DOI:
10.1039/D4QI01243G
(Research Article)
Inorg. Chem. Front., 2024,
11, 5336-5344
Temperature-tunable multiple dielectric switch in hybrid rare-earth perovskites regulated by hierarchical guest dynamics, lanthanide contraction and doping†
Received
17th May 2024
, Accepted 6th July 2024
First published on 8th July 2024
Abstract
Dielectric switches play an important role in electrical and electronic devices. Here we report a unique class of cage-like hybrid rare-earth perovskites, (i-PrNHMe2)2[RbLn(NO3)6] (Ln = La, Ce, Nd or Sm), capable of functioning as temperature-responsive multiple dielectric switches. The strategic incorporation of a conformationally flexible sphere-like guest (i-PrNHMe2)+ cation into the cage-like perovskite framework is in favor of the occurrence of hierarchical guest dynamics accompanied by stepwise phase transitions, leading to seldom observed multiple dielectric switching of “Low ↔ High ↔ Low ↔ High” upon heating. Furthermore, the utilizing of lanthanide contraction or rare-earth doping allows for fine tuning of the switching temperature. In addition, multiaxial ferroelasticity is found in these perovskites. Overall, this study provides a good approach for the design of temperature-tunable multiple dielectric switches.
Introduction
Dielectric switches, as a unique type of electrically polarizable materials, are capable of reversibly modulating an electrical signal between two distinct dielectric states triggered by external thermal, mechanical, or optical stimuli. Such a reconfigurable function renders them ideal candidates for use in smart stimulus-responsive system, sensing technology, and signal storage.1–10 While mechanically activated dielectric switches, known as piezoelectrics, have played crucial roles in contemporary electrical and electronic devices, their temperature-driven counterparts are less developed but have aroused a growing interest in recent years.11–24
From the perspective of polarization mechanism, temperature-driven dielectric switching in crystalline molecule-based materials usually stems from the variation of a dipole unit from frozen to thermally activated, or an alteration of its rotational dynamics that is often accompanied by a structural phase transition. The switching temperatures (Ts) in most of these switches are restrictively fixed at the phase transition temperatures. Nevertheless, for practical application, dielectric switches with multiple and/or adjustable Ts are more desirable yet challenging to achieve. To overcome this deficiency, we noticed that cage-like hybrid double perovskites (especially the rare-earth double perovskites),25–39 as a well-known class of phase-change compounds, may be good targets for the design of temperature-tunable multiple dielectric switches. As shown in Chart 1, three combined approaches may be implemented to afford such switches in the hybrid rare-earth perovskite system. Firstly, a guest cation with flexible and diverse conformations, regulated by different degrees of rotational energy barrier in the cage-confined space, is more preferable to exhibit hierarchical molecular dynamics. Also, a sphere-like characteristic is considerable, which is conducive to structural phase transition according to the “quasi-spherical theory” proposed by Xiong et al.40,41 Secondly, we aim to further tune the Ts through the lanthanide contraction effect of rare-earth ions, a systematic decrease in ionic radii across the lanthanide series.42–45 Thirdly, considering the similarity of lanthanide ions, an effortless solutionizing strategy, i.e., the doping of different rare-earth ions within these rare-earth perovskites, can also be employed to effectively tune the Ts.
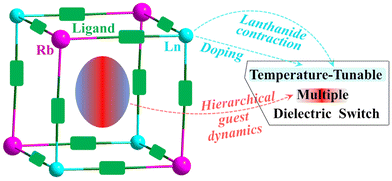 |
| Chart 1 Triply combined approach for the design of temperature-tunable multiple dielectric switch in cage-like hybrid rare-earth perovskites. | |
Till now, only one single phase transition at most was observed for the reported cage-like hybrid rare-earth perovskites.34–39 The reason may be due to the lack of conformational flexibility/diversity for the incorporated ammonium guests within these perovskites. Here we adopt (Me2HC–NHMe2)+, i.e. (i-PrNHMe2)+ cation, which exhibits a flexible “C–N” core with a highly variable torsion angle θH–N–C–H, as a conformationally flexible sphere-like guest,46–48 to induce stepwise phase transitions in the hybrid rare-earth perovskite system. With this strategy, we synthesized a series of hybrid rare-earth perovskites composed of trivalent rare-earth ions and monovalent alkali Rb+ ions, namely, (i-PrNHMe2)2[RbLn(NO3)6] (Ln = La, 1; Ce, 2; Nd, 3; Sm, 4). Further studies revealed that 1 is a two-step dielectric switch and the isomorphic 2–4 function as three-step dielectric switches due to the hierarchical guest dynamics, which are sensitively tuned by lanthanide contraction as well as lanthanide doping.
Results and discussion
Thermal analyses of 1–4
The thermogravimetric analyses of 1–4 show that they are stable up to at least 450 K (Fig. S1†). DSC measurement was used to detect the possible phase transitions. As shown in Fig. 1, the DSC curves of 1 exhibit a pair of endothermic/exothermic peaks at heating/cooling run, revealing that it undergoes a reversible phase transition at T1. For convenience, we label the phases below/above T1 as 1α/1γ phase, respectively. In contrast, two reversible phase transitions at T1 and T2 are recorded for all of the homogeneous 2–4. In other words, a metastable intermediate phase emerges in 2–4. For convenience, we label the phases of 2–4 below T1 as α phase, between T1 and T2 as β phase, and above T2 as γ phase. As shown in Table S1,† the phase transition temperatures of T1 for 1–4 are roughly similar (282–287 K for heating process), while those of T2 for 2–4 on heating run decrease from 258 K to 229 K, and further to 206 K. For all of these transitions, they belong to a first-order phase transition, with moderate thermal hysteresis of ΔT1 between the heating and cooling runs for 1–4 (ca. 3–4 K) and relatively large thermal hysteresis of ΔT2 for 2–4 (about 13, 20, and 26 K, respectively).
