DOI:
10.1039/C6AN00773B
(Critical Review)
Analyst, 2016,
141, 3126-3140
Functionalization of metal nanoclusters for biomedical applications
Received
2nd April 2016
, Accepted 20th April 2016
First published on 21st April 2016
Abstract
Metal nanoclusters (NCs) are emerging as a new class of functional nanomaterials in the area of biological sensing, labelling, imaging and therapy due to their unique physical and chemical properties, such as ultrasmall size, HOMO–LUMO transition, strong luminescence together with good photostability and biocompatibility. A recent surge of interest in this field is the surface functionalization of these metal NCs through which one can tailor their physicochemical properties, such as stability in solution, and strong luminescence, as well as their biodistribution and toxicity in biological systems, which in turn can empower these functionalized NCs with desirable targeting, imaging, and therapeutic ability for biomedical applications. In this review, we first introduce the functionalization strategies for the metal NCs developed in the past few years, followed by highlighting some biomedical applications of these functionalized metal NCs. We then discuss the difference of in vitro and in vivo fate as well as toxicity between various functionalized metal NCs. Finally, we present a short discussion on the current challenges and provide an outlook of the future developments of these functional metal NCs.
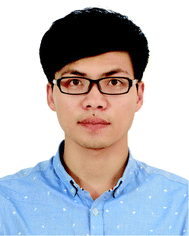 Xiao-Rong Song | Xiao-Rong Song received his BS (2012) from the College of Chemistry and Chemical Engineering at Fuzhou University (China). He continued to undertake his PhD study majoring in Analytical Chemistry in the Department of Chemistry at Fuzhou University under the supervision of Prof. Huang-Hao Yang, and currently he is an exchange student studying at the Department of Chemical & Biomolecular Engineering, National University of Singapore, under the supervision of Prof. Jianping Xie. His research focuses on multifunctional nanomaterials, biosensors and nanomedicine. |
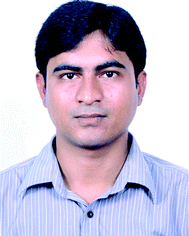 Nirmal Goswami | Nirmal Goswami is currently a postdoctoral fellow in Prof. Jianping Xie's group at the Department of Chemical and Biomolecular Engineering, National University of Singapore. He obtained his PhD (2014) in Chemistry from the S. N. Bose National Centre for Basic Sciences, India. He is interested in noble metal nanoclusters and their biomedical applications. |
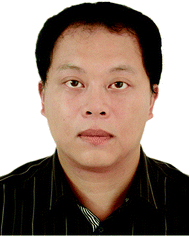 Huang-Hao Yang | Huang-Hao Yang received his PhD from Xiamen University in 2002 and performed postdoctoral research in The Hong Kong University of Science and Technology from 2002 to 2004. He visited the University of Florida from 2007 to 2008 as a guest professor, and then joined Fuzhou University in 2008. His research interests mostly focus on biosensors, molecular diagnosis, nanomedicine, and cancer therapy. He has authored/co-authored over 150 peer-reviewed papers and total citations exceed 6300 (H-Index of 40). He was selected as a Changjiang Scholar Professor by Education Ministry of China in 2013 and became a fellow of the RSC in 2014. |
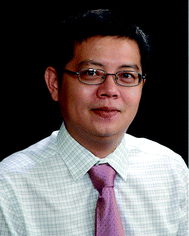 Jianping Xie | Jianping Xie received his BS and MS degree from the Department of Chemical Engineering in Tsinghua University (China). He graduated with a PhD from the Singapore–MIT Alliance program. He joined the National University of Singapore as an Assistant Professor in 2010 and established the “Noble Metal Nanoclusters” research group. His major research interest is engineering ultrasmall metal nanoclusters for biomedical and environmental applications (http://cheed.nus.edu.sg/stf/chexiej/index.html). |
1. Introduction
Metal nanoclusters (NCs), consisting of several to tens of atoms with a core size below 2 nm, have recently emerged as a new class of functional materials. Due to their ultrasmall size approaching the Fermi-wavelength of electrons, metal NCs exhibit discrete energy levels and a multitude of molecular-like properties,1,2 such as HOMO–LUMO transition, tunable luminescence, large Stokes shift, quantized charging, molecular chirality and magnetism.3,4 Recently, various kinds of metal NCs including gold (Au), silver (Ag) and copper (Cu) NCs have been widely exploited for applications in the area of biological sensing, labelling, imaging and therapy.5,6 These metal NCs can produce multitudinous measurable signals, such as luminescence and chemiluminescence, and have some distinctive features such as ultrafine size with narrow size distribution, and good photo-stability and biocompatibility. Because of all these unique attributes, metal NC based platforms have shown some superior performances over nanoparticles (NPs) in biomedical applications.7 For example, the use of large NPs may cause severe side effects in the body particularly in the liver and spleen when they are used in the in vivo set-up, since these NPs might not be able to escape from the kidney barrier. In contrast, the ultrafine size of metal NCs allows them to be efficiently cleared from the body without causing severe side effects.
In biomedical research, it is highly desired to develop reliable, sensitive, and biocompatible platforms. Sometimes, the materials need to maintain their intrinsic properties, such as stability, imaging, and therapeutic ability as well as targeting ability while facing the challenges of a complex matrix, cellular media or an in vivo environment, which are known to be consisting of a large number of proteins, thiols, amino acids, cells and other species or having a strong ionic strength. Therefore, it is extremely important to firmly control the surface chemistry of these materials since they play a major role in controlling many of the physicochemical properties of functional nanomaterials. Similar to other nanomaterials, the physicochemical properties of metal NCs could also be significantly influenced by the alteration of their surface chemistry.1 For example, the luminescence properties of Au NCs would not only be affected by the nature of the surface ligands, but also be susceptible to the environmental pH and temperature.8,9 The stability of these metal NCs could be further improved if the ligand shells are properly engineered on their surface.10–12 In fact, the ensuing research revealed the importance of functionalization of metal NCs to empower them with new biomedical functions as well as to finally improve their performance in applications including sensing, imaging, and therapeutics.7,13,14
While the synthesis, properties and biomedical applications of NCs have been published in some recent reviews, a concrete review related to their surface functionalization has not been summarized to date.5,13–15 Therefore, it would be of great importance to shed light on how to functionalize these metal NCs to fulfil the desired biomedical applications. The understanding of their functionalization properties can not only provide impactful insight into the surface properties of these metal NCs with respect to their interaction in the cellular medium but also can offer the basis to further design smart multifunctional metal NCs for practical applications. In line with this effort, this review will first focus on discussing the strategies related to the functionalization of metal NCs, followed by highlighting some biomedical applications of these functionalized NCs. Thereafter, we will summarize recent studies about the biodistribution and toxicity of metal NCs and then discuss the difference of the in vivo fate between various functionalized NCs. Finally, we will present a short discussion on the current challenges and give the outlook of the future developments of these functional metal NCs.
2. Functionalization of metal NCs
Benefiting from the contributions toward the developments of surface functionalization strategies, a number of methods have been established to either customize the metal NCs with necessary functional groups such as biomolecules and polymers, or to immobilize these metal NCs with a variety of inorganic nanostructures to further improve their performance in the biological set-up. In general, the surface functionalization of these metal NCs can be accomplished through two different ways: in situ functionalization during the synthesis and post-synthesis functionalization (after the synthesis). In the following, we will discuss these two strategies by highlighting some examples.
2.1
In situ functionalization during synthesis
2.1.1 Thiols as protecting ligands.
The use of thiols as surface protecting ligands can be traced back to the last century during the study of self-assembled monolayers on gold surfaces.16 It is now generally accepted that the strength of a thiolate–gold bond is close to that of a gold–gold bond, which means that thiolate ligands can modify the gold–gold bonding and result in the formation of a gold–sulfur interface.17 The robust covalent bond between gold and thiols first followed the exploitation of thiols as protecting ligands for the synthesis and functionalization of metal NPs and later made an impact on the pace of subsequent development of the thiolate-protected metal NCs (or thiolated NCs for short). In their early work, Whetten, Murray and other groups reported the successful synthesis and characterization of a number of thiolated Au NCs.18–20 Since then thiol-containing ligands remain as one of the most commonly used ligands for the synthesis, stabilization as well as the functionalization of metal NCs.8 The ease of availability as well as the rich surface chemistry of many thiol-containing compounds, including thiol-containing organosulfur compounds (e.g., mercaptobenzoic acid (MBA) and lipoic acid (LA)), thiol-containing peptides (e.g., tri-peptide, glutathione (GSH)), thiol-containing polymers (e.g., thiol terminated poly(ethylene glycol) (SH-PEG)), and thiol-containing amino acids (e.g., cysteine) have allowed researchers to use them as functional ligands for metal NCs with a potential for their use in many biomedical applications.
The strong bond between thiols and metal atoms as well as their facile formation has stimulated the accumulated achievements on the functionalization and applications of thiolated metal NCs. At the early stage, mono-thiolate ligands such as GSH were typically used as the ligand for the synthesis of metal NCs. However, advancement in the field by many researchers has led to the development of a number of metal NCs protected by various thiolate ligands. For instance, we have developed a unique NaOH-mediated NaBH4-reduction method for producing various thiolated Au NCs. In fact, by using this method, additional surface functionalities could also be achieved by incorporating two different thiolate ligands into the NC surface (Fig. 1a).21 We found that the stability of thiolated Au NCs can be significantly improved by employing two thiol-terminated ligands having oppositely charged functional groups on the surface of the NCs.12 More recently, we also observed an intriguing optical absorption at about 780 and 980 nm of Au25 NCs protected by negatively charged thiolate ligands collocating with positively or neutrally charged thiolate ligands, which may be caused by the collocation of different charged ligands and surface charge anisotropy of thiolated Au NCs (Fig. 1b).22 These studies may open a new window in the investigation of the physicochemical properties of metal NCs and further facilitate their practical applications by designing their surface ligands.
