DOI:
10.1039/C5RA02935J
(Paper)
RSC Adv., 2015,
5, 24655-24660
A new anionic metal–organic framework showing tunable emission by lanthanide(III) doping and highly selective CO2 adsorption properties†
Received
15th February 2015
, Accepted 27th February 2015
First published on 27th February 2015
Abstract
A new three-dimensional porous metal–organic framework, {[NH2(CH3)2][Zn(atz)(ox)]·H2O}n (1) (Hatz = 5-amino-1H-tetrazole, H2ox = oxalic acid), has been solvothermally synthesized and characterized by elemental analysis, IR, TG, and single-crystal X-ray diffraction analysis. Complex 1 exhibits an anionic framework that can be simplified as a rarely reported 4-connected lon network. Complex 1 could be utilized as a platform for emission tuning by doping different lanthanide ions. In addition, gas adsorption studies reveal that 1 has a high isosteric heat of CO2 and good adsorption selectivity for CO2 over CH4 and N2.
Introduction
Due to their potential applications in gas storage and separation,1,2 catalysis,3 luminescence,4 proton conductivity5 and drug delivery,6 metal–organic frameworks (MOFs) as a unique class of porous material have become a hot research field in recent years. Among the numerous MOFs with diverse structures and components, the ones with cation guests have been focused on since they are good platforms for targeted cation exchange or inclusion purposes, which has been developed as an efficient method for the properties modulation of materials. Very recently, we have reported a dynamic MOF with a cationic state, which show enhanced adsorption properties due to the separated positive and negative charges.7
On the other hand, in virtue of the unique features of long excited-state luminescence lifetime,8 sharp and intense luminescence,9 lanthanide (Ln) based luminescent MOFs (LnMOFs) have been widely investigated as light-emitting material. However, the targeted design and construction of luminescence LnMOFs with desired structures and properties is still a great challenge because of the flexible coordination behavior of the Ln ions. Also, though they reveal similar dimensions and coordination behaviors, different Ln3+ cations may result in MOFs with distinct structures and properties, which makes it relatively difficult to combine different Ln3+ cations in one MOF for the tuning of emission.
Recently, the encapsulation of lanthanide cations in the as-synthesized anionic MOFs has attracted great attention since the introduction of specific Ln ions as well as the tuning of the emission could be achieved more readily with this method. Some examples can be highlighted, for example: Rosi and coworkers have investigated the encapsulation of various Ln3+ cations into bio-MOF-1 for fabricating guest-induced luminescent materials;10 Su and coworkers have reported a fluorescence sensor by encapsulating Ln3+ cations in an anionic porous framework IMFC-3;11 Dong and coworkers have also reported the ion-exchange of [Mn(H2O)6]2+ cations by [Ln(H2O)8]3+ cations in anionic Ln-based luminescent materials.12 However, though many examples of emission tuning with lanthanide cations as guests have been reported, it should be noted that the encapsulation of two or more kinds of cations in one complex for desired emissions is quite rare. Also, as the Ln3+ cations included in the MOF may show stronger interactions with the gas molecules adsorbed, the gas sorption properties of Ln3+ encapsulated MOF is worth to be investigated for the purpose of the improvement of gas sorption performances of MOFs.
In this contribution, we report a three dimensional (3D) Zn(II)–MOF, {[NH2(CH3)2][Zn(atz)(ox)]·H2O}n (1) (Hatz = 5-amino-1H-tetrazole, H2ox = oxalic acid). Complex 1 reveals an anionic porous framework with disordered [NH2(CH3)2]+ cations scattering in its channels, which provides the favorable condition for encapsulation of metal cations via exchange process. As we expected, 1 can serve as both a host for encapsulation and an “antenna” for the sensitizing of Ln3+ cations. Emission of characteristic red and green emissions could be achieved by introducing Eu3+ or Tb3+ cations respectively, and these cations could be introduced simultaneously to result in yellow emission. Beside the tunable emission properties, this MOF also shows high CO2 isosteric heat of adsorption, which might be attributed to the enhanced interactions originated from the N atom decorated pore surface and the charged framework.13 Also, it was found that the CO2 isosteric heat of adsorption could be further increased after the introduction of Eu3+ cations.
