What are the effects of environmental factors on Co speciation at the magnetite surface?†
Received
20th December 2023
, Accepted 18th March 2024
First published on 22nd March 2024
Abstract
Magnetite nanoparticles are abundant in the environment and are notably used for environmental applications due to their unique magnetic, adsorption and redox properties. The stoichiometry of magnetite (Fe(II)/Fe(III)) is dependent on environmental factors (pH, presence of organic ligands, redox conditions), which largely affects not only magnetite nanoparticles' physicochemical properties, such as redox reaction, but also adsorption of contaminants. However, the joint effects of environmental factors and magnetite stoichiometry on magnetite–metal cation interaction are elusive. This study focuses on Co as an important contaminant and because Co-doped magnetite nanoparticles are of high interest for nanotechnology applications. Magnetite nanoparticles (∼10 nm) with different stoichiometries were synthesized by coprecipitation and partially oxidized using H2O2 to obtain the desired stoichiometry. Batch studies were carried out with different [Co], using three different stoichiometries (0.1, 0.3 and 0.5) at different pH values and in the presence or the absence of natural organic matter (OM) or atmospheric O2(g). Experimental and modeling results and XAS and XMCD analyses revealed that interactions between Co and magnetite varied, with oligomer formation and surface precipitation favored at higher stoichiometries. It was also highlighted that the formation of different species of Co depended on environmental conditions with the adsorption of monomers favored at low pH and in the presence of organic matter, and the partial oxidation of Co(II) to Co(III) under aerobic conditions. These results will help to predict the behavior and fate of Co in the environment and to understand the impact of environmental factors for an appropriate use of magnetite nanoparticles for environmental applications, or to synthesize Co-modified magnetite nanoparticles using water as solvent for high technology applications.
Environmental significance
Magnetite nanoparticles are increasingly used and studied for the remediation of contaminated environments, due to their high affinity for metals, magnetic properties and natural presence in the environment. However, environmental factors such as pH, presence of natural organic molecules, and redox conditions have an impact on the stoichiometry of magnetite, and determine the adsorption of contaminants such as cobalt. Adsorption experiments and X-ray adsorption spectroscopy coupled with X-ray magnetic circular dichroism allowed us to determine the amount and the speciation of Co bound to magnetite as a function of various environmental factors. The results of this work could help improve remediation using magnetite nanoparticles and better predict Co behavior in natural systems.
|
1. Introduction
Iron (oxyhydr)oxide nanoparticles are ubiquitous and largely known to exert a strong control on the transport, speciation, (bio)availability, and biotoxicity of many contaminants in the environment though adsorption processes.1–3 Contaminants interaction with iron (oxyhydr)oxide surfaces highly depends on physicochemical factors such as pH, redox potential, ionic strength or the presence of organic coatings that can considerably vary between different natural systems. Interaction mechanisms between contaminants and iron (oxyhydr)oxides and the effects of environmental factors on the extent of contaminants bound to the surface have been relatively well documented. This allowed, for instance, the development of mechanistic surface complexation models that account for the impact of surface charging (related notably to surface protonation and background electrolyte concentration) to predict the chemical and electrostatic contribution to the ion surface complexation,1,4 to account for polymer and surface precipitate formation,5,6 the adsorption of Fe2+ and associated redox effects,7,8 the formation of surface–metal–ligand ternary complexes with small organic or inorganic ligands9,10 as well as humic-like substances.11–13 Although these surface speciation models are powerful tools to predict the behavior and fate of contaminants in natural systems, further work is required to account for the impact of important adsorbing phases such as spinel iron oxide nanoparticles, including magnetite.
Spinel iron oxide nanoparticles (Fe3−δO4) are mixed compounds of Fe(II) and Fe(III), which occur naturally in the environment. Their structure is inverse with Fe(II) in the octahedral sites and Fe(III) in the octahedral and tetrahedral sites.14–16 The full stoichiometric structure, which characterizes magnetite, is defined by an Fe(II)-to-Fe(III) ratio (R) equal to 0.5 (or δ = 0).17 Non-stoichiometric magnetite shows R values between 0 and 0.5 (or 0 ≤ δ ≤ 1/3). When the R value tends to 0 (δ → 1/3), the magnetite structure is close to that of maghemite (Fe2O3), with the presence of iron vacancies in the octahedral sites and the absence of Fe(II).17 The stoichiometry is dependent on various environmental factors such as pH,18 redox potential (e.g. presence or absence of oxidants such as O2 or H2O2 to reduce stoichiometry, or Fe2+ to restore stoichiometry),17,19 microbial activity,20 or organic ligands.21,22 Thus, magnetites with different stoichiometries can exist in subsurface environments or in temporarily flooded soils, where conditions influence Fe valence.23
Besides the substantial changes in the magnetite structure imposed by the environmental conditions and related changes in its electronic and magnetic properties,3,24 magnetite stoichiometry also drastically affects its reactivity toward contaminants. The redox properties make magnetite very interesting for the reduction or oxidation of redox-sensitive chemical compounds at the magnetite surface in industrial and environmental processes19,25–30 or in medical applications.31,32 Therefore, much effort has been made to characterize the impact of the stoichiometry on the thermodynamics and kinetics of the reduction reactions of inorganic and organic compounds, such as reduction of U(VI) to U(IV)26 or reduction of nitrobenzene to aniline,19 or on its capacity to promote advanced oxidation processes through Fenton-like reactions.25,33 However, the impact of magnetite stoichiometry on surface complexation, polymerization and precipitation of metal ions has been largely overlooked. For instance, the adsorption of several ligands (nalidixic acid, salicylate, and humic acid) was shown to increase with magnetite stoichiometry.34 A similar observation was made by comparing the adsorption of Co2+ onto pristine and fully oxidized magnetite (i.e. maghemite).35 However, available surface complexation models consider only pure magnetite or maghemite surfaces,36,37 so the impact of magnetite stoichiometry on contaminant adsorption is not accurately predicted. In contrast with other iron (oxyhydr)oxides, the development of such a model for magnetite is more challenging due to the dynamic behavior of the magnetite–maghemite solid solution. Further work is required to decouple the direct effects of environmental factors from the indirect ones, occurring through a modification of magnetite stoichiometry, on the surface speciation of metal ions such as Co2+. First, this would allow better prediction of the impact of magnetite on their environmental behavior and fate, particularly in Fe-rich subsurface environments or temporarily flooded soils (e.g., wetlands experiencing redox potential fluctuation). Second, as magnetite nanoparticles are widely used as adsorbents in water treatment due to various advantages (e.g. high adsorption capacity, easy magnetic separation from water, reusability, low toxicity and biocompatibility),38–42 a better prediction of magnetite adsorption capacity towards contaminants like Co might help to optimize its use in various physicochemical conditions.
Predicting the surface speciation of Co is also interesting for the synthesis of nanomaterials for high technology applications. Indeed, surface-bound Co may improve the magnetic properties of magnetite nanoparticles by decreasing their magnetic anisotropy at ambient temperature while keeping a small size, which is of great interest for magneto-optic devices,43,44 electronic devices,45 data storage,46–48 high-density recording49 or even for replacing the rare earth metals contained in certain permanent magnets.46,50 A previous study evidenced the role of Co surface loading on Co speciation on stoichiometric magnetite,51 which drastically affected the magnetic behavior of the nanoparticle. Surface-complexed or incorporated Co2+ had a ferrimagnetic behavior at low loadings, magnetically silent small Co polymers formed at intermediate loadings and precipitation of an antiferromagnetic Co(OH)2(s)-like phase onto the magnetite surface occurred for the highest Co concentrations. Therefore, understanding the effects of physicochemical parameters might allow one to better constrain the properties of Co-modified magnetite nanoparticles.
The present study investigated the sorption of Co(II) on magnetite in aqueous suspensions with the aim of unraveling the impacts of magnetite stoichiometry and important environmental factors (pH, organic ligands and oxidation) on the sorption and surface speciation of Co. Following a previous study on stoichiometric magnetite, adsorption isotherm experiments and modeling were performed and compared for non-stoichiometric magnetite, at pH 8 and under an inert atmosphere, to avoid Fe2+ oxidation or H+ promoted dissolution.17,18 The effects of pH and humic acid concentration on both magnetite stoichiometry and Co(II) adsorption were investigated under an inert atmosphere, starting from a fully stoichiometric magnetite. Finally, the effect of O2 on the redox speciation of Co at the surface of oxidized magnetite (quasi-maghemite) was also probed. All samples were characterized by X-ray absorption spectroscopy (XAS) and magnetic circular dichroism (XMCD) at Fe and Co L2,3 edges. This allows determination of the oxidation state distribution of both elements (including magnetite stoichiometry),17,18 their occurrence in octahedral or tetrahedral sites in spinel structure or at the surface of magnetite, and the surface speciation of Co, such as monomer, oligomer and Co(OH)2-like phase formation.51 The results provide important data for predicting Co speciation in the presence of magnetite in aqueous systems depending on environmental factors.