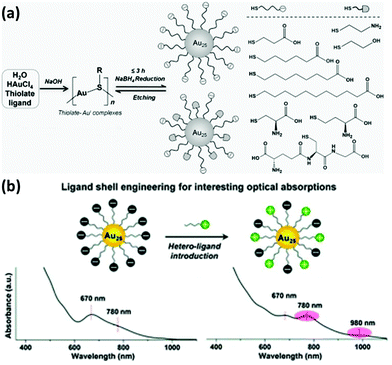 |
| Fig. 1 (a) Schematic illustration of the NaOH-mediated NaBH4-reduction method for the synthesis of thiolated Au NCs. Reprinted with permission from ref. 21. Copyright (2014) Wiley-VCH. (b) Schematic illustration and the intriguing optical absorption spectra of Au25 NCs protected by negatively charged thiolate ligands collocating with positively charged thiolate ligands. Reprinted with permission from ref. 22. Copyright (2016) Royal Society of Chemistry. | |
In addition to the mono-thiolate ligands, di-thiolate ligands (i.e., a ligand containing two thiol groups) also gained significant attention for the functionalization of metal NCs due to their distinctive properties such as high stability and strong luminescence. For example, Au NCs functionalized with di-thiolate ligand LA exhibited good long-term colloidal stability over a wide range of pH (2–13) and in the presence of high electrolyte concentration, making these LA-functionalized Au NCs promising for use in imaging and sensing applications.23 Similarly, dihydrolipoic acid (DHLA)-protected Ag NCs also showed high stability, which can be possibly ascribed to the bidentate chelating effect afforded by the di-thiolate group.24 Interestingly, the formation of the Au–di-thiolate bonding manner can also affect the physical properties of the NCs such as their optical properties. Wang and coworkers reported the synthesis of di-thiolate ligand 2,3-dimercaptopropane sulfonic (DMPS) protected Au4 clusters with high purity.25 Later, mixed di-thiolate durene and mono-thiolate phenylethanethiolate protected Au130 clusters synthesized by Wang's group was demonstrated to display multiple discrete absorption bands at about 355, 490, 584, and 718 nm, which may be attributed to the unique structural constraints imposed by the di-thiolate ligand on the interfacial bonding structures at the gold core surface.26 Despite the progress of di-thiolate-ligand-protected metal NCs, the studies in the field are still limited as compared to those studies in the field of mono-thiolated metal NCs, and therefore, more investigations should be carried out in future to provide more detailed and deeper information about their structures and properties in order to make them useful for practical applications.27,28
2.1.2 Functionalization with biomolecules.
The functionalization of biomolecules on metal NCs is of crucial significance in chasing biocompatible platforms with minimal toxicity for biomedical applications. In the next section, we will discuss various biomolecule-based platforms such as nucleic acids, proteins, and peptides that have been used as templates for the synthesis and in situ functionalization of various metal NCs.
2.1.2.1 DNA oligonucleotides.
DNA oligonucleotides have been used for a long time for the functionalization of various nanomaterials such as Au NPs and silica NPs.29 With the development of DNA-based architectures, studies on the binding between a metal ion/complex and DNA have also drawn much research interest. In 2004, Dickson and coworkers used DNA as templates for the synthesis of DNA-encapsulated Ag NCs (DNA-Ag NCs) due to the strong interaction between Ag+ and DNA sequences prior to the reduction (Fig. 2a).30 Thereafter, more studies have been carried out to synthesize luminescent Ag NCs by using different DNA sequences, as well as to unravel the binding mechanism between DNA and the encapsulated Ag NCs.6 It has been reported that Ag NCs prefer to be functionalized with cytosine- or thymine-rich single stranded DNA due to the strong binding between Ag(I) and the N3 of cytosine or thymine.14 In fact, Ag NCs can be encapsulated in DNA with different structures, which include duplex, hairpin, G-quadruples and i-motif structures.6 DNA functionalization enables the Ag NCs to be well designed to fulfil different applications, such as incorporating the DNA tail with some aptamers to achieve specific binding and cell targeting.31
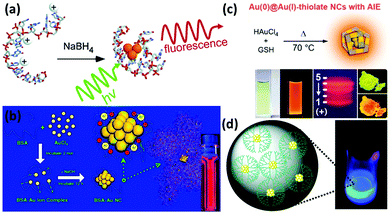 |
| Fig. 2 (a) Schematic illustration of the formation of DNA-Ag NCs. Reprinted with permission from ref. 30. Copyright (2004) American Chemical Society. (b) Schematic illustration of the formation of Au NCs in BSA solution. Reprinted with permission from ref. 37. Copyright (2009) American Chemical Society. (c) Schematic illustration of the synthesis of highly luminescent Au NCs with aggregation-induced emission (AIE) properties. Reprinted with permission from ref. 50. Copyright (2012) American Chemical Society. (d) G4-OH PAMAM-encapsulated Au NCs and their luminescence under UV irradiation. Reprinted with permission from ref. 53. Copyright (2003) American Chemical Society. | |
Motivated by the successful synthesis of DNA-Ag NCs, other metal NCs such as Au and Cu functionalized with DNA have also been reported. For example, Liu and coworkers demonstrated the preparation of DNA-encapsulated Au NCs with blue emission under mild reduction conditions.32 The formation of Au and Cu NCs is also highly dependent on the DNA sequences.33 Interestingly, both double stranded DNA and poly-thymine single stranded DNA are proven to be able to protect the Cu NCs.34–36 Compared to Ag NCs that could be functionalized with ample DNA sequences, the functionalization of Au and Cu NCs with DNA still has a long way to go, especially for the exploitation of their physicochemical properties for practical applications.
2.1.2.2 Proteins and peptides.
Proteins and peptides are biological macromolecules with an abundance of functional groups, such as carboxyl, thiol and amino groups. Some of these functional groups can be either used as reducing agents or served as protecting ligands/capping agents for metal NCs. For example, based on this unique property, we firstly reported the synthesis of protein-protected Au NCs by using bovine serum albumin (BSA) as a model protein (Fig. 2b).37 In this system, the protein layer on the NC surface could not only provide a number of outer functional groups (for their further functionalization if necessary), but also endow them with good biocompatibility in the biological set-up. The as-synthesized BSA-protected Au NCs (BSA-Au NCs) exhibited strong red emission with a high quantum yield (QY) of ∼6%, and they also featured good stability in various media. Each of these properties made crucial contributions of protein-protected Au NCs in sensing, imaging and therapeutic applications.5,38,39 Following this work, various Au NCs have been synthesized and functionalized with different proteins, such as lysozyme,40 horseradish peroxidase,41 insulin,42 glucose oxidase,43 and ovalbumin.44 Some other metal NCs such as Ag and Cu have also been reported by using proteins as functionalizing ligands.45,46 Interestingly, the bioactivity of these proteins can be largely preserved in some cases, even after the synthesis of metal NCs. For example, the insulin-protected Au NCs (with red emission) can be used for fluorescence imaging, computed tomography (CT) imaging, as well as for in vivo blood-glucose regulation.42 The results strongly imply that the as-synthesized insulin-protected Au NCs largely retained the bioactivity as that of the commercial insulin. This is probably due to the ultrasmall size of Au NCs and the mild reaction conditions for the synthesis, which would not disturb the conformation and active sites of the protein.
Like protein, peptide is another class of attractive biomolecules that could be used to functionalize metal NCs. In particular, the amine and thiol groups of peptides could contribute to the protection and functionalization of metal NCs. Among the newly developed peptides for metal NCs, GSH is probably the most common choice for the preparation of metal NCs, including Au, Ag, Pt, and Cu NCs.47–49 For example, we recently developed a facile synthesis strategy for the ultra-bright Au NCs (GSH-Au NCs, QY ∼ 15%) by using GSH as both protecting and reducing agents under the reaction at 70 °C for 24 h (Fig. 2c).50 In some cases, long peptides can also be introduced to the surface of metal NCs.51 It should be noted that the designable sequence of peptides allows the synthesis of metal NCs with some unique properties, further endowing them with a certain charge and targeting capability for various applications, particularly in the biological set-up.52
2.1.2.3 Dendrimers and polymers.
Dendrimers and polymers are also able to encapsulate and functionalize metal NCs. The presence of a myriad of functional groups within polymers and dendrimers makes them as promising templates for the facile synthesis of metal NCs with good water-solubility and high biocompatibility. For instance, poly(amidoamine) (PAMAM) dendrimers have been used as a scaffold for the synthesis and functionalization of Au (Fig. 2d) and Ag NCs.53,54 These NCs showed high stability in solution and exhibited high QY. In addition, their surface functionality can also be easily changed due to the availability of diverse generations of dendrimers. Another interesting fact is that these metal NCs are generally formed in the cavity of dendrimers, resulting in a highly charged outer surface. The dendrimer shell can provide a steric protection to improve the stability of metal NCs, and allow a further functionalization of these metal NCs through either a simple electrostatic interaction or a chemical conjugation for carrying the therapeutic agents.55 Apart from Au and Ag NCs, functionalized bimetallic NCs can also be obtained by using dendrimers as templates since dendrimers may possess robust metal chelating capability. For example, Au–Pd and Ag–Cu NCs have been successfully encapsulated in PAMAM dendrimers.56,57 Similar to dendrimers, polymers with abundant functional groups are also proven to be competent agents for stabilizing metal NCs. Several synthetic methods such as photo-reduction, chemical etching and microwave-assisted are reported for the preparation of polymer-encapsulated metal NCs.58–60 And a variety of polymers, including poly(ethylenimine) (PEI)61,62 and poly(vinylpyrrolidone) (PVP)63 have been largely used as protecting agents for the functionalization of metal NCs with various metal compositions.64
2.2 Post-synthesis functionalization
2.2.1 Bioconjugation.
Bioconjugation is a powerful means to provide additional and useful functionalities on metal NCs. After the synthesis of metal NCs, a delicate selection of good stabilizers with desirable terminal functionalities, such as primary amine, carboxylic acid, and alcohol, is generally required to prevent the metal NCs from aggregation in solution and in a complicated biological environment. Started from the already formed metal NCs, it is sometimes necessary or beneficial to make further modifications with bio-related agents to broaden their applications in biomedical science. These bio-related agents have a wide spectrum of choices, ranging from small molecules (e.g., folic acid) to macromolecules (e.g., proteins and DNA) and genes. Conjugation chemistry mainly relies on the interaction between the terminal functional groups of the metal NCs and the functional groups of the desired biomolecules. One such post conjugation protocol is N-(3-dimethylaminopropyl)-N-ethyl carbodiimide (EDC) activated coupling between amine and carboxylic groups. For example, LA-protected Au NCs have been further functionalized with photosensitizers, namely protoporphyrin IX through the EDC and the N-hydroxysuccinimide (NHS) coupling reaction, which were designed for cancer treatment (Fig. 3).65 In addition to the small molecules, proteins and viruses are also effective to be integrated into functional metal NCs. For example, a model virus was recently functionalized on the surface of the as-designed water-soluble Au NCs.66 In particular, Au NCs with a carboxylic acid on the surface were first reacted with a six-carbon alcohol with terminal maleimide, resulting in the formation of maleimide-functionalized Au NCs. The as-functionalized Au NCs were further conjugated with a model virus, leading to the formation of virus-functionalized Au NCs. Over the past several years, bioconjugation of metal NCs has risen as one of the most popular strategies to functionalize the NC surfaces with desirable functionalities as the formation of irreversible bonds could largely benefit their practical applications.67
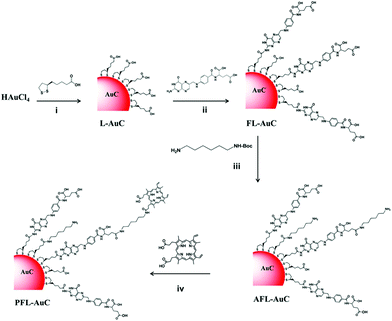 |
| Fig. 3 Synthetic scheme of the photosensitizer-conjugated Au NCs. Reprinted with permission from ref. 65. Copyright (2015) American Chemical Society. | |
2.2.2 Post-synthesis ligand exchange.
Post-synthesis ligand exchange is another efficient route for surface functionalization of metal NCs in solution. Ligand exchange reactions are known to be vital for the surface modification of quantum dots (QDs) and metal nanocrystals.68,69 In the NC research, the early work of Murray and coworkers70,71 has proven the ligand exchange reaction to be effective to functionalize the as-obtained metal NCs with new ligands and additional functionalities.72–75
Generally, the original ligands on metal NCs could be partially or completely exchanged by the incoming ligands depending on the NC species and the reaction conditions such as the concentration of NCs and the type of incoming ligand.76,77 In the case of partial ligand exchange, a selective functionality is incorporated into the NCs by exchanging some ligands on certain sites of NCs, without affecting the structures of the NCs. The complete exchange process, however, can replace the original monolayers with the desired functional groups along with a change of composition, structure, and properties of the resultant metal NCs in most cases.