Experimental
Materials and methods
The following chemical reagents in this work were acquired from commercial sources and used without subsequent purification: zinc nitrate hexahydrate (>99.0%), 5-amino-1H-tetrazole (98%), oxalic acid dihydrate (>99.5%), terbium(III) nitrate hexahydrate (99.99%), europium(III) nitrate hexahydrate (99.99%), DMF (>99.5%), ethanol (99.9%). Infrared spectra were obtained on a TENSOR 27 OPUS (Bruker) FT-IR spectrometer by using KBr pellets in the 4000–400 cm−1 range. Powder X-ray-diffraction (PXRD) patterns were collected on a Rigaku D/Max-2500 diffractometer at 40 kV, 100 mA with a Cu-target tube and a graphite monochromator at room temperature. Thermogravimetric analyses (TGA) were performed on a Rigaku standard TG-DTA analyzer with a heating rate of 10 °C min−1, with an empty Al2O3 crucible used as a reference in the range of 25 °C–700 °C. Elemental analyses (C, H and N) were performed on a Perkin–Elmer analyzer. All fluorescence measurements were performed on a Hitachi F-4500 fluorescence spectrophotometer equipped with a plotter unit. Inductively coupled plasma atomic emission spectra (ICP-AES) analysis was performed on ICP-9000 (N + M) simultaneous ICP atomic emission spectrometers. Gas adsorption studies were performed on an ASAP 2020 surface area and porous analyzer.
Synthesis of {[NH2(CH3)2][Zn(atz) (ox)]·H2O}n (1)
Zinc nitrate hexahydrate (80 mg, 0.27 mmol), 5-amino-1H-tetrazole (49 mg, 0.58 mmol) and oxalic acid dihydrate (37.8 mg, 0.30 mmol) were added in the mixed solvent of 5 mL DMF and 2.5 mL distilled water. The mixture was stirred thoroughly for 30 min at ambient temperature, and then placed in a stainless steel teflon-lined reaction vessel (25 mL). After heated at 120 °C for 3 days, colorless block crystals of 1 were obtained with ca. 86% yield based on Zn(NO3)2·6H2O. FT-IR (KBr pellets, cm−1): 3446 s, 3347 s, 2796 w, 2487 w, 2361 w, 1659 s, 1532 s, 1459 s, 1365 s, 1317 s, 1151 w, 1104 w, 1020 w, 794 s, 591 w, 494 w, 464 w, 418 w. Anal. Calcd. for C5H12N6O5Zn
:
C, 19.91; H, 4.01; N, 27.86. Found: C, 20.49; H, 3.49; N, 27.38.
Synthesis of Tb3+@1
The dried 1 (40 mg) was soaked in an ethanol solution of TbCl3 (0.05 mol L−1) for six hours, and the collected crystals were rinsed by ethanol for several times, then dried in air to give Tb3+@1.
Synthesis of Eu3+@1
The procedure is similar to the synthesis of Tb3+@1 except that TbCl3 (0.05 mol L−1) solution was replaced by EuCl3 (0.05 mol L−1) solution.
Synthesis of Tb3+/Eu3+@1
The dried Tb3+@1 was socked in an ethanol solution of EuCl3 (0.05 mol L−1) for another two hours. After rinsed by ethanol for several times, the sample was dried in air.