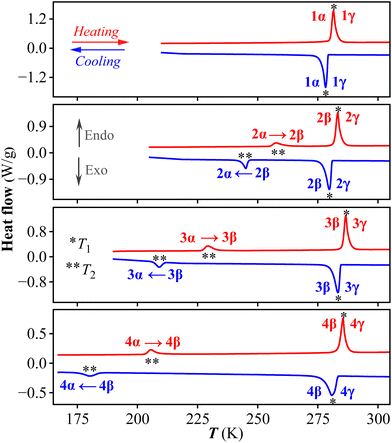 |
| Fig. 1 The DSC measurement of 1–4 in a heating–cooling cycle. | |
Stepwise phase transitions of 2 and its crystal structures in three phases
To understand the phase transitions detected by DSC measurement, single-crystal X-ray diffraction was performed for 1–4. It was uncovered that 1–4 are isomorphous and the last three have similar phase transition behavior (note: different to 2–4 which contain α/β/γ three phases, 1 only features α/γ two phases), hence here only the structure of 2 is discussed in detail as a representative. Compound 2 crystallizes in the space group C2/m at 173 K (α phase), R
m at 273 K (β phase), and Fm
m at 293 K (γ phase), respectively (Table S2†). The crystal structure of 2 can be roughly described as an organic–inorganic hybrid double perovskite, with a general formula of A2(B′B′′)Y6 (A = monovalent organic cation, B′/B′′ = monovalent/trivalent metal cation, arranged orderly and alternately over two types of B sublattices, and Y = monovalent anionic bridging ligand), which is topologically similar to (RM3HQ)2RbLa(NO3)6 reported by Zhang and Ye, et al.34 The Ce3+ center here is twelve-coordinated by six bidentate chelating NO3− ions, while the Rb+ ion is surrounded by six O atoms from six NO3− ions. For each NO3− ion, it bidentately chelates with one Ce3+ ion and further bridges with a Rb+ ion through its third O atom, which acts as an end-to-end bridging ligand between the Ce3+ and Rb+ ions. The interconnection of Ce3+ and Rb+ ions by the bridging NO3− ligands leads to a three-dimensional cage-like framework, with per (i-PrNHMe2)+ cation residing in a cage unit (Fig. 2). Each [Ce0.5Rb0.5(NO3)3]− cage unit is enclosed by twelve Ce–(NO3)–Rb fragments, with a length of ∼7.0 Å.
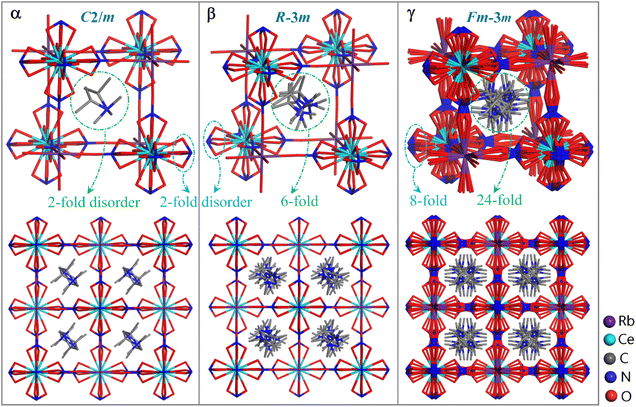 |
| Fig. 2 Cage units and packing diagrams of 2 at 173 K (α phase), 273 K (β phase), and 293 K (γ phase), respectively. The (i-PrNHMe2)+ and NO3− ions exhibit varying degrees of disorder in all phases. H atoms are omitted for clarity. | |
The driving force for the structural phase transition of 2 can mainly be attributed to the different degrees of disorder of the (i-PrNHMe2)+ and NO3− ions in its three phases. In 2α, 2β and 2γ phases, the (i-PrNHMe2)+ ion features 2-, 6- and 24-fold disorder, respectively, while the NO3− ion exhibits 2-, 2- and 8-fold disorder, respectively (Fig. 2). The synergic disorder of these components leads to symmetry breaking in the perovskite-like structure, with the total symmetric elements of the crystallographic point group increasing from 4 (E, C2, i, σh) in 2α phase to 12 (E, 2C3, 3C2, i, 2S6, 3σv) in 2β phase, and further to 48 (E, 8C3, 3C2, 6C′2, 6C4, i, 8S6, 3σh, 6σd, 6S4) in 2γ phase. Correspondingly, the occupancy factors for both Ce3+ and Rb+ ions in the three phases are reduced from 1/4 (2α phase) to 1/12 (2β phase), and further to 1/48 (2γ phase). Each pair of the 2-fold disordered (i-PrNHMe2)+ ions in 2α phase are related by a mirror symmetry, and six of the 6-fold disordered (i-PrNHMe2)+ ions in 2β phase are related by C3v symmetry, while twenty-four of the 24-fold disordered (i-PrNHMe2)+ ions in 2γ phase are related by Td symmetry. Overall, the organic–inorganic hybrid hetero-metallic perovskite structures in 2α, 2β and 2γ phases are characterized by symmetries of C2h, D3d and Oh, respectively.
