Achieving high-rate hydrogen recovery from wastewater using customizable alginate polymer gel matrices encapsulating biomass†
Received
30th May 2018
, Accepted 29th July 2018
First published on 13th August 2018
Abstract
In addition to methane gas, higher-value resources such as hydrogen gas are produced during anaerobic wastewater treatment. They are, however, immediately consumed by other organisms. To recover these high-value resources, not only do the desired phenotypes need to be retained in the anaerobic reactor, but the undesired ones need to be washed out. In this study, a well-established alginate-based polymer gel, with and without a coating layer, was used to selectively encapsulate hydrogen-producing biomass in beads to achieve high-rate recovery of hydrogen during anaerobic wastewater treatment. The effect of cross-linking agents, Ca2+, Sr2+, and Ba2+, as well as a composite coating on the beads, consisting of alternating layers of polyethylenimine and silica hydrogel, were investigated with respect to their performance, specifically, their mass transfer characteristics and their differential ability to retain the encapsulated biomass. Although the coating reduced the escape rate of encapsulated biomass from the beads, all alginate polymer matrices without coating effectively retained biomass. Fast diffusion of dissolved organic carbon (DOC) through the polymer gel was observed in both Ca-alginate and Sr-alginate without coating. The coating, however, decreased either the diffusivity or the permeability of the DOC depending on whether the DOC was from synthetic wastewater (more lipids and proteins) or real brewery wastewater (more sugars). Consequently, the encapsulation system with coating became diffusion limited when brewery wastewater with high chemical oxygen demand was fed, resulting in a lower hydrogen production rate than the uncoated encapsulation systems. In all cases the encapsulated biomass was able to produce hydrogen, even at a hydraulic residence time of 45 min. Although there are limitations to this system, the used of encapsulated biomass for resource recovery from wastewater shows promise, particularly for high-rate systems in which the retention of specific phenotypes is desired.
Water impact
The encapsulation of selected biomass in highly customizable alginate polymer matrices provides a versatile solution for reliable recovery of resources from wastewater. This expands the possibilities of sustainable wastewater treatment and simplifies the operation of anaerobic systems, potentially making decentralized anaerobic wastewater treatment viable for facilities too small to afford the high operating requirements of conventional anaerobic technologies.
|
Introduction
Wastewater treatment is an energy-intensive process, but it is possible to recover energy and resources from wastewater via specialized treatment systems. Wastewater is rich in organic carbon from which energy is captured as methane gas through anaerobic treatment.1 In addition to methane, other high-value resources are produced by specialized phenotypes, but these compounds are typically consumed by other organisms before recovery.2,3 Hydrogen gas is a more desirable energy carrier than methane because of its high specific energy content (142 MJ kg−1vs. 55 MJ kg−1).4 Through the combination of waste pre-treatment and the selection of spore-forming hydrogen-producing Clostridium species, hydrogen production and collection from pure glucose and actual high strength waste has been achieved.5–15 In addition to hydrogen, other high-value intermediates are also recoverable from wastewater treated anaerobically, such as medium chain fatty acids (MCFA), which are used to produce biokerosene and biodiesel.16–18
Conventional resource recovery systems tend to mimic and intensify natural anaerobic systems, and hence, can be limited. State-of-the-art technologies, such as granulation, attached growth systems, and anaerobic membrane bioreactors, retain biomass, separating the solids (or biomass) retention time (SRT) from the hydraulic retention time (HRT); this reduces the risk of biomass washout and enables more flexible process control.7,13,14,19–21 These systems, however, still provide little control over the microbial community present or biomass concentration.22,23 This leads to organisms outcompeting desired phenotypes with a loss of resource production. Cell encapsulation, however, retains a selected microbial community or specific organism at a desired biomass concentration within an encapsulation matrix, separating them from other microorganisms in the system and facilitating the washout of competing or undesirable organisms.24–26
Encapsulation of catalysts, enzymes, and pure and mixed culture bacterial cells in alginate, a well characterized material consisting of alternating blocks of mannuronic acid and guluronic acid, has been successfully used to achieve contaminant biodegradation, nutrient removal, and metal sequestration.27–33 If used in wastewater treatment, alginate encapsulation could facilitate resource recovery. The sodium salt of alginate is highly soluble in water, but alginate is cross-linked by various divalent cations in a sol–gel process to form a non-toxic hydrophilic polymer that is stable in water.34,35 Ca2+ is the most widely used cross-linking agent, but is vulnerable to chelation by PO43− or exchange with monovalent cations (e.g., Na+), resulting in the disintegration of the encapsulating gel matrix.36,37 Sr2+ and Ba2+ have been reported to have higher affinities for alginate than Ca2+, and therefore yield alginate polymers with higher stabilities.28,38–40 Polyethylenimine (PEI), a polycation, has also been used to coat alginate polymers, achieving even higher stability.31,41–44 A layer-by-layer composite coating of silica gel and PEI has been developed by Lee and coworkers, further increasing the structural rigidity and longevity of encapsulation matrices.45
In this research we tested the hypothesis that encapsulation could facilitate resource recovery from wastewater in the form of hydrogen production during anaerobic treatment. Encapsulation was expected to facilitate the retention of high concentrations of specialized microorganisms in a flow-through reactor. We also hypothesized that the encapsulation chemistry, both in terms of the crosslinking cation used and the presence or absence of coating, would affect the retention of hydrogen-producing biomass and the diffusivities of substrate and product through the encapsulating matrix. Different optimal encapsulation matrices were expected, depending on operating conditions. Experiments were performed with synthetic and real brewery wastewater to determine the viability of this approach.
Materials and methods
Wastewater
The synthetic wastewater composition was modified from that of Klatt and LaPara, such that gelatin (Bovine Type B, Sigma-Aldrich), starch (Difco, BD), polysorbate 80 (Tween 80, Sigma-Aldrich), casamino acids (Fisher BioReagents) and yeast extract (Acumedia) were added to a final concentration of 3.75 g L−1, 1.75 g L−1, 0.96 g L−1, 0.08 g L−1 and 0.08 g L−1, respectively.46 All other components of the synthetic wastewater remained the same. The brewery wastewater was collected from a local brewery with a bottling line. The chemical oxygen demand (COD), total and dissolved organic carbon (TOC and DOC), NH4+ concentration, and PO43− concentration of the brewery wastewater were approximately 14 g L−1, 4 g L−1 (both TOC and DOC), 10 mg L−1, and 50 mg L−1 respectively. NH4OH (BDH, VWR Analytical, 28–30%) and CaCl2 (Merck, ≥98%) were added to the brewery wastewater to adjust the pH, increase the NH4+ concentration, and maintain bead integrity. One experiment was also performed with diluted brewery wastewater with a COD of 4 g L−1. Both synthetic wastewater and brewery wastewater were autoclaved and stored until use at 4 °C.