2. Materials and methods
2.1. Chemicals
All chemicals used were purchased from Sigma-Aldrich and were of analytical grade or better. Sample solutions were prepared with Milli-Q ultrapure water (specific resistivity 18.2 MΩ cm). All the experiments, except the oxidation experiment, were performed in an anaerobic chamber (N2 glovebox, Jacomex, O2(g) <1 ppm) and all the solutions were purged with N2(g) for at least 12 h inside the glovebox before use. The pH of all the samples was adjusted by HCl and NaOH (no buffer was used). For TEM measurements, hexadecyltrimethylammonium bromide (CTAB) was used as a surfactant. The Co hydroxide reference used was synthesized in a previous study.51 The Co3O4 reference was purchased from Sigma-Aldrich. Natural organic matter (OM) was purchased from the International Humic Substances Society (IHSS) and corresponded to Leonardite humic acid (1S104H).
2.2. Synthesis of nanomagnetites with various stoichiometries
All syntheses were performed in an N2 glovebox at room temperature, following a well-known co-precipitation method of iron salts.17,52,53 The full stoichiometric magnetite (R = Fe(II)/Fe(III) = 0.5) was synthesized with the dissolution of FeCl2 and FeCl3 in HCl solution, which was then added to NaOH solution leading to the instantaneous precipitation of ∼10 nm magnetite nanoparticles. For non-stoichiometric nanoparticles (R = 0.1 and R = 0.3), known amounts of H2O2 were added to R0.5. The synthesized solutions were washed with ultrapure water (at pH ∼8.5 to avoid the release of Fe2+). The stoichiometry of the synthesized nanoparticles was verified by spectrophotometric determination of dissolved [Fe(II)] and total [Fe] (i.e., [Fe(III)] + [Fe(II)]) by the 1,10-phenanthroline colorimetric method.54,55
2.3. Sorption experiments
Aqueous suspensions of Fe3−δO4 nanoparticles (R0.1, R0.3, and R0.5) were prepared, for a total Fe concentration of 6.5 mM (∼0.5 g L−1 magnetite), in 15 mL tubes, with 10 mM NaCl. Isotherm experiments were performed at pH 8 and varying total Co concentrations ([Co]tot) from 0.01 to 3 mM (using a 100 mM CoCl2 stock solution) for R0.1 and R0.3. Data for R0.5 were already obtained in a previous study.51 The effects of pH were investigated using the three stoichiometries for [Co]tot = 0.4 mM, and pH was adjusted between 3 and 9. To understand the impact of OM, it was added to stoichiometric magnetite with concentrations ranging from 0 to 50 mg L−1. Co was then added at a concentration of 0.04 mM and pH was adjusted to 8. The concentrations of OM adsorbed on magnetites were measured by dissolved organic carbon (DOC) analysis after sample filtration using a Shimadzu TOC-L carbon analyzer. To test the effect of oxidation on the Co adsorption process, a sample was prepared from R0.1 with [Co]tot = 3 mM, and the pH was adjusted to 8, under aerobic conditions. N2(g) bubbling was used to limit carbonate dissolution. After 7 days of equilibrium, a magnet was used to collect the solid fraction of all the samples. The supernatant was filtered at 0.2 μm using cellulose PES filters (Sartorius Minisart). Cobalt concentrations were determined using a UV-vis spectrophotometric method51 adapted from Zahir and Keshtkar (1998).56 It is based on a complexation of Co2+ with 1-nitroso-2-naphthol-3,6-disulfonic acid disodium salt hydrate (nitroso-R salt). The absorbance was recorded at 520 nm using a Shimadzu UV2600 spectrophotometer. A cobalt calibration curve was determined with concentrations ranging from 5 to 100 μM for the quantification of aqueous Co concentration in the filtered solutions ([Co]aq). For the samples with very small [Co]aq (i.e. <5 μM), a quadrupole ICP-MS (Agilent Technologies 7700X) was used. Before Co quantification, calibration curves were recorded and validated using certified material references (SLRS-6, National Research Council). A rhodium solution was used as an internal standard to correct the instrumental drift and potential matrix effects. The limit of Co quantification was determined at 18 nM (1.04 ppt) (AFNOR Certification).57 The total amount of Co associated with the solid phase was expressed in terms of Co surface density onto Fe3−δO4 ([Co]s, in atoms per nm2) and calculated as follows: |  | (1) |
where [Co]tot and [Co]aq are given in mol L−1, V is the sample volume (L), m is the magnetite mass (g), SSA is the surface specific area (m2 g−1) and NA is the Avogadro constant (mol−1). On the basis of repetition of some experiments, uncertainty associated with Co adsorption was 5%.
A cobalt ferrite suspension was prepared by following the magnetite synthesis method (see section 2.2), replacing 10% of the Fe(II) (FeCl2·4H2O) with Co(II) (CoCl2·6H2O), in a N2 glovebox at room temperature. NaOH was added until the pH was stable at 8.5 and the solution was kept as is.
2.4. Characterization by TEM
Different nanoparticle samples were characterized by transmission electron microscopy (HR-TEM; Jeol JEM 2100 HR microscope). A small volume of sample was taken (Co-magnetite, Co-OM-magnetite), which was then diluted with ultrapure water. All samples were sonicated for 15 min. A droplet of the diluted suspension was deposited on a holey carbon film 300 mesh copper grid and dried inside an anaerobic chamber, except for the oxidized sample, which was made under aerobic conditions. Samples were transported to the microscope in hermetically sealed glass bottles, preserving them under a N2 atmosphere. The average particle diameter of the pristine magnetite was found to be 11.5 ± 1.5 nm for R0.5, 10.6 ± 2.6 nm for R0.3 and 9.6 ± 2.6 nm for R0.1 by measuring 100 particles.17,18
2.5. XAS and XMCD analyses
Solid samples were analyzed by XAS and XMCD, covering the soft X-ray range and probing the L2,3 range (2p → 3d) absorption edges of Fe and Co transition metals. XAS and XMCD analyses, which are chemically selective and sensitive to valence states, helped to better understand the contribution of Co and Fe cations to the structure and magnetic behavior of nanoparticles. XAS and XMCD spectra were recorded on the DEIMOS beamline at synchrotron SOLEIL.58 Measurements were carried out on dried nanoparticles by dropping colloidal suspensions on silicon plates which were dried at room temperature in an Ar glovebox (Jacomex O2(g) <1 ppm) connected to the DEIMOS station. The silicon plates were fixed to a copper sample holder, which was then introduced into the superconducting magnet of the DEIMOS end station. All the spectra were collected in total electron yield (TEY) at 4.2 K and under UHV conditions (10−10 mbar). XAS spectra were recorded by reversing the full circular polarization of the X-rays to the right (σR) or left (σL), and by varying the external magnetic field (H = +6 or −6 T). Isotropic XAS spectra were plotted as (σ+ + σ−)/2, while the XMCD results were plotted as (σ+ − σ−), where σ+ = [σL(H−) + σR(H+)]/2 and σ− = [σL(H+) + σR(H−)]/2. For all the Co spectra, the background of XAS and XMCD at the Co L2,3 edges were corrected by subtracting from the raw signal the XAS and XMCD results of the corresponding pure magnetite (R0.1, R0.3 or R0.5, without Co) sample measured at Co edges. Then, XAS and XMCD were normalized by dividing the raw signal by the edge jump of the isotropic XAS. By measuring XMCD intensity as a function of external magnetic fields (from +6 to −6 T), XMCD magnetization curves at specific sites on the Co L3 edge were plotted. At DEIMOS beamline, fully circularly polarized X-rays were provided by an Apple-II HU52 undulator for XAS and XMCD, while EMPHU65 with a polarization flipping rate of 10 Hz was used to record the magnetization curves. The beam size was 800 μm × 800 μm and the photon energy resolution was 100 meV.
The different peaks obtained by Fe XMCD at the L3 edge were used to estimate the stoichiometry of the samples. The evolution of peak intensities were be monitored using the S indicator:17,59
|  | (2) |
The S
1 peak is attributed to Fe(
II) and Fe(
III) in octahedral sites, although Fe(
II) dominates. The S
2 peak corresponds to Fe(
III) in tetrahedral sites, while the S
3 peak corresponds to the contribution of Fe(
III) in octahedral sites.