Multiple dielectric switching of 2 and the related mechanism
The above-mentioned varied disordered state of the guest (i-PrNHMe2)+ ion in 2 upon thermal stimulation strongly signifies that it is swingable or rotatable in the cage-confined space, which should cause a sensitive dielectric response owing to its changing orientation polarization under an AC electric field. We thus performed the variable-temperature dielectric measurement on the powder-pressed pellet sample of 2, with its complex permittivity ε* (ε′ − iε′′, where ε′ and ε′′ are the real and imaginary parts, respectively) being measured over a frequency range from 0.5 to 1000 kHz. As shown in Fig. 3, from 100 to 340 K, the dielectric constants under all test frequencies switch from a dielectric-inactive region I (roughly below 153 K, 2α phase) to a dielectric-active region II (153–253 K, 2α phase), followed by another dielectric-inactive region III (253–285 K, 2β phase), and culminating in a new dielectric-active state IV (above 285 K, 2γ phase). It is worthy of note that the dielectric-inactive phenomena in regions I and III are distinct in essence (vide infra). In region II, the ε′ and ε′′ both show notable frequency dispersion, which can be explicated in terms of the dielectric relaxation of a swingable (i-PrNHMe2)+ ion. The peak maxima of ε′′ are recorded at the increasing temperatures (Tpeak) from 180 to 248 K for the increasing f “0.5 → 250 kHz”. Overall, a seldom observed pattern of multiple dielectric switching characterized by the transitions of “Low ↔ High ↔ Low ↔ High” was revealed in 2.
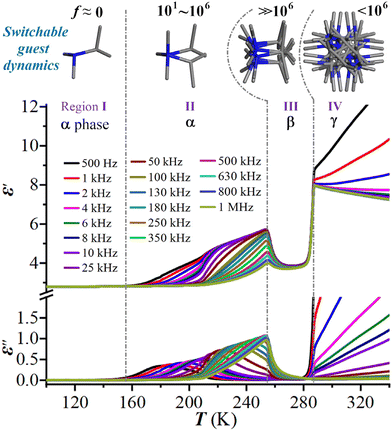 |
| Fig. 3 Temperature-dependent ε′ and ε′′ of 2 at various AC frequencies and the related switchable guest dynamics. | |
From the perspective of molecular dynamics, region I is indicative of a “frozen” state for the (i-PrNHMe2)+ ion, where its motion is significantly restricted. In contrast, region II corresponds to a swingable (i-PrNHMe2)+ ion between bistable states, exhibiting an increase in motion frequency from 101 to 106 as temperature rises. For region III, the (i-PrNHMe2)+ ion is in a more disordered, swing state, crystallographically characterized by 6-fold disorder. This state is marked by a motion frequency substantially exceeding 106, a rate too rapid to be responded by the applied AC frequency. Transitioning into region IV, the (i-PrNHMe2)+ ion enters a fully rotating state, distinguished crystallographically by a 24-fold disorder. At this stage, the alteration in motion mode results in an increased rotational energy barrier, consequently reducing the motion frequency to below 106. This change brings the (i-PrNHMe2)+ ion back to a dielectric responsible state.
To deeply characterize the dielectric relaxation in region II, the ε* was further measured as a function of f at a series of constant temperatures. The results uncover that there exists a single relaxation process, which can be fitted by a Havriliak–Negami equation:49ε* = ε∞ + (εs − ε∞)/{[1 + (iωτ)(1−α)]β} (0 ≤ α, β ≤ 1), where ω is the angular frequency, τ is the relaxation time, α/β are related to the distribution of relaxation time, and εs/ε∞ are the low/high-frequency limits of dielectric permittivity, respectively. The resultant fitted parameters (Table S6†) and fitted curves (Fig. 4a) match well with the experimental data.
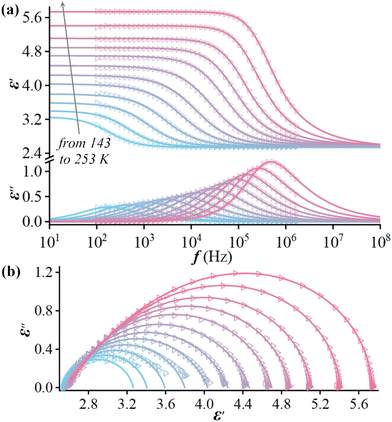 |
| Fig. 4 Frequency-dependent ε′ and ε′′ for 2 at selected temperatures from 143 to 253 K in an increasing step of 10 K (a), and the Cole–Cole diagrams are plotted as a series of arcs to show the relationship between ε′ and ε′′ (b). Solid curves represent the fit of the experimental data to the Havriliak–Negami equation. | |
The parameter ε∞, as the contribution of ionic polarization apart from electronic polarization, almost remains constant upon heating, suggesting that the electrostatic binding force between the cage-like [RbCe(NO3)6]2− framework and the embedded (i-PrNHMe2)+ ion changes little with rising temperature. The relaxation in region II is mainly attributed to the dipolar polarization of the dynamically disordered (i-PrNHMe2)+ ion. The increasing εs upon heating means that the continuously strengthened molecular thermal motion of the disordered (i-PrNHMe2)+ ion promotes the dipolar polarization. The value of α is roughly in the range of 0.1–0.2, suggesting a polarization mechanism with non-single relaxation time, and the value of β gradually deviates from 1.0 above 213 K can be mainly ascribed to the thermally enhanced interaction between the host [RbCe(NO3)6]2− framework and the guest (i-PrNHMe2)+ ion. Upon heating, the relaxation time τ turns short from 8.9 × 10−4 s at 143 K to 9.0 × 10−7 s at 243 K. The relaxation process can also be intuitively demonstrated by the so-called Cole–Cole formalisms (ε′ vs. ε′′) drawn in an Argand diagram, which are plotted as a series of arcs (Fig. 4b). The relaxation times τ obey the Arrhenius equation: 1/τ = ω0
exp[−Ea/(kBT)], where ω0 is a pre-exponential factor, Ea is an activation energy, and kB is the Boltzmann constant. By linearly fitting the plots of 1000/T vs. ln(1/τ) (Fig. S4†), an ω0 of 2.6 × 1010 s−1, and an Ea of 0.21 eV, were estimated for this relaxation. Such a relatively low energy barrier can mainly be ascribed the near-spherical (i-PrNHMe2)+ ion with a flexible “C–N” core.
