.
- X. Liu, Y. Yang and S. Guan, An Efficient Electrode Based on One-Dimensional CoMoO4 Nanorods for Oxygen Evolution Reaction, Chem. Phys. Lett., 2017, 675, 11 CrossRef CAS.
- Y. Cui, Y. Xue, R. Zhang, J. Zhang, X. Li and X. Zhu, Vanadium–Cobalt Oxyhydroxide Shows Ultralow Overpotential for the Oxygen Evolution Reaction, J. Mater. Chem. A, 2019, 7, 21911 RSC.
- L. Ai, X. Gao and J. Jiang, In Situ Synthesis of Cobalt Stabilized on Macroscopic Biopolymer Hydrogel as Economical and Recyclable Catalyst for Hydrogen Generation from Sodium Borohydride Hydrolysis, J. Power Sources, 2014, 257, 213 CrossRef CAS.
- G. Kresse and J. Hafner, Ab Initio Molecular Dynamics for Liquid Metals, Phys. Rev. B: Condens. Matter Mater. Phys., 1993, 4, 558 CrossRef PubMed.
- R. Subbaraman, D. Tripkovic, K.-C. Chang, D. Strmcnik, A. P. Paulikas, P. Hirunsit, M. Chan, J. Greeley, V. Stamenkovic and N. M. Markovic, Trends in Activity for the Water Electrolyser Reactions on 3d M(Ni,Co,Fe,Mn) Hydr(oxy)oxide Catalysts, Nat. Mater., 2012, 11, 550 CrossRef CAS PubMed.
- F. Song, L. Bai, A. Moysiadou, S. Lee, C. Hu, L. Liardet and X. Hu, Transition Metal Oxides as Electrocatalysts for the Oxygen Evolution Reaction in Alkaline Solutions: An Application-Inspired Renaissance, J. Am. Chem. Soc., 2018, 140, 7748 CrossRef CAS PubMed.
- S. J. Trasatti, Electrocatalysis by Oxides-Attempt at a Unifying Approach, J. Electroanal. Chem. Interfacial Electrochem., 1980, 111, 125 CrossRef CAS.
- S. Trasatti, Electrocatalysis in the Anodic Evolution of Oxygen and Chlorine, Electrochim. Acta, 1984, 29, 1503 CrossRef CAS.
- P. Rüetschi and P. Delahay, Influence of Electrode Material on Oxygen Overvoltage: A Theoretical Analysis, J. Chem. Phys., 1955, 23, 556 CrossRef.
-
R. F. W. Bader, Atoms in Molecules: A Quantum Theory, Oxford University Press, Oxford, 1990 Search PubMed.
- G. Henkelman, A. Arnaldsson and H. Jónsson, A Fast and Robust Algorithm for Bader Decomposition of Charge Density, Comput. Mater. Sci., 2006, 36, 354 CrossRef.
- R. Jana, C. Chowdhury, S. Malik and A. Datta, Pt/Co3O4 Surpasses Benchmark Pt/C: An Approach Toward Next Generation Hydrogen Evolution Electrocatalyst, ACS Appl. Energy Mater., 2019, 2, 5613 CrossRef CAS.
- M. Mavrikakis, B. Hammer and J. K. Nørskov, Effect of Strain on the Reactivity of Metal Surfaces, Phys. Rev. Lett., 1998, 81, 2819 CrossRef.
- H. Lv, Z. Xi, Z. Chen, S. Guo, Y. Yu, W. Zhu, Q. Li, X. Zhang, M. Pan, G. Lu, S. Mu and S. A. Sun, New Core/Shell NiAu/Au Nanoparticle Catalyst with Pt-like Activity for Hydrogen Evolution Reaction, J. Am. Chem. Soc., 2015, 137, 5859 CrossRef CAS PubMed.
Footnotes |
† Electronic supplementary information (ESI) available. See DOI: 10.1039/d1na00034a |
‡ Sourav and Rajkumar contributed equally. |
|
This journal is © The Royal Society of Chemistry 2021 |
Click here to see how this site uses Cookies. View our privacy policy here.