17 The S indicator was then used to calculate the effective stoichiometry according to the method described by Jungcharoen
et al. (2021).
17
3. Results and discussion
3.1. Effect of magnetite stoichiometry on Co adsorption
Co adsorption as a function of their concentrations was studied on three different stoichiometries: R0.1, R0.3 and R0.5. TEM images are shown in Fig. S2† for the two extreme Co concentrations ([Co]s = 0.5, 18.9 and 25.7 atoms per nm2, respectively) for R0.1 and R0.5. The Co adsorption isotherms measured for the three stoichiometries, at pH 8, are shown in Fig. 1 using linear scales and in Fig. S3† using log scales that allow visualization of Co sorption behavior at trace levels, relevant to natural systems (data in Table S1†). Data for R0.5 were taken from our previous study.51 The evolution of the data as a function of Co concentration reveals three steps with (i) a large amount of Co adsorbed at low concentration, (ii) a change of slope with more Co in solution at intermediate concentration and (iii) a linear adsorption at high Co concentration. At low Co surface loadings (<0.1 nm−2), adsorption is similar and strong for all three stoichiometries because Co either forms monomeric surface complexes or incorporates into the Fe3−δO4 surface structure.35,51 As Co loading increases, adsorption on R0.1 shows a plateau at around 4 nm−2, compared with 10 nm−2 for R0.3 and R0.5. A previous study dedicated to Co adsorption on magnetite at pH 8, but under oxidizing conditions, observed an adsorption plateau between 1.25 and 3.45 atoms per nm2, depending on the surface area of the magnetite.60 As the surface of these magnetites was very likely oxidized under an ambient atmosphere, the adsorption data compare well with the present ones obtained for R0.1.60 At such loadings, Co was shown to form small polymers on R0.5.51 This implies that in contrast to monomeric Co species, surface polynuclear Co species formation is affected by the stoichiometry of magnetite. A similar observation was made with an organic ligand (nalidixic acid), for which the adsorption increased from 0% to 100% at pH 8 when the stoichiometry increased.34 Above the plateau, as the Co concentration in solution increases, the amount of Co in the solid phase rises non-linearly for [Co]aq >0.2 mM for R0.3 and R0.5, and [Co]aq >0.6 mM for R0.1. This Co behavior can be attributed to Co surface precipitation. The fact that the onset of Co surface precipitation is found at higher [Co]aq suggests that the surface of R0.1 serves less efficiently as a template for Co (hydr)oxide precipitation than for higher stoichiometry magnetites. This observation is consistent with the smaller capacity of R0.1 to promote the formation of polynuclear Co species, compared to R0.3 and R0.5, because the latter species might be regarded as a first step before further polymerization process.
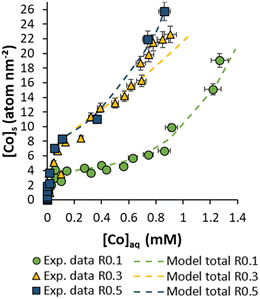 |
| Fig. 1 Cobalt adsorption isotherms on R0.1 and R0.3 (6.5 mM Fe) at pH 8 in 10 mM NaCl. Symbols represent the experimental data (“Exp. data”). The dotted lines correspond to the model results, which is a combination of two Langmuir and one Freundlich models. Results are compared with data obtained for R0.5, taken from Fablet et al. (2023).51 Analytical error bars of 5% are plotted, although not visible for some data and at logarithmic scale. Error was set to 50% for data with [Co]aq < 0.001 mM on the logarithmic scale (i.e. ±0.2 log unit)61 to acknowledge the fact that larger error may be encountered at low concentrations. | |
The experimental data are compared with model results obtained by the combination of the two Langmuir and Freundlich adsorption isotherm equations, with the following equations, as described in a previous study and applied to R0.5:51
| [Co]s = QL,1 + QL,2 + QF | (3) |
where
QL,i (
i = 1 or 2) and
QF are magnetite-bound Co amounts.
Eqn (4) describes
QL,i:
| 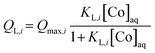 | (4) |
where
Qmax,i is the adsorption capacity (here, in atoms per nm
2) and
KL,i is the Langmuir constant (in L mmol
−1).
Eqn (3) describes
QF:
where
KF is the Freundlich constant, and
n is the non-ideality parameter.
Results of the fit are shown in Fig. 1 for [Co]s, and the contributions of the three considered mechanisms on the overall adsorption are shown in Fig. S3.† The parameters of eqn (3)–(5) for each stoichiometry are shown in Table 1. Preliminary tests revealed that KL1 and KL2 values were similar for all stoichiometries and were subsequently constrained equal in order to follow only the evolution of Qmax,i, KF and n. Although the first Langmuir equation seems negligible at a linear scale, it appears crucial to predict Co behavior at naturally relevant trace levels, which are visualized using log scales (Fig. S3†). The first adsorption mechanism, at low Co concentrations where surface complexation of Co monomers is expected to be the prevalent mechanism, is similar for the three different stoichiometries, with Qmax,1 = 0.52 atoms per nm2 and log
KL1 = 4.18. The second mechanism differs for R0.1, R0.3 and R0.5, with Qmax,L2 values equal to 4.16, 9.48 and 10.51 atoms per nm2, respectively. Finally, the third mechanism, which used the Freundlich equation to account for surface precipitation (as it can be seen as a multi-layer formation)62–64 also differs between the three stoichiometries, with n–KF couples leading to an onset of Co(OH)2 precipitation at [Co]aq = 0.4 mM for R0.1, and approximately 0.1 mM for R0.3 and R0.5. This difference in Co behavior could be explained by the fact that R0.1 magnetite, which has a structure close to that of maghemite, has less Fe on its surface replaced by vacancies, which might limit Co oligomer and precipitate formation on its surface. As already pointed out,51 the present model is simple and cannot be considered as a predictive model of Co interaction with magnetite because it does not include electrostatic contribution to the surface Co–magnetite binding, for instance. However, the model is used as a semi-quantitative guide for interpreting isotherm and spectroscopic data by accounting for three distinct surface species.
Table 1 Optimized parameters of eqn (3) for Langmuir models (L1 and L2) and Freundlich model (F), for R0.1, R0.3 and R0.5. Qmax is the adsorption capacity (atoms per nm2)
Stoichiometry |
Parameters |
L1 |
L2 |
F |
R0.1 |
n
|
|
|
3.27 |
log K |
4.18 |
1.39 |
0.34 |
Q
max
|
0.52 |
4.16 |
|
R0.3 |
n
|
|
|
1.43 |
log K |
4.18 |
1.39 |
0 |
Q
max
|
0.52 |
9.48 |
|
R0.5 |
n
|
|
|
2.41 |
log K |
4.18 |
1.39 |
0.25 |
Q
max
|
0.52 |
10.51 |
|
The speciation of Fe3−δO4-bound Co as a function of its stoichiometry was investigated at several Co concentrations by XAS and XMCD analysis. All isotropic XAS and XMCD spectra at the Co L3 edge are presented in Fig. S4.† Selected results obtained for the three stoichiometries at three different [Co]s for which each surface species determined by the surface speciation model prevail are shown in Fig. 2 ([Co]tot = 0.04, 0.2 and 3 mM, corresponding to [Co]s = 0.5, 2–2.3 and 18.9–25.7 atoms per nm2, respectively). The XAS spectra show four contributions at approximately 772.1 eV (peak A), 773.4 eV (peak B), 773.8 eV (peak C), and 774.7 eV (peak D), which are multiplet peaks characteristic of the presence of Co2+ (Fig. 2a–c).65 All XAS spectra were normalized to the C peak in order to study the evolution of the other peaks. The XAS spectra of the samples show few differences at low (Fig. 2a) and medium (Fig. 2b) Co concentrations, which are similar to the reference spectrum of CoFe2O4 (Fig. S1†) or that of adsorbed Co organic complexes to magnetite.66,67 Large A and D peaks at high Co concentrations (Fig. 2c), as observed for Co(OH)2 (data taken from Fablet et al. (2023)),51 point to the formation of a Co(OH)2-like phase on the surface of all the magnetites. However, slightly more intense A and D peaks observed for R0.1 might suggest a larger extent of Co(OH)2 formation. XMCD spectra provide complementary information to XAS spectra and exhibit two peaks, E (773.5 eV) and F (773.9 eV) (Fig. 2d and e). For low and intermediate Co concentrations (e.g. [Co]tot = 0.04 and 0.2 mM, Fig. 2d and e), the XMCD spectra of all stoichiometries resemble either the CoFe2O4 reference spectrum or the spectrum of adsorbed Co complexes to magnetite.46,66,67 At high Co concentrations (e.g. [Co]tot = 3 mM in Fig. 2f), the XMCD spectra of the samples resemble that of Co(OH)2, which is characterized by a smaller peak E (as probed by the E/F peak ratio in Fig. S5†) that is slightly shifted to lower energy. Hence, XMCD results also confirm surface precipitation at high [Co]tot for all magnetites.