Temperature-tunable dielectric switching regulated by lanthanide contraction or rare-earth doping
As implied in the above-mentioned DSC measurement, the Ts of the multiple dielectric switch 2 may be sensitively tuned by lanthanide contraction. Thus, a detailed comparison of the temperature-dependent multiple dielectric switches of 1–4 at various AC frequencies is performed and depicted in Fig. 5. With a steady decrease in the size (ionic radii) of the rare-earth ions from 1 to 4 (i.e., 1.061, 1.034, 0.0995, and 0.0964 Å for La3+, Ce3+, Nd3+, Sm3+, respectively), a dramatic confined effect is realized in such a hybrid rare-earth perovskite system. The temperature ranges of metastable β phase in 2–4 increase from 254–285 K to 218–289 K, and to 191–289 K. Overall, the utilizing of lanthanide contraction allows for the fine-tuning of Ts.
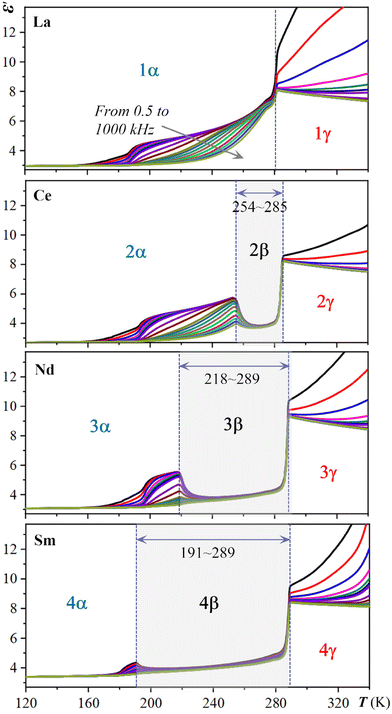 |
| Fig. 5 Comparison of the temperature-dependent multiple dielectric switches 1–4 at various AC frequencies. For display detail, see Fig. 3. | |
To further tune the Ts of the multiple dielectric switches within these hybrid rare-earth perovskites, exemplary lanthanide doping experiments between 1 and 3 are performed in a wide range of doping ratio. The PXRD measurements of these doping samples show that continuous solid solutions can be readily formed between them (Fig. S6†). The comparison of the DSC curves (Fig. S3†) as well as the variable-temperature dielectric spectra for 3 and its lanthanum-doped solid solutions (Fig. S4†) clearly illustrated that the phase transition temperature T1 in 3 can be continuously tuned via lanthanum doping (for instance, from 218 K in 3 to 266 K in (i-PrNHMe2)2[Rb(La0.5Nd0.5)(NO3)6], while the T2 has hardly changed). Such distinct effects can be explained as follows: in the high-temperature phase, the nitrate ligand is highly disordered with significant atomic thermal vibrations, which results in a relatively loose perovskite-like coordination framework, making the influence of rare-earth ion radii on the structure less noticeable. For the low-temperature phase, the atomic thermal vibrations of nitrate ligand are weakened substantially. Consequently, the perovskite-like coordination framework becomes more inflexible, which should be sensitively influenced by the size of rare-earth ion. Thus, the effect of lanthanide contraction or doping is more pronounced for the low-temperature phase than the high-temperature one.
Multiaxial ferroelasticity in 1–4
The phase transition from 1γ to 1α is theoretically ferroelastic, characterized by the Aizu notation “m
mF2/m”,50 with up to 12 equivalent ferroelastic directions. To investigate its ferroelastic characteristics, polarized light microscopy was employed to examine the variable-temperature evolution of the ferroelastic domain on a single crystal of 1. Due to the birefringence effect, the ferroelastic domain can exhibit contrasting light and dark patterns under orthogonally polarized light. As shown in Fig. 6a, in the paraelastic 1γ phase, no observable domains are present at 300 K. However, upon cooling to the 1α phase at 240 K, the image becomes darker, indicating the emergence of multiple domain patterns. Similarly, the transitions of β → α and γ → β in 2, 3 and 4 are also theoretically ferroelastic, with Aizu notations of “
mF2/m” and “m
mF
m”, respectively, and the number of equivalent ferroelastic directions for these transitions are 3 and 4, respectively. Fig. 6b–d demonstrate that in the paraelastic γ phase at 300 K, no domains are visible. Cooling to the β phase leads to the appearance of ferroelastic domains. Further cooling to the α phase darkens the image under the polarizing microscope owing to increased domain formation. Overall, the thermal reversibility of these domain patterns during a consecutive cooling–heating cycle confirms the presence of ferroelasticity in the α and β phases of these hybrid rare-earth perovskites.