Functionalization of metal NCs by ligand exchange can further benefit their applications since the most stable NC species and desirable surface functionalities can be readily achieved in a delicate ligand exchange design. In addition, unlike the polydisperse chemical compositions in the products obtained from a typical direct synthesis method, the ligand exchange process might make possible the generation of NCs with a unique size distribution.74,78 For example, Tsukuda and coworkers carried out an interesting experiment, in which phosphine-stabilized Au11 NC was the ligand exchanged with GSH in water (Fig. 4a).74 The resulting Au25(SG)18 NCs were selectively produced in a large yield and high quality. Moreover, the new functionality imparted on metal NCs after the ligand exchange process can also be further modified with some specific agents for targeting purpose.79–81 As indicated above, the understanding of the ligand exchange process is of great importance for the functionalization and application of metal NCs.
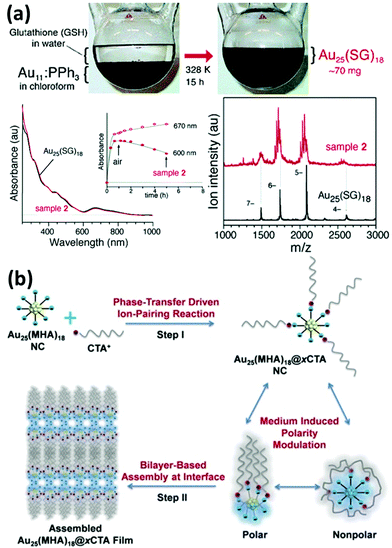 |
| Fig. 4 (a) Schematic illustration and characterization of the ligand exchange reaction between phosphine-stabilized Au11 NCs (in chloroform) and GSH in water. Reprinted with permission from ref. 74. Copyright (2005) American Chemical Society. (b) Schematic illustration of the synthesis of amphiphilic Au25(MHA)18@xCTA NCs by the phase-transfer driven ion-pairing reaction. Reprinted with permission from ref. 83. Copyright (2015) American Chemical Society. | |
2.2.3 Non-covalent interaction.
A non-covalent interaction such as electrostatic interaction is also a good means to further functionalize the as-synthesized metal NCs in solution. Generally, proteins with a certain charge can be functionalized on the surface of NPs and NCs carrying an opposite charge, through a direct electrostatic interaction. For example, Chang and coworkers have functionalized the 11-mercaptoundecanoic acid (MUA)-protected Au NCs with platelet derived growth factor AA (PDGF AA) after a simple incubation at room temperature.82 Recently, we have developed a simple surface modification method for the preparation of an amphiphilic metal NC (Fig. 4b).83 In particular, 6-mercaptohexanoic acid (MHA)-protected Au NCs with a certain number of anionic surface groups (carboxylate, −COO−) can be ion-paired with some hydrophobic cations (e.g., cetyl-trimethyl-ammonium, CTA+), generating amphiphilic Au NCs largely due to the coexistence of flexible hydrophilic MHA and hydrophobic MHA⋯CTA ligands in a comparable amount onto the NC surface. With the imparted amphiphilicity, the as-designed metal NCs may be attractive in the design of NC-based hybrid nanomaterials and self-assemblies, finding potential in both basic and applied researches. In another elegant study, Lee and coworkers demonstrated an effective post functionalization strategy to enhance the luminescence efficiencies of well-defined gold clusters.84 In particular, Au22(SG)18 NCs functionalized with tetraoctylammonium (TOA) cations showed a luminescence QY greater than 60% due to the rigidified Au(I)–thiolate shell caused by the significant intramolecular interaction between the alkyl chains of TOA cations.
2.3 Metal NC-based nanocomposites
The improvement of materials’ performance can provide further opportunity to broaden their utility in practical applications. The influence of inorganic materials based nanocomposites on the physicochemical properties of metal NCs has received worldwide research interest owing to the possibility of generating multiple functions in a single entity. Compared with individual metal NCs, these hybrid nanocomposites may feature improved performance along with some new emerged properties resulting from the synergistic effects of metal NCs and other components. Some examples are discussed below.
2.3.1 Carbon-based nanomaterials.
Carbon-based nanomaterials have been taken into account for the development of NC-based nanocomposites. Among the carbon-based materials, graphene and its water-soluble derivative, graphene oxide (GO), has been particularly considered as an important class of materials for the construction of NC-based hybrid nanocomposites with a desired function. Due to the presence of large specific surface areas and sp2-hybridized carbons in GO, recently, non-covalent fabrication has emerged as a promising method for developing such a hybrid nanocomposite of metal NCs and GO.85–87 This fabrication can be easily managed through the design of NC surface functionalities such as DNA and protein for integrating the NCs with GO. For example, Willner and coworkers synthesized the nanocomposites of DNA-Ag NCs and GO, which provide a versatile platform for the detection of genes and proteins, as well as the analysis of aptamer–substrate complexes (Fig. 5a).88 In their study, the single stranded nucleic acids acted as templates to prepare Ag NCs, followed by their absorption by GO owing to the π–π stacking interactions between hexagonal graphitic units of GO and nucleotides. Proteins could also facilitate the assembly of metal NCs and GO. For instance, Yan and coworkers used transferrin-functionalized Au NCs (Tf-Au NCs) and GO to form nanocomposites (Tf-Au NCs/GO) through the hydrogen bond and a hydrophobic interaction between Tf and GO.89 Due to the diversity of proteins, the surface charge of proteins can also contribute to the fabrication of nanocomposites of metal NCs and GO. Another good example is from Qu and coworkers. They used the lysozyme-stabilized Au NCs (lysozyme-Au NCs) to integrate with GO through the electrostatic interactions in which lysozyme is positively charged while GO is negatively charged at neutral pH (Fig. 5b).90 Alternatively, the easy synthesis process could also make possible the straightforward growth of metal NCs on the surface of GO.91–93 It is reported that the adsorption of metal salts on the preformed DNA/GO complexes, followed by the reduction of metal ions could construct Pt NCs and GO nanocomposites with a good environmental stability and durability.94 Apart from GO, carbon nanotubes (CNTs) were also widely used for fabricating NC-based nanocomposites. Various metal NCs, including Ag, Au, Pt, and Cu, have been functionalized with CNTs through multifarious strategies.95–97 For example, DNA-Ag NCs have been functionalized with CNTs to form CNT-Ag NC nanocomposites, which can be used as an efficient probe for an amplified detection of analytes.95
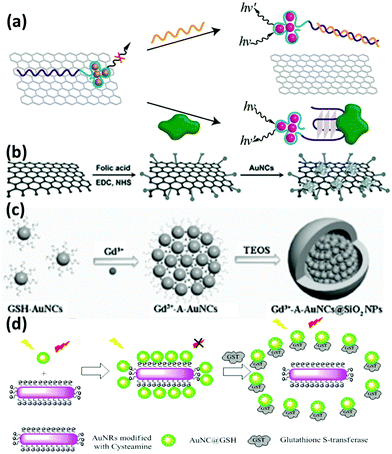 |
| Fig. 5 Schematic illustration of the preparation of the nanocomposites of (a) DNA-Ag NCs/GO; reprinted with permission from ref. 88. Copyright (2013) American Chemical Society. (b) Lysozyme-Au NCs/GO; reprinted with permission from ref. 90. Copyright (2013) Wiley-VCH. (c) Gd3+-A-Au NCs@SiO2 NPs; reprinted with permission from ref. 100. Copyright (2014) Wiley-VCH. (d) GSH-Au NCs and Au NRs based FRET system. Reprinted with permission from ref. 104. Copyright (2015) American Chemical Society. | |
2.3.2 Silica coating.
Silica coating is one of the most used strategies for the surface modification of nanomaterials for biological applications.98 NPs with a silica shell often exhibit good water-solubility and stability as well as a low nonspecific binding with the biological matrix. Different from metal NPs with a relatively larger size, metal NCs are very small and it is a great challenge to coat a silica shell on metal NCs in a well-controlled manner.99,100 Recently, Wang and Lin reported the Gd3+-induced aggregation of Au NCs, followed by the encapsulation of the resulting aggregated Au NCs within a silica shell, leading to the formation of Gd3+-A-Au NCs@SiO2 NPs; the as-designed nanocomposites could serve as in vitro and in vivo multimodal probes for cancer cell imaging (Fig. 5c).100 In this system, the silica shell not only endows the nanocomposites with further surface modifications but also plays a crucial role in improving the photo and chemical stability of the Au NCs in solution. Similarly, some groups carried out the surface coating of silica on the protein-encapsulated Au NCs or the aggregated Au NCs.101–103 The as-designed silica coating has a number of traits, including good stability and ease of surface modifications with a variety of functional groups using silane chemistry and commercially available silane coupling agents.
2.3.3 Metal nanomaterials and metal oxides.
Metal nanomaterials and metal oxides are another class of good candidates that could be integrated into metal NCs to form multifunctional NC-based nanocomposites. For example, GSH-Au NCs have been functionalized with amine-terminated Au nanorods (Au NRs) to form a fluorescence resonance energy transfer (FRET) system that can be applied for the detection of glutathione S-transferase (GST) (Fig. 5d).104 Notably, semiconductor–metal composites have been widely studied in the development of catalysts. It has been reported that reducing the size of metal NPs would improve the photo-induced charge separation and then enhance the catalytic activity of the nanocomposites.105 Recently, the semiconductor–metal NC composites have been increasingly recognised as good catalysts.106 More recently, GSH-Au NCs, being photosensitizers, were loaded into TiO2 films for efficient visible light-induced hydrogen generation in neutral water.107 Overall, the construction of NC-based nanocomposites pushes forward the study of multifunctional nano-platforms and further facilitates their applications.
3. Biomedical applications
The functionalization strategies discussed above could not only improve the properties of metal NCs, such as stability in solution and biological media, and biocompatibility, but also play a vital role in the development of sensors, imaging probes and theranostic agents. The abundant surface functionalities on metal NCs can be designed either during the synthesis or through the post-synthesis treatments, making these multifunctional metal NCs a promising platform for fulfilling the differently desired applications. Since several nice reviews covering the applications of metal NCs for biomedical science have already been contributed, we will focus our discussions on their biomedical applications (e.g., imaging and therapeutic application), particularly based upon the endowed functions of functionalized metal NCs in solution as discussed above.
3.1 For special binding and targeting
With the rapid development of nanotechnology, nanomaterials based platforms are emerging as effective tools for highly specific and sensitive analysis of biological agents. Due to high QYs, good photostability, and biocompatibility, metal NCs have been widely used as signal reporters for biological analysis. However, a specific recognition in the complex biological environment still remains a great challenge since such a complexity can affect the detection limit and even sometimes induce a falsified positive result. To make these metal NCs more effective, their surfaces are often functionalized by means of biocompatible molecules.