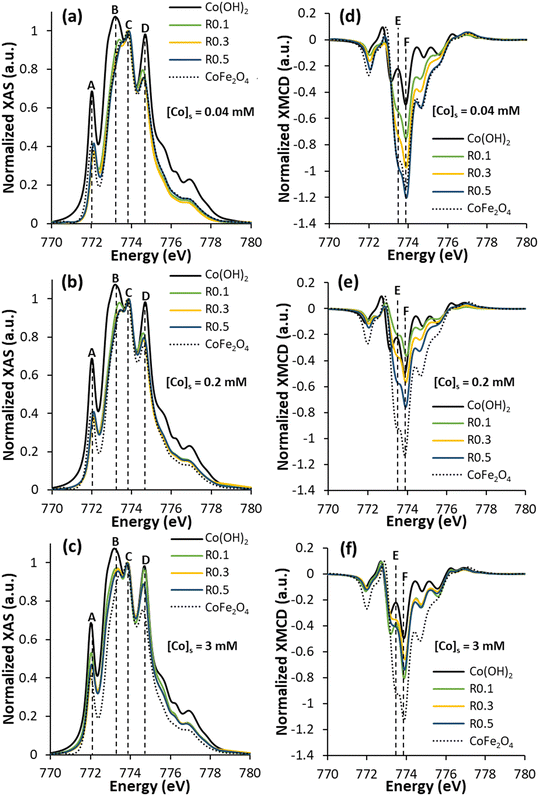 |
| Fig. 2 Normalized XAS spectra at the Co L3 edge at (a) [Co]tot = 0.04 mM, (b) [Co]tot = 0.2 mM, and (c) [Co]tot = 3 mM, and normalized XMCD spectra at the Co L3 edge at (d) [Co]tot = 0.04 mM, (e) [Co]tot = 0.2 mM, and (f) [Co]tot = 3 mM, for the three magnetite stoichiometries: R0.1 in green, R0.3 in yellow, and R0.5 in blue (data for R0.5 from Fablet et al. (2023)).51 The dotted line corresponds to the XAS and XMCD of CoFe2O4 and the solid line to Co(OH)2 references. XAS signals are normalized by dividing the raw signal by the maximum XAS peak, and XMCD signals are normalized by dividing the raw signal by the maximum XAS peak. | |
Important variations in the intensity of the XMCD signal are observed depending on R and [Co]tot. The largest signal is observed for R0.5 at low [Co]tot (Fig. 2d), and was attributed to Co adsorption in the form of a monomer, whose structure is close to that of a ferrimagnetic CoFe2O4 structure.51 At low and intermediate [Co] (Fig. 2d and e), that is, in the absence of Co(OH)2 precipitation, the XMCD signal decreases with decreasing R, which is probably due to smaller magnetic Fe–Co coupling for non-stoichiometric than for stoichiometric magnetites. In contrast, the XMCD signal is only little affected by R for high [Co]tot (Fig. 2f), which confirms that Co(OH)2 prevails at the surface of all stoichiometries. As previously observed for R0.5, XAS and XMCD spectra at the Fe L2,3 edge show no significant effect of Co on Fe solid speciation (Fig. S6†).17
The XMCD signal intensity is plotted against [Co]tot for each stoichiometry in Fig. 3 and is compared with the predicted surface speciation of Co calculated with the isotherm model (eqn (1)). For all three magnetite stoichiometries, the decrease in the XMCD signal seems to be linked to the decrease in the first species (i.e. Co monomers) and the formation of the second species (i.e. Co oligomers). Moreover, the XMCD signal increases when the models predict Co(OH)2 formation. These results confirm previous observation that XMCD can be used to probe Co speciation at the surface of magnetite.51 These data were also compared with the results of linear combination fit (LCF) on normalized Co L3 edge XAS spectra for each stoichiometry (from 770 to 780 eV) to determine the percentage of Co(OH)2versus the Co surface loading, used with the CoFe2O4 and Co(OH)2 references (Fig. S7, Table S2†).51 The results obtained agree qualitatively with those of surface modeling on the increase in the amount of Co(OH)2. This is because linear combinations of XAS do not allow differentiation of the second species, Co oligomers, as shown for R0.5 magnetite.51
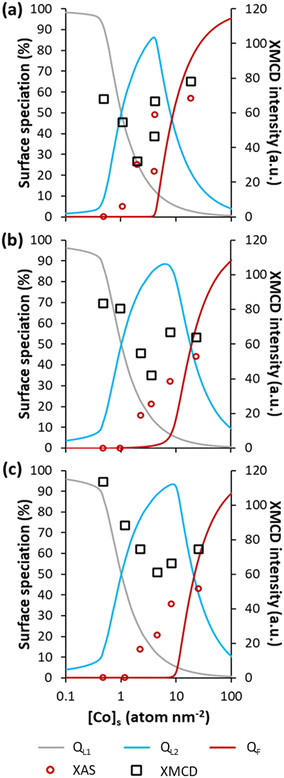 |
| Fig. 3 Percentage of the different species identified using the adsorption equation (eqn (1)) versus Co surface loading in log scale (in atoms per nm2) compared to the percentage of the Co(OH)2(s)-like phase calculated from LCF of XAS data (red circles) and, on the secondary axis, the XMCD intensity (black squares) for (a) R0.1, (b) R0.3 and (c) R0.5 (data for R0.5 from Fablet et al. (2023)).51 | |
The XMCD magnetization curves versus the magnetic field at the Co2+(Oh) site for R0.1, R0.3 and R0.5 with [Co]tot = 0.8 mM are shown in Fig. 4. The magnetic properties depend on the valence states of the elements and the alignment of cation moments in the crystal structure. Thus, the high-field saturation provides confirmation on the sorption behavior of Co on the surface of the various stoichiometries. For R0.5 the magnetization curve tends to saturation at high magnetic field which is in great agreement with a ferrimagnetic structure similar to CoFe2O4. However, with decreasing stoichiometry the magnetization curves tend to non-saturation at high field. In particular, for R0.1 the magnetization curve shows no saturation, similarly to that of Co(OH)2, which exhibits an antiferromagnetic structure. This is due to a larger proportion of Co(OH)2 on R0.1 compared to R0.3 and R0.5, which limits saturation.
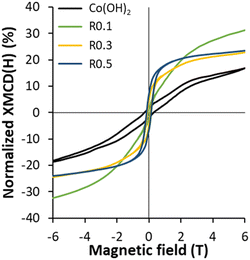 |
| Fig. 4 Normalized XMCD magnetization in percentage versus magnetic field measurement at the Co L3 edge at 4.2 K for [Co]s = 4.16, 7.88 and 8.30 atoms per nm2 for R0.1, R0.3 and R0.5, respectively (corresponding to [Co]tot = 0.8 mM), and for Co(OH)2. Spectra are plotted as 2 × (CR − CL)/(CR + CL), where CR is the spectrum of circular polarization (right) and CL the spectrum of circular polarization (left). | |
3.2. Effect of pH on Co adsorption onto magnetites
To obtain insights into the stability of Co oligomers versus monomers, the effect of pH on Co adsorption was investigated for intermediate [Co]tot (0.4 mM, where Co oligomers occur at pH 8), over a pH range from 3 to 10 for R0.1 and R0.5 (Fig. 5a). For the two stoichiometries, the effect of pH on Co adsorption is similar, with almost no adsorption at pH values below 5, followed by an increase in adsorbed amount up to a complete Co removal from the solution at pH 8. However, Co adsorption was slightly higher at 6.5 ≤ pH ≤ 8 onto R0.5 than onto R0.1. XAS and XMCD spectra at the Fe and Co L2,3 edges were recorded for R0.5 at pH = 6.5, the pH at which [Co]s = 2 atoms per nm2 (∼40% adsorbed). According to the Fe data (Fig. S8†), no significant change in magnetite stoichiometry was observed between pH 6.5 and 8 (Reff = 0.48 and 0.53 ± 0.05, respectively).17 The XAS spectra of Co show no clear differences between the two pH values (Fig. S9†). However, the XMCD spectrum (Fig. 5b) recorded at pH 6.5 shows an intense F peak and exhibits similar features to CoFe2O4, in contrast to the less intense signal, and a spectrum that resembles Co(OH)2 at pH 8. This difference is due to the smaller amount of Co adsorbed on the magnetite surface at pH 6.5 than at pH 8, which favors the formation of surface Co monomeric species. In addition, oligomer formation and surface precipitation reactions of metal ions onto Fe-(hydro)oxides generally involve a larger stoichiometric number of OH− and, hence, occur only at relatively high pH values, in contrast to monomeric surface complexation that can occur at comparatively lower pH values.11 Finally, because (i) magnetite surface charge evolves with pH,36,37 and (ii) surface Co oligomers and monomers might not carry the same charge, electrostatic effects can be expected to play a combined role with pH on the Co surface speciation.