X-ray crystallography
The single crystal X-ray data of 1 was collected on a Rigaku SCX-mini diffractometer at 293(2) K with Mo-Kα radiation (λ = 0.71073 Å) by ω scan mode. The structure was solved using the SHELXS-97 program and refined anisotropically by a full-matrix least-squares technique based on F2 using SHELXL-97.14 All non-hydrogen atoms on the framework were located successfully from Fourier maps. Crystallographic crystal data and structure processing parameters were given in Table 1, and the selected bond length and angle data are given in Table S1.† It should be noted that the guest molecules in the channels of 1 are highly disordered and could not be modeled properly, so the diffused electron densities resulting from them were removed by the SQUEEZE routine in PLATON15 and the results were appended in the CIF file. The asymmetric unit of complex 1 consists of one Zn2+, one ox2− and one atz−, which indicates that 1 is an anionic framework, and cations should exist in the channel to keep the charge balance. Combined with the charge balance considerations, volume/count electrons analysis, TG analyses result and elemental analysis result, the cations were assigned to [NH2(CH3)2]+ generated from the decomposition of solvent DMF. The decomposition of DMF in the high temperature solvothermal reaction with H2O appearance is a common phenomenon, which has been widely reported.16 Then the formula for 1 is given in the present form.†
Table 1 Crystallographic Data for 1
|
1 |
Formula |
C5H12N6O5Zn |
Mr (g mol−1) |
301.60 |
Space group |
Pnma |
Crystal system |
Orthorhombic |
a (Å) |
17.621(3) |
b (Å) |
15.958(3) |
c (Å) |
8.7728(18) |
V (Å3) |
2466.9(8) |
Z |
8 |
Dc (g cm−3) |
1.279 |
μ (mm−1) |
1.986 |
Rint |
0.0862 |
Reflns. Collected |
24208 |
Indep. reflns. |
2927 |
GOF on F2 |
1.093 |
R1, wR2 [I > 2σ(I)] |
0.0581, 0.1416 |
R1, wR2 [all data] |
0.0923, 0.1560 |
Results and discussion
Syntheses of MOF
Targeted at the construction of functional porous MOFs, Hatz and H2ox were selected as ligands for the construction of MOFs in this work. Hatz is selected based on its N rich nature, which could facilitate the host–guest interaction of the MOFs.17 On the other hand, H2ox was selected based on the various coordination configuration of ox2− and its strong interactions with metal centers, which may produce robust MOFs with unique structures. We expected that the combination of these two ligands could result in MOFs with increased CO2 sorption performances. After many attempts, complex 1 was obtained with solvothermal method. As we expected, the resulted MOF is highly porous though the pore volume of 1 is partly occupied by [NH2(CH3)2]+ as counter ions. It should be noted that the presence of water in the reaction system could be a critical factor for the formation of complex 1 since no crystalline product could be obtained without water. This might be attributed to the absence of [NH2(CH3)2]+ based on the restrained hydrolysis of DMF in low water content conditions.
Crystal structure description
Single-crystal X-ray diffraction analysis reveals that complex 1 crystallizes in orthorhombic space group Pnma. Complex 1 is a 3D anionic porous MOF. As shown in Fig. 1a, each Zn(II) ion with distorted octahedral geometry is coordinated to four O atoms from two different ox2− and two N atoms from two distinct atz− ligands. The Zn–N lengths are 2.101 Å and 2.103 Å, and the Zn–O lengths ranges from 2.094 Å to 2.208 Å. All these bonds distance are well consistent with those previously reported Zn(II) complexes.18 Each carboxylate group of ox2− ligand chelates to one Zn(II) ion to give a bidentate coordination mode, while the atz− ligand binds to two Zn(II) ions in a μ2-1,4 bridging coordination mode. It should be noted that there is one excess negative charge per Zn(II) center in the framework according to the component. Then the charge balance of the complex is achieved by the presence of protonated [NH2(CH3)2]+ cations, generated from the decomposition of solvent DMF, in the channels, which has been proved by the results of TG analyses and elemental analysis.
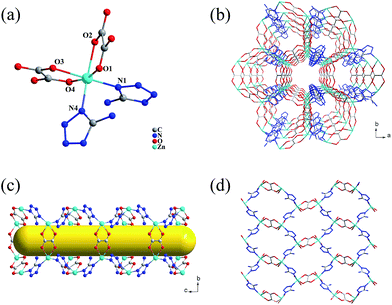 |
| Fig. 1 The structure of 1: (a) The coordination environment of the Zn(II) atom (symmetry code: #1: −x, −y + 1/2, z; #2: −x, −y, −z + 2; #3: −x + 1/2, −y, z − 1/2; #4: −x + 1/2, −y, z + 1/2); (b) The 3D framework of complex 1; (c) the 1D channel along c-axis. (d) The shape of the pore along b-axis. | |
On the basis of the above mentioned connection modes, a 3D network with open channels in a, b, and c direction is formed (Fig. 1b). The channels along the c-axis are cylindroid, and the opening is defined by the ring formed with four ox2− and two atz− ligands (Fig. 1c and S3†). Notably, all the amino groups of atz− ligands point to the center of the channel (Fig. 1c and S3†), which is conducive to the interaction between the framework and guest molecules/ions in the channels. On the other hand, the shape of the pores along b-axis is distorted tetragon, and the aperture is defined by the ring formed by four atz− and two ox2− ligands (Fig. 1d). The framework is high porosity, and the solvent accessible volume of 1 without the guest molecules is 51.0%, as calculated by PLATON.