Oligonucleotide is one such biomolecule that has been used for surface functionalization of these metal NCs. DNA-Ag NCs, particularly, have drawn much attention in the specific analysis and targeted imaging because of the simple synthesis of these NCs. To functionalize these NCs with recognizable elements, DNA aptamer sequences were often designed in the tail of a particular DNA template to achieve specific binding to various targets.108,109 Among them, an intriguing phenomenon of luminescence enhancement induced by the proximity of the G-rich overhang to Ag NCs has been exploited to develop the various target responsive sensors and imaging probes (Fig. 6a & b).110,111 Moreover, the easy coupling between template DNA and luminescence quenchers, such as organic dyes and nanomaterials, facilitates the construction of aptamer-functionalized NC-based platforms for targeted imaging.112,113
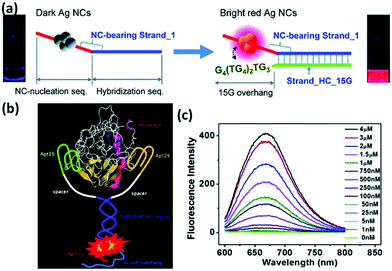 |
| Fig. 6 (a) Schematic illustration showing the red emission enhancement of DNA-Ag NCs caused by the guanine proximity. Reprinted with permission from ref. 110. Copyright (2010) American Chemical Society. (b) Schematic illustration of the proximity of G-rich overhang to Ag NCs induced by the binding of two aptamers with thrombin, and (c) fluorescence spectra of Ag NCs in the presence of different concentrations of thrombin. Reprinted with permission from ref. 111. Copyright (2012) American Chemical Society. | |
Apart from the oligonucleotides, proteins and peptide functionalized metal NCs have also made important contributions to specific binding and targeting.114 One of the advantages of protein-functionalized metal NCs is that some proteins can directly target the cancer cells and also provide a platform for further functionalization with other specific agents for targeting. For example, Irudayaraj and coworkers reported the conjugation of BSA-Au NCs with Herceptin (Her-Au NCs), which can achieve specific targeting and nuclear localization of the as-designed Au NCs in ErbB2 over-expressing breast cancer cells (Fig. 7).115 Notably, Her-Au NCs retained both the luminescence properties and the targeting capability. It was interesting that the as-designed Her-Au NCs exhibited an endosomal escape behaviour and targeted at nucleus of the cells with high efficiency, while the Alexa647 dye-labelled Herceptin primarily localized on the cell membranes since the free Herceptin has very limited ability to pass through the nuclear pore complex, indicating that functionalization of the target agents on Au NCs can in turn improve the targeting capability of the targeting agents. Likewise, the targetable Au NCs can be obtained by conjugating BSA-Au NCs with other agents, such as folic acid and TAT peptide, which can selectively bind with specific receptor expressing cancer cells and tumor tissues.116–120
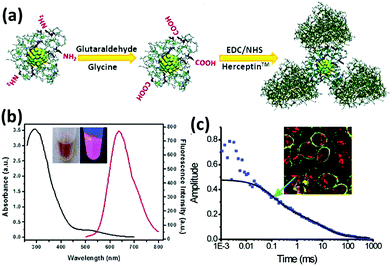 |
| Fig. 7 (a) Schematic illustration of the preparation of Her-Au NCs. (b) UV-vis absorption and luminescence spectra of Au NCs. (c) Typical autocorrelation curve (solid squares) of Her-Au NCs diffusing inside the nucleus fitted with two components (black solid line). Inset is the image of SK-BR3 cells incubated with Her-Au NC-Alexa647 for 4 h. Reprinted with permission from ref. 115. Copyright (2011) American Chemical Society. | |
3.2 For multi-modal imaging
Over the past few years, unprecedented efforts have been undertaken to improve the resolution and sensitivity of the bioimaging techniques, ranging from the traditional computed tomography (CT), ultrasound (US) imaging, and magnetic resonance imaging (MRI), to emerging optical fluorescence imaging (FL), positron emission tomography (PET), and photoacoustic imaging (PAI). With the purpose of conquering the drawbacks of a single modal imaging and ultimately improving the diagnostic accuracy, many multimodal-imaging platforms have been developed by integrating different imaging agents into a single system. In the following, we will discuss some examples in the pursuit of metal NC-based multimodal imaging platforms.
Among the various available medical imaging platforms, CT is one of the most frequently used hospital diagnostic tools with cost-effectiveness. Because of the higher atomic number and electron density of gold compared to conventionally used materials such as iodine, Au NPs have been proven to be a class of effective CT contrast agents. Since Au NCs intrinsically possess both FL and CT imaging capability, recent efforts have been geared up for designing Au NC-based multimodal imaging agents. For example, Cui and coworkers demonstrated a simple synthesis of folic acid conjugated GSH-Au NCs, which not only possess the targeting ability but also provide a versatile platform for FL and CT imaging (Fig. 8a).121 Such a dual-modal imaging probe can also be achieved by utilizing other luminescent Au NCs, such as insulin-protected Au NCs.42 For example, Zheng and coworkers reported the study of in vivo renal clearance properties of ultrasmall Au NPs by using FL and CT imaging.122
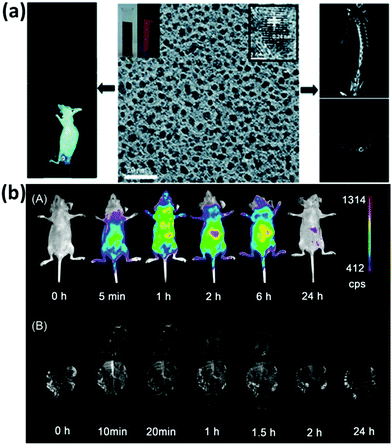 |
| Fig. 8 (a) Dual-modal FL/CT imaging of gastric cancer using folic acid conjugated GSH-Au NCs. Reprinted with permission from ref. 121. Copyright (2013) Royal Society of Chemistry. (b) (A) NIR FL and (B) MR blood in vivo imaging using BSA-Gd2O3/Au nanoprobes. Reprinted with permission from ref. 38. Copyright (2013) American Chemical Society. | |
To construct a reliable multimodal imaging probe, MRI has drawn much attention owing to its fine spatial resolution.123,124 In general, the combination of MRI contrast agents with Au NCs can be achieved through coupling Au NCs with paramagnetic agents or integrating Au NCs into magnetic NPs to form hybrid materials. Starting with the synthesis of Au NCs protected by proteins, Cai and coworkers reported the functionalization of Gd3+ on the surface of Au NCs through an electrostatic interaction to achieve FL/MRI dual-modal imaging.125 Similar tri-modal imaging probes were constructed by Kong and coworkers by functionalizing the peptide-protected Au NCs with Gd3+.126 The as-prepared hybrid Au NCs retained good stability, good biocompatibility, and low toxicity, and no obvious Gd3+ leaching was observed in different solutions. In an alternative strategy, Yan and coworkers used BSA as a template for an in situ synthesis of a multifunctional Gd2O3/Au hybrid probe, which enables a successful near-infrared (NIR) FL and MR blood pool imaging attributing to their long blood circulation time (Fig. 8b).38 Further modification of the probe with arginine–glycine–aspartic acid peptide c(RGDyK) (RGD) also enabled the nanoprobe for in vivo targeted tumor imaging. Very recently, GSH-Au NCs were also functionalized with magnetic iron oxides through an electrostatic interaction to form a composite material for FL and MRI based dual-modal imaging.127
Apart from the above-mentioned methods, Au NCs have also been functionalized with PET contrast agents to achieve the goal of multi-modal imaging. For example, Wu and coworkers described a novel strategy for the synthesis of 64Cu-decorated Au NCs through the reduction of 64CuCl followed by decoration on the surface of Au NCs.128 The as-synthesized 64Cu-decorated Au NCs can serve as self-illuminating NCs for dual-modal PET and NIR FL imaging based on Cerenkov resonance energy transfer (CRET). Other kinds of NCs, such as Ag and Cu NCs have also attracted much research interest in multi-modal imaging since Ag and Cu NCs both can be luminescent, and the radioactive [64Cu]Cu NCs can be intrinsically used for PET imaging.129,130 Due to the diverse metal NCs and the rich functionalization strategies for designing functional NCs, the developments of NC-based multifunctional contrast agents are continuously becoming popular with time.
3.3 For therapeutic application
Functionalized nanomaterials have the potential to revolutionize the diagnosis and treatment of many diseases, including cancer which still remains as a major cause of human death. The development of therapeutic nanocomposite agents offers a new hope to elucidate drug resistance mechanisms and improve treatment outcomes. There have been many efforts to employ functionalized Au NCs for the construction of therapeutic platforms. In the following section, we will highlight some examples in details.
3.3.1 Radiotherapy (RT).
Radiotherapy is one of the most effective treatments following surgical removal of tumors. However, a major challenge in RT is that the ionizing radiation cannot differentiate between the healthy tissues and solid tumors.131 Therefore, a high dose of radiation often kills the healthy cells. One efficient way to overcome this problem is to use radiosensitizers, and localize them in the tumor cells which could make tumor cells more vulnerable under radiation. It is now well-recognized that elements with a higher atomic number (Z) will be a better radiosensitizer with improved RT effects. Compared to the commonly used radiosensitizers such as carbon (Z = 6), iodine (Z = 53), gadolinium (Z = 64) and platinum (Z = 78), gold has a larger atomic number (Z = 79), therefore, it should have a stronger radiation enhancement effect. However, Au NPs with particle sizes above 50 nm were reported to be trapped by the reticuloendothelial system (RES) organs, which could result in low tumor uptake. Although the Au NPs with a size below 20 nm would escape RES absorption, a long term potential toxicity still remains since they may not be efficiently eliminated from the body.132,133 Due to the small size and low toxicity of Au NCs, recently our group used the GSH-protected Au25 NCs as radiosensitizers for cancer RT. The as-designed Au NC-based radiosensitizers have shown attractive features such as high tumor accumulation, strong radiation enhancement, good renal clearance, and low toxicity in the in vivo set-up (Fig. 9).134 Of note, the GSH-Au25 NCs showed better accumulation in tumor cells, and therefore show a stronger enhancement for cancer RT as compared to that of BSA-Au25 NCs in both in vitro and in vivo set-ups. In a more recent study, Au10–12SG10–12 and Au29–43SG27–37 NCs were also developed as efficient radiosensitizers for cancer RT, showing significant RT enhancement.132,133 It should be noted that some differences in biodistribution of Au NCs still can be observed between these three kinds of Au NCs. The data highlight that the composition and surface functionalization of Au NCs can affect their biodistribution and eventually determine their efficacy as radiosensitizers in cancer RT.