Hydrogen-producing culture
Spore-forming hydrogen-producing organisms were selected from anaerobic digester solids collected from the Empire Wastewater Treatment Plant (Empire Township, MN). Solids were incubated at 80 °C for 1 hour.47 The resultant biomass was inoculated into the synthetic or brewery wastewater. After cultivating the organisms at 37 °C for 1 d, the biomass was pelletized and washed three times in synthetic wastewater made without carbon sources (i.e., no gelatin, starch, polysorbate 80, casamino acids, or yeast extract). After washing, the biomass was re-suspended in the organic carbon-free synthetic wastewater and incubated again at 80 °C for 1 h to eliminate additional bacteria that could have been introduced during washing.
Cell encapsulation
A 4% (w/v) Na-alginate (Sigma, Medium viscosity) solution was made by dissolving Na-alginate into sterilized deionized (DI) water. This solution was then mixed with the buffer solution containing washed hydrogen-producing biomass at a 1
:
1 (w/w) ratio. After homogenizing, the biomass-alginate solution was added to a sterilized 60 mL syringe fit with an 18-gauge blunt needle. The solution was extruded drop by drop into at least 200 mL of CaCl2, SrCl2 (Ward's Science), or BaCl2 (ChemCruz) solution at a commonly used concentration of 0.4 M to cross-link for 24 h, forming solidified Ca-alginate, Sr-alginate, or Ba-alginate beads with typical pore sizes ranging from 5 to 200 nm (Fig. S1†).28,44,48,49 Beads were approximately 2.5 mm in diameter (Fig. S2†), with no observed size differences between Ca-alginate, Sr-alginate, or Ba-alginate beads. Abiotic beads were also made by mixing the Na-alginate solution with sterilized organic carbon-free synthetic wastewater without biomass.
To produce sheets for use in diffusion experiments, 10 g of the abiotic Na-alginate solution was placed under vacuum for 30 min, then poured into a sterile petri dish (60 mm diameter). Autoclaved BaCl2, SrCl2 or CaCl2 solutions (4% w/v) were then poured into the petri dishes to cross-link the alginate After curing for 1 h, the sheets were carefully removed from the petri dishes for analysis of their diffusion properties and thickness (described below).
To apply the composite coating, 1% (w/v) polyethyleneimine (PEI) solution was made by mixing 50% (w/v) PEI solution (Aldrich) with sterilized DI water. Tetraethyl orthosilicate (TEOS, Aldrich, 98%) was hydrolyzed by mixing with 5 mM HCl (BDH, VWR Analytical, 36.5–38%) at a 2
:
7 ratio (w/w). The hydrolyzed TEOS (HTEOS) was then diluted with DI water by 90%. The pH of the diluted HTEOS was adjusted to 7.0 with NaOH. One layer of the PEI coating was applied on the alginate beads by continuously mixing the beads in 1% PEI solution for 5 min, after which they were rinsed with DI water. To apply more layers of PEI, alternating layers of silica hydrogel, used as a bonding layer, and PEI were applied to the beads. Each silica hydrogel layer was applied by mixing the PEI-coated beads in the diluted HTEOS solution for 10 min, rinsing them with DI water, then applying another PEI layer as described above.45 Coating layers were applied to the alginate sheets in a similar manner.
Experimental set-up
Biomass retention.
Alginate beads made from 30 mL of biomass containing 2% Na-alginate solution were incubated anaerobically at 37 °C in 50 mL of synthetic wastewater that had been made without carbon sources. Abiotic alginate beads were incubated under the same conditions and served as background controls. Every other day, samples (2 mL) of the bulk solution were removed and centrifuged to pelletize any organisms present. The supernatant was decanted and replaced with 1 mL of 25% sodium dodecyl sulfate (SDS, Sigma-Aldrich, ≥85%) solution. After 3 freeze–thaw cycles, the samples were incubated in 70 °C water for 1.5 hours dissolve the cell membrane, after which the protein released from the organisms was measured with the Pierce BCA protein assay.50 The escaped biomass was calculated at each time point in the encapsulated biomass treatments by subtracting the background biomass in the bulk solution of the abiotic control reactors with error propagated.
After 30 d of incubation, the beads were dissolved in sodium citrate to release the encapsulated biomass, and the protein concentration of the encapsulated biomass was measured after the organisms were pelleted and lysed. The ratio of escaped to encapsulated biomass was calculated by dividing the escaped biomass by the total biomass (escaped plus encapsulated) with error propagated.
Substrate and hydrogen diffusivity.
The diffusivity of dissolved hydrogen and the dissolved organic carbon (DOC) in the synthetic wastewater and brewery wastewater were measured through alginate polymer sheets using the methods described by Shimotori and coworkers.51 Alginate polymer sheets both with and without PEI coating were analyzed. Briefly, after measuring the thickness of the polymer sheet with a Mitutoyo micrometer (Sakato, Japan) at multiple locations, the polymer sheet was placed in a diffusion cell apparatus (Fig. S3†), separating two compartments: a large 600 mL compartment and a small 60 mL compartment. To determine the diffusivity of hydrogen, the larger 600 mL compartment was filled with DI water saturated with hydrogen gas (Matheson, ultra-high purity) while the small 60 mL compartment was filled with 50 mL DI water with no hydrogen present, leaving a 10 mL headspace initially filled with air. Gas samples (200 μL) were taken from the headspace of the small compartment over time to analyze for hydrogen and were replaced with air to maintain the headspace pressure at 1 atm. To determine the diffusivity of DOC, synthetic wastewater or brewery wastewater was placed in the larger compartment, while synthetic wastewater made without carbon sources was placed in the small compartment. Aqueous samples were taken over time and analyzed for DOC, with replacement of the volume removed to maintain a headspace-free system.
Batch hydrogen production.
Hydrogen production from encapsulated biomass was monitored in batch using 100 mL serum bottles. Each bottle contained alginate beads, either PEI-coated (3 layers) or uncoated, made from 30 mL of alginate solution; 50 mL synthetic wastewater was also added to each bottle. Both beads encapsulating biomass and abiotic beads were studied. The headspace of the serum bottles was purged with nitrogen gas (Matheson, ultra-high purity), after which the bottles were sealed. The bottles were incubated at 37 °C while continuously stirred. The volume of gas produced and the hydrogen concentration in the gas were measured daily via water displacement and gas chromatography, respectively. Every day the beads were washed thoroughly with DI water and fresh sterilized synthetic wastewater was added. This was done to allow longer term monitoring and avoid inhibition from hydrogen build-up or substrate depletion.