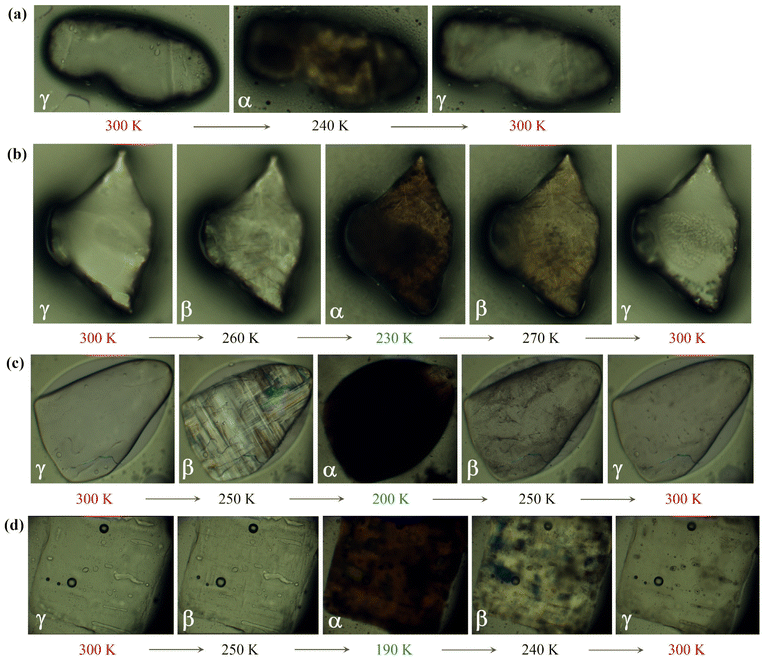 |
| Fig. 6 Variable-temperature evolution of the ferroelastic domains for 1 (a), 2 (b), 3 (c) and 4 (d), respectively, observed under polarization microscope. Crystal sizes: 1.4 × 0.4 × 0.2 mm for 1, 1.6 × 0.7 × 0.3 mm for 2, 2.2 × 1.3 × 0.5 mm for 3, and 2.5 × 2.3 × 0.3 mm for 4. | |
Experimental
Materials and methods
All chemicals were obtained from commercial sources and used without further purification. FT-IR spectra were recorded on a Nicolet 6700 FT-IR spectrometer using KBr pellets from 4000 to 400 cm−1. Powder X-ray diffraction (PXRD) patterns (Cu-Kα) were collected on a Rigaku MiniFlex600 θ–2θ diffractometer. Thermogravimetric analysis (TGA) was carried out on a TA Q50 system at a heating rate of 10 °C min−1 under a dinitrogen atmosphere. Differential scanning calorimeter (DSC) measurements were performed by heating and cooling the powder sample on a TA DSC 250 instrument, at a rate of 10 °C min−1. The complex permittivities were measured under a nitrogen gas atmosphere, using a Tonghui TH2828A LCR meter in a Mercury iTC cryogenic environment controller of Oxford Instrument, and the samples were ground and pressed into tablets under a pressure of 3 MPa. Energy dispersive X-ray spectroscopy (EDS) elemental mapping was carried out on a ZEISS EVO10 scanning electron microscope (SEM). Photoluminescence analysis was performed on an Edinburgh FLS980 fluorescence spectrometer.
Syntheses of 1–4
In a 50 mL beaker, RbNO3 (5.0 mmol), La(NO3)3·6H2O (5.0 mmol) and (i-PrNHMe2)NO3 (10.0 mmol) were dissolved in H2O (18 mL). The resultant clear solution was allowed to stand at room temperature. Three days later, colorless block-shaped crystals of 1 were deposited from the solution, in a ca. 87% yield based on La. IR data (KBr, cm−1): 3424(s), 2984(m), 2738(m), 2369(m), 1635(s), 1380(vs), 1335(s), 1156(m), 1039(m), 931(m), 826(s), 742(m). When a similar procedure was performed but with Ce(NO3)3·6H2O/Nd(NO3)3·6H2O/Sm(NO3)3·6H2O in place of La(NO3)3·6H2O, block-shaped crystals of 2/3/4 were obtained. The PXRD for the bulky crystals of them indicated that their experimental patterns match well with the simulated ones (Fig. S5†).
Syntheses of the molecular solid solutions of 1 and 3
The slow evaporation of the aqueous solution of 1 and 3 at 308 K, in the mixing ratios of x
:
(1
−
x), afforded the molecular solid solutions (i-PrNHMe2)2[Rb(LaxNd1−x)(NO3)6] in 100% yield, where x = 0.2, 0.35 and 0.5, respectively. The PXRD for these doping samples displayed that their experimental patterns match well with the patterns of 1 and 3 (Fig. S6†). The relative contents of La and Nd in these molecular solid solutions were semi quantitatively checked by SEM-EDS (Fig. S7†), showing that the ratios of La and Nd in them are roughly equivalent to the proportion of the starting experimental materials (for x = 0.20, 0.35, and 0.50, the corresponding xmapping are about 0.26, 0.40 and 0.55, respectively).
Luminescent property of 4
The solid-state luminescent spectra of 2–4 were investigated at room temperature. Under excitation of 403 nm, compound 4 displays the characteristic emission bands for the samarium(III) ion (Fig. S8†): two sharp emission bands at 561 nm (4G5/2 → 6H5/2) and 595 nm (4G5/2 → 6H7/2), respectively, and a middle emission band at 641 nm (4G5/2 → 6H9/2) as well as a weak band at 704 nm (4G5/2 → 6H11/2). For 2 and 3, only somewhat weak emissions were detected.
Single-crystal X-ray crystallography
The in situ variable-temperature single-crystal X-ray diffraction intensities for 2 at 293/273/173 K and those for 1/3/4 at 293 K were collected on a Rigaku synergistic diffractometer equipped with a graphite-monochromated Mo-Kα radiation (λ = 0.71073 Å), respectively. Absorption corrections were applied by using multi-scan program CrysAlisPro.51 The structures were solved by direct methods and refined using full-matrix least-squares technique with the SHELX program package.52 All hydrogen atoms were generated geometrically. Crystallographic data and structural refinements for 2 at 293/273/173 K, 1 at 293/223 K, and 3/4 at 293 K are summarized in Tables S2 and S3,† respectively. Selected bond lengths are listed in Tables S4 and S5,† respectively. CCDC numbers 2353399, 2353482–2353484, and 2353693–2353695† contain the supplementary crystallographic data for 1–4 in this paper. Note: the collection of low-temperature crystallographic data for 3 and 4 failed because the peaks of single-crystal diffraction became complicatedly multiple (from single to more than 6) after phase transition. This occurrence may be caused by the internal stress during the multiaxial ferroelastic phase transition, which gradually increased as the cage's size decreased progressively from 1 to 4.