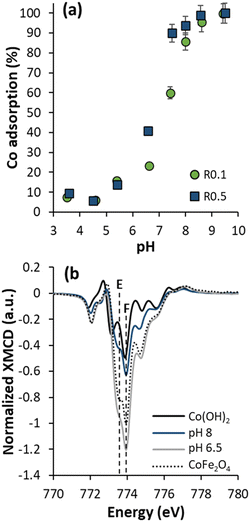 |
| Fig. 5 (a) Effect of pH on the percentage of adsorbed Co for two stoichiometries of magnetite: R0.1 (green circles) and R0.5 (blue squares) for [Co]tot = 0.4 mM, and (b) corresponding normalized XMCD spectra at the Co L3 edge at pH 6.5 (gray line) and 8 (blue line) for stoichiometric magnetite (R0.5). The dotted line corresponds to the XMCD of CoFe2O4 and the solid line to Co(OH)2 references. XMCD signals are normalized by dividing the raw signal by the maximum XAS peak. | |
3.3. Organic matter (OM) effects on Co adsorption on stoichiometric magnetite (R0.5)
The effects of OM on Co surface complexation onto stoichiometric magnetite was studied for low [Co]tot (0.04 mM, where Co monomers prevail), with OM concentrations ranging from 2 to 50 mg L−1, at pH 8. Magnetite and OM were pre-equilibrated before the addition of Co in order to maximize the effects of OM on Co surface speciation. Fig. 6a compares the percentage of adsorbed Co and the OM concentration in the solid phase in mg C per g versus the total OM concentration. The amount of adsorbed OM increases with total concentration, with over 90% of OM adsorbed for [OM] ≤10 mg L−1, but with a low slope, suggesting surface site saturation at high [OM], with only 59% of OM adsorbed for [OM] = 50 mg L−1. The percentage of Co adsorbed is only little affected by OM for [OM] ≤25 mg L−1, as it remains between 95% and 90%. This can be due either to the strong interaction between Co and magnetite or ternary complexes (FeO–Co–L or Fe–L–Co) often formed in the presence of different ligands (L), including natural organic matter.11,13,66,67 For [OM] = 50 mg L−1, Co adsorption decreases to 46%.68,69 XAS and XMCD analyses at the Fe L2,3 edge (Fig. S11a and c†) revealed that OM dissolves magnetite by preferential adsorption with Fe(II), inducing a more oxidized surface of the magnetite. However, because magnetite stoichiometry (R) decreases from 0.48 to 0.42 for 2 ≤ [OM] ≤ 25 mg L−1 and decreases to 0.32 when [OM] = 50 mg L−1, this alone cannot explain the decrease in Co adsorption, but instead this can be attributed to solution complexation between Co and OM.70,71 It is interesting to note that while TEM images for [OM] = 2 mg L−1 show no significant effect of OM on magnetite nanoparticle aggregation, for [OM] = 50 mg L−1 very small Fe nanoparticles (around 2 nm-sized) appear. These small nanoparticles might arise from the released Fe(II) under the influence of OM and subsequent oxidation to Fe(III). Fe(III) nanoparticles formed from Fe(II)–OM complexes are known to exhibit very small sizes.72,73
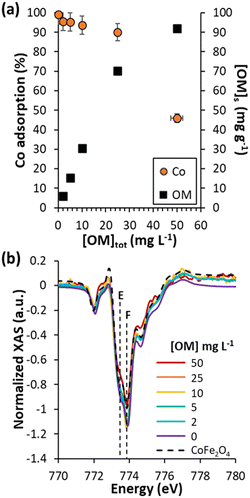 |
| Fig. 6 (a) Adsorption of organic matter (OM) in mg g−1 Fe3O4 (black squares) and percentage of adsorbed Co (orange circles) and (b) XMCD at the Co L3 edge on stoichiometric magnetite (R0.5) (6.5 mM Fe), at pH 8, for [OM]tot = 2 to 50 mg L−1 and [Co]tot = 0.04 mM. XMCD of Co signals are normalized by dividing the raw signal by the maximum XAS peak. | |
The XAS (Fig. S11b†) and XMCD (Fig. 6b) spectra of Co are similar for all [OM], except for [OM] = 50 mg L−1, which has a slightly less intense XMCD signal. It is recalled that the intensity of the XMCD signal cannot be related to the smaller amount of adsorbed Co at high [OM] (see, e.g.Fig. 4 and related discussion). Instead this might be due to the binding of Co at the outer shell of a thick layer of OM, which can only weakly interact magnetically with the particle, as previously observed when the particle was covered with a layer of an organic molecule.74
3.4. Oxidation effects on Co adsorption on R0.1 magnetite
Redox conditions, especially the occurrence of O2(g), might also have an impact on Co interaction with magnetite. Indeed, TEM images (Fig. S12†) reveal that R0.1 equilibrated with [Co]tot = 3 mM under aerobic conditions at pH 8 is highly aggregated, with the formation of some leaflet structures. No evolution of magnetite stoichiometry was observed from Fe XAS and XMCD spectra because the already oxidized magnetite R0.1 was used. Adsorption measurements showed that under aerobic conditions, 7.1 atoms per nm2 were associated with magnetite, compared with 19.9 atoms per nm2 under anaerobic conditions, which points to a different Co speciation in the absence or presence of O2. The corresponding Co XAS spectrum (Fig. 7a) shows a small peak A and a very intense peak at 775.3 eV (denoted G). It shares common characteristics with the XAS spectrum of Co3O4, a mixed compound of Co(II) and Co(III), which has no peak A and a very intense peak G, characteristic of Co(III). Corresponding XMCD spectra are shown in Fig. 7b. Co3O4 has no signal, being an antiferromagnetic material. A XMCD signal is measured for the sample under aerobic conditions, although weaker than that of the sample stored under anaerobic conditions, which evidences the co-occurrence of Co(II) magnetically coupled to magnetite. Furthermore, the XMCD spectrum of the oxidized sample is similar to the spectrum of CoFe2O4, in contrast to that of the sample prepared in the absence of O2, which has a shape similar to that of Co(OH)2. Previous studies have shown that Co(II) rapidly oxidized to Co(III) at room temperature, forming a Co3O4 phase on the particle surface.70,71 Total oxidation of Co to Co3O4 can be limited by the presence of CoO on the outermost layers.70,75 XAS and XMCD results suggest that under aerobic conditions, partial oxidation of Co(II) to Co(III) limits Co(OH)2 precipitation in favor of Co(II) monomers and a Co3O4-like precipitate.