Flow-through hydrogen production.
Four flow-through reactors were used to compare hydrogen production as a function of HRT in coated and uncoated alginate beads containing encapsulated biomass (Fig. S4†). The experiment was performed at room temperature (22 ± 2 °C). A reactor containing suspended hydrogen-producing organisms served as the control. A single peristaltic pump was used to add sterilized brewery wastewater continuously to the bottom of all reactors. The effluent was allowed to flow out of the top of each reactor, where it was fed into a bottle containing concentrated phosphoric acid (Sigma-Aldrich, ≥85%) to stop biological activity. This bottle also allowed the separation of gas and liquid, and the gas volume was measured via water displacement in an inverted graduated cylinder (Fig. S4†). All reactors were mixed via a magnetic stir bar (approximately 150 rpm). The pH of the reactor was measured daily and adjusted with powdered CaCO3 (Fisher Chemicals, 99.33%) to >5.0. The HRT ranged from a 6 h to 45 min, as described below. For two experiments, HRT was decreased step-wise after steady gas production had been observed for approximately 15 HRT. For a third experiment the HRT was held steady at 45 min. The hydrogen concentration in the gas produced was measured, as was the volatile fatty acid (VFA) concentration in the liquid effluent. Three experiments were performed. In the first experiment single reactors fed undiluted brewery wastewater were operated, containing: suspended biomass, uncoated Ca-alginate beads, uncoated Sr-alginate beads, coated (3 layers) Ca-alginate beads, and coated (3 layers) Sr-alginate beads. For this experiment the HRT started at 6 h and was decreased step-wise to 45 min. The second experiment consisted of triplicate reactors fed diluted brewery wastewater with the following treatments: suspended biomass, uncoated Ca-alginate beads, and coated (3 layers) Ca-alginate beads. In this experiment the HRT started at 3 h and was decreased step-wise to 45 min. The final experiment was performed at an HRT of 45 min and consisted of single reactors fed undiluted brewery wastewater, containing: uncoated Ca-alginate beads and Ca-alginate beads coated with 1 layer, 2 layers, or 3 layers of the composite coating.
Statistical analysis
For the biomass retention experiment, Shapiro–Wilk's tests indicated that the results were normally distributed (P-value > 0.05); therefore, Student's t-tests were used to compare different treatments. Biomass retention experiments with uncoated and coated Ca-alginate beads were conducted twice under the same conditions, hence meta-analysis was used to pool the data of these two experiments for statistical comparison.52 For the flow-through hydrogen production experiment comparing beads with different layers of coating, the results were normally distributed with equal variance. Therefore, Student's t-test was used. For the mass transport and the rest of hydrogen production experiments, the data were not normally distributed; therefore, Wilcoxon Mann–Whitney tests were conducted to compare treatments. Both Levene tests and Fligner–Killeen tests were conducted to confirm the equality of variances before the Wilcoxon Mann–Whitney tests. For multiple comparisons within experiments, p-values were corrected by the false discovery rate method (FDR) to reduce the type I error.53
Analytical methods
Gas volume and composition.
Gas production was measured volumetrically using water displacement.15 Gas composition was measured by gas chromatography coupled to a thermal conductivity detector (GC-TCD) (model 6890; Agilent Technologies). Separation was achieved with a packed column (Supelco molecular sieve 13 × 45/60, 10 ft × 1/8 in × 2.1 mm).15 Nitrogen was used as the carrier gas with a flow rate of 19.4 mL min−1. The oven temperature was constant at 50 °C. Gas samples (200 μL) were injected with a gas-tight syringe. The detection limits for methane and hydrogen were 0.004 ppm and 0.002 ppm, respectively.
Water quality parameters.
The TOC in the unfiltered samples and the DOC in samples filtered with 0.2 μm nylon filters (Pall) were measured using a total organic carbon (TOC) analyzer (Shimadzu TOC-L). Potassium hydrogen phthalate was used as the calibration standard. Samples (28 μL) were injected by autosampler. The detection limit of the TOC analyzer was 0.3 mg C L−1. The protein concentration was determined with the Pierce BCA protein assay (Thermo Scientific). COD was determined using Hach high range plus digestion vials. Ammonium nitrogen was measured with the Hach Test 'N Tube method. PO43− concentration was determined by ion chromatography (Dionex 120) according to USEPA Method 300.0.54
Results
Biomass retention
Table 1 shows the percent of encapsulated cells that escaped to the bulk solution over the 30 d experiment. When the alginate polymer gel matrix was cross-linked by Ca2+, cells were retained significantly better than when the matrix was cross-linked by Sr2+ (p = 0.035). There was no statistical difference in the biomass escape rate between Ca-alginate and Ba-alginate matrices (p = 0.070) and between Sr-alginate and Ba-alginate matrices (p = 0.231). The 3-layer composite coating reduced the average biomass escape rate in all three kinds of alginate beads, but this reduction was only statistically significant in the beads cross-linked with Sr2+ (p = 0.021), in which nearly all of the cells were retained. Most of the biomass escape occurred during the first 10 to 20 days, with little escape observed after this (Fig. S5†). This suggests that the biomass encapsulated in the outer layer of the beads migrated out in the beginning of the experiment, while the biomass present deeper in the beads remained encapsulated. The highest biomass escape rate of 33 ± 7% in the 30 d period was observed in the reactors containing uncoated Ba-alginate beads, which translated to an average daily escape rate of 1.3%. This cell loss rate is comparable to reported cell decay rates.55,56 Therefore, although the composite coating had the benefit of improving the biomass retention efficiency in general, all of the different alginate beads effectively retained the biomass, with cell leakage rates similar to or less than the expected cell decay rate. Additionally, although biomass was observed in the bulk solution of the abiotic controls, no biomass was observed in the abiotic beads, except for the coated Ca-alginate beads (Fig. S6†), suggesting that most of the alginate encapsulation matrices were effective in limiting intrusion of external biomass into the beads.