From topological point of view, each Zn(II) ion can be considered as a tetrahedral 4-connected node, while ox2− and atz− as straight linkers. Thus, the overall network of 1 can be simplified as a lonsdaleite (lon) topology with the long Schläfli symbol of {62·62·62·62·62} (Fig. 2). This topology is similar to the well-known diamond (dia) topology. However, the lon topology has both chair and boat conformations six-membered rings, which is clearly different from the classical 4-connected dia net that only has rings with chair conformation. Compared with the common dia net, the lon net is rarely reported in the MOFs.19
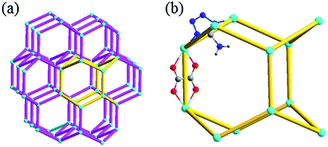 |
| Fig. 2 (a) Lonsdaleite network with a 65 cage unit highlighted in yellow. (b) The basic unit of lonsdaleite topology. | |
Thermal property
In order to determine the thermal stability and verify the formula of the complex, thermogravimetric analysis (TGA) of complex 1 was carried out (Fig. S2†). In the case of TGA, the result profile reveals a weight loss of 4.9% from 50 to 190 °C, which corresponding to the weight of free H2O molecules (calculated: 5.8%), and then a weight loss of 15.8% in the temperature of 170–280 °C, in agreement with the weight of countered [NH2(CH3)2]+ (calculated: 15.3%). And then the framework structure decomposed gradually in the temperature range of 280–600 °C.
Encapsulation of lanthanide(III) ions
Since anionic MOFs could be suitable platform for the fabrication of Ln based light-emitting material by encapsulation of Ln cations, we investigated the potential of 1 as a host to encapsulate Ln3+ cations for preparing tunable luminescence material.
The emission spectrum of complex 1 was investigated. As shown in Fig. 3a, 1 displays an emission band with the maximum at 474 nm upon excitation at 280 nm. This emission could be mainly attributed to the emission of atz− ligand based on its high similarity to that of the free Hatz ligand (Fig. S8†).
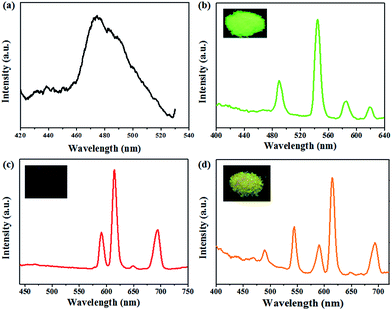 |
| Fig. 3 (a) Solid-state emission spectra of 1 (λex = 280 nm). (b) Tb3+@1 (λex = 340 nm). (c) Eu3+@1 (λex = 395 nm). (d) Tb3+/Eu3+@1 (λex = 380 nm). The emissions of samples illuminated under 254 nm UV light are inserted. | |
To load the Ln3+ cations into the channels of complex 1, the as synthesized samples of 1 were soaked in ethanol solution of LnCl3 for ion exchange, and Eu3+ and Tb3+ were selected for their characterized red and green emissions in visible light region. After the exchange process, the sample reveals corresponding colors of the metal ions loaded, indicating the success of cations exchange. The PXRD patterns of the as-synthesized 1, Tb3+@1, Eu3+@1 and Tb3+/Eu3+@1 samples are in good agreement with each other (Fig. S6†), indicating the stability of the framework of these samples and the crystalline integrity upon the lanthanide adsorption. Furthermore, ICP-AES measurements were performed to quantify the exchange performances. The results indicate that the doped amount of Tb3+ cations is 8.07% and 5.72% for Eu3+ (mol% compared with Zn2+ cations). The differences in the doped amount might be due to the different dimensions of the Ln3+ ions, which affect their diffusion rates in the exchange process.
The emission properties of the Ln3+ doped MOFs were also investigated. For Tb3+@1, four typical emission spectrums of Tb3+ cations are detected in the visible region upon excitation at 340 nm (Fig. 3b), corresponding to emission transitions of 5D4 → 7F6 (490 nm), 5D4 → 7F5 (544 nm), 5D4 → 7F4 (585 nm), and 5D4 → 7F3 (619 nm), respectively. The most prominent emission is observed at 544 nm. Under UV lamp emitting at 254 nm, the sample exhibits bright green emission, which is the feature color of Tb3+ cations. In the case of Eu3+@1, emission bands at 591 nm, 614 nm, 649 nm and 694 nm are observed upon excitation at 395 nm (Fig. 3c), which are in accord with the characteristic emission of Eu3+ cations arising from the 5D0 → 7FJ (J = 1, 2, 3, 4) transitions, respectively. Among these transitions, 5D0 → 7F2 (614 nm) is the strongest, which corresponds to red color when observed under UV light.