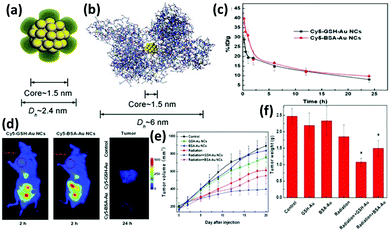 |
| Fig. 9 Schematic illustration of the core–shell structure of (a) GSH-Au25 NCs and (b) BSA-Au25 NCs. (c) In vivo pharmacokinetics studies of GSH-Au25 NCs (black line) and BSA-Au25 NCs (red line). (d) Fluorescence images of mice treated with GSH-Au25 NCs (left panel) and BSA-Au25 NCs (middle panel) at 2 h after injection; the right panel is the tumor images (false colour) at 24 h after injection of the control group (top), GSH-Au25 NC group (middle), and BSA-Au25 NC group (down). Time-course studies of tumor (e) volumes and (f) weights of mice treated with GSH- and BSA-Au25 NCs at a concentration of 10 mg Au per kg body. Reprinted with permission from ref. 134. Copyright (2014) Wiley-VCH. | |
3.3.2 Photodynamic therapy (PDT).
Photodynamic therapy, a clinically approved non-invasive therapeutic strategy, is also an alternative therapeutic method exploited for metal NCs.135 In PDT, the photosensitizers should have capability in generating reactive oxygen species (ROS) when they are irradiated with an appropriate light source. Therefore, when they are localizing at the targeted area, a selective destruction of that targeted area can be achieved by PDT from ROS generated by the photosensitizers. With the recognition that several functionalized Au NCs, such as Au NCs embedded in a polymer film, BSA-Au NCs, and thiolated Au NCs, can generate singlet oxygen species (1O2),136–138 researchers have started exploiting this class of materials as photosensitizers for PDT. For example, Jin and coworkers demonstrated that 1O2 can be efficiently produced by a direct photosensitization of captopril/phenylethanethiol-protected Au25 NCs under visible/NIR light irradiation (532, 650, and 808 nm).139 Based on this unique observation, they further used the captopril-protected Au25 NCs as biocompatible photosensitizers for PDT combating cancer cells. The advantage of using Au NCs as photosensitizers over traditional organic photosensitizers can also be evident from their tumor targeting capability. In a separate study, Hwang and coworkers developed a theranostic platform based on a nucleus-targeting Au NC (TAT peptide-Au NC) (Fig. 10).120 The as-prepared TAT peptide-Au NCs can generate 1O2 under the irradiation of 980 nm light, from a direct observation of phosphorescence emission at 1261 nm with a QY of 0.046. The as-designed peptide-Au NCs have been used for the nucleus targeted PDT.
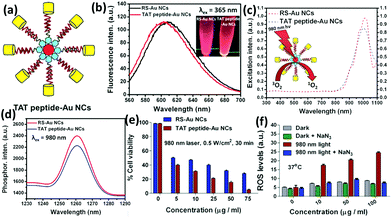 |
| Fig. 10 (a) Schematic illustration of the nucleus-targeting TAT peptide-Au NCs. (b) Photoemission spectrum of alkyl thiolated Au NCs (RS-Au NCs) and TAT peptide-Au NCs. (c) Photoexcitation spectrum of singlet oxygen phosphorescence of RS-Au NCs and TAT peptide-Au NCs (λem = 1261 nm). (d) Singlet oxygen photoemission spectra of RS-Au NCs and TAT peptide-Au NCs at 980 nm (excitation wavelength). (e) Cell viability of RS-Au NCs and TAT peptide-Au NCs internalized HeLa cells under photo-irradiation conditions at 37 °C. (f) ROS generation monitored by the mean fluorescence using flow cytometry for TAT peptide-Au NC internalized HeLa cells followed by photo-irradiation with and without NaN3 pre-treatment. Reprinted with permission from ref. 120. Copyright (2015) Wiley-VCH. | |
Apart from their use as therapeutic agents, metal NCs can also serve as carriers to deliver biomedical agents such as drugs (e.g., chemotherapeutics and photosensitizers) and genes. Therapeutic targeted delivery vehicles have made a great contribution in the fight against cancers and often showed a better performance than a single therapeutic agent which often suffers from the lack of targeting, limited tumor uptake, and sometimes severe side effects.140–142 Among the most commonly used nanomaterials to deliver drugs into the desired sections, metal NCs show some merits owing to their ultrasmall size, rich surface functionalities, low toxicity, and intrinsic strong luminescence, which could be used to develop a safe and efficient platform for imaging-guided therapy. Generally, the therapeutic agents can be functionalized on metal NCs either by a direct electrostatic interaction or by a chemical conjugation. In terms of electrostatic interactions, polymers are mainly used as linkers to bind with NCs and therapeutic agents for the formation of nanocarriers.143–147 Notably, these NC-based therapeutic vehicles can achieve a stimuli responsive delivery to reduce the systematic toxicity. In terms of chemical conjugation, thiols and proteins play an important role for the protection of metal NCs and they could be readily conjugated with a certain therapeutic agent.65,148 The metal NC-based drug delivery vehicles show great potential as a tumor-targeted drug delivery carrier and they could further act as a promising theranostic platform for multi-modal therapy and imaging-guided therapy that could improve the treatment outcome.
4. Biodistribution and toxicity
With the development of nanotechnology in biomedical research and clinical science, it is greatly desired to understand the influence of nanomaterials on human health. In order to evaluate the risks caused by the nanomaterials, plenty of efforts have been made to delineate the cellular uptake, cytotoxicity, biodistribution, as well as long term toxicity of nanomaterials. To date, Au nanomaterials are one of the most popular model materials in the systematic investigations of toxicity in biological systems. However, there are some inconsistencies existing due to the difference in the synthetic process, surface chemistry, cell lines, and evaluated methods. In contrast to the nanomaterials (or NPs) with a relatively larger size, ultrasmall metal NCs with well-defined size and structure have provided some new insights into the safety evaluation due to their attractive feature of small size with narrow size distribution, rich surface chemistry, and intriguing physical and chemical properties, allowing us to understand the related information at molecular and atomic levels.
4.1 Cellular uptake and cytotoxicity
The evaluation of toxicity would be relatively easier in the case of metal NCs as compared to the NP system due to the fact that the narrow size distribution of NCs would allow researchers to slip the size dependent toxicity that always needs to be considered in the studies of NPs. As an emerging luminescent probe for in vitro imaging and targeting, Au NCs show extremely low cytotoxicity on various cell lines. In order to systemically study the cytotoxicity of these Au NCs, it is critical to understand their cellular uptake mechanism and to study the interaction between Au NCs with the intracellular environments. Significantly, some recent studies emphasized the importance of surface functionalization towards their uptake manner and cytotoxicity.149 For example, Nienhaus and coworkers studied the cellular uptake mechanism by using LA-protected Au NCs and HeLa cells.150 It was demonstrated that Au NCs can enter cells through pinocytosis involving multiple mechanisms, making Au NCs reside firstly in endosome and later in lysosome. However, some differences can be seen when these Au NCs were conjugated with targeting agents such as Herceptin to form Her-Au NCs, which could escape the endo–lysosomal pathway and enter the nucleus of cancer cells.115,151 The difference in the uptake behaviour would also affect their cytotoxicity by generating toxic intracellular species such as ROS. Recently, we also described the different cellular internalization between Au NCs functionalized with different ligands.11 It was observed that intracellular ROS was higher in cells exposed to mercaptopropionic acid (MPA)-protected Au NCs than that in the case of GSH-Au NCs. However, this effect did not induce any detrimental cellular effect, and instead, it enhanced the cell metabolism leading to a higher cell proliferation. In another example, Tao and coworkers systematically studied the BSA-Au NCs and found that Au NCs induced the decline of cell viability to varying degrees in a cell-, dose- and time-dependent manner.152 More recently, Guével and coworkers used human derived-monocyte dendritic cells (DCs) as a model to investigate the intracellular accumulation and immune response of Au NCs functionalized with zwitterionic ligands and PEG.153 Their results revealed that zwitterionic ligand functionalized Au NCs showed better performance than the PEG-functionalized Au NCs, exhibiting low cytotoxicity and a strong immunosuppressive response, which may make the as-functionalized Au NCs a novel vaccine. Even though there is no consensus yet about the cytotoxicity of Au NCs, the surface chemistry of Au NCs does play pivotal roles in affecting the cellular uptake manner and cytotoxicity.
4.2
In vivo biodistribution and clearance
A clinical acceptable agent approved by the US Food and Drug Administration (FDA) should be effectively cleared from the body after injection in a reasonable time period.154–156 During the past few years, some NPs with a hydrodynamic diameter (HD) below 5.5 nm have shown effective and rapid clearance through kidney.154,157–159 Au NCs also have drawn much attention due to their small size and multifunctional applications in biomedicine.134,160–162 However, the surface chemistry would affect their biodistribution and clearance.163 The prime consideration is whether the size of functionalized Au NCs can satisfy the kidney filtration threshold (∼5.5 nm). It is worthy to be mentioned that some zwitterionic ligands (e.g., GSH), proteins (e.g., BSA), and PEG are the mainly used surface functionalization of Au NCs. Due to the relatively large size of proteins, protein-functionalized Au NCs would face a great challenge to be renally cleared. For example, Zhang and coworkers compared the in vivo fate and toxicity of Au NCs functionalized with GSH and BSA (Fig. 11a).160 They found that 90% of Au from GSH-Au NCs can be cleared through the renal clearance after 28 days, while only less than 5% of Au from BSA-Au NCs can be cleared in the same time period. The difference could be ascribed to the less stability of BSA-Au NCs than GSH-Au NCs in serum, resulting in the formation of aggregated BSA-Au NCs that could be absorbed by the RES organs like liver and spleen. Considering the benefits from the PEGylation of NPs, such as the reduced nonspecific accumulation and prolonged blood circulation, Zheng and coworkers showed that the PEG-functionalized Au NPs (PEG-Au NPs) demonstrated an efficient renal clearance and low RES accumulation (Fig. 11b).164 PEG-Au NPs also showed an enhanced tumor targeting than GSH-Au NPs, since the blood distribution half-life of PEG-Au NPs was an order of magnitude longer than that of the GSH-Au NPs. As revealed by these studies, the in vivo toxicity study is quite different and more complicated than the in vitro cytotoxicity study. The development of a suitable and generic functionalization strategy for metal NCs is still a challenge to further improve tumor targeting and renal clearance of the as-designed functional metal NCs.
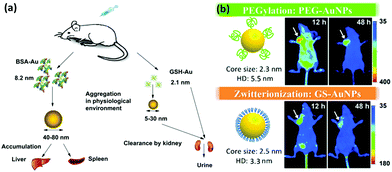 |
| Fig. 11 (a) Outline of the biodistribution and renal clearance the GSH- and BSA-Au25 NCs. Reprinted with permission from ref. 160. Copyright (2012) Elsevier. (b) PEGylation and zwitterionization have distinct effects on the pharmacokinetic and tumor-targeting properties of luminescent Au NPs. Reprinted with permission from ref. 164. Copyright (2013) Wiley-VCH. | |
5. Summary and perspective
In summary, we have attempted to give a brief overview on the recent achievements in the functionalization of metal NCs and their related biomedical applications. Owing to the unique properties of metal NCs including their attractive physical and chemical properties and good biocompatibility,165–167 the recent rapid developments in the synthesis and surface functionalization of metal NCs have enabled the exploitation of these functionalized NCs for biomedical applications. In one way, these designable surface functionalization strategies can adjust their properties such as stability in solution, strong luminescence, as well as biodistribution and toxicity in the biological set-up, which in turn can empower these functional metal NCs with desirable targeting, imaging, and therapeutic ability for biomedical applications.