Conclusion
In summary, our study on a series of unique cage-like hybrid rare-earth perovskites (i-PrNHMe2)2[RbLn(NO3)6] (Ln = La, Ce, Nd or Sm), has successfully demonstrated their potential as multiple dielectric switches with flexible temperature tunability. The confinement of a conformationally flexible sphere-like guest, the (i-PrNHMe2)+ cation, within the perovskite framework initiates hierarchical guest dynamics, facilitating stepwise phase transitions and enabling multiple switching states that alternate between “Low ↔ High ↔ Low ↔ High” with heating. The adaptability of the switching temperatures can be precisely modulated through lanthanide contraction and doping with different lanthanide ions, highlighting their prospective utility in responsive electronic devices. Furthermore, the observation of multiaxial ferroelasticity in these compounds adds to their functional versatility. Overall, the unique hybrid perovskite system in this study offers an interesting platform for the exploitation of temperature-tunable multiple dielectric switches.
Data availability
The data are available from the corresponding author on reasonable request. The ESI† contains the crystallographic data and characterization details for the title compounds.
Conflicts of interest
The authors declare no conflict of interest.
Acknowledgements
This work was supported by the National Natural Science Foundation of China (21971091 and 22071273), the Natural Science Foundation of Jiangxi Province (20224ACB203002), Guangzhou Science and Technology Programme (2024A04J6499), and Fundamental Research Funds for the Central Universities, Sun Yat-Sen University (23lgzy001).
References
-
Z. G. Ye, Handbook of Advanced Dielectric, Piezoelectric and Ferroelectric Materials, Woodhead Publishing Ltd, Cambridge, 2008 Search PubMed.
-
Y. Poplavko and Y. Yakymenko, Functional Dielectrics for Electronics: Fundamentals of Conversion Properties, Woodhead Publishing Ltd, Cambridge, 2020 Search PubMed.
- W. Zhang, H. Y. Ye, R. Graf, H. W. Spiess, Y. F. Yao, R. Q. Zhu and R. G. Xiong, Tunable and Switchable Dielectric Constant in an Amphidynamic Crystal, J. Am. Chem. Soc., 2013, 135, 5230 CrossRef CAS.
- J. Harada, M. Ohtani, Y. Takahashi and T. Inabe, Molecular Motion, Dielectric Response, and Phase Transition of Charge-Transfer Crystals: Acquired Dynamic and Dielectric Properties of Polar Molecules in Crystals, J. Am. Chem. Soc., 2015, 137, 4477 CrossRef CAS.
- N. F. Polizzi, M. J. Eibling, J. M. Perez Aguilar, J. Rawson, C. J. Lanci, H. C. Fry, D. N. Beratan, J. G. Saven and M. J. Therien, Photoinduced Electron Transfer Elicits a Change in the Static Dielectric Constant of a de Novo Designed Protein, J. Am. Chem. Soc., 2016, 138, 2130 CrossRef CAS.
- H. Y. Ye, W. Q. Liao, Q. Zhou, Y. Zhang, J. Wang, Y. M. You, J. Y. Wang, Z. N. Chen, P. F. Li, D. W. Fu, S. D. Huang and R. G. Xiong, Dielectric and ferroelectric sensing based on molecular recognition in Cu(1,10-phenlothroline)2SeO4·(diol) systems, Nat. Commun., 2017, 8, 14551 CrossRef CAS.
- W. Q. Liao, D. Zhao, Y. Y. Tang, Y. Zhang, P. F. Li, P. P. Shi, X. G. Chen, Y. M. You and R. G. Xiong, A molecular perovskite solid solution with piezoelectricity stronger than lead zirconate titanate, Science, 2019, 363, 1206 CrossRef CAS.
- Y. Zhang, X. J. Song, Z. X. Zhang, D. W. Fu and R. G. Xiong, Piezoelectric Energy Harvesting Based on Multiaxial Ferroelectrics by Precise Molecular Design, Matter, 2020, 2, 697 CrossRef.
- Y. Y. Tang, J. C. Liu, Y. L. Zeng, H. Peng, X. Q. Huang, M. J. Yang and R. G. Xiong, Optical Control of Polarization Switching in a Single-Component Organic Ferroelectric Crystal, J. Am. Chem. Soc., 2021, 143, 13816 CrossRef CAS PubMed.
- H. Y. Zhang, Y. Y. Tang, Z. X. Gu, P. Wang, X. G. Chen, H. P. Lv, P. F. Li, Q. Jiang, N. Gu, S. Ren and R. G. Xiong, Biodegradable ferroelectric molecular crystal with large piezoelectric response, Science, 2024, 383, 1492 CrossRef CAS.
- J. Y. Liu, S. Y. Zhang, Y. Zeng, X. Shu, Z. Y. Du, C. T. He, W. X. Zhang and X. M. Chen, Molecular Dynamics, Phase Transition and Frequency-Tuned Dielectric Switch of an Ionic Co-Crystal, Angew. Chem., Int. Ed., 2018, 57, 8032 CrossRef CAS PubMed.
- P. Meng, Y. Wu, Q. Zhang, G. Cheng, X. Wu and R. Zheng, Room Temperature Dielectric Pulse Effect in Organic Mixtures, Adv. Funct. Mater., 2018, 28, 1801421 CrossRef.
- T. Zhang, L. Chu, Z. Zhang, J. Li, W. Zhang, P. Shi, Q. Ye and D. W. Fu, Full-temperature covered switching material with triple optic-dielectric states in a lead-free hybrid perovskite, Sci. China Mater., 2020, 63, 2281 CrossRef CAS.