To further confirm the possibility of emission tuning by the combination of different Ln3+ anions, Tb3+/Eu3+@1 was prepared and investigated. The ICP-AES measurement indicates that the doped amount of Tb3+ and Eu3+ cations are 5.49% and 6.64% (mol% compared with Zn2+ cations), respectively. Solid-state emission spectra of the Tb3+/Eu3+@1 exhibits emission peaks of 469 nm, 490 nm, 544 nm, 590 nm, 615 nm, 648 nm and 694 nm (Fig. 3d), which correspond to the characteristic emissions of both Eu3+ and Tb3+. Based on the combination of the emissions, Tb3+/Eu3+@1 reveals bright yellow emission under the radiation of UV lamp, indicating the success of emission tuning.
Gas adsorption properties
The presence of free amino groups and uncoordinated nitrogen decorated channels suggest that 1 might be a potential candidate for selective CO2 capture.20 To proof our hypothesis, gas sorption studies were performed (see ESI for detail†). The CO2 adsorption isotherm of 1 at 195 K reveals a reversible type-I characteristic of microporous nature (Fig. S9†). The BET and Langmuir surface areas were calculated to be 125 and 165 m2 g−1, respectively.
The adsorption isotherms of CO2, CH4 and N2 for 1 were measured up to 900 mm Hg at 273 K. (Fig. 4) The CO2 uptake value was 32.3 cm3 g−1 at 273 K/1 atm, while the adsorption amount of CH4 and N2 were only 4.6 and 2.3 cm3 g−1 under the same condition. The adsorption capacity of CO2 for complex 1 is 7 and 13.9 times higher than CH4 and N2 at 273 K/1 atm, respectively. These results demonstrate that complex 1 is a potential material for selective separation of CO2/CH4 and CO2/N2 at standard condition. Besides, the ratios of the initial slopes were also used to estimate the adsorption selectivity at low pressure. The calculated low pressure CO2/CH4 selectivity was 40
:
1 and CO2/N2 selectivity was 92
:
1 at 273 K (1 atm). The CO2/CH4 selectivity of complex 1 is even better than the famous CAU-1 (ref. 21) with the selectivity of 28
:
1, and the CO2/N2 selectivity is just slightly smaller than the selectivity of 101
:
1 for CAU-1 at 273 K (1 atm).
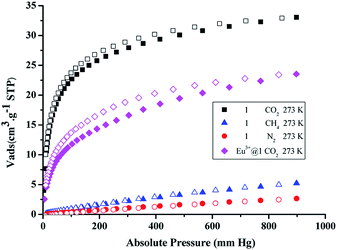 |
| Fig. 4 Gas adsorption isotherms of CO2, CH4 and N2 for complex 1 and CO2 for Eu3+@1 at 273 K (adsorption and desorption branches are shown with closed and open symbols, respectively). | |
To further estimate the interaction between the adsorbent and adsorbate, the adsorption isothermal of CO2 under 298 K was measured to calculate the isosteric heat of adsorption (Qst) for 1 (Fig. S16†). The adsorption heat of CO2 was calculated by the Clausisus–Clapeyron equation. Complex 1 exhibits the Qst value of 45.4 kJ mol−1 at initial loading, highlighting 1 has a strong binding affinity for CO2. The Qst value of 1 is much higher than many well-known anionic frameworks, such as the 28.2 kJ mol−1 in CPF-13 (ref. 22) and 25.38 kJ mol−1 in [(CH3)2NH2]3[(Cu4Cl)3(btc)8]·9DMA.23 It is also higher than that of many other famous azole-based MOFs, such as 30.7 kJ mol−1 in IFMC1 (ref. 24) and 40.8 kJ mol−1 in Zn2(C2O4) (C2N4H3)2·(H2O)0.5.25 The Qst is quite comparable to those famous complexes with open metal sites, such as 47 kJ mol−1 in Mg/DOBDC26 and 44 kJ mol−1 in MIL101.27 The high CO2 adsorption heat and excellent selectivity for CO2 over N2 and CH4 properties of complex 1 could be ascribe to the following reasons: (1) the interaction between the localized dipole of the exposed N-containing groups (–NH2 groups and uncoordinated N atoms in the atz− ligands) and the quadrupole moment of CO2 would induce the dispersion and electrostatic forces to enhance CO2 adsorption and separation abilities on MOFs;28 (2) the strong interactions of the CO2 molecules with the charged anionic framework.