Although the surface functionalization of metal NCs has been extensively studied, and it has contributed significantly to push forward the biomedical applications of metal NCs in the past decade, there are still many aspects that should be understood in future. Since the physical and chemical properties of metal NCs could be obviously affected by their surface chemistry, it is desirable to have a deeper fundamental understanding on the principles about how to tailor metal NCs with desired properties, such as stability, structure, and luminescence. Moreover, it remains a challenge to precisely incorporate functional metal NCs with a certain number of functional ligands (or controllable functionalities on the NC surface). To fulfil the practical need and translate metal NCs into clinical practice, more systematic studies should be carried out to figure out the effects of functionalities of metal NCs on their targeting, biodistribution, and toxicity in the complicated biological set-up. By integrating the intrinsic luminescence of metal NCs with other imaging and therapeutic agents, multifunctional metal NC-based nanocomposites would be achieved for multi-modal imaging guided therapy. We can anticipate that the functionalized metal NCs would serve as an important platform in the area of biomedical applications.
Acknowledgements
The authors acknowledge the financial support from the Ministry of Education, Singapore, under Grant No. R-279-000-409-112.
References
- R. Jin, Nanoscale, 2015, 7, 1549 RSC
.
- C. M. Aikens, J. Phys. Chem. Lett., 2011, 2, 99 CrossRef CAS PubMed
.
- A. Desireddy, B. E. Conn, J. Guo, B. Yoon, R. N. Barnett, B. M. Monahan, K. Kirschbaum, W. P. Griffith, R. L. Whetten, U. Landman and T. P. Bigioni, Nature, 2013, 501, 399 CrossRef CAS PubMed
.
- R. W. Murray, Chem. Rev., 2008, 108, 2688 CrossRef CAS PubMed
.
- Z. Luo, K. Zheng and J. Xie, Chem. Commun., 2014, 50, 5143 RSC
.
- B. Han and E. Wang, Anal. Bioanal. Chem., 2012, 402, 129 CrossRef CAS PubMed
.
- N. Goswami, K. Zheng and J. Xie, Nanoscale, 2014, 6, 13328 RSC
.
- Z. Wu and R. Jin, Nano Lett., 2010, 10, 2568 CrossRef CAS PubMed
.
- T. D. Green, C. Yi, C. Zeng, R. Jin, S. McGill and K. L. Knappenberger, J. Phys. Chem. A, 2014, 118, 10611 CrossRef CAS PubMed
.
- W. Kurashige, M. Yamaguchi, K. Nobusada and Y. Negishi, J. Phys. Chem. Lett., 2012, 3, 2649 CrossRef CAS PubMed
.
- C. Y. Tay, Y. Yu, M. I. Setyawati, J. Xie and D. T. Leong, Nano Res., 2014, 7, 805 CrossRef CAS
.
- X. Yuan, N. Goswami, I. Mathews, Y. Yu and J. Xie, Nano Res., 2015, 8, 3488 CrossRef CAS
.
- L.-Y. Chen, C.-W. Wang, Z. Yuan and H.-T. Chang, Anal. Chem., 2015, 87, 216 CrossRef CAS PubMed
.
- L. Shang, S. Dong and G. U. Nienhaus, Nano Today, 2011, 6, 401 CrossRef CAS
.
- S. Knoppe and T. Bürgi, Acc. Chem. Res., 2014, 47, 1318 CrossRef CAS PubMed
.
- C. D. Bain, E. B. Troughton, Y. T. Tao, J. Evall, G. M. Whitesides and R. G. Nuzzo, J. Am. Chem. Soc., 1989, 111, 321 CrossRef CAS
.
- H. Häkkinen, Nat. Chem., 2012, 4, 443 CrossRef PubMed
.
- C. J. Ackerson, P. D. Jadzinsky and R. D. Kornberg, J. Am. Chem. Soc., 2005, 127, 6550 CrossRef CAS PubMed
.
- T. G. Schaaff, G. Knight, M. N. Shafigullin, R. F. Borkman and R. L. Whetten, J. Phys. Chem. B, 1998, 102, 10643 CrossRef CAS
.
- M. J. Hostetler, J. E. Wingate, C.-J. Zhong, J. E. Harris, R. W. Vachet, M. R. Clark, J. D. Londono, S. J. Green, J. J. Stokes, G. D. Wignall, G. L. Glish, M. D. Porter, N. D. Evans and R. W. Murray, Langmuir, 1998, 14, 17 CrossRef CAS
.
- X. Yuan, B. Zhang, Z. Luo, Q. Yao, D. T. Leong, N. Yan and J. Xie, Angew. Chem., Int. Ed., 2014, 53, 4623 CrossRef CAS PubMed
.
- X. Yuan, N. Goswami, W. Chen, Q. Yao and J. Xie, Chem. Commun., 2016, 52, 5234 RSC
.
- F. Aldeek, M. A. Muhammed, G. Palui, N. Zhan and H. Mattoussi, ACS Nano, 2013, 7, 2509 CrossRef CAS PubMed
.
- Z. Wu, E. Lanni, W. Chen, M. E. Bier, D. Ly and R. Jin, J. Am. Chem. Soc., 2009, 131, 16672 CrossRef CAS PubMed
.
- Z. Tang, B. Xu, B. Wu, M. W. Germann and G. Wang, J. Am. Chem. Soc., 2010, 132, 3367 CrossRef CAS PubMed
.
- Z. Tang, D. A. Robinson, N. Bokossa, B. Xu, S. Wang and G. Wang, J. Am. Chem. Soc., 2011, 133, 16037 CrossRef CAS PubMed
.
- H. Yang, Y. Wang, H. Huang, L. Gell, L. Lehtovaara, S. Malola, H. Häkkinen and N. Zheng, Nat. Commun., 2013, 4, 2422 Search PubMed
.
- X.-K. Wan, S.-F. Yuan, Q. Tang, D.-E. Jiang and Q.-M. Wang, Angew. Chem., Int. Ed., 2015, 54, 5977 CrossRef CAS PubMed
.
- S. Y. Park, A. K. Lytton-Jean, B. Lee, S. Weigand, G. C. Schatz and C. A. Mirkin, Nature, 2008, 451, 553 CrossRef CAS PubMed
.
- J. T. Petty, J. Zheng, N. V. Hud and R. M. Dickson, J. Am. Chem. Soc., 2004, 126, 5207 CrossRef CAS PubMed
.
- J. Liu, TrAC, Trends Anal. Chem., 2014, 58, 99 CrossRef CAS
.
- T. A. C. Kennedy, J. L. MacLean and J. Liu, Chem. Commun., 2012, 48, 6845 RSC
.
- G. Liu, Y. Shao, K. Ma, Q. Cui, F. Wu and S. Xu, Gold Bull., 2012, 45, 69 CrossRef CAS
.
- G. Liu, Y. Shao, J. Peng, W. Dai, L. Liu, S. Xu, F. Wu and X. Wu, Nanotechnology, 2013, 24, 345502 CrossRef PubMed
.
- Z. Qing, X. He, D. He, K. Wang, F. Xu, T. Qing and X. Yang, Angew. Chem., Int. Ed., 2013, 52, 9719 CrossRef CAS PubMed
.
- A. Rotaru, S. Dutta, E. Jentzsch, K. Gothelf and A. Mokhir, Angew. Chem., Int. Ed., 2010, 49, 5665 CrossRef CAS PubMed
.
- J. Xie, Y. Zheng and J. Y. Ying, J. Am. Chem. Soc., 2009, 131, 888 CrossRef CAS PubMed
.
- S.-K. Sun, L.-X. Dong, Y. Cao, H.-R. Sun and X.-P. Yan, Anal. Chem., 2013, 85, 8436 CrossRef CAS PubMed
.
- J. Xie, Y. Zheng and J. Y. Ying, Chem. Commun., 2010, 46, 961 RSC
.
- Y.-H. Lin and W.-L. Tseng, Anal. Chem., 2010, 82, 9194 CrossRef CAS PubMed
.
- F. Wen, Y. Dong, L. Feng, S. Wang, S. Zhang and X. Zhang, Anal. Chem., 2011, 83, 1193 CrossRef CAS PubMed
.
- C.-L. Liu, H.-T. Wu, Y.-H. Hsiao, C.-W. Lai, C.-W. Shih, Y.-K. Peng, K.-C. Tang, H.-W. Chang, Y.-C. Chien, J.-K. Hsiao, J.-T. Cheng and P.-T. Chou, Angew. Chem., Int. Ed., 2011, 50, 7056 CrossRef CAS PubMed
.
- X. Xia, Y. Long and J. Wang, Anal. Chim. Acta, 2013, 772, 81 CrossRef CAS PubMed
.
- L.-L. Wang, J. Qiao, L. Qi, X.-Z. Xu and D. Li, Sci. China: Chem., 2015, 58, 1508 CrossRef CAS
.
- N. Goswami, A. Giri, M. S. Bootharaju, P. L. Xavier, T. Pradeep and S. K. Pal, Anal. Chem., 2011, 83, 9676 CrossRef CAS PubMed
.
- C. Guo and J. Irudayaraj, Anal. Chem., 2011, 83, 2883 CrossRef CAS PubMed
.
- S. Kumar, M. D. Bolan and T. P. Bigioni, J. Am. Chem. Soc., 2010, 132, 13141 CrossRef CAS PubMed
.
- Y. Negishi, K. Nobusada and T. Tsukuda, J. Am. Chem. Soc., 2005, 127, 5261 CrossRef CAS PubMed
.
- X. Yuan, Z. Luo, Q. Zhang, X. Zhang, Y. Zheng, J. Y. Lee and J. Xie, ACS Nano, 2011, 5, 8800 CrossRef CAS PubMed
.
- Z. Luo, X. Yuan, Y. Yu, Q. Zhang, D. T. Leong, J. Y. Lee and J. Xie, J. Am. Chem. Soc., 2012, 134, 16662 CrossRef CAS PubMed
.
- Q. Wen, Y. Gu, L.-J. Tang, R.-Q. Yu and J.-H. Jiang, Anal. Chem., 2013, 85, 11681 CrossRef CAS PubMed
.
- S. Lee, J. Xie and X. Chen, Biochemistry, 2010, 49, 1364 CrossRef CAS PubMed
.
- J. Zheng, J. T. Petty and R. M. Dickson, J. Am. Chem. Soc., 2003, 125, 7780 CrossRef CAS PubMed
.
- J. Zheng and R. M. Dickson, J. Am. Chem. Soc., 2002, 124, 13982 CrossRef CAS PubMed
.
- J. Li, W. Wang, D. Sun, J. Chen, P.-H. Zhang, J.-R. Zhang, Q. Min and J.-J. Zhu, Chem. Sci., 2013, 4, 3514 RSC
.
- G. Li and Y. Luo, Inorg. Chem., 2008, 47, 360 CrossRef CAS PubMed
.
- R. W. Scott, O. M. Wilson, S. K. Oh, E. A. Kenik and R. M. Crooks, J. Am. Chem. Soc., 2004, 126, 15583 CrossRef CAS PubMed
.
