Singlet oxygen is not the main source of electrolyte degradation in lithium–oxygen batteries†
Received
19th May 2024
, Accepted 14th August 2024
First published on 27th August 2024
Abstract
The lithium–air (oxygen) battery could offer significant improvements in gravimetric energy density compared to lithium-ion technology. A major barrier to realising this goal is the oxidative degradation of the electrolyte solution and the carbon at the positive electrode. Recently, the lithium–oxygen field has been focused on the formation of singlet oxygen within the cell, its impact as a major source of degradation, and strategies to mitigate this. Here we have investigated the reactivity of components within the lithium–oxygen cell by exposure to photochemically generated singlet oxygen. We find no significant reaction between the singlet oxygen and tetraglyme, lithium bis(trifluoromethanesulfonyl)imide, or carbon, standard electrode components, and confirm that singlet oxygen is not the major source of degradation in the lithium–oxygen battery. Our studies bring into question the need for strategies to mitigate the impact of singlet oxygen in the cell and highlight the need to refocus on the discovery of electrolyte solutions with stability against lithium peroxide.
Broader context
Singlet oxygen, a high energy state of dioxygen, has been shown to form during the oxygen redox reactions within the lithium–oxygen battery and has been linked to degradation, specifically of the solvent, salt and carbon in the positive electrode. This has triggered a pivot in the field towards additives to deactivate these proposed antagonistic species, despite a lack of understanding of the role of singlet oxygen in the cell. To quantify the impact of singlet oxygen in the lithium–oxygen battery, we have exposed each component, solvent, salt and carbon, to photocatalytically generated singlet oxygen, analyzed the resulting product, and determined effective rates of any singlet oxygen reactions. Our data shows almost no reaction between singlet oxygen and the components of the cell. Analysis of the rate of reaction between singlet oxygen and the solvent suggests that during a typical cycle of the lithium–oxygen battery, singlet oxygen would be responsible for approximately 0.002% capacity loss each cycle, which is not consistent with the 5–10% found in practice. Our studies suggest that singlet oxygen is not responsible for the failure of the lithium–oxygen battery and further innovation in this direction will not yield the improvement in cycle life required for commercialisation utilization.
|
Introduction
The high theoretical specific energy density of lithium–air (Li–air, Li–O2) batteries, 3500 Wh kg−1, makes them ideal for weight-sensitive applications such as in the aerospace sector.1,2 The battery operates through the oxidation of a lithium negative electrode and the reduction of oxygen to lithium peroxide at the positive electrode, with the reactions being reversed on charge. Despite the promise of this battery technology, several challenges hinder its realisation including the development of a stable protected lithium electrode, operation with CO2 and H2O contaminants, efficient oxidation of insulating Li2O2, and development of a gas diffusion electrode able to deliver oxygen to an electrode containing an organic solvent.3–11 A key challenge in the development of the Li–O2 battery is the degradation of the electrolyte solution when exposed to the oxygenic reactants at the positive electrode.
The degradation of the electrolyte solution and carbon electrode in the Li–O2 battery has been extensively studied, with the majority of common organic solvents being shown to be unstable within the cell.12–17 In fact, only glyme ethers are widely accepted as being relatively stable and even these state-of-the-art systems are known to undergo degradation. The specifics of degradation within the cell remain an area of active debate. Various oxygenic species have been implicated in the degradation of glyme ethers as cells containing ethers display a Li2O2% yield of no more than 95% on discharge.18–24 Recently, reactive singlet oxygen (1O2) has been shown to form during Li2O2 and Li2CO3 oxidation,25–29 LiO2 disproportionation,30,31 and LiO2 oxidation by a redox mediator.32 Due to a correlation between 1O2 formation in the cell and degradation, 1O2 has been implicated as a major source of electrolyte solution degradation, in addition to degrading other cell components.28,29,33–38 The addition of 1O2 traps and quenchers have been used to reduce the impact of 1O2 and appear to show some benefit to cell stability.28,34,39 Kwak and co-workers demonstrated that glyme ethers degrade in the presence of in-situ generated 1O2 when using 30% H2O2 in water and NaOCl, however, these reagents may not be innocent.40 Further support from computation studies by Mullinax et al. suggest that hydrogen atom abstraction at the secondary carbons of dimethoxyethane (monoglyme ether) by 1O2 would yield H2O2.38 Despite the reported links between 1O2 and electrolyte degradation, there is currently no consensus on its reactivity within the cell and no specific reactions have been proven. Moreover, methods used to detect 1O2 in the cell have been questioned,41 where the stability and selectivity of traps such as 4-oxo-2,2,6,6-tetramethyl-1-piperidinyloxy (4-oxo-TEMPO) and 9,10-dimethylanthracene (DMA) towards 1O2 have been shown to depend on the solution environment.42–46 It is important to understand the true cause of degradation to work towards practical Li–air batteries.