In order to investigate the effect of the presence of Ln3+ on the adsorption properties, gas adsorption isotherms of Eu3+@1 were also measured. As shown in Fig. 4, the CO2 uptake reduce to 22.4 cm3 g−1 at 273 K/1 atm, about 10 cm3 g−1 less than the original adsorption amount of complex 1. At 298 K, the CO2 adsorption also decreases to 14.7 cm3 g−1 (Fig. S17†). The adsorption capacities of Eu3+@1 for CH4 and N2 are 4.0 and 2.4 cm3 g−1 at 273 K/1 atm (Fig. S17†), respectively, which has almost no change from that of complex 1. According to the conventional calculated method of initial slopes of adsorption isotherms, the selective separation ration of CO2/CH4 is 28
:
1, while the CO2/N2 is 37
:
1. Compare with that of complex 1, the adsorption capacity of Eu3+@1 has a significant decrease (Table 2), which might be attribute to the increased framework density since the hydrated Eu3+ ion introduced has increased formula weight compared with that of the corresponding [NH2(CH3)2]+ exchanged. However, in the case of Eu3+@1, the CO2 Qst value is increased (Fig. S20†). This phenomenon should be attributed to the presence of hydrated Eu3+ ion in the pore structure which further enhanced the interactions between CO2 and framework by increased regional polarity originated from the aqua molecules.
Table 2 Gas uptake of 1 and Eu3+@1 at 273 K and 1 atm
Sample |
Vads (cm3 g−1) |
Selectivity (273 K) |
CO2 |
CH4 |
N2 |
CO2/CH4 |
CO2/N2 |
1 |
32.3 |
4.7 |
2.3 |
40 : 1 |
92 : 1 |
Eu3+@1 |
22.4 |
4.0 |
2.4 |
28 : 1 |
37 : 1 |
Conclusions
We have synthesized and characterized a 3D porous anionic MOF, which represents a rare lon topology. Utilizing the anionic nature of this MOF, Eu3+ and Tb3+ cations could be doped into the framework to obtain red and green luminescent MOFs, respectively. Furthermore, these cations could be doped simultaneously to get a luminescent MOF with yellow light emission. Gas adsorption measurements revealed that complex 1 has a high CO2 adsorption heat and good adsorption selectivity for CO2 over CH4 and N2. All these results indicate that this MOF can be a potential multifunctional material for light emission and selective gas sorption applications.
Acknowledgements
This work was financially supported by the 973 program of China (2014CB845600), NNSF of China (21290171, 21202088, and 21421001), and MOE Innovation Team of China (IRT13022).
Notes and references
-
(a) S. S. Kaye, A. Dailly, O. M. Yaghi and J. R. Long, J. Am. Chem. Soc., 2007, 129, 14176 CrossRef CAS PubMed;
(b) S. Q. Ma, D. F. Sun, J. M. Simmons, C. D. Collier, D. Q. Yuan and H.-C. Zhou, J. Am. Chem. Soc., 2008, 130, 1012 CrossRef CAS PubMed;
(c) J. L. C. Rowsell and O. M. Yaghi, Angew. Chem., Int. Ed., 2005, 44, 4670 CrossRef CAS PubMed;
(d) M. P. Suh, H. J. Park, T. K. Prasad and D.-W. Lim, Chem. Rev., 2012, 112, 782 CrossRef CAS PubMed.
-
(a) P. Ju, L. Jiang and T.-B. Lu, Chem. Commun., 2013, 49, 1820 RSC;
(b) T. Borjigin, F. X. Sun, J. L. Zhang, K. Cai, H. Ren and G. S. Zhu, Chem. Commun., 2012, 48, 7613 RSC;
(c) K. Sumida, D. L. Rogow, J. A. Mason, T. M. McDonald, E. D. Bloch, Z. R. Herm, T.-H. Bae and J. R. Long, Chem. Rev., 2012, 112, 724 CrossRef CAS PubMed.