Table 1 Percentage of biomass that was released from alginate beads cross-linked by Ca2+, Sr2+, and Ba2+, with and without the 3-layer composite coating after 30 d. All treatments were set up in triplicate and the data from two separate experiments quantifying biomass escape from Ca-alginate were pooled with meta-analysis
Encapsulation matrix |
Percentage of escaped biomass |
Uncoated Ca-alginate |
11.8 ± 2.2% |
Coated Ca-alginate |
0.7 ± 2.1% |
Uncoated Ba-alginate |
33.2 ± 7.6% |
Coated Ba-alginate |
28.8 ± 12.5% |
Uncoated Sr-alginate |
27.0 ± 10.7% |
Coated Sr-alginate |
−2.2 ± 1.3% |
Diffusivity and permeability
Fig. 1 shows the diffusivity and permeability of hydrogen (Fig. 1A) and DOC (Fig. 1B and C for the synthetic and brewery wastewater, respectively) through the alginate polymers. The composite coating did not have a statistically significant effect on the diffusion coefficient of dissolved hydrogen through the polymers, which, although an order of magnitude lower than the diffusivity of hydrogen through water (4 × 10−6 cm2 s−1versus 4.5 × 10−5 cm2 s−1), was still rapid.57 The coating layers did affect the diffusion of DOC through alginate cross-linked by both Ca2+ and Sr+2, however, with the DOC in the brewery wastewater (Fig. 1C) behaving differently than the DOC in synthetic wastewater (Fig. 1B). These differences were thought to be a result of the brewery wastewater containing mainly polysaccharides and sugar from grains and some protein from yeast while the synthetic wastewater contained larger percentages of lipid and protein.46,58 This was not confirmed, however. With respect to the diffusion of the DOC in synthetic wastewater, the application of 1, 2, and 3 layers of coating all decreased the permeability (diffusion coefficient × partition coefficient, derived from the slope of the breakthrough curve) of the DOC significantly (corrected p = 0.007, 0.012, and 0.006 for 1, 2, and 3 layers of coating, respectively) but did not change its diffusivity. This suggests that the coating caused a decrease in the membrane–water partition coefficient of the synthetic wastewater DOC. In the brewery wastewater, both the permeability and diffusivity of the DOC was significantly higher than that of the synthetic wastewater (corrected p = 0.012 and 0.012, for the permeability and diffusivity, respectively), again, likely because of the predominance of sugars in the brewery wastewater, as opposed to the complex lipids and proteins in the synthetic wastewater. The coating also decreased the diffusivity of the brewery wastewater DOC (corrected p = 0.05 for both Ca-alginate and Sr-alginate, respectively), but had no effect on its permeability (corrected p = 0.15 and 0.20 for Ca-alginate and Sr-alginate, respectively). This suggests that the coating increased the membrane–water partition coefficient of the brewery wastewater DOC. As a result of the different transport behaviors observed between the coated and uncoated polymer matrices, the application of composite coating around the encapsulated cells may make it possible to tune the transport of substrate through the encapsulation matrix, thereby tuning the hydrogen production rate as well.
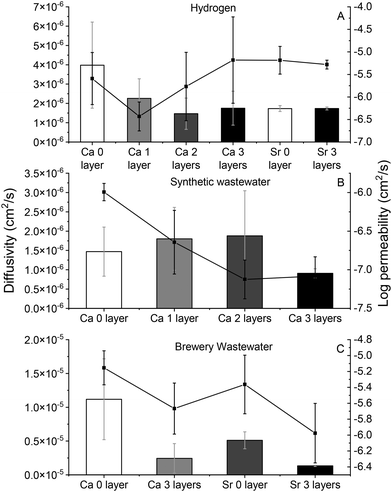 |
| Fig. 1 The diffusivity (bar) and permeability (line) of dissolved hydrogen (A), DOC in synthetic wastewater (B), and DOC in brewery wastewater (C) through alginate gel polymer sheets cross-linked by Ca2+ and Sr2+. Polymer sheets without coating or with coatings of 1, 2, or 3 layers of PEI are shown. Each experiment was performed a minimum of three times. The error bars represent the standard deviation. | |
Hydrogen production
Fig. 2 shows the average daily quantity of hydrogen produced in batch reactors. The hydrogen production and bulk biomass concentration over time are shown in Fig. S7.† The reactors containing encapsulated biomass in uncoated Ca-alginate beads produced more hydrogen than those containing biomass encapsulated in Ca-alginate beads coated with 3 layers of the composite coating (p = 2.24 × 10−16). This was likely a result of the faster DOC transport through the uncoated Ca-alginate (Fig. 1), which allowed rapid DOC consumption and production of hydrogen.
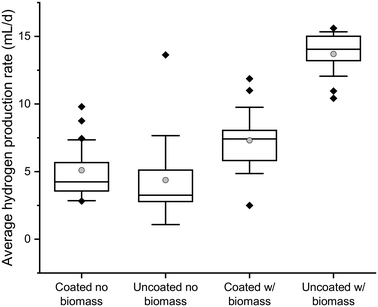 |
| Fig. 2 The average hydrogen production rate in batch reactors fed synthetic wastewater and containing biomass encapsulated in coated (3-layer) and uncoated Ca-alginate beads and coated and uncoated abiotic Ca-alginate beads. Boxes represent the 25–75 percentiles of the data. The error bars represent the standard deviation. The means are shown as grey circles, outliers as diamonds, and lines in boxes represent the median. All reactors were operated in triplicate. | |
Hydrogen was produced in the control reactors containing no encapsulated biomass (as a result of suspended biomass (Fig. S7†)), though it was statistically less than that produced in reactors with encapsulated biomass (p = 1.23 × 10−5 and 1.46 × 10−15 for the coated and uncoated beads, respectively). Interestingly, the reactors with encapsulated, but uncoated, biomass also contained much less suspended biomass (Fig. S7†), presumably because the rapid substrate transport and utilization by encapsulated biomass resulted in either a lack of bulk substrate or more rapid accumulation of acetic acid, either of which would limit bulk growth of hydrogen-producing organisms.
At the end of the experiment, the rapid substrate transport and degradation in the uncoated Ca-alginate beads was confirmed by operating the batch reactors for another 24 h (for a total of 48 h) without replacing the synthetic wastewater. The hydrogen production, as well as the bulk solution biomass concentration, increased in all the reactors except those containing biomass encapsulated in uncoated Ca-alginate. The lack of bulk solution growth in the uncoated Ca-alginate reactors over this period confirms that rapid substrate transport and consumption by encapsulated biomass had already occurred (Fig. S7B†). These results show that the encapsulation system provided a high biomass concentration that, when coupled with rapid substrate transport to the biomass, enabled a higher hydrogen production rate to be maintained.