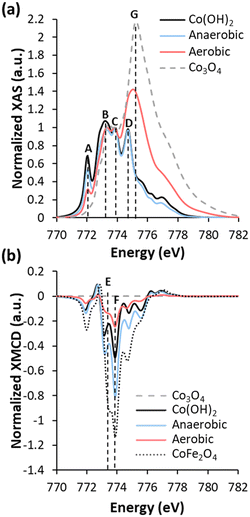 |
| Fig. 7 Normalized (a) XAS and (b) XMCD spectra at the Co L2 edge of non-stoichiometric magnetite (R0.1) under anaerobic conditions (blue), with [Co]s = 19.9 atoms per nm2, and aerobic conditions (red), with [Co]s = 7.1 atoms per nm2 ([Fe]tot = 6.5 mM and [Co]tot = 3 mM; pH 8), with references of Co(OH)2 (black line), Co3O4 (dashed gray line) and CoFe2O4 (dotted black line). XAS and XMCD of Co signals are normalized by dividing the raw signal by the XAS peak C. | |
4. Conclusions
Magnetite nanoparticles are increasingly being studied for environmental applications due to their unique properties and natural presence in the environment. Their affinity with metals and their easy synthesis make them ideal candidates for water and soil remediation. However, the adsorption of metals onto magnetite is highly dependent on environmental factors. This study is focused on the interactions between Co and nanomagnetite as a function of magnetite stoichiometry (Fe(II)/Fe(III) = 0.1, 0.3 and 0.5), pH, the presence of organic matter and redox conditions. The impact of various environmental factors was probed by adsorption experiments and XAS and XMCD analyses. The results show that magnetite stoichiometry plays a crucial role in Co adsorption, which depended on Co surface loading. While no important effects are observed at trace Co levels, where monomers in ferrimagnetic structure prevail, non-stoichiometric magnetite had a smaller capacity to promote the formation of magnetically silent polynuclear Co species and appeared to serve less efficiently as a template for antiferromagnetic Co-(hydr)oxide precipitation than higher stoichiometry magnetites. In addition, results revealed a significant impact of the other environmental factors studied, with Co adsorption limited to acid pH and monomer adsorption favored due to both competition with H+ for magnetite surface sites and the larger stoichiometric number of H+ involved in the cation polymerization reaction. Co adsorption was little affected by [OM], unless very large concentrations were tested ([OM] = 50 mg L−1), which might be due to the formation of ternary complexes between surface sites, Co and OM. Nevertheless, Co2+ remained magnetically interacting with magnetite at all [OM]. Finally, partial oxidation of Co(II) to Co(III) occurred on the magnetite surface under aerobic conditions, hence limiting the formation of a Co(OH)2-like in favor of a Co3O4-like phase. This study has shed light onto the joint effects of magnetite stoichiometry and environmental factors on Co adsorption, surface speciation and magnetic behavior on the magnetite surface. The present data might also be used for the development of a mechanistic surface complexation model that should not only account for the various phenomena listed in the introduction (e.g. surface charging and related electrostatic effects, competition with cations and protons, ternary complexes with inorganic ligands and OM) but also the indirect effects (through a modification of magnetite stoichiometry) of pH, redox conditions, and OM on Co(II) adsorption to magnetite. Indeed, as magnetite stoichiometry is strongly correlated with pH and redox conditions, knowledge of Co speciation as a function of magnetite stoichiometry and environmental factors is essential to better control remediation from magnetite nanoparticles, to predict environmental mechanisms, and potentially for the synthesis of Co-modified magnetite nanoparticles using water as solvent.
Author contributions
Laura Fablet: writing – review & editing, original draft, data curation, investigation, visualization. Mathieu Pédrot: writing – review & editing, resources, supervision, project administration, funding acquisition. Fadi Choueikani: writing – review & editing, resources, supervision, project administration, funding acquisition. Rémi Marsac: writing – review & editing, resources, supervision, project administration, funding acquisition.
Conflicts of interest
There are no conflicts to declare.
Acknowledgements
This work was supported by the French Brittany Region (ARED project “NANOMAG”), SOLEIL synchrotron, the “COLOSSAL” project funded by ANR (project number ANR-23-CE01-0001) and the SURFNANO project funded by the CNRS-INSU EC2CO program. Through the support of the GeOHeLiS analytical platform of Rennes University, this publication is also supported by the European Union through the European Regional Development Fund (FEDER), the French Ministry of Higher Education and Research, and the French Region of Brittany and Rennes Metropole. The authors further acknowledge the SOLEIL synchrotron staff for beamtime allocation at the DEIMOS beamline (proposal no. 20210864). The authors are grateful to M. Bouhnik-Le Coz and M. Pattier for assistance in ICP-MS and UV-vis analysis, V. Dorcet and L. Rault for assistance in TEM experiments performed on the THEMIS platform (ScanMAT, UMS 2011 University of Rennes-CNRS; CPER-FEDER 2007–2014).
References
-
D. A. Dzombak and F. M. M. Morel, Surface Complexation Modeling: Hydrous Ferric Oxide, John Wiley & Sons, New York, 1991 Search PubMed.
- M. Ponthieu, F. Juillot, T. Hiemstra, W. H. van Riemsdijk and M. F. Benedetti, Metal ion binding to iron oxides, Geochim. Cosmochim. Acta, 2006, 70, 2679–2698 CrossRef CAS.
-
R. M. Cornell and U. Schwertmann, The Iron Oxides: Structure, Properties, Reactions, Occurences and Uses, Wiley-VCH, Berlin, 1st edn, 2003 Search PubMed.
- T. Hiemstra and W. H. Van Riemsdijk, A Surface Structural Approach to Ion Adsorption: The Charge Distribution (CD) Model, J. Colloid Interface Sci., 1996, 179, 488–508 CrossRef CAS.
- I. M. Ugwu and D. M. Sherman, Irreversibility of sorption of cobalt to goethite (α-FeOOH) and disparities in dissolution of aged synthetic Co-goethite, Chem. Geol., 2017, 467, 168–176 CrossRef CAS.
- J. Lutzenkirchen and Ph. Behra, On the surface precipitation model for cation sorption at the (hydr)oxide water interface, Aquat. Geochem., 1996, 1, 375–397 CrossRef.
- T. Hiemstra and W. H. van Riemsdijk, Adsorption and surface oxidation of Fe(II) on metal (hydr)oxides, Geochim. Cosmochim. Acta, 2007, 71, 5913–5933 CrossRef CAS.
- W. Cheng, J. Li, J. Sun, T. Luo, R. Marsac, J.-F. Boily and K. Hanna, Nalidixic Acid and Fe(II)/Cu(II) Coadsorption at Goethite and Akaganéite Surfaces, Environ. Sci. Technol., 2023, 57, 15680–15692 CrossRef CAS PubMed.
- W. Cheng, E. L. Kalahroodi, R. Marsac and K. Hanna, Adsorption of Quinolone Antibiotics to Goethite under Seawater Conditions: Application of a Surface Complexation Model, Environ. Sci. Technol., 2019, 53, 1130–1138 CrossRef CAS PubMed.
- L. Zhou, W. Cheng, R. Marsac, J.-F. Boily and K. Hanna, Silicate surface coverage controls quinolone transport in saturated porous media, J. Colloid Interface Sci., 2022, 607, 347–356 CrossRef CAS PubMed.
- L. Weng, W. H. Van Riemsdijk and T. Hiemstra, Cu2+ and Ca2+adsorption to goethite in the presence of fulvic acids, Geochim. Cosmochim. Acta, 2008, 72, 5857–5870 CrossRef CAS.
- J. Li, L. Weng, Y. Deng, J. Ma, Y. Chen and Y. Li, NOM-mineral interaction: Significance for speciation of cations and anions, Sci. Total Environ., 2022, 820, 153259 CrossRef CAS PubMed.
- P. E. Reiller, Modelling metal–humic substances–surface systems: reasons for success, failure and possible routes for peace of mind, Mineral. Mag., 2012, 76, 2643–2658 CrossRef CAS.
- C. A. Gorski and M. M. Scherer, Influence of Magnetite Stoichiometry on FeII Uptake and Nitrobenzene Reduction, Environ. Sci. Technol., 2009, 43, 3675–3680 CrossRef CAS PubMed.
- G. F. Goya, T. S. Berquó, F. C. Fonseca and M. P. Morales, Static and dynamic magnetic properties of spherical magnetite nanoparticles, J. Appl. Phys., 2003, 94, 3520–3528 CrossRef CAS.
- B. Issa, I. M. Obaidat, B. A. Albiss and Y. Haik, Magnetic Nanoparticles: Surface Effects and Properties Related to Biomedicine Applications, Int. J. Mol. Sci., 2013, 14, 21266–21305 CrossRef CAS PubMed.
- P. Jungcharoen, M. Pédrot, F. Choueikani, M. Pasturel, K. Hanna, F. Heberling, M. Tesfa and R. Marsac, Probing the effects of redox conditions and dissolved Fe 2+ on nanomagnetite stoichiometry by wet chemistry, XRD, XAS and XMCD, Environ. Sci.: Nano, 2021, 8, 2098–2107 RSC.
- P. Jungcharoen, M. Pédrot, F. Heberling, K. Hanna, F. Choueikani, C. Catrouillet, A. Dia and R. Marsac, Prediction of nanomagnetite stoichiometry (Fe(II)/Fe(III)) under contrasting pH and redox conditions, Environ. Sci.: Nano, 2022, 9, 2363–2371 RSC.