- I. Díez, M. Pusa, S. Kulmala, H. Jiang, A. Walther, A. S. Goldmann, A. H. E. Müller, O. Ikkala and R. H. A. Ras, Angew. Chem., Int. Ed., 2009, 48, 2122 CrossRef PubMed
.
- S. Liu, F. Lu and J.-J. Zhu, Chem. Commun., 2011, 47, 2661 RSC
.
- H. Duan and S. Nie, J. Am. Chem. Soc., 2007, 129, 2412 CrossRef CAS PubMed
.
- F. Qu, N. B. Li and H. Q. Luo, Anal. Chem., 2012, 84, 10373 CrossRef CAS PubMed
.
- F. Qu, N. B. Li and H. Q. Luo, Langmuir, 2013, 29, 1199 CrossRef CAS PubMed
.
- B. Santiago González, M. J. Rodríguez, C. Blanco, J. Rivas, M. A. López-Quintela and J. M. G. Martinho, Nano Lett., 2010, 10, 4217 CrossRef PubMed
.
- Y. Ling, N. Zhang, F. Qu, T. Wen, Z. F. Gao, N. B. Li and H. Q. Luo, Spectrochim. Acta, Part A, 2014, 118, 315 CrossRef CAS PubMed
.
- L. V. Nair, S. S. Nazeer, R. S. Jayasree and A. Ajayaghosh, ACS Nano, 2015, 9, 5825 CrossRef CAS PubMed
.
- V. Marjomäki, T. Lahtinen, M. Martikainen, J. Koivisto, S. Malola, K. Salorinne, M. Pettersson and H. Häkkinen, Proc. Natl. Acad. Sci. U. S. A., 2014, 111, 1277 CrossRef PubMed
.
- R. Ban, E. S. Abdel-Halim, J. Zhang and J.-J. Zhu, Analyst, 2015, 140, 1046 RSC
.
- F. Dubois, B. Mahler, B. Dubertret, E. Doris and C. Mioskowski, J. Am. Chem. Soc., 2007, 129, 482 CrossRef CAS PubMed
.
- R. Sardar, A. M. Funston, P. Mulvaney and R. W. Murray, Langmuir, 2009, 25, 13840 CrossRef CAS PubMed
.
- M. J. Hostetler, A. C. Templeton and R. W. Murray, Langmuir, 1999, 15, 3782 CrossRef CAS
.
- A. C. Templeton, M. P. Wuelfing and R. W. Murray, Acc. Chem. Res., 2000, 33, 27 CrossRef CAS PubMed
.
- L. G. AbdulHalim, N. Kothalawala, L. Sinatra, A. Dass and O. M. Bakr, J. Am. Chem. Soc., 2014, 136, 15865 CrossRef CAS PubMed
.
- S. Knoppe, R. Azoulay, A. Dass and T. Bürgi, J. Am. Chem. Soc., 2012, 134, 20302 CrossRef CAS PubMed
.
- Y. Shichibu, Y. Negishi, T. Tsukuda and T. Teranishi, J. Am. Chem. Soc., 2005, 127, 13464 CrossRef CAS PubMed
.
- G. H. Woehrle, L. O. Brown and J. E. Hutchison, J. Am. Chem. Soc., 2005, 127, 2172 CrossRef CAS PubMed
.
- E. S. Shibu, M. A. H. Muhammed, T. Tsukuda and T. Pradeep, J. Phys. Chem. C, 2008, 112, 12168 CAS
.
- A. Fernando and C. M. Aikens, J. Phys. Chem. C, 2015, 119, 20179 CAS
.
- M. S. Bootharaju, V. M. Burlakov, T. M. D. Besong, C. P. Joshi, L. G. AbdulHalim, D. M. Black, R. L. Whetten, A. Goriely and O. M. Bakr, Chem. Mater., 2015, 27, 4289 CrossRef CAS
.
- S.-I. Tanaka, J. Miyazaki, D. K. Tiwari, T. Jin and Y. Inouye, Angew. Chem., Int. Ed., 2011, 50, 431 CrossRef CAS PubMed
.
- S.-Y. Lin, N.-T. Chen, S.-P. Sum, L.-W. Lo and C.-S. Yang, Chem. Commun., 2008, 4762 RSC
.
- C.-A. J. Lin, T.-Y. Yang, C.-H. Lee, S. H. Huang, R. A. Sperling, M. Zanella, J. K. Li, J.-L. Shen, H.-H. Wang, H.-I. Yeh, W. J. Parak and W. H. Chang, ACS Nano, 2009, 3, 395 CrossRef CAS PubMed
.
- C.-C. Huang, C.-K. Chiang, Z.-H. Lin, K.-H. Lee and H.-T. Chang, Anal. Chem., 2008, 80, 1497 CrossRef CAS PubMed
.
- Q. Yao, X. Yuan, Y. Yu, Y. Yu, J. Xie and J. Y. Lee, J. Am. Chem. Soc., 2015, 137, 2128 CrossRef CAS PubMed
.
- K. Pyo, V. D. Thanthirige, K. Kwak, P. Pandurangan, G. Ramakrishna and D. Lee, J. Am. Chem. Soc., 2015, 137, 8244 CrossRef CAS PubMed
.
- K. Yang, L. Feng, X. Shi and Z. Liu, Chem. Soc. Rev., 2013, 42, 530 RSC
.
- C. Wang, J. Li, C. Amatore, Y. Chen, H. Jiang and X.-M. Wang, Angew. Chem., Int. Ed., 2011, 50, 11644 CrossRef CAS PubMed
.
- A. Chandrasekar and T. Pradeep, J. Phys. Chem. C, 2012, 116, 14057 CAS
.
- X. Liu, F. Wang, R. Aizen, O. Yehezkeli and I. Willner, J. Am. Chem. Soc., 2013, 135, 11832 CrossRef CAS PubMed
.
- Y. Wang, J.-T. Chen and X.-P. Yan, Anal. Chem., 2013, 85, 2529 CrossRef CAS PubMed
.
- Y. Tao, Y. Lin, Z. Huang, J. Ren and X. Qu, Adv. Mater., 2013, 25, 2594 CrossRef CAS PubMed
.
- H. Yin, H. Tang, D. Wang, Y. Gao and Z. Tang, ACS Nano, 2012, 6, 8288 CrossRef CAS PubMed
.
- P. Zhao, K. He, Y. Han, Z. Zhang, M. Yu, H. Wang, Y. Huang, Z. Nie and S. Yao, Anal. Chem., 2015, 87, 9998 CrossRef CAS PubMed
.
- L.-X. Zhang, J.-L. Shi, J. Yu, Z.-L. Hua, X.-G. Zhao and M.-L. Ruan, Adv. Mater., 2002, 14, 1510 CrossRef CAS
.
- J. N. Tiwari, K. Nath, S. Kumar, R. N. Tiwari, K. C. Kemp, N. H. Le, D. H. Youn, J. S. Lee and K. S. Kim, Nat. Commun., 2013, 4, 2221 Search PubMed
.
- G. Wang, G. Xu, Y. Zhu and X. Zhang, Chem. Commun., 2014, 50, 747 RSC
.
- A. V. Ellis, K. Vjayamohanan, R. Goswaimi, N. Chakrapani, L. S. Ramanathan, P. M. Ajayan and G. Ramanath, Nano Lett., 2003, 3, 279 CrossRef CAS
.
- H. Huang, Z. He, X. Lin, W. Ruan, Y. Liu and Z. Yang, Appl. Catal., A, 2015, 490, 65 CrossRef CAS
.
- N. Erathodiyil and J. Y. Ying, Acc. Chem. Res., 2011, 44, 925 CrossRef CAS PubMed
.
- X. Wu, L. Li, L. Zhang, T. Wang, C. Wang and Z. Su, J. Mater. Chem. B, 2015, 3, 2421 RSC
.
- X. Wu, C. X. Li, S. Liao, L. Li, T. Wang, Z. Su, C. Wang, J. Zhao, C. Sui and J. Lin, Chem. – Eur. J., 2014, 20, 8876 CAS
.
- P. Huang, J. Lin, S. Wang, Z. Zhou, Z. Li, Z. Wang, C. Zhang, X. Yue, G. Niu, M. Yang, D. Cui and X. Chen, Biomaterials, 2013, 34, 4643 CrossRef CAS PubMed
.
- X. L. Guével, B. Hötzer, G. Jung and M. Schneider, J. Mater. Chem., 2011, 21, 2974 RSC
.
- Y. Wu, J. Huang, T. Zhou, M. Rong, Y. Jiang and X. Chen, Analyst, 2013, 138, 5563 RSC
.
- L. Qin, X. He, L. Chen and Y. Zhang, ACS Appl. Mater. Interfaces, 2015, 7, 5965 CAS
.
- V. Subramanian, E. E. Wolf and P. V. Kamat, J. Am. Chem. Soc., 2004, 126, 4943 CrossRef CAS PubMed
.
- L. L. Chng, N. Erathodiyil and J. Y. Ying, Acc. Chem. Res., 2013, 46, 1825 CrossRef CAS PubMed
.
- Y.-S. Chen and P. V. Kamat, J. Am. Chem. Soc., 2014, 136, 6075 CrossRef CAS PubMed
.
- J.-J. Liu, X.-R. Song, Y.-W. Wang, A.-X. Zheng, G.-N. Chen and H.-H. Yang, Anal. Chim. Acta, 2012, 749, 70 CrossRef CAS PubMed
.
- J. Yin, X. He, K. Wang, F. Xu, J. Shangguan, D. He and H. Shi, Anal. Chem., 2013, 85, 12011 CrossRef CAS PubMed
.
- H.-C. Yeh, J. Sharma, J. J. Han, J. S. Martinez and J. H. Werner, Nano Lett., 2010, 10, 3106 CrossRef CAS PubMed
.
- J. Li, X. Zhong, H. Zhang, X. C. Le and J.-J. Zhu, Anal. Chem., 2012, 84, 5170 CrossRef CAS PubMed
.
- L. Zhang, J. Zhu, S. Guo, T. Li, J. Li and E. Wang, J. Am. Chem. Soc., 2013, 135, 2403 CrossRef CAS PubMed
.
- J. Li, X. Zhong, F. Cheng, J.-R. Zhang, L.-P. Jiang and J.-J. Zhu, Anal. Chem., 2012, 84, 4140 CrossRef CAS PubMed
.
- A. Retnakumari, S. Setua, D. Menon, P. Ravindran, H. Muhammed, T. Pradeep, S. Nair and M. Koyakutty, Nanotechnology, 2010, 21, 055103 CrossRef PubMed
.
- Y. Wang, J. Chen and J. Irudayaraj, ACS Nano, 2011, 5, 9718 CrossRef CAS PubMed
.
- J. Qiao, X. Mu, L. Qi, J. Deng and L. Mao, Chem. Commun., 2013, 49, 8030 RSC
.
- D. An, J. Su, J. K. Weber, X. Gao, R. Zhou and J. Li, J. Am. Chem. Soc., 2015, 137, 8412 CrossRef CAS PubMed
.
- C. Sun, Y. Yuan, Z. Xu, T. Ji, Y. Tian, S. Wu, J. Lei, J. Li, N. Gao and G. Nie, Bioconjugate Chem., 2015, 26, 193 CrossRef CAS PubMed
.