Fig. 3 shows that, when operated under continuous flow conditions, reactors containing biomass encapsulated in uncoated beads consistently produced large amounts of hydrogen from brewery wastewater, regardless of the HRT. In Fig. 3A, when operating at a 6 h HRT, all the reactors containing encapsulated biomass produced more hydrogen than the control reactor containing suspended biomass (p = 0.011, 0.008 and 0.008 for uncoated Ca-alginate, coated Ca-alginate, and uncoated Sr-alginate), with the exception of the reactor with coated Sr-alginate beads (corrected p = 0.39). Although the 3-layer coating did not affect the hydrogen production from Ca-alginate reactors (corrected p = 0.58), it decreased the hydrogen production from Sr-alginate reactors (corrected p = 0.008). When the HRT was decreased to 3 h and again to 1.5 h, the hydrogen production rate from the reactors with uncoated beads started to surpass that of reactors containing coated beads. At an HRT of 45 min, washout of suspended biomass occurred, while the reactors containing uncoated beads continued producing hydrogen at a high-rate (∼240 mL per day; Fig. 3A). At this low HRT, the reactor with coated Ca-alginate beads produced less hydrogen than its uncoated counterpart (corrected p = 0.005), but still more than the reactor containing coated Sr-alginate beads (corrected p = 0.005). During this experiment, undiluted brewery wastewater (14 g L−1 COD) was fed to the reactors. Other than at the 6 h HRT, the reactors containing biomass encapsulated in uncoated alginate consistently produced more hydrogen than biomass encapsulated in PEI-coated alginate (Fig. 3A). This was likely a result of the diffusion barrier created by the PEI coating to the transport of the DOC in the brewery wastewater to the encapsulated biomass (Fig. 1). Biomass encapsulated in the uncoated alginate beads also produced more hydrogen than the suspended biomass at these high substrate concentrations, with 200–300 mL more hydrogen produced per day, regardless of the HRT (Fig. 3A).
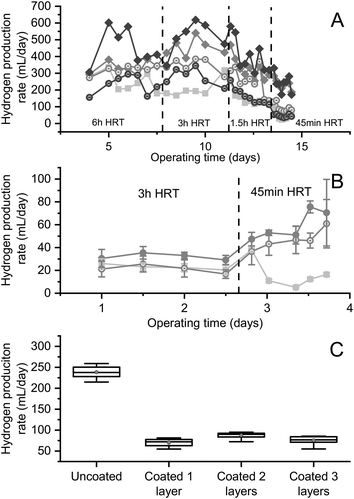 |
| Fig. 3 The daily hydrogen production rate from flow-through continuous stirred-tank reactors. Panels (A) and (C) show results from reactors fed with brewery wastewater (single reactors) and panel (B) shows results from reactors fed diluted brewery wastewater (triplicate reactors). Panels (A) and (B), contain biomass encapsulated in uncoated Ca-alginate beads (grey diamonds) and Sr-alginate beads (black diamonds), in addition to those encapsulated and then coated with 3 layers of the composite coating (circles). Reactors containing suspended biomass (boxes) served as controls. Panel (C) shows results from uncoated Ca-alginate beads and beads coated with 1, 2, and 3 layers of the composite coating operating at 45 min HRT; boxes represent the 25–75 percentiles of the data, the mean values are shown as grey circles, and the lines in the boxes represent the median value. Error bars in all of the panels represent the standard deviation of the data. | |
When diluted brewery wastewater (4 g L−1 COD) was fed to the encapsulated and suspended biomass (Fig. 3B), the effect of coating on the hydrogen production rate was still significant (corrected p = 0.0005 and 0.014 for 3 h and 45 min HRT, respectively) but much less apparent. This was likely because the coated beads were no longer diffusion limited as a result of the lower substrate consumption rate. This was also supported by the observed increase in hydrogen production rate from the encapsulated biomass at an HRT of 45 min, indicating that the encapsulated biomass was growth-, rather than diffusion-limited at this lower substrate concentration (Fig. 3B).
Fig. 3C shows, again, that the reactor fed undiluted brewery wastewater and containing uncoated beads operating at 45 min HRT produced significantly greater quantities of hydrogen than any of the reactors containing coated beads (p = 3.30 × 10−12, 5.60 × 10−11, and 2.66 × 10−12 for reactors with 1, 2, and 3 layers of coated beads, respectively). Moreover, no statistical difference existed in the hydrogen production rate of reactors containing beads with 1 layer of coating versus 3 layers of coating (p = 0.23). The reactor containing beads coated with 2 layers of composite coating did produce statistically more hydrogen than the reactors containing beads coated with 1 or 3 layers of composite (p = 0.0023 and 0.0073); nevertheless, this increase in hydrogen production was small (Fig. 3C) and is likely an experimental artifact.
These results clearly showed that encapsulated biomass achieved high and stable hydrogen production from real (brewery) wastewater. Results indicated that at high substrate concentrations (14 g L−1 COD) the encapsulated biomass was diffusion-limited, while at low substrate concentrations (4 g L−1 COD), they were growth-limited. The diffusion barrier created by the coating significantly reduced the hydrogen production rate under diffusion-limited conditions (i.e., high substrate concentrations) but had less of an effect under growth-limited conditions.
Discussion
The alginate encapsulation matrices effectively retained hydrogen-producing biomass and allowed sufficiently fast diffusion of substrate, enabling reliable high-rate hydrogen production from brewery wastewater, even at a low HRT that resulted in washout of suspended biomass. Moreover, the fact that little bulk solution biomass intruded into the encapsulation matrices suggested that the population of the desired phenotypes can potentially be maintained for long-term generation and recovery of high-value intermediates. Further study, however, is needed to investigate the stability of the encapsulated microbial community over time and the stability of operation without regular Ca2+ addition. Although different divalent cross-linking ions resulted in different biomass retention abilities, the cross-linking ion used did not affect the resulting hydrogen production rate. Both Ca-alginate and Sr-alginate exhibited similar diffusion performance. Ba-alginate exhibited satisfactory biomass retention ability but was not further investigated because there is a risk of releasing toxic Ba2+ over time when using this ion as a cross-linking agent. The composite coating consistently improved biomass retention but also influenced the mass transport rate of the substrates, with different substrates influenced differently. Overall, the coating created a diffusion barrier that lowered the hydrogen production rate for encapsulated biomass. The diffusion barrier was insignificant when the system was substrate-limited but appeared to become rate-limiting when sufficient substrate was provided and the system became diffusion-limited; this diffusion barrier also appeared to be relatively insensitive to the number of coating layers applied. These end points could be used to develop a customizable encapsulation system allowing adaption to various operating conditions.