-
(a) Y. Zhao, K. Chen, J. Fan, T.-A. Okamura, Y. Lu, L. Luo and W.-Y. Sun, Dalton Trans., 2014, 43, 2252 RSC;
(b) C.-D. Wu, A. G. Hu, L. Zhang and W. B. Lin, J. Am. Chem. Soc., 2005, 127, 8940 CrossRef CAS PubMed;
(c) C.-D. Wu and W. B. Lin, Angew. Chem., Int. Ed., 2007, 46, 1075 CrossRef CAS PubMed.
-
(a) J.-H. Wang, M. Li and D. Li, Chem. Sci., 2013, 4, 1793 RSC;
(b) Y. Chen, H.-X. Li, D. Liu, L.-L. Liu, N.-Y. Li, H.-Y. Ye, Y. Zhong and J.-P. Lang, Cryst. Growth Des., 2008, 8, 3810 CrossRef CAS;
(c) B. L. Chen, L. B. Wang, Y. Q. Xiao, F. R. Fronczek, M. Xue, Y. J. Cui and G. D. Qian, Angew. Chem., Int. Ed., 2009, 48, 500 CrossRef CAS PubMed;
(d) Y.-N. Gong, L. Jiang and T.-B. Lu, Chem. Commun., 2013, 49, 11113 RSC.
-
(a) N. C. Jeong, B. Samanta, C. Y. Lee, O. K. Farha and J. T. Hupp, J. Am. Chem. Soc., 2012, 134, 51 CrossRef CAS PubMed;
(b) R. M. P. Colodrero, K. E. Papathanasiou, N. Stavgianoudaki, P. Olivera-Pastor, E. R. Losilla, M. A. G. Aranda, L. Léon-Reina, J. Sanz, I. Sobrados, D. Choquesillo-Lazarte, J. M. García-Ruiz, P. Atienzar, F. Rey, K. D. Demadis and A. Cabeza, Chem. Mater., 2012, 24, 3780 CrossRef CAS.
-
(a) W. J. Rieter, K. M. Pott, K. M. L. Taylor and W. B. Lin, J. Am. Chem. Soc., 2008, 130, 11584 CrossRef CAS PubMed;
(b) C.-Y. Sun, C. Qin, C.-G. Wang, Z.-M. Su, S. Wang, X.-L. Wang, G.-S. Yang, K.-Z. Shao, Y.-Q. Lan and E.-B. Wang, Adv. Mater., 2011, 23, 5629 CrossRef CAS PubMed;
(c) J. D. Rocca, D. M. Liu and W. B. Lin, Acc. Chem. Res., 2011, 44, 957 CrossRef PubMed.
- Q. Chen, Z. Chang, W. C. Song, H. Song, H. B. Song, T. L. Hu and X. H. Bu, Angew. Chem., Int. Ed., 2013, 52, 11550 CrossRef CAS PubMed.
- S. Chen, R. Q. Fan, C. F. Sun, P. Wang, Y.-L. Yang, Q. Su and Y. Mu, Cryst. Growth Des., 2012, 12, 1337 CAS.
- T.-F. Liu, W. J. Zhang, W.-H. Sun and R. Cao, Inorg. Chem., 2011, 50, 5242 CrossRef CAS PubMed.
- J. An, C. M. Shade, D. A. Chengelis-Czegan, S. Petoud and N. L. Rosi, J. Am. Chem. Soc., 2011, 133, 1220 CrossRef CAS PubMed.
- J.-S. Qin, S.-J. Bao, P. Li, W. Xie, D. Y. Du, Z. Liang, Y.-Q. Lan and Z.-M. Su, Chem.–Asian J., 2014, 9, 749 CrossRef CAS PubMed.
- P. Wang, J.-P. Ma, Y.-B. Dong and R.-Q. Hang, J. Am. Chem. Soc., 2007, 129, 10620 CrossRef CAS PubMed.
-
(a) P. Pachfule, Y.F. Chen, J.W. Jiang and R. Banerjee, J. Mater. Chem., 2011, 21, 17737 RSC;
(b) M.-H. Choi, H. J. Park, D. H. Hong and M. P. Suh, Chem.–Eur. J., 2013, 19, 17432 CrossRef CAS PubMed.