Here we investigate the reaction between 1O2 and the commonly used Li–O2 electrode components, tetraglyme, lithium bis(trifluoromethanesulfonyl)imide (LiTFSI), and carbon. 1O2 is formed in-situ photochemically, with and without the application of an oxidising potential in three-electrode cells, and O2 consumption is monitored using on-line mass spectrometry for signs of degradation. Our analysis shows that 1O2 does not readily react with either carbon, tetraglyme, or the TFSI anion, in contradiction to recent studies. Using nuclear magnetic resonance (NMR) spectroscopy, we show that 1O2 will have a negligible impact on the cell, confirming that it is not the major cause of degradation in Li–O2 cells containing linear ethers and TFSI salts.
Results and discussion
To investigate the reactivity of 1O2 with tetraglyme and the commonly used electrolyte solution 1 M LiTFSI in tetraglyme, 1O2 was generated in each solution from ground state triplet oxygen (3O2) using the photosensitiser, rose bengal (RB) while illuminated with a 530 nm light source (see ESI† for further details). On-line mass spectrometry (MS) was used to monitor the changes in the O2 concentration, which in the absence of a degradation reaction involving 1O2, should remain constant (Fig. 1). Notably, the steady-state concentration of 1O2 using this method is ∼1000 times greater than that expected to form in the cell at 100 μA (see ESI† for details).31 To ensure comparability, the electrolyte components were of grade consistent with that used for Li–O2 cells, thus, commercial tetraglyme (≥99%) and LiTFSI (99.99%) were further dried over molecular sieves (H2O < 10 ppm) and at 85 °C under vacuum, respectively (see ESI† for details). To demonstrate the efficacy of the analysis we first examine a solvent with known instability to 1O2, dimethyl sulfoxide (DMSO), which showed a marked decrease in O2 concentration during the illumination period, consistent with the instability of DMSO against 1O2. NMR and infrared spectroscopy (Fig. S2, ESI†) of the resulting solution confirmed the formation of the oxidation product, dimethyl sulfone (see ESI† for details).12 When repeating the measurement with tetraglyme, no significant change in O2 concentration was observed, indicating no notable reaction between 1O2 and the solvent, although a small drop in O2 concentration was occasionally observed upon initial exposure to light, likely due to the reaction of 1O2 with impurities in the liquid (Fig. 1). Similar results were obtained for LiTFSI in tetraglyme, suggesting both LiTFSI and tetraglyme are stable towards 1O2 under these chemical conditions.
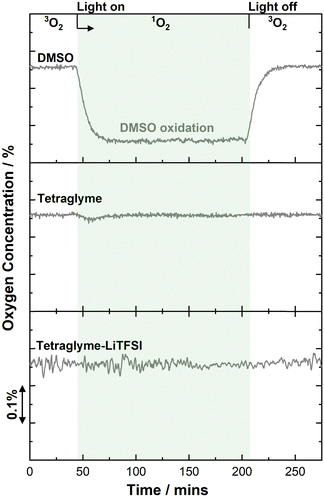 |
| Fig. 1 On-line MS showing that DMSO readily reacts with 1O2 whereas tetraglyme and 1 M LiTFSI in tetraglyme show no significant reactivity. The O2 concentration versus time as oxygen is bubbled through the relevant liquid and 1O2 photochemically generated using a photosensitiser. A loss of O2 signal indicates degradation due to reactions with 1O2. | |
While LiTFSI in tetraglyme shows no detectable reaction with 1O2, degradation may still occur in the cell during the application of an oxidising potential. To simulate conditions within the Li–O2 cell, 1O2 was formed photochemically within the electrolyte solution while also applying an oxidising potential of 3.8 V vs. Li|Li+, where 1O2 has been shown to evolve and CO2 release linked to degradation occurs,25 to a submerged carbon-13 electrode (7 mm diameter carbon disk, geometric area 0.769 m2) for 12 h (Fig. 2a). Measurements above 4.0 V were avoided as the electrolyte solution is known to break down in this region,25b,47 and in practice lithium–air cells should avoid cycling above this voltage. Again, O2 consumption was measured to monitor degradation as shown in Fig. 2b, where O2 flux remained constant under these conditions, suggesting that the electrolyte appears to be relatively stable towards 1O2 even with an oxidising potential.