- C. A. Gorski, J. Nurmi, P. Tratnyek, T. Hofstetter and M. M. Scherer, Redox Behavior of Magnetite: Implications for Contaminant Reduction, Environ. Sci. Technol., 2010, 44, 55–60 CrossRef CAS PubMed.
- J. M. Byrne, N. Klueglein, C. Pearce, K. M. Rosso, E. Appel and A. Kappler, Redox cycling of Fe(II) and Fe(III) in magnetite by Fe-metabolizing bacteria, Science, 2015, 347, 1473–1476 CrossRef CAS PubMed.
- P. Jungcharoen, R. Marsac, F. Choueikani, D. Masson and M. Pédrot, Influence of organic ligands on the stoichiometry of magnetite nanoparticles, Nanoscale Adv., 2023, 5, 4213–4223 RSC.
- A. Sundman, J. M. Byrne, I. Bauer, N. Menguy and A. Kappler, Interactions between magnetite and humic substances: redox reactions and dissolution processes, Geochem. Trans., 2017, 18, 6 CrossRef PubMed.
- B. A. Maher and R. M. Taylor, Formation of ultrafine-grained magnetite in soils, Nature, 1988, 336, 368–370 CrossRef CAS.
- W. Kündig and R. Steven Hargrove, Electron hopping in magnetite, Solid State Commun., 1969, 7, 223–227 CrossRef.
- M. Usman, J. M. Byrne, A. Chaudhary, S. Orsetti, K. Hanna, C. Ruby, A. Kappler and S. B. Haderlein, Magnetite and Green Rust: Synthesis, Properties, and Environmental Applications of Mixed-Valent Iron Minerals, Chem. Rev., 2018, 118, 3251–3304 CrossRef CAS PubMed.
- D. E. Latta, C. A. Gorski, M. I. Boyanov, E. J. O'Loughlin, K. M. Kemner and M. M. Scherer, Influence of Magnetite Stoichiometry on UVI Reduction, Environ. Sci. Technol., 2012, 46, 778–786 CrossRef CAS PubMed.
- C.-H. Liu, Y.-H. Chuang, T.-Y. Chen, Y. Tian, H. Li, M.-K. Wang and W. Zhang, Mechanism of Arsenic Adsorption on Magnetite Nanoparticles from Water: Thermodynamic and Spectroscopic Studies, Environ. Sci. Technol., 2015, 49, 7726–7734 CrossRef CAS PubMed.
- M. L. Peterson, A. F. White, E. Brown Gordon and G. A. Parks, Surface Passivation of Magnetite by Reaction with Aqueous Cr(VI): XAFS and TEM Results, Environ. Sci. Technol., 1997, 31, 1573–1576 CrossRef CAS.
- Z. Pan, B. Bártová, T. LaGrange, S. M. Butorin, N. C. Hyatt, M. C. Stennett, K. O. Kvashnina and R. Bernier-Latmani, Nanoscale mechanism of UO2 formation through uranium reduction by magnetite - à lire, Nat. Commun., 2020, 11, 4001 CrossRef CAS PubMed.
- L. Gao, J. Zhuang, L. Nie, J. Zhang, Y. Zhang, G. Ning, T. Wang, J. Feng, D. Yang, S. Perrett and X. Yan, Intrinsic peroxidase-like activity of ferromagnetic nanoparticles, Nat. Nanotechnol., 2007, 2, 577–583 CrossRef CAS PubMed.
- R. A. Revia and M. Zhang, Magnetite nanoparticles for cancer diagnosis, treatment, and treatment monitoring: recent advances, Mater. Today, 2016, 19, 157–168 CrossRef CAS PubMed.
- Z. R. Stephen, F. M. Kievit and M. Zhang, Magnetite nanoparticles for medical MR imaging, Mater. Today, 2011, 14, 330–338 CrossRef CAS PubMed.
- S. G. Ardo, S. Nélieu, G. Ona-Nguema, G. Delarue, J. Brest, E. Pironin and G. Morin, Oxidative Degradation of Nalidixic Acid by Nano-magnetite via Fe2+/O2-Mediated Reactions, Environ. Sci. Technol., 2015, 49, 4506–4514 CrossRef CAS PubMed.
- W. Cheng, R. Marsac and K. Hanna, Influence of Magnetite Stoichiometry on the Binding of Emerging Organic Contaminants, Environ. Sci. Technol., 2018, 52, 467–473 CrossRef CAS PubMed.
- A. Uheida, G. Salazar-Alvarez, E. Björkman, Z. Yu and M. Muhammed, Fe3O4 and γ-Fe2O3 nanoparticles for the adsorption of Co2+ from aqueous solution, J. Colloid Interface Sci., 2006, 298, 501–507 CrossRef CAS PubMed.
- R. Jolsterå, L. Gunneriusson and A. Holmgren, Surface complexation modeling of Fe3O4–H+ and Mg(II) sorption onto maghemite and magnetite, J. Colloid Interface Sci., 2012, 386, 260–267 CrossRef PubMed.
- N. Morelová, N. Finck, J. Lützenkirchen, D. Schild, K. Dardenne and H. Geckeis, Sorption of americium/europium onto magnetite under saline conditions: Batch experiments, surface complexation modelling and X-ray absorption spectroscopy study, J. Colloid Interface Sci., 2020, 561, 708–718 CrossRef PubMed.
- S. C. N. Tang and I. M. C. Lo, Magnetic nanoparticles: Essential factors for sustainable environmental applications, Water Res., 2013, 47, 2613–2632 CrossRef CAS PubMed.
-
L. Carlos, F. S. G. Einschlag, M. C. González, D. O. Mártire, L. Carlos, F. S. G. Einschlag, M. C. González and D. O. Mártire, in Waste Water - Treatment Technologies and Recent Analytical Developments, IntechOpen, 2013 Search PubMed.
- M. Namdeo, Magnetite Nanoparticles as Effective Adsorbent for Water Purification-A Review, Advances in Recycling & Waste Management, 2017, 2, 1–13 Search PubMed.
- Y.-M. Hao, C. Man and Z.-B. Hu, Effective removal of Cu (II) ions from aqueous solution by amino-functionalized magnetic nanoparticles, J. Hazard. Mater., 2010, 184, 392–399 CrossRef CAS PubMed.
- J. Hu, G. Chen and I. M. C. Lo, Removal and recovery of Cr(VI) from wastewater by maghemite nanoparticles, Water Res., 2005, 39, 4528–4536 CrossRef CAS PubMed.
- F. Choueikani, F. Royer, D. Jamon, A. Siblini, J. J. Rousseau, S. Neveu and J. Charara, Magneto-optical waveguides made of cobalt ferrite nanoparticles embedded in silica/zirconia organic-inorganic matrix, Appl. Phys. Lett., 2009, 94, 051113 CrossRef.
- H. Amata, F. Royer, F. Choueikani, D. Jamon, F. Parsy, J.-E. Broquin, S. Neveu and J. Jacques Rousseau, Hybrid magneto-optical mode converter made with a magnetic nanoparticles-doped SiO2/ZrO2 layer coated on an ion-exchanged glass waveguide, Appl. Phys. Lett., 2011, 99, 251108 CrossRef.
- M. Sugimoto, The Past, Present, and Future of Ferrites, J. Am. Chem. Soc., 1999, 82, 269–280 CAS.
- K. Sartori, G. Cotin, C. Bouillet, V. Halté, S. Bégin-Colin, F. Choueikani and B. P. Pichon, Strong interfacial coupling through exchange interactions in soft/hard core–shell nanoparticles as a function of cationic distribution, Nanoscale, 2019, 11, 12946–12958 RSC.
- S. Staniland, W. Williams, N. Telling, G. Laan, A. Harrison and B. Ward, Controlled cobalt doping of magnetosomes in vivo, Nat. Nanotechnol., 2008, 3, 158–162 CrossRef CAS PubMed.
- B. Babukutty, N. Kalarikkal and S. S. Nair, Studies on structural, optical and magnetic properties of cobalt substituted magnetite fluids (CoxFe1−xFe2O4), Mater. Res. Express, 2017, 4, 035906 CrossRef.
- S. P. Gubin, Y. I. Spichkin, G. Y. Yurkov and A. M. Tishin, Nanomaterial for High-Density Magnetic Data Storage, Russ. J. Inorg. Chem., 2002, 47, 32–67 Search PubMed.
- F. Choueikani, D. Jamon, S. Neveu, M.-F. Blanc-Mignon, Y. Lefkir and F. Royer, Self-biased magneto-optical films based on CoFe2O4–silica nanocomposite, J. Appl. Phys., 2021, 129, 023101 CrossRef CAS.