- Z. X. Zhang, C. Y. Su, J. Li, X. J. Song, D. W. Fu and Y. Zhang, Ferroelastic Hybrid Bismuth Bromides with Dual Dielectric Switches, Chem. Mater., 2021, 33, 5790 CrossRef CAS.
- Y. Wang, T. Zhang, M. M. Lun, F. L. Zhou, D. W. Fu and Y. Zhang, Halogen regulation triggers NLO and dielectric dual switches in hybrid compounds with green fluorescence, Inorg. Chem. Front., 2021, 8, 4230 RSC.
- Y. Gong, Z. Li, H. Li, W. Wu, W. Zhou, J. Zhao, C. He and M. Jiang, Ultra-Tough Room-Temperature Dielectric Switching Ionic Gels with Long-Cycle Stability, Adv. Funct. Mater., 2022, 32, 2207452 CrossRef CAS.
- T. Shao, H. F. Ni, C. Y. Su, Q. Q. Jia, L. Y. Xie, D. W. Fu and H. F. Lu, Integrated Reversible Thermochromism, High TC, Dielectric Switch and Narrow Band Gap in One Multifunctional Ferroic, Chem. – Eur. J., 2022, 28, e202202533 CrossRef CAS.
- M. Liberka, M. Zychowicz, J. Hooper, K. Nakabayashi, S. Ohkoshi and S. Chorazy, Synchronous Switching of Dielectric Constant and Photoluminescence in Cyanidonitridorhenate-Based Crystals, Angew. Chem., Int. Ed., 2023, 62, e202308284 CrossRef CAS.
- H. Pan, Z. Tian, M. Acharya, X. Huang, P. Kavle, H. Zhang, L. Wu, D. Chen, J. Carroll, R. Scales, C. J. G. Meyers, K. Coleman, B. Hanrahan, J. E. E. Spanier and L. W. W. Martin, Defect-Induced, Ferroelectric-Like Switching and Adjustable Dielectric Tunability in Antiferroelectrics, Adv. Mater., 2023, 35, 2300257 CrossRef CAS PubMed.
- Y. Gong, J. Zhao, Z. Li, J. Huang, Y. Zhang, L. Dong, C. Xiong and M. Jiang, Unparalleled Dielectric-Switching Effects Caused by Dual Polarization Synergy, Adv. Funct. Mater., 2023, 33, 2214544 CrossRef CAS.
- J. Guo, S. Y. Zhang, C. H. Zeng, Z. G. Zhou, M. Xie, Z. Y. Du, C. T. He, W. X. Zhang and X. M. Chen, Temperature-Tuned Variable Confined Space for Modulating Dipolar Polarization of a Disc-Shaped Ammonium Ion, J. Phys. Chem. Lett., 2023, 14, 8009 CrossRef CAS.
- W. J. Wei, H. Q. Gao, X. H. Luo and Y. Z. Tang, Dielectric Relaxation and Dielectric Switching Behaviors in (N,N-Diisopropylethylamine) Tetrachloroantimonate(III), Chem. – Eur. J., 2023, 29, e202202916 CrossRef CAS.
- T. Y. Ju, C. D. Liu, C. C. Fan, B. D. Liang, C. Y. Chai and W. Zhang, Halogen Substitution Regulates High Temperature Dielectric Switch in Lead-Free Chiral Hybrid Perovskites, Chem. – Eur. J., 2024, 30, e202303415 CrossRef CAS PubMed.
- P. Wang, X. Li, H. Ye, Q. Guan, Y. Wang, Y. Geng, C. Zhang, H. Li and J. Luo, Durable dielectric switching and photo-responsivity in a Dion-Jacobson hybrid perovskite semiconductor, Inorg. Chem. Front., 2024, 11, 2436 RSC.
- C. Shi, C. H. Yu and W. Zhang, Predicting and Screening Dielectric Transitions in a Series of Hybrid Organic-Inorganic Double Perovskites via an Extended Tolerance Factor Approach, Angew. Chem., Int. Ed., 2016, 55, 5798 CrossRef CAS.
- F. Wei, Z. Deng, S. Sun, F. Xie, G. Kieslich, D. M. Evans, M. A. Carpenter, P. D. Bristowe and A. K. Cheetham, The synthesis, structure and electronic properties of a lead-free hybrid inorganic-organic double perovskite (MA)2KBiCl6 (MA = methylammonium), Mater. Horiz., 2016, 3, 328 RSC.
- W. J. Xu, Z. Y. Du, W. X. Zhang and X. M. Chen, Structural phase transitions in perovskite compounds based on diatomic or multiatomic bridges, CrystEngComm, 2016, 18, 7915 RSC.
- W. Li, Z. Wang, F. Deschler, S. Gao, R. H. Friend and A. K. Cheetham, Chemically diverse and multifunctional hybrid organic-inorganic perovskites, Nat. Rev. Mater., 2017, 2, 16099 CrossRef.
- X. G. Zhao, D. Yang, J. C. Ren, Y. Sun, Z. Xiao and L. Zhang, Rational Design of Halide Double Perovskites for Optoelectronic Applications, Joule, 2018, 2, 1662 CrossRef CAS.
- X. Zhang, L. Li, Z. Sun and J. Luo, Rational chemical doping of metal halide perovskites, Chem. Soc. Rev., 2019, 48, 517 RSC.
- J. Gebhardt and A. M. Rappe, Mix and Match: Organic and Inorganic Ions in the Perovskite Lattice, Adv. Mater., 2019, 31, 1802697 CrossRef CAS.
- Y. Zhao, K. Cruse, M. Abdelsamie, G. Ceder and C. M. Sutter Fella, Synthetic approaches for thin-film halide double perovskites, Matter, 2021, 4, 1801 CrossRef CAS.
