DOI:
10.1039/D4CC01303D
(Feature Article)
Chem. Commun., 2024,
60, 4999-5009
Cascade ring expansion reactions for the synthesis of medium-sized rings and macrocycles
Received
21st March 2024
, Accepted 16th April 2024
First published on 18th April 2024
Abstract
This Feature Article discusses recent advances in the development of cascade ring expansion reactions for the synthesis of medium-sized rings and macrocycles. Cascade ring expansion reactions have much potential for use in the synthesis of biologically important medium-sized rings and macrocycles, most notably as they don’t require high dilution conditions, which are commonly used in established end-to-end macrocyclisation methods. Operation by cascade ring expansion method can allow large ring products to be accessed via rearrangements that proceed exclusively by normal-sized ring cyclisation steps. Ensuring that there is adequate thermodynamic driving force for ring expansion is a key challenge when designing such