- L. Fablet, F. Choueikani, M. Pédrot, M. Kerdiles, M. Pasturel and R. Marsac, Investigation of magnetite–Co interactions: from environmentally relevant trace Co levels to core–shell Fe3O4@Co(OH)2 nanoparticles with magnetic applications, Environ. Sci.: Nano, 2023, 10, 3051–3061 RSC.
- R. Massart, Preparation of aqueous magnetic liquids in alkaline and acidic media, IEEE Trans. Magn., 1981, 17, 1247–1248 CrossRef.
- E. Demangeat, M. Pédrot, A. Dia, M. Bouhnik-le-Coz, F. Grasset, K. Hanna, M. Kamagate and F. Cabello-Hurtado, Colloidal and chemical stabilities of iron oxide nanoparticles in aqueous solutions: the interplay of structural, chemical and environmental drivers, Environ. Sci.: Nano, 2018, 5, 992–1001 RSC.
- W. B. Fortune and M. G. Mellon, Determination of Iron with o-Phenanthroline: A Spectrophotometric Study, Ind. Eng. Chem., Anal. Ed., 1938, 10, 60–64 CrossRef CAS.
- H. Tamura, N. Katayama and R. Furuichi, The Co2+Adsorption Properties of Al2O3, Fe2O3, Fe3O4, TiO2, and MnO2Evaluated by Modeling with the Frumkin Isotherm, J. Colloid Interface Sci., 1997, 195, 192–202 CrossRef CAS PubMed.
- K. O. Zahir and H. Keshtkar, A Colorimetric Method for Trace Level Determination of Cobalt in Natural and Waste Water Samples, Int. J. Environ. Anal. Chem., 1998, 72, 151–162 CrossRef CAS.
- D. Yeghicheyan, D. Aubert, M. Bouhnik-Le Coz, J. Chmeleff, S. Delpoux, I. Djouraev, G. Granier, F. Lacan, J.-L. Piro, T. Rousseau, C. Cloquet, A. Marquet, C. Menniti, C. Pradoux, R. Freydier, E. Vieira da Silva-Filho and K. Suchorski, A New Interlaboratory Characterisation of Silicon, Rare Earth Elements and Twenty-Two Other Trace Element Concentrations in the Natural River Water Certified Reference Material SLRS-6 (NRC-CNRC), Geostand. Geoanal. Res., 2019, 43, 475–496 CrossRef CAS.
- P. Ohresser, E. Otero, F. Choueikani, K. Chen, S. Stanescu, F. Deschamps, T. Moreno, F. Polack, B. Lagarde, J.-P. Daguerre, F. Marteau, F. Scheurer, L. Joly, J.-P. Kappler, B. Muller, O. Bunau and Ph. Sainctavit, DEIMOS: A beamline dedicated to dichroism measurements in the 350–2500 eV energy range, Rev. Sci. Instrum., 2014, 85, 013106 CrossRef CAS PubMed.
- S. Brice-Profeta, M.-A. Arrio, E. Tronc, N. Menguy, I. Letard, C. Cartier dit Moulin, M. Noguès, C. Chanéac, J.-P. Jolivet and Ph. Sainctavit, Magnetic order in γ-Fe2O3 nanoparticles: a XMCD study, J. Magn. Magn. Mater., 2005, 288, 354–365 CrossRef CAS.
- A. Motl, F. Šebesta, J. D. Navratil and J. Hlavicova, Sorption of cobalt on magnetite, Czech. J. Phys., 2003, 53, A515–A523 CrossRef CAS.
- M. H. Bradbury and B. Baeyens, Sorption modelling on illite. Part II: Actinide sorption and linear free energy relationships, Geochim. Cosmochim. Acta, 2009, 73, 1004–1013 CrossRef CAS.
- K. J. Farley, D. A. Dzombak and F. M. M. Morel, A surface precipitation model for the sorption of cations on metal oxides, J. Colloid Interface Sci., 1985, 106, 226–242 CrossRef CAS.
- M. R. Matsumoto, P. M. McGinley, B. E. Reed and J. N. Jensen, Physicochemical Processes, Water Environ. Res., 1992, 64, 337–346 CrossRef CAS.
- J.-S. Kwon, S.-T. Yun, J.-H. Lee, S.-O. Kim and H. Y. Jo, Removal of divalent heavy metals (Cd, Cu, Pb, and Zn) and arsenic(III) from aqueous solutions using scoria: Kinetics and equilibria of sorption, J. Hazard. Mater., 2010, 174, 307–313 CrossRef CAS PubMed.
-
M. W. Haverkort, PhD, University Koeln, 2005 Search PubMed.
- Y. Prado, N. Daffé, A. Michel, T. Georgelin, N. Yaacoub, J.-M. Grenèche, F. Choueikani, E. Otero, P. Ohresser, M.-A. Arrio, C. Cartier-dit-Moulin, P. Sainctavit, B. Fleury, V. Dupuis, L. Lisnard and J. Fresnais, Enhancing the magnetic anisotropy of maghemite nanoparticles via the surface coordination of molecular complexes, Nat. Commun., 2015, 6, 1–8 Search PubMed.
- V. E. Campbell, M. Tonelli, I. Cimatti, J.-B. Moussy, L. Tortech, Y. J. Dappe, E. Rivière, R. Guillot, S. Delprat, R. Mattana, P. Seneor, P. Ohresser, F. Choueikani, E. Otero, F. Koprowiak, V. G. Chilkuri, N. Suaud, N. Guihéry, A. Galtayries, F. Miserque, M.-A. Arrio, P. Sainctavit and T. Mallah, Engineering the magnetic coupling and anisotropy at the molecule–magnetic surface interface in molecular spintronic devices, Nat. Commun., 2016, 7, 13646 CrossRef CAS PubMed.
- I. Christl and R. Kretzschmar, Interaction of copper and fulvic acid at the hematite-water interface, Geochim. Cosmochim. Acta, 2001, 65, 3435–3442 CrossRef CAS.
- S. Masset, F. Monteil-Rivera, L. Dupont, J. Dumonceau and M. Aplincourt, Influence of humic acid on sorption of Co(II), Sr(II), and Se(IV) on goethite, Agronomie, 2000, 20, 525–535 CrossRef.
- V. Papaefthimiou, T. Dintzer, V. Dupuis, A. Tamion, F. Tournus, A. Hillion, D. Teschner, M. Hävecker, A. Knop-Gericke, R. Schlögl and S. Zafeiratos, Nontrivial Redox Behavior of Nanosized Cobalt: New Insights from Ambient Pressure X-ray Photoelectron and Absorption Spectroscopies, ACS Nano, 2011, 5, 2182–2190 CrossRef CAS PubMed.
-
H. Singh, PhD, Homi Bhabha National Institute, 2015 Search PubMed.
- D. Vantelon, M. Davranche, R. Marsac, C. L. Fontaine, H. Guénet, J. Jestin, G. Campaore, A. Beauvois and V. Briois, Iron speciation in iron–organic matter nanoaggregates: a kinetic approach coupling Quick-EXAFS and MCR-ALS chemometrics, Environ. Sci.: Nano, 2019, 6, 2641–2651 RSC.
- H. Guénet, M. Davranche, D. Vantelon, J. Gigault, S. Prévost, O. Taché, S. Jaksch, M. Pédrot, V. Dorcet, A. Boutier and J. Jestin, Characterization of iron–organic matter nano-aggregate networks through a combination of SAXS/SANS and XAS analyses: impact on As binding, Environ. Sci.: Nano, 2017, 4, 938–954 RSC.
- Y.-J. Hsu, Y.-L. Lai, C.-H. Chen, Y.-C. Lin, H.-Y. Chien, J.-H. Wang, T.-N. Lam, Y.-L. Chan, D. H. Wei, H.-J. Lin and C.-T. Chen, Enhanced Magnetic Anisotropy via Quasi-Molecular Magnet at Organic-Ferromagnetic Contact, J. Phys. Chem. Lett., 2013, 4, 310–316 CrossRef CAS PubMed.
- S. Zafeiratos, T. Dintzer, D. Teschner, R. Blume, M. Hävecker, A. Knop-Gericke and R. Schlögl, Methanol oxidation over model cobalt catalysts: Influence of the cobalt oxidation state on the reactivity, J. Catal., 2010, 269, 309–317 CrossRef CAS.
|
This journal is © The Royal Society of Chemistry 2024 |