-
(a) G. M. Sheldrick, SHELXS-97, 1997;
(b) G. M. Sheldrick, Acta Crystallogr., Sect. A: Found. Crystallogr., 2008, 64, 112 CrossRef CAS PubMed.
- A. L. Spek, Acta Crystallogr., Sect. A: Found. Crystallogr., 1990, 46, C34 Search PubMed.
-
(a) Y.-W. Li, J.-R. Li, L.-F. Wang, B.-Y. Zhou, Q. Chen and X.-H. Bu, J. Mater. Chem. A, 2013, 1, 495 RSC;
(b) H. Jeong and M. P. Suh, Chem. Sci., 2013, 4, 685 RSC.
-
(a) Q. P. Lin, T. Wu, S.-T. Zheng, X. H. Bu and P. Y. Feng, J. Am. Chem. Soc., 2012, 134, 784 CrossRef CAS PubMed;
(b) P. Serra-Crespo, E. V. Ramos-Fernandez, J. Gascon and F. Kapteijn, Chem. Mater., 2011, 23, 2565 CrossRef CAS.
-
(a) Q. Chu, G.-X. Liu, Y.-Q. Huang, X.-F. Wang and W.-Y. Sun, Dalton Trans., 2007, 4302 RSC;
(b) H. M. He, F. X. Sun, H. M. Su, J. T. Jia, Q. Li and G. S. Zhu, CrystEngComm, 2014, 16, 339 RSC;
(c) Q. F. Zhang, A. J. Geng, H. N. Zhang, F. L. Hu, Z.-H. Lu, D. Z. Sun, X. L. Wei and C. L. Ma, Chem.–Eur. J., 2014, 20, 4885 CrossRef CAS PubMed.
-
(a) H. Kim, Y. Y. Sun, Y. Kim, T. Kajiwara, M. Yamashita and K. Kim, CrystEngComm, 2011, 13, 2197 RSC;
(b) N. W. Ockwig, O. Delgado-Friedrichs, M. O'Keeffe and O. M. Yaghi, Acc. Chem. Res., 2005, 38, 176 CrossRef CAS PubMed;
(c) F. Wang, D.-C. Hou, H. Yang, Y. Kang and J. Zhang, Dalton Trans., 2014, 43, 3210 RSC.
- H.-R. Fu, F. Wang and J. Zhang, Dalton Trans., 2014, 43, 4668 RSC.
- X. L. Si, C. L. Jiao, F. Li, J. Zhang, S. Wang, S. Liu, Z. B. Li, L. X. Sun, F. Xu, Z. Gabelica and C. Schick, Energy Environ. Sci., 2011, 4, 4522 CAS.
- Q. G. Zhai, Q. P. Lin, T. Wu, L. Wang, S. T. Zheng, X. H. Bu and P. Y. Feng, Chem. Mater., 2012, 24, 2624 CrossRef CAS.
- Y. X. Tan, Y. P. He and J. Zhang, Chem. Commun., 2011, 47, 10647 RSC.
- J. S. Qin, D. Y. Du, W. L. Li, J. P. Zhang, S. L. Li, Z. M. Su, X. L. Wang, Q. Xu, K. Z. Shao and Y. Q. Lan, Chem. Sci., 2012, 3, 2114 RSC.
- R. Vaidhyanathan, S. S. Iremonger, K. W. Dawson and G. K. H. Shimizu, Chem. Commun., 2009, 5230 RSC.
- S. R. Caskey, A. G. Wong-Foy and A. J. Matzger, J. Am. Chem. Soc., 2008, 130, 10870 CrossRef CAS PubMed.
- P. L. Llewellyn, S. Bourrelly, C. Serre, A. Vimont, M. Daturi, L. Hamon, G. De Weireld, J.-S. Chang, D.-Y. Hong, Y. K. Hwang, S. H. Jhung and G. Férey, Langmuir, 2008, 24, 7245 CrossRef CAS PubMed.
- Z. J. Zhang, Y. G. Zhao, Q. H. Gong, Z. Li and J. Li, Chem. Commun., 2013, 49, 653 RSC.
Footnote |
† Electronic supplementary information (ESI) available: CCDC 1008358. For ESI and crystallographic data in CIF or other electronic format see DOI: 10.1039/c5ra02935j |
|
This journal is © The Royal Society of Chemistry 2015 |
Click here to see how this site uses Cookies. View our privacy policy here.