Compared to other state-of-the art technologies that retain biomass, such as membrane bioreactors, the encapsulation system described herein also effectively retains biomass and therefore should be able to achieve high-rate operation and a small reactor footprint.59 In fact, similar hydrogen production rates were produced in the system described here at room temperature when compared to other (non-encapsulated) systems operated at 37 °C.9,10,60 The use of encapsulated biomass, however, has additional advantages. Different specific microbial consortia can be encapsulated in alginate beads.61 Encapsulated biomass has also been reported to survive months of storage, making long-term storage and long distance transport of encapsulated biomass possible.62 Reactors using encapsulated biomass should be able to easily resume operation after the growth of undesired phenotypes in the bulk solution, simply by rinsing the encapsulated beads and beginning operation again. In addition, the system should not suffer from fouling, resulting in lower energy costs and consistent flows.63–65 Finally, it has been reported that alginate polymers chelate free heavy metal ions.29 It is currently unknown whether this would protect encapsulated biomass from heavy metal inhibition, but if so, this could be another advantage, depending on the operating conditions.
There are, however, also limitations of using encapsulated biomass. Culturing the desired biomass is required, as is encapsulation, both of which require time and cost. The encapsulation material itself also limits the application; alginate crosslinked by Ca2+ and Sr2+ is still susceptible to exchange with monovalent cations such as NH4+ and Na+, and chelators such as PO43−. Alginate cross-linked with heavy metals has been reported to be stable, but could leach toxic heavy metal ions over time.66 Finally, the customizability of this system suggests complexity in designing it. A model that describes the activity of encapsulated biomass needs to be developed to enable prediction of performance and optimization of system configuration.
The application of encapsulated specialized phenotypes to achieve reliable high-rate resource recovery is not limited to hydrogen and could be expanded to include other high-value intermediates easily consumed during wastewater treatment. For example, researchers have successfully enriched chain elongating consortia and used them to recover MCFAs such as hexanoic acid and octanoic acid.17,67,68 Encapsulated chain-elongating bacteria could enable more predictable and robust operation of such a system through physically isolating desired phenotypes from undesired ones. Finally, the simplicity of operation, once started, could make decentralized wastewater treatment using encapsulated biomass a viable option for industries, such as food processing industries and remote communities.
Conflicts of interest
The authors declare no conflict of interest.
Acknowledgements
The authors acknowledge the funding provided by the University of Minnesota and the Minnesota Department of Commerce (Project COMM-RESEARCH01-20160104). We would like to thank Julian Preciado and Alptekin Aksan for their insights into the encapsulation of bacteria, Abby Kargol and Akia Vang for their help with laboratory work, and Fulton Beer for the use of their wastewater.
References
- D. J. Batstone, J. Keller, I. Angelidaki, S. V. Kalyuzhnyi, S. G. Pavlostathis, A. Rozzi, W. T. Sanders, H. Siegrist and V. A. Vavilin, The IWA Anaerobic Digestion Model No 1 (ADM1), Water Sci. Technol., 2002, 45, 65–73 CrossRef PubMed.
- D. Das and T. N. Vezirolu, Hydrogen production by biological processes: a survey of literature, Int. J. Hydrogen Energy, 2001, 26, 13–28 CrossRef.
- M. M. Alves, M. A. Pereira, D. Z. Sousa, A. J. Cavaleiro, M. Picavet, H. Smidt and A. J. M. Stams, Waste lipids to energy: How to optimize methane production from long-chain fatty acids (LCFA), Microb. Biotechnol., 2009, 2, 538–550 CrossRef PubMed.
- T. N. Vezirolu and F. Barbir, Hydrogen: the wonder fuel, Int. J. Hydrogen Energy, 1992, 17, 391–404 CrossRef.
- J. J. Lay, Biohydrogen generation by mesophilic anaerobic fermentation of microcrystalline cellulose, Biotechnol. Bioeng., 2001, 74, 280–287 CrossRef PubMed.
- B. E. Logan, S. E. Oh, I. S. Kim and S. Van Ginkel, Biological hydrogen production measured in batch anaerobic respirometers, Environ. Sci. Technol., 2002, 36, 2530–2535 CrossRef PubMed.
- P. Intanoo, P. Chaimongkol and S. Chavadej, Hydrogen and methane production from cassava wastewater using two-stage upflow anaerobic sludge blanket reactors (UASB) with an emphasis on maximum hydrogen production, Int. J. Hydrogen Energy, 2016, 41, 6107–6114 CrossRef.
- S.-E. Oh, S. Van Ginkel and B. E. Logan, The relative effectiveness of ph control and heat treatment for enhancing biohydrogen gas production, Environ. Sci. Technol., 2003, 37, 5186–5190 CrossRef PubMed.
- H. Yu, Z. Zhu, W. Hu and H. Zhang, Hydrogen production from rice winery wastewater in an upflow anaerobic reactor by using mixed anaerobic cultures, Int. J. Hydrogen Energy, 2002, 27, 1359–1365 CrossRef.
- I. Hussy, F. R. Hawkes, R. Dinsdale and D. L. Hawkes, Continuous fermentative hydrogen production from a wheat starch co-product by mixed microflora, Biotechnol. Bioeng., 2003, 84, 619–626 CrossRef PubMed.
- D. Y. Lee, Y. Y. Li and T. Noike, Continuous H2 production by anaerobic mixed microflora in membrane bioreactor, Bioresour. Technol., 2009, 100, 690–695 CrossRef PubMed.
- G. Luo, L. Xie, Q. Zhou and I. Angelidaki, Enhancement of bioenergy production from organic wastes by two-stage anaerobic hydrogen and methane production process, Bioresour. Technol., 2011, 102, 8700–8706 CrossRef PubMed.
- V. Perna, E. Castelló, J. Wenzel, C. Zampol, D. M. Fontes Lima, L. Borzacconi, M. B. Varesche, M. Zaiat and C. Etchebehere, Hydrogen production in an upflow anaerobic packed bed reactor used to treat cheese whey, Int. J. Hydrogen Energy, 2013, 38, 54–62 CrossRef.
- J. Zhong, D. K. Stevens and C. L. Hansen, Optimization of anaerobic hydrogen and methane production from dairy processing waste using a two-stage digestion in induced bed reactors (IBR), Int. J. Hydrogen Energy, 2015, 40, 15470–15476 CrossRef.
- A. L. Prieto, L. H. Sigtermans, B. R. Mutlu, A. Aksan, W. A. Arnold and P. J. Novak, Performance of a composite bioactive membrane for H 2 production and capture from high strength wastewater, Environ. Sci.: Water Res. Technol., 2016, 2, 848–857 RSC.