- C. Sun, H. Yang, Y. Yuan, X. Tian, L. Wang, Y. Guo, L. Xu, J. Lei, N. Gao, G. J. Anderson, X.-J. Liang, C. Chen, Y. Zhao and G. Nie, J. Am. Chem. Soc., 2011, 133, 8617 CrossRef CAS PubMed
.
- R. Vankayala, C.-L. Kuo, K. Nuthalapati, C.-S. Chiang and K. C. Hwang, Adv. Funct. Mater., 2015, 25, 5934 CrossRef CAS
.
- C. Zhang, Z. Zhou, Q. Qian, G. Gao, C. Li, L. Feng, Q. Wang and D. Cui, J. Mater. Chem. B, 2013, 1, 5045 RSC
.
- C. Zhou, M. Long, Y. Qin, X. Sun and J. Zheng, Angew. Chem., Int. Ed., 2011, 50, 3168 CrossRef CAS PubMed
.
- L.-S. Lin, X. Yang, Z.-S. Cong, J.-B. Cao, K.-M. Ke, Q.-L. Peng, J. Gao, H.-H. Yang, G. Liu and X. Chen, ACS Nano, 2014, 8, 3876 CrossRef CAS PubMed
.
- X.-R. Song, S.-X. Yu, G.-X. Jin, X. Wang, J. Chen, J. Li, G. Liu and H.-H. Yang, Small, 2016, 12, 1506 CrossRef CAS PubMed
.
- D.-H. Hu, Z.-H. Sheng, P.-F. Zhang, D.-Z. Yang, S.-H. Liu, P. Gong, D.-Y. Gao, S.-T. Fang, Y.-F. Ma and L.-T. Cai, Nanoscale, 2013, 5, 1624 RSC
.
- G. Liang, D. Ye, X. Zhang, F. Dong, H. Chen, S. Zhang, J. Li, X. Shen and J. Kong, J. Mater. Chem. B, 2013, 1, 3545 RSC
.
- C. Wang, Y. Yao and Q. Song, J. Mater. Chem. C, 2015, 3, 5910 RSC
.
- H. Hu, P. Huang, O. J. Weiss, X. Yan, X. Yue, M. G. Zhang, Y. Tang, L. Nie, Y. Ma, G. Niu, K. Wu and X. Chen, Biomaterials, 2014, 35, 9868 CrossRef CAS PubMed
.
- J. Li, J. You, Y. Dai, M. Shi, C. Han and K. Xu, Anal. Chem., 2014, 86, 11306 CrossRef CAS PubMed
.
- F. Gao, P. Cai, W. Yang, J. Xue, L. Gao, R. Liu, Y. Wang, Y. Zhao, X. He, L. Zhao, G. Huang, F. Wu, Y. Zhao, Z. Chai and X. Gao, ACS Nano, 2015, 9, 4976 CrossRef CAS PubMed
.
- K. T. Butterworth, S. J. McMahon, F. J. Currellab and K. M. Prise, Nanoscale, 2012, 4, 4830 RSC
.
- X.-D. Zhang, Z. Luo, J. Chen, S. Song, X. Yuan, X. Shen, H. Wang, Y. Sun, K. Gao, L. Zhang, S. Fan, D. T. Leong, M. Guo and J. Xie, Sci. Rep., 2015, 5, 8669 CrossRef CAS PubMed
.
- X.-D. Zhang, Z. Luo, J. Chen, X. Shen, S. Song, Y. Sun, S. Fan, F. Fan, D. T. Leong and J. Xie, Adv. Mater., 2014, 26, 4565 CrossRef CAS PubMed
.
- X.-D. Zhang, J. Chen, Z. Luo, D. Wu, X. Shen, S.-S. Song, Y.-M. Sun, P.-X. Liu, J. Zhao, S. Huo, S. Fan, F. Fan, X.-J. Liang and J. Xie, Adv. Healthcare Mater., 2014, 3, 133 CrossRef CAS PubMed
.
- L.-S. Lin, Z.-X. Cong, J. Li, K.-M. Ke, S.-S. Guo, H.-H. Yang and G.-N. Chen, J. Mater. Chem. B, 2014, 2, 1031 RSC
.
- M. Sakamoto, T. Tachikawa, M. Fujitsuka and T. Majima, Langmuir, 2009, 25, 13888 CrossRef CAS PubMed
.
- T. Das, P. Ghosh, M. S. Shanavas, A. Maity, S. Mondal and P. Purkayastha, Nanoscale, 2012, 4, 6018 RSC
.
- E. S. Shibu, S. Sugino, K. Ono, H. Saito, A. Nishioka, S. Yamamura, M. Sawada, Y. Nosaka and V. Biju, Angew. Chem., Int. Ed., 2013, 52, 10559 CrossRef CAS PubMed
.
- H. Kawasaki, S. Kumar, G. Li, C. Zeng, D. R. Kauffman, J. Yoshimoto, Y. Iwasaki and R. Jin, Chem. Mater., 2014, 26, 2777 CrossRef CAS
.
- P. Zhang, Y. Chen, Y. Zeng, C. Shen, R. Li, Z. Guo, S. Li, Q. Zheng, C. Chu, Z. Wang, Z. Zheng, R. Tian, S. Ge, X. Zhang, N.-S. Xia, G. Liu and X. Chen, Proc. Natl. Acad. Sci. U. S. A., 2015, 112, 6129 CrossRef PubMed
.
- J. Li, C. Zheng, S. Cansiz, C. Wu, J. Xu, C. Cui, Y. Liu, W. Hou, Y. Wang, I. Teng, H.-H. Yang and W. Tan, J. Am. Chem. Soc., 2015, 137, 1412 CrossRef CAS PubMed
.
- X.-R. Song, X. Wang, S.-X. Yu, J. Cao, S.-H. Li, J. Li, G. Liu, H.-H. Yang and X. Chen, Adv. Mater., 2015, 27, 3285 CrossRef CAS PubMed
.
- T. Chen, S. Xu, T. Zhao, L. Zhu, D. Wei, Y. Li, H. Zhang and C. Zhao, ACS Appl. Mater. Interfaces, 2012, 4, 5766 CAS
.
- Y. Tao, Z. Li, E. Ju, J. Ren and X. Qu, Nanoscale, 2013, 5, 6154 RSC
.
- N. Li, Y. Chen, Y.-M. Zhang, Y. Yang, Y. Su, J.-T. Chen and Y. Liu, Sci. Rep., 2014, 4, 4164 Search PubMed
.
- Y. Tao, E. Ju, Z. Li, J. Ren and X. Qu, Adv. Funct. Mater., 2014, 24, 1004 CrossRef CAS
.
- D. Chen, Z. Luo, N. Li, J. Y. Lee, J. Xie and J. Lu, Adv. Funct. Mater., 2013, 23, 4324 CrossRef CAS
.
- C. Zhang, C. Li, Y. Liu, J. Zhang, C. Bao, S. Liang, Q. Wang, Y. Yang, H. Fu, K. Wang and D. Cui, Adv. Funct. Mater., 2015, 25, 1314 CrossRef CAS
.
- C. Zhang, Z. Zhou, X. Zhi, Y. Ma, K. Wang, Y. Wang, Y. Zhang, H. Fu, W. Jin, F. Pan and D. Cui, Theranostics, 2015, 5, 134 CrossRef PubMed
.
- L. Yang, L. Shang and G. U. Nienhaus, Nanoscale, 2013, 5, 1537 RSC
.
- J.-Y. Zhao, R. Cui, Z.-L. Zhang, M. Zhang, Z.-X. Xie and D.-W. Pang, Nanoscale, 2014, 6, 13126 RSC
.
- L. Dong, M. Li, S. Zhang, J. Li, G. Shen, Y. Tu, J. Zhu and J. Tao, Small, 2015, 11, 2571 CrossRef CAS PubMed
.
- T. D. Fernández, J. R. Pearson, M. P. Leal, M. J. Torres, M. Blanca, C. Mayorga and X. L. Guével, Biomaterials, 2015, 43, 1 CrossRef PubMed
.
- H. S. Choi, W. Liu, P. Misra, E. Tanaka, J. P. Zimmer, B. I. Ipe, M. G. Bawendi and J. V. Frangioni, Nat. Biotechnol., 2007, 25, 1165 CrossRef CAS PubMed
.
- J. Liu, M. Yu, C. Zhou, S. Yang, X. Ning and J. Zheng, J. Am. Chem. Soc., 2013, 135, 4978 CrossRef CAS PubMed
.
- J. Liu, M. Yu, C. Zhou and J. Zheng, Mater. Today, 2013, 16, 477 CrossRef CAS
.
- M. Zhou, J. Li, S. Liang, A. K. Sood, D. Liang and C. Li, ACS Nano, 2015, 9, 7085 CrossRef CAS PubMed
.
- F. Liu, X. He, H. Chen, J. Zhang, H. Zhang and Z. Wang, Nat. Commun., 2015, 6, 8003 CrossRef CAS PubMed
.
- H. Chen, G. D. Wang, W. Tang, T. Todd, Z. Zhen, C. Tsang, K. Hekmatyar, T. Cowger, R. B. Hubbard, W. Zhang, J. Stickney, B. Shen and J. Xie, Adv. Mater., 2014, 26, 6761 CrossRef CAS PubMed
.
- X.-D. Zhang, D. Wu, X. Shen, P.-X. Liu, F.-Y. Fan and S.-J. Fan, Biomaterials, 2012, 33, 4628 CrossRef CAS PubMed
.
- X.-D. Zhang, Z. Luo, J. Chen, H. Wang, S.-S. Song, X. Shen, W. Long, Y.-M. Sun, S. Fan, K. Zheng, D. T. Leong and J. Xie, Small, 2015, 11, 1683 CrossRef CAS PubMed
.
- O. A. Wong, R. J. Hansen, T. W. Ni, C. L. Heinecke, W. S. Compel, D. L. Gustafson and C. J. Ackerson, Nanoscale, 2013, 5, 10525 RSC
.
- X.-D. Zhang, J. Yang, S.-S. Song, W. Long, J. Chen, X. Shen, H. Wang, Y.-M. Sun, P.-X. Liu and S. Fan, Int. J. Nanomed., 2014, 9, 2069 CrossRef PubMed
.
- J. Liu, M. Yu, X. Ning, C. Zhou, S. Yang and J. Zheng, Angew. Chem., Int. Ed., 2013, 52, 12572 CrossRef CAS PubMed
.
- W. Wei, Y. Lu, W. Chen and S. Chen, J. Am. Chem. Soc., 2011, 133, 2060 CrossRef CAS PubMed
.
- S. Wang, X. Meng, A. Das, T. Li, Y. Song, T. Cao, X. Zhu, M. Zhu and R. Jin, Angew. Chem., Int. Ed., 2014, 53, 2376 CrossRef CAS PubMed
.
- S. Choi, R. M. Dickson and J. Yu, Chem. Soc. Rev., 2012, 41, 1867 RSC
.
|
This journal is © The Royal Society of Chemistry 2016 |
Click here to see how this site uses Cookies. View our privacy policy here.