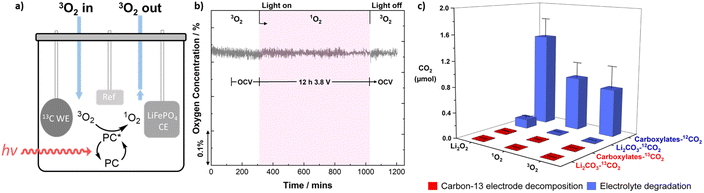 |
| Fig. 2 Exploring the impact of an oxidising potential on the reaction of 1O2 with LiTFSI, tetraglyme and carbon. (a) A schematical representation of the cell used to generate 1O2 at a carbon-13 electrode with an applied potential (3.8 V vs. Li|Li+). The cell contains a carbon-13 working electrode (WE), LiFePO4 counter electrode (CE), delithiated LiFePO4 reference electrode (REF), 0.1 M LiTFSI in tetraglyme containing 7 μM photosynthesiser, tetraphenylporphyrin (TPP), that were irradiated at 660 nm. A carbon-13 electrode was used to separate degradation products from the electrode (13C) and the electrolyte solution (12C). (b) On-line MS data showing the O2 concentration during OCV and 1O2 generation at 3.8 V vs. Li|Li+. (c) Plot of CO2 released by Li2CO3 and carboxylate degradation products formed at the electrode from electrolyte degradation (12CO2) and carbon-13 electrode degradation (13CO2) during application of a potential (3.8 V vs. Li|Li+) and exposure to 3O2, 1O2, or preloaded Li2O2 carbon-13 electrode (argon atmosphere). | |
After exposure to 1O2 under an applied potential, the carbon-13 electrodes were analysed for common degradation products by evolution of CO2 (Fig. 2c) produced from: Li2CO3 by treatment with H2PO4, and carboxylates by treatment with Fenton's reagent (see ESI† for the details).47 The origin of the degradation could be determined by the respective CO2 isotope, with 12CO2 originating from electrolyte degradation and 13CO2 formed from the decomposition of the carbon electrode. Control measurements were performed using 3O2 (without illumination) with no notable carbon-13 electrode degradation observed, however, 12CO2 originating from carboxylates was observed, likely from organic electrolyte impurities. No additional degradation of either the carbon electrode or electrolyte solutions were observed upon introduction of 1O2, suggesting that singlet oxygen does not play a significant role in degradation during the application of an oxidising potential. In contrast, preloading the electrode with Li2O2 and applying a potential under an argon atmosphere resulted in a marked increase in 12CO2 originating from Li2CO3 and carboxylate formation from the electrolyte solution. In all cases, the carbon-13 electrode remained relatively stable with no significant formation of 13CO2 detected.
While our on-line MS analysis rules out 1O2 as a major source of degradation in the cell, it does not preclude a slow reaction between 1O2 and the electrolyte solution components which may impact the long-term performance of the cell. To quantify the rate of reaction between 1O2 and tetraglyme or LiTFSI in tetraglyme, these components were reacted over a longer time frame with 1O2, which was generated from 3O2 by energy transfer photocatalysis using RB (Scheme 1, see ESI† for details).48 Expectedly, tetraglyme was found to be robust towards reactions with 1O2, however, new species were identified by 1H NMR spectroscopy in the regions of 11.0–8.5 ppm and 5.5–4.5 ppm (Fig. 3a and b). These resonances are in the regions typical for organic hydroperoxide proton (OO
) and associated tertiary proton (OC
) environments, respectively, which is consistent with the predicted products for the 1O2 reaction (Scheme 1). Given tetraglyme contains multiple sites vulnerable to C–H insertion (eight ethereal CH2 positions), a series of mono, bis, or greater hydroperoxide containing species are likely the major contributors to the observed signals, but further decomposition products cannot be ruled out. Analysis of the NMR spectra indicates a combined concentration of approximately 2.8 mM for these degradation products (see Supplementary Note 1, ESI†). Based on the rate of tetraglyme degradation by 1O2 in this reaction, and by comparing the relative amount of 1O2 in the reactions with the solvent and the Li–O2 cell (see Supplementary Note 1, ESI†), we estimate that 1O2 would be responsible for ca. 2.2 × 10−3% of degradation compared to the 5–10% degradation reported during a typical discharge, hence its impact on degradation is effectively negligible. Moreover, when exposing 1 M LiTFSI to 1O2, the TFSI anion showed complete stability throughout the reaction despite the abundance of 1O2 and the presence of reactive organic hydroperoxides (Fig. 3c and d). We do not consider the impact of 1O2 on the negative electrode due to its short lifetime; we expect 1O2 to relax back to 3O2 before crossing the separator. While we cannot rule out reactions occurring between 1O2 and redox mediators or decomposition products formed during cycling, but based on our analysis, 1O2 is not the main source of degradation in the commonly used carbon electrode cell containing 1 M LiTFSI in tetraglyme.
 |
| Scheme 1 General reaction of photochemically generated 1O2 with ethereal hydrocarbons. | |
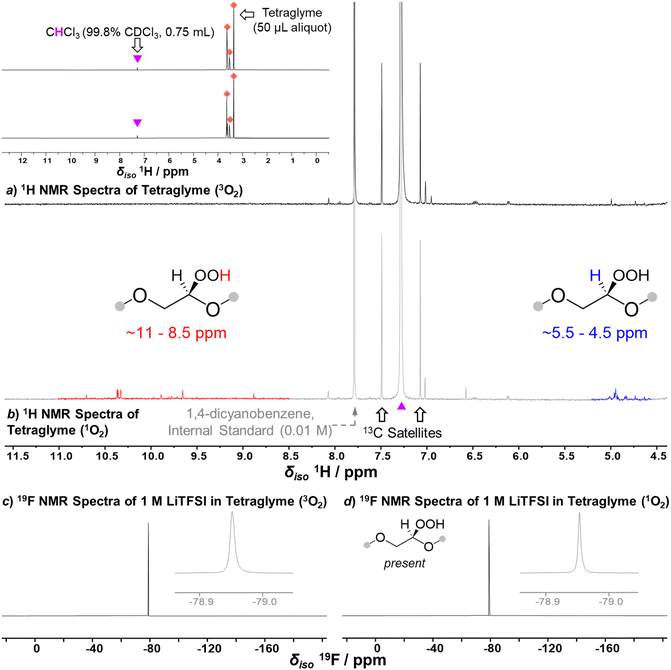 |
| Fig. 3 Spectroscopic analysis of tetraglyme and LiTFSI degradation by 1O2 generated photochemically from 3O2. 1H NMR Spectra (500 MHz, CDCl3, 298 K) of the tetraglyme solution (a) without and (b) with singlet oxygen generation. (inset) Expanded 1H NMR spectra of a (top) and b (bottom) highlighting the relative intensity of signals to tetraglyme. 19F NMR spectra (377 MHz, CDCl3, 298 K) of the LiTFSI tetraglyme solution (c) without and (d) with singlet oxygen generation. Reaction conditions: Rose Bengal (1 × 10−5 M), tetraglyme (4 mL) or 1 M LiTFSI in tetraglyme (4 mL), O2 (1 atm), irradiated with green LEDs for 18 h. 1H NMR spectroscopy (500 MHz, CDCl3, 298 K) of a 50 μL aliquot of reaction solution with 0.01 M 1,4-dicyanobenzene as an internal standard. | |
Conclusion
We have investigated the reactivity of 1O2 with tetraglyme, and LiTFSI in tetraglyme during the application of an oxidising potential at a carbon electrode, which represent the common components and conditions within the lithium–oxygen battery. Generation of 1O2in-situ at an amount a thousand times greater than that formed within the cell, including an extended exposure period, demonstrates negligible reaction with LiTFSI tetraglyme solutions or carbon electrodes. By analysing the product of the reaction between 1O2 and tetraglyme, we estimate that singlet oxygen in the battery is responsible for as little as 2.2 × 10−3% of degradation. Comparatively, we are unable to observe any reaction between 1O2 and TFSI anion indicating that it is effectively robust against reaction with 1O2. In summary, this data suggests that singlet oxygen is not responsible for the 5–10% coulombic efficiency loss per cycle seen in current lithium–air cells and may be relatively innocent. Our studies suggest that the primary products from the reaction of tetraglyme and 1O2 are organic hydroperoxides, despite being incredibly low in concentration, are reactive in their own right, and may impact mature lithium–oxygen cells when performing hundreds of cycles. We suggest that future lithium–oxygen research should focus on finding new electrolytes stable towards lithium peroxide-derived species.
Data availability
The data that support the findings of this study are available from the corresponding authors, PGB and LRJ, upon reasonable request.
Conflicts of interest
There are no conflicts to declare.
Acknowledgements
P. G. B. acknowledges financial support from the EPSRC (EP/M009521/1) and the Henry Royce Institute for Advanced Materials (EP/R00661X/1, EP/S019367/1, EP/R010145/1). LRJ thanks the University of Nottingham's Propulsion Futures Beacon of Excellence. CZ thanks the British Federation of Women Graduates and GCRF Bursary for their support during the author's postgraduate studies.
References
- D. Aurbach, B. D. McCloskey, L. F. Nazar and P. G. Bruce, Advances in Understanding Mechanisms Underpinning Lithium–Air Batteries, Nat. Energy, 2016, 1(9), 16128, DOI:10.1038/nenergy.2016.128
.
- W. J. Kwak, Rosy, D. Sharon, C. Xia, H. Kim, L. R. Johnson, P. G. Bruce, L. F. Nazar, Y. K. Sun, A. A. Frimer, M. Noked and S. A. Freunberger, Aurbach, D. Lithium–Oxygen Batteries and Related Systems: Potential, Status, and Future, Chem. Rev., 2020, 120(14), 6626–6683, DOI:10.1021/acs.chemrev.9b00609
.
- T. Zhang and H. Zhou, A Reversible Long-Life Lithium–Air Battery in Ambient Air, Nat. Commun., 2013, 4, 1817, DOI:10.1038/ncomms2855
.
- J. W. Jordan, G. Vailaya, C. Holc, M. Jenkins, R. C. McNulty, C. Puscalau, B. Tokay, A. Laybourn, X. Gao, D. A. Walsh, G. N. Newton, P. G. Bruce and L. R. Johnson, A Lithium–Air Battery and Gas Handling System Demonstrator, Faraday Discuss., 2024, 248, 381–391, 10.1039/d3fd00137g
.
- Z. Guo, C. Li, J. Liu, Y. Wang and Y. Xia, A Long-Life Lithium–Air Battery in Ambient Air with a Polymer Electrolyte Containing a Redox Mediator, Angew. Chem., Int. Ed., 2017, 56(26), 7505–7509, DOI:10.1002/anie.201701290
.
- B. G. Kim, J.-S. Kim, J. Min, Y.-H. Lee, J. H. Choi, M. C. Jang, S. A. Freunberger and J. W. Choi, A Moisture- and Oxygen-Impermeable Separator for Aprotic Li-O2 Batteries, Adv. Funct. Mater., 2016, 26(11), 1747–1756, DOI:10.1002/adfm.201504437
.
- Z. Huang, Z. Deng, Y. Shen, W. Chen, W. Liu, M. Xie, Y. Li and Y. Huang, A Li-O2 Battery Cathode with Vertical Mass/Charge Transfer Pathways, J. Mater. Chem. A, 2019, 7(7), 3000–3005, 10.1039/c9ta00017h
.
- Y. Wang, N. C. Lai, Y. R. Lu, Y. Zhou, C. L. Dong and Y. C. Lu, A Solvent-Controlled Oxidation Mechanism of Li2O2 in Lithium–Oxygen Batteries, Joule, 2018, 2(11), 2364–2380, DOI:10.1016/j.joule.2018.07.021
.
- B. J. Bergner, A. Schurmann, K. Peppler, A. Garsuch and J. Janek, TEMPO: A Mobile Catalyst for Rechargeable Li-O2 Batteries, J. Am. Chem. Soc., 2014, 136(42), 15054–15064, DOI:10.1021/ja508400m
.
- Y. Chen, X. Gao, L. R. Johnson and P. G. Bruce, Kinetics of Lithium Peroxide Oxidation by Redox Mediators and Consequences for the Lithium–Oxygen Cell, Nat. Commun., 2018, 9(1), 767, DOI:10.1038/s41467-018-03204-0
.
- R. B. Jethwa, S. Mondal, B. Pant and S. A. Freunberger, To DISP or Not? The Far-Reaching Reaction Mechanisms Underpinning Lithium–Air Batteries, Angew. Chem., Int. Ed., 2023, e202316476, DOI:10.1002/anie.202316476
.
- D. Sharon, M. Afri, M. Noked, A. Garsuch, A. A. Frimer and D. Aurbach, Oxidation of Dimethyl Sulfoxide Solutions by Electrochemical Reduction of Oxygen, J. Phys. Chem. Lett., 2013, 4(18), 3115–3119, DOI:10.1021/Jz4017188
.
- D. G. Kwabi, T. P. Batcho, C. V. Amanchukwu, N. Ortiz-Vitoriano, P. Hammond, C. V. Thompson and Y. Shao-Horn, Chemical Instability of Dimethyl Sulfoxide in Lithium–Air Batteries, J. Phys. Chem. Lett., 2014, 5(16), 2850–2856, DOI:10.1021/jz5013824
.
- S. A. Freunberger, Y. Chen, Z. Peng, J. M. Griffin, L. J. Hardwick, F. Barde, P. Novak and P. G. Bruce, Reactions in the Rechargeable Lithium-O2 Battery with Alkyl Carbonate Electrolytes, J. Am. Chem. Soc., 2011, 133(20), 8040–8047, DOI:10.1021/ja2021747
.
- B. D. McCloskey, D. S. Bethune, R. M. Shelby, G. Girishkumar and A. C. Luntz, Solvents’ Critical Role in Nonaqueous Lithium–Oxygen Battery Electrochemistry, J. Phys. Chem. Lett., 2011, 2(10), 1161–1166, DOI:10.1021/jz200352v
.
- D. Sharon, D. Hirsberg, M. Afri, F. Chesneau, R. Lavi, A. A. Frimer, Y. K. Sun and D. Aurbach, Catalytic Behavior of Lithium Nitrate in Li-O2 Cells, ACS Appl. Mater. Interfaces, 2015, 7(30), 16590–16600, DOI:10.1021/acsami.5b04145
.
- W. Walker, V. Giordani, J. Uddin, V. S. Bryantsev, G. V. Chase and D. Addison, A Rechargeable Li-O2 Battery Using a Lithium Nitrate/N,N-Dimethylacetamide Electrolyte, J. Am. Chem. Soc., 2013, 135(6), 2076–2079, DOI:10.1021/ja311518s
.
- V. S. Bryantsev and F. Faglioni, Predicting Autoxidation Stability of Ether- and Amide-Based Electrolyte Solvents for Li–Air Batteries, J. Phys. Chem. A, 2012, 116(26), 7128–7138, DOI:10.1021/jp301537w
.
- N. Kumar, M. D. Radin, B. C. Wood, T. Ogitsu and D. J. Siegel, Surface-Mediated Solvent Decomposition in Li-Air Batteries: Impact of Peroxide and Superoxide Surface Terminations, J. Phys. Chem. C, 2015, 119(17), 9050–9060, DOI:10.1021/acs.jpcc.5b00256
.
- V. S. Bryantsev, V. Giordani, W. Walker, M. Blanco, S. Zecevic, K. Sasaki, J. Uddin, D. Addison and G. V. Chase, Predicting Solvent Stability in Aprotic Electrolyte Li–Air Batteries: Nucleophilic Substitution by the Superoxide Anion Radical (O2•–), J. Phys. Chem. A, 2011, 115(44), 12399–12409, DOI:10.1021/jp2073914
.
- X. Zhang, L. Guo, L. Gan, Y. Zhang, J. Wang, L. R. Johnson, P. G. Bruce and Z. Peng, LiO2: Cryosynthesis and Chemical/Electrochemical Reactivities, J. Phys. Chem. Lett., 2017, 8(10), 2334–2338, DOI:10.1021/acs.jpclett.7b00680
.
- D. Sharon, V. Etacheri, A. Garsuch, M. Afri, A. A. Frimer and D. Aurbach, On the Challenge of Electrolyte Solutions for Li–Air Batteries: Monitoring Oxygen Reduction and Related Reactions in Polyether Solutions by Spectroscopy and EQCM, J. Phys. Chem. Lett., 2012, 4(1), 127–131, DOI:10.1021/jz3017842
.
- R. Younesi, M. Hahlin, F. Björefors, P. Johansson and K. Edström, Li-O2 Battery Degradation by Lithium Peroxide (Li 2O2): A Model Study, Chem. Mater., 2013, 25(1), 77–84, DOI:10.1021/cm303226g
.
- R. S. Assary, K. C. Lau, K. Amine, Y.-K. Sun and L. A. Curtiss, Interactions of Dimethoxy Ethane with Li2O2 Clusters and Likely Decomposition Mechanisms for Li–O2 Batteries, J. Phys. Chem. C, 2013, 117(16), 8041–8049, DOI:10.1021/jp400229n
.
-
(a) J. Wandt, P. Jakes, J. Granwehr, H. A. Gasteiger and R.-A. Eichel, Singlet Oxygen Formation during the Charging Process of an Aprotic Lithium–Oxygen Battery, Angew. Chem., Int. Ed., 2016, 55, 1–5, DOI:10.1002/anie.201602142
;
(b) D. Cao, C. Tan and Y. Chen, Oxidative decomposition mechanisms of lithium carbonate on carbon substrates in lithium battery chemistries, Nat. Commun., 2022, 13, 4908, DOI:10.1038/s41467-022-32557-w
.
- N. Mahne, B. Schafzahl, C. Leypold, M. Leypold, S. Grumm, A. Leitgeb, G. A. Strohmeier, M. Wilkening, O. Fontaine, D. Kramer, C. Slugovc, S. M. Borisov and S. A. Freunberger, Singlet Oxygen Generation as a Major Cause for Parasitic Reactions during Cycling of Aprotic Lithium–Oxygen Batteries, Nat. Energy, 2017, 2, 17036, DOI:10.1038/nenergy.2017.36
.
- N. Mahne, S. E. Renfrew, B. D. McCloskey and S. A. Freunberger, Electrochemical Oxidation of Lithium Carbonate Generates Singlet Oxygen, Angew. Chem., Int. Ed. Engl., 2018, 57(19), 5529–5533, DOI:10.1002/anie.201802277
.
- D. Córdoba, H. B. Rodríguez and E. J. Calvo, Operando Fluorescence Detection of Singlet Oxygen inside High-Performance Li-O2 Batteries, J. Phys. Chem. C, 2023, 127(1), 78–84, DOI:10.1021/acs.jpcc.2c06821
.
- K. Nishioka, M. Tanaka, H. Fujimoto, T. Amaya, S. Ogoshi, M. Tobisu and S. Nakanishi, Overlooked Factors Required for Electrolyte Solvents in Li–O2 Batteries: Capabilities of Quenching 1O2 and Forming Highly-Decomposable Li2O2, Angew. Chem., Int. Ed., 2022, 61, e202112769, DOI:10.1002/anie.202112769
.
- S. Dong, S. Yang, Y. Chen, C. Kuss, G. Cui, L. R. Johnson, X. Gao and P. G. Bruce, Singlet Oxygen and Dioxygen Bond Cleavage in the Aprotic Lithium–Oxygen Battery, Joule, 2022, 6(1), 185–192, DOI:10.1016/j.joule.2021.12.012
.
- E. Mourad, Y. K. Petit, R. Spezia, A. Samojlov, F. F. Summa, C. Prehal, C. Leypold, N. Mahne, C. Slugovc, O. Fontaine, S. Brutti and S. A. Freunberger, Singlet Oxygen from Cation Driven Superoxide Disproportionation and Consequences for Aprotic Metal-O2 Batteries, Energy Environ. Sci., 2019, 12, 2559–2568, 10.1039/c9ee01453e
.
- S. Ahn, C. Zor, S. Yang, M. Lagnoni, D. Dewar, T. Nimmo, C. Chau, M. Jenkins, A. J. Kibler, A. Pateman, G. J. Rees, X. Gao, P. Adamson, N. Grobert, A. Bertei, L. R. Johnson and P. G. Bruce, Why Charging Li–Air Batteries with Current Low-Voltage Mediators Is Slow and Singlet Oxygen Does Not Explain Degradation, Nat. Chem., 2023, 15, 1022–1029, DOI:10.1038/s41557-023-01203-3
.
- N. Mahne, B. Schafzahl, C. Leypold, M. Leypold, S. Grumm, A. Leitgeb, G. A. Strohmeier, M. Wilkening, O. Fontaine, D. Kramer, C. Slugovc, S. M. Borisov and S. A. Freunberger, Singlet Oxygen Generation as a Major Cause for Parasitic Reactions during Cycling of Aprotic Lithium–Oxygen Batteries, Nat. Energy, 2017, 2, 17036, DOI:10.1038/nenergy.2017.36
.
- Y. K. Petit, C. Leypold, N. Mahne, E. Mourad, L. Schafzahl, C. Slugovc, S. M. Borisov and S. A. Freunberger, DABCOnium: An Efficient and High-Voltage Stable Singlet Oxygen Quencher for Metal–O2 Cells, Angew. Chem., Int. Ed., 2019, 58(20), 6535–6539, DOI:10.1002/anie.201901869
.
- H. W. Lee, J. Y. Kim, J. E. Kim, Y. J. Jo, D. Dewar, S. Yang, X. Gao, P. G. Bruce and W. J. Kwak, Effect of Singlet Oxygen on Redox Mediators in Lithium–Oxygen Batteries, J. Mater. Chem. A, 2023, 11, 16003–16008, 10.1039/d3ta01284k
.
- W. J. Kwak, H. Kim, Y. K. Petit, C. Leypold, T. T. Nguyen, N. Mahne, P. Redfern, L. A. Curtiss, H. G. Jung, S. M. Borisov, S. A. Freunberger and Y. K. Sun, Deactivation of Redox Mediators in Lithium–Oxygen Batteries by Singlet Oxygen, Nat. Commun., 2019, 10, 1380, DOI:10.1038/s41467-019-09399-0
.
- A. Y. Tesio, W. Torres, M. Villalba, F. Davia, M. del Pozo, D. Córdoba, F. J. Williams and E. J. Calvo, Role of Superoxide and Singlet Oxygen on the Oxygen Reduction Pathways in Li−O2 Cathodes at Different Li+ Ion Concentration, ChemElectroChem, 2022, 9, e202201037, DOI:10.1002/celc.202201037
.
- J. W. Mullinax, C. W. Bauschlicher and J. W. Lawson, Modeling Singlet Oxygen-Induced Degradation Pathways Including Environmental Effects of 1,2-Dimethoxyethane in Li–O2 Batteries through Density Functional Theory, J. Phys. Chem. A, 2022, 126(43), 7997–8006, DOI:10.1021/acs.jpca.2c06386
.
- Z. Liang, Q. Zou, J. Xie and Y. C. Lu, Suppressing Singlet Oxygen Generation in Lithium–Oxygen Batteries with Redox Mediators, Energy Environ. Sci., 2020, 13, 2870–2877, 10.1039/d0ee01114b
.
- W. J. Kwak, S. Chae, R. Feng, P. Gao, J. Read, M. H. Engelhard, L. Zhong, W. Xu and J. G. Zhang, Optimized Electrolyte with High Electrochemical Stability and Oxygen Solubility for Lithium–Oxygen and Lithium–Air Batteries, ACS Energy Lett., 2020, 5(7), 2182–2190, DOI:10.1021/acsenergylett.0c00809
.
- A. Schürmann, B. Luerßen, D. Mollenhauer, J. Janek and D. Schröder, Singlet Oxygen in Electrochemical Cells: A Critical Review of Literature and Theory, Chem. Rev., 2021, 121(20), 12445–12464, DOI:10.1021/acs.chemrev.1c00139
.
- S. Zhang, M. J. Nava, G. K. Chow, N. Lopez, G. Wu, D. R. Britt, D. G. Nocera and C. C. Cummins, On the Incompatibility of Lithium-O2 Battery Technology with CO2, Chem. Sci., 2017, 8, 6117–6122, 10.1039/c7sc01230f
.
- G. Nardi, I. Manet, S. Monti, M. A. Miranda and V. Lhiaubet-Vallet, Scope and Limitations of the TEMPO/EPR Method for Singlet Oxygen Detection: The Misleading Role of Electron Transfer, Free Radic. Biol. Med., 2014, 77, 64–70, DOI:10.1016/j.freeradbiomed.2014.08.020
.
- R. L. Donkers and M. S. Workentin, Elucidation of the Electron Transfer Reduction Mechanism of Anthracene Endoperoxides, J. Am. Chem. Soc., 2004, 126(6), 1688–1698, DOI:10.1021/ja035828a
.
- J. M. Aubry, C. Pierlot, J. Rigaudy and R. Schmidt, Reversible Binding of Oxygen to Aromatic Compounds, Acc. Chem. Res., 2003, 36(9), 668–675, DOI:10.1021/ar010086g
.
- P. F. Southern and W. A. Waters, 844. The Thermal Decomposition of Some Meso-Substituted Anthracene Photo-Oxides, J. Chem. Soc. (Resumed), 1960, 4340–4346, 10.1039/jr9600004340
.
- M. M. Ottakam Thotiyl, S. A. Freunberger, Z. Peng and P. G. Bruce, The Carbon Electrode in Nonaqueous Li-O2 Cells, J. Am. Chem. Soc., 2013, 135(1), 494–500, DOI:10.1021/ja310258x
.
- A. Sagadevan, K. C. Hwang and M. Su, Singlet Oxygen-Mediated Selective C-H Bond Hydroperoxidation of Ethereal Hydrocarbons, Nat. Commun., 2017, 8(1), 1812, DOI:10.1038/s41467-017-01906-5
.
|
This journal is © The Royal Society of Chemistry 2024 |
Click here to see how this site uses Cookies. View our privacy policy here.