- J. Lay, Y. Lee and T. Noike, Feasibility of biological hydrogen production from organic fraction of municipal solid, Water Res., 1999, 33, 2579–2586 CrossRef.
- K. J. J. Steinbusch, H. V. M. Hamelers, C. M. Plugge and C. J. N. Buisman, Biological formation of caproate and caprylate from acetate: fuel and chemical production from low grade biomass, Energy Environ. Sci., 2011, 4, 216–224 RSC.
- T. I. M. Grootscholten, K. J. J. Steinbusch, H. V. M. Hamelers and C. J. N. Buisman, Chain elongation of acetate and ethanol in an upflow anaerobic filter for high rate
MCFA production, Bioresour. Technol., 2013, 135, 440–445 CrossRef PubMed.
- H. N. Gavala, I. V. Skiadas and B. K. Ahring, Biological hydrogen production in suspended and attached growth anaerobic reactor systems, Int. J. Hydrogen Energy, 2006, 31, 1164–1175 CrossRef.
- K. S. Lee, J. F. Wu, Y. S. Lo, Y. C. Lo, P. J. Lin and J. S. Chang, Anaerobic hydrogen production with an efficient carrier-induced granular sludge bed bioreactor, Biotechnol. Bioeng., 2004, 87, 648–657 CrossRef PubMed.
- S.-E. Oh, P. Iyer, M. A. Bruns and B. E. Logan, Biological hydrogen production using a membrane bioreactor, Biotechnol. Bioeng., 2004, 87, 119–127 CrossRef PubMed.
- K. Calderón, B. Rodelas, N. Cabirol, J. González-López and A. Noyola, Analysis of microbial communities developed on the fouling layers of a membrane-coupled anaerobic bioreactor applied to wastewater treatment, Bioresour. Technol., 2011, 102, 4618–4627 CrossRef PubMed.
- L. Raskin, R. I. Amann, L. K. Poulsen, B. E. Rittmann and D. A. Stahl, Use of ribosomal RNA-based molecular probes for characterization of complex microbial communities in anaerobic biofilms, Water Sci. Technol., 1995, 31, 261–272 CrossRef.
- R. G. Duff, Microencapsulation Technology: A Novel Method for Monoclonal Antibody Production, Trends Biotechnol., 1985, 3, 167–170 CrossRef.
- P. S. J. Cheetham, C. Garrett and J. Clark, Isomaltulose production using immobilized cells, Biotechnol. Bioeng., 1985, 27, 471–481 CrossRef PubMed.
- I. Nilsson, S. Ohlson, L. Haggstrom, N. Molin and K. Mosbach, Columnar denitrification of water by immobilized Pseudomonas denitrificans cells, Eur. J. Appl. Microbiol. Biotechnol., 1980, 10, 261–274 CrossRef.
- A. Haug, B. Larsen and O. Smidsrod, Study of the constitution of alginic acid by partial acid hydrolysis, Acta Chem. Scand., 1966, 20, 183–190 CrossRef.
- O. Smidsrod and G. Skjåk-Bræk, Alginate as immobilization matrix for cells, Trends Biotechnol., 1990, 8, 71–78 CrossRef PubMed.
-
I. Fry and R. Mehlhorn, Polyurethane and Alginate Immobilized Algal Biomass for the Removal of Aqueous Toxic Metals, International symposium in situ and on-site bioreclamation, San Diego, CA, 1992, https://inis.iaea.org/search/search.aspx?orig_q=RN:24059077 Search PubMed.
- B. T. Stokke, K. I. Draget, O. Smidsrød, Y. Yuguchi, H. Urakawa and K. Kajiwara, Small-angle X-ray scattering and rheological characterization of alginate gels. 1. Ca-alginate gels, Macromolecules, 2000, 33, 1853–1863 CrossRef.
- H. J. Kong and D. J. Mooney, On reinforcement of alginate hydrogels, Cell Transplant., 2003, 12, 779–785 Search PubMed.
- P. Sikorski, F. Mo, G. Skjåk-Bræk and B. T. Stokke, Evidence for egg-box-compatible interactions in calcium - Alginate gels from fiber x-ray diffraction, Biomacromolecules, 2007, 8, 2098–2103 CrossRef PubMed.
- A. M. D. Sergio and T. Y. Bustos, Biodegradation of wastewater pollutants by activated sludge encapsulated inside calcium-alginate beads in a tubular packed bed reactor, Biodegradation, 2009, 20, 709–715 CrossRef PubMed.
- A. Haug, J. Bjerrum, O. Buchardt, G. E. Olsen, C. Pedersen and J. Toft, The affinity of some divalent metals for different types of alginates, Acta Chem. Scand., 1961, 15, 1794–1795 CrossRef.
- O. Smidsrød, Molecular basis for some physical properties of alginates in the gel state, Faraday Discuss. Chem. Soc., 1974, 57, 263–274 RSC.
- A. L. Dainty, K. H. Goulding, P. K. Robinson, I. Simpkins and M. D. Trevan, Stability of alginate-immobilized algal cells, Biotechnol. Bioeng., 1986, 28, 210–216 CrossRef PubMed.
- A. Martinsen, G. Skjåk-Braek and O. Smidsrød, Alginate as immobilization material: I. Correlation between chemical and physical properties of alginate gel beads, Biotechnol. Bioeng., 1989, 33, 79–89 CrossRef PubMed.
- S. K. Bajpai and S. Sharma, Investigation of swelling/degradation behaviour of alginate beads crosslinked with Ca2+and Ba2+ions, React. Funct. Polym., 2004, 59, 129–140 CrossRef.
- Ý. A. Mørch, I. Donati, B. L. Strand and G. Skjåk-Bræk, Effect of Ca 2+, Ba 2+, and Sr 2+ on Alginate Microbeads, Biomacromolecules, 2006, 7, 1471–1480 CrossRef PubMed.
- S. M. Moreira, M. Moreira-Santos, L. Guilhermino and R. Ribeiro, Immobilization of the marine microalga Phaeodactylum tricornutum in alginate for in situ experiments: Bead stability and suitability, Enzyme Microb. Technol., 2006, 38, 135–141 CrossRef.
- S. Birnbaum, R. Pendleton, P. O. Larsson and K. Mosbach, Covalent stabilization of alginate gel for the entrapment of living whole cells, Biotechnol. Lett., 1981, 3, 393–400 CrossRef.