- J. Y. Lee, S. Ling, S. P. Argent, M. S. Senn, L. Canadillas Delgado and M. J. Cliffe, Controlling multiple orderings in metal thiocyanate molecular perovskites Ax{Ni[Bi(SCN)6]}, Chem. Sci., 2021, 12, 3516 RSC.
- C. Shi, J. J. Ma, J. Y. Jiang, M. M. Hua, Q. Xu, H. Yu, Y. Zhang and H. Y. Ye, Large Piezoelectric Response in Hybrid Rare-Earth Double Perovskite Relaxor Ferroelectrics, J. Am. Chem. Soc., 2020, 142, 9634 CAS.
- C. F. Wang, C. Shi, A. Zheng, Y. Wu, L. Ye, N. Wang, H. Y. Ye, M. G. Ju, P. Duan, J. Wang and Y. Zhang, Achieving circularly polarized luminescence and large piezoelectric response in hybrid rare-earth double perovskite by a chirality induction strategy, Mater. Horiz., 2022, 9, 2450 RSC.
- Q. Xu, L. Ye, L. P. Miao, C. Shi and Y. Zhang, H/F Substitution Induced Large Increase of TC in a 3D Hybrid Rare-Earth Double Perovskite Multifunctional Compound, Chem. – Eur. J., 2022, 28, e202103913 CrossRef CAS PubMed.
- Z. B. Hu, C. F. Wang, T. T. Sha, C. Shi, L. Ye, H. Y. Ye, Y. Song, Y. M. You and Y. Zhang, An Effective Strategy of Introducing Chirality to Achieve Multifunctionality in Rare-Earth Double Perovskite Ferroelectrics, Small Methods, 2022, 6, 2200421 CrossRef CAS.
- J. J. Ma, Q. Xu, L. Ye, Q. W. Wang, Z. X. Gong, C. Shi, H. Y. Ye and Y. Zhang, Structural phase transition and dielectric switching in an organic-inorganic hybrid rare-earth double perovskite-type compound: (DMP)2LaRb(NO3)6 (DMP = N,N-dimethylpyrrolidinium cation), J. Rare Earths, 2022, 40, 937 CrossRef CAS.
- Z. Y. Yue, L. Xiang, N. Wang, L. L. Zou, L. P. Miao, H. Y. Ye and C. Shi, Series of Cage-Like Double Perovskite-Type Hybrid Perovskites with Reversible Structural Phase Transition and Switchable Dielectric Constant Property, Cryst. Growth Des., 2023, 23, 6860 CrossRef CAS.
- H. Y. Zhang, Y. Y. Tang, P. P. Shi and R. G. Xiong, Toward the Targeted Design of Molecular Ferroelectrics: Modifying Molecular Symmetries and Homochirality, Acc. Chem. Res., 2019, 52, 1928 CrossRef CAS PubMed.
- H. Y. Liu, H. Y. Zhang, X. G. Chen and R. G. Xiong, Molecular Design Principles for Ferroelectrics: Ferroelectrochemistry, J. Am. Chem. Soc., 2020, 142, 15205 CrossRef CAS.
- M. Seitz, A. G. Oliver and K. N. Raymond, The lanthanide contraction revisited, J. Am. Chem. Soc., 2007, 129, 11153 CrossRef CAS PubMed.
- G. Ferru, B. Reinhart, M. K. Bera, M. O. de la Cruz, B. Qiao and R. J. Ellis, The Lanthanide Contraction beyond Coordination Chemistry, Chem. – Eur. J., 2016, 22, 6899 CrossRef CAS.
- G. S. Silva, J. D. L. Dutra, N. B. da Costa, S. Alves and R. O. Freire, Lanthanide Contraction in Lanthanide Organic Frameworks: A Theoretical and Experimental Study, J. Phys. Chem. A, 2020, 124, 7678 CrossRef CAS PubMed.
- R. B. Jordan, Lanthanide Contraction: What is Normal?, Inorg. Chem., 2023, 62, 3715 CrossRef CAS PubMed.
- S. Y. Zhang, X. Shu, Y. Zeng, Q. Y. Liu, Z. Y. Du, C. T. He, W. X. Zhang and X. M. Chen, Molecule-based nonlinear optical switch with highly tunable on-off temperature using a dual solid solution approach, Nat. Commun., 2020, 11, 2752 CrossRef CAS PubMed.
- H. Xiao, L. W. Ding, J. Y. Liu, Z. H. Jia, L. M. Cao, Z. Y. Du and C. T. He, Solid-state molecular dynamics of a torsion-variable ammonium embedded in a deformable supramolecular framework, Chin. J. Struct. Chem., 2023, 42, 100003 CrossRef.
- H. Xiao, W. Y. Hu, Q. Wang, C. H. Zeng, H. H. Li, H. M. Liu, Z. Y. Du and C. T. He, Molecular rotators anchored on a rod-like anionic coordination polymer adhered by charge-assisted hydrogen bonds, Phys. Chem. Chem. Phys., 2024, 26, 3974 RSC.
- S. Havriliak and S. Negami, A complex plane representation of dielectric and mechanical relaxation processes in some polymer, Polymer, 1976, 8, 161 CrossRef.
- K. Aizu, Possible Species of “Ferroelastic” Crystals and of Simultaneously Ferroelectric and Ferroelastic Crystals, J. Phys. Soc. Jpn., 1969, 27, 387 CrossRef CAS.
-
CrysAlisPro, Rigaku Oxford Diffraction, The Woodlands, TX, 2015 Search PubMed.
-
G. M. Sheldrick, SHELX 96 Program for Crystal Structure Determination, 1996 Search PubMed.
|
This journal is © the Partner Organisations 2024 |