- S. Mohamed and A. B. Salleh, Physical properties of polyethyleneimine-alginate gels, Biotechnol. Lett., 1982, 4, 611–614 CrossRef.
- J. J. Joung, C. Akin and G. P. Royer, Immobilization of growing cells by polyethyleneimine-modified alginate, Appl. Biochem. Biotechnol., 1987, 14, 259–275 CrossRef.
- Y. Yan, Q. An, Z. Xiao, W. Zheng and S. Zhai, Flexible core-shell/bead-like alginate@PEI with exceptional adsorption capacity, recycling performance toward batch and column sorption of Cr(VI), Chem. Eng. J., 2017, 313, 475–486 CrossRef.
- H. Lee, D. Hong, J. Y. Choi, J. Y. Kim, S. H. Lee, H. M. Kim, S. H. Yang and I. S. Choi, Layer-by-layer-based silica encapsulation of individual yeast with thickness control, Chem. - Asian J., 2015, 10, 129–132 CrossRef PubMed.
- C. G. Klatt and T. M. LaPara, Aerobic biological treatment of synthetic municipal wastewater in membrane-coupled bioreactors, Biotechnol. Bioeng., 2003, 82, 313–320 CrossRef PubMed.
- T. Noike, H. Takabatake, O. Mizuno and M. Ohba, Inhibition of hydrogen fermentation of organic wastes by lactic acid bacteria, Int. J. Hydrogen Energy, 2002, 27, 1367–1371 CrossRef.
- J. Klein, J. Stock and K. D. Vorlop, Pore size and properties of spherical Ca-alginate biocatalysts, Eur. J. Appl. Microbiol. Biotechnol., 1983, 18, 86–91 CrossRef.
- H. Tanaka, M. Matsumura and I. A. Veliky, Diffusion characteristics of substrates in Ca-alginate gel beads, Biotechnol. Bioeng., 1984, 26, 53–58 CrossRef PubMed.
- P. K. Smith, R. I. Krohn, G. T. Hermanson, A. K. Mallia, F. H. Gartner, M. D. Provenzano, E. K. Fujimoto, N. M. Goeke, B. J. Olson and D. C. Klenk, Measurement of protein using bicinchoninic acid, Anal. Biochem., 1985, 150, 76–85 CrossRef PubMed.
- T. Shimotori, E. L. Cussler and W. A. Arnold, High-Density Polyethylene Membrane Containing Fe0 as a Contaminant Barrier, Environ. Sci. Technol., 2006, 38, 803–810 Search PubMed.
- S. B. Thacker, Meta-analysis A Quantitative Approach to Research Integration, JAMA, J. Am. Med. Assoc., 1993, 39, 766–772 Search PubMed.
- Y. Benjamini and Y. Hochberg, Controlling the false discovery rate: a practical and powerful approach to multiple testing, J. R. Stat. Soc. Series B Stat. Methodol., 1995, 57, 289–300 Search PubMed.
-
J. D. Pfaff, Method 300.0 Determination of Inorganic Anions By Ion Chromatography, U.S. Environmental Protection Agency, 1993, p. 28.
- W. Gujer and A. J. B. Zehnder, Conversion Processes in Anaerobic Digestion, Water Sci. Technol., 1983, 15, 127–167 CrossRef.
- J. T. Novak and D. A. Carlson, The Kinetics of Anaerobic Long Chain Fatty Acid Degradation, J. - Water Pollut. Control Fed., 1970, 42, 1932–1943 Search PubMed.
- R. T. Ferrell and D. M. Himmelblau, Diffusion coefficients of hydrogen and helium in water, AIChE J., 1967, 13, 702–708 CrossRef.
- G. S. Simate, J. Cluett, S. E. Iyuke, E. T. Musapatika, S. Ndlovu, L. F. Walubita and A. E. Alvarez, The treatment of brewery wastewater for reuse: State of the art, Desalination, 2011, 273, 235–247 CrossRef.
- K. S. Lee, P. J. Lin, K. Fangchiang and J. S. Chang, Continuous hydrogen production by anaerobic mixed microflora using a hollow-fiber microfiltration membrane bioreactor, Int. J. Hydrogen Energy, 2007, 32, 950–957 CrossRef.
- S. K. Han and H. S. Shin, Performance of an innovative two-stage process converting food waste to hydrogen and methane?, J. Air Waste Manage. Assoc., 2004, 54, 242–249 CrossRef.
- T. H. Hsia, Y. J. Feng, C. M. Ho, W. P. Chou and S. K. Tseng, PVA-alginate immobilized cells for anaerobic ammonium oxidation (anammox) process, J. Ind. Microbiol. Biotechnol., 2008, 35, 721–727 CrossRef PubMed.
- K. Melzoch, M. Rychtera and V. Hábová, Effect of immobilization upon the properties and behaviour of Saccharomyces cerevisiae cells, J. Biotechnol., 1994, 32, 59–65 CrossRef.
- I.-S. Chang, P. L. Clech, B. Jefferson and S. Judd, Membrane fouling in membrane bioreactors for wastewater treatment, J. Environ. Eng., 2002, 128, 1018–1029 CrossRef.
- F. Meng, S. R. Chae, A. Drews, M. Kraume, H. S. Shin and F. Yang, Recent advances in membrane bioreactors (MBRs): Membrane fouling and membrane material, Water Res., 2009, 43, 1489–1512 CrossRef PubMed.
- Z. Huang, S. L. Ong and H. Y. Ng, Submerged anaerobic membrane bioreactor for low-strength wastewater treatment: Effect of HRT and SRT on treatment performance and membrane fouling, Water Res., 2011, 45, 705–713 CrossRef PubMed.
- G. R. Seely and R. L. Hart, The Binding of Alkaline Earth Metal Ions to Alginate, Macromolecules, 1974, 7, 706–710 CrossRef PubMed.
- M. T. Agler, C. M. Spirito, J. G. Usack, J. J. Werner and L. T. Angenent, Chain elongation with reactor microbiomes: upgrading dilute ethanol to medium-chain carboxylates, Energy Environ. Sci., 2012, 5, 8189 RSC.
- M. T. Agler, C. M. Spirito, J. G. Usack, J. J. Werner and L. T. Angenent, Development of a highly specific and productive process for n-caproic acid production: Applying lessons from methanogenic microbiomes, Water Sci. Technol., 2014, 69, 62–68 CrossRef PubMed.
Footnote |
† Electronic supplementary information (ESI) available. See DOI: 10.1039/c8ew00351c |
|
This journal is © The Royal Society of Chemistry 2018 |
Click here to see how this site uses Cookies. View our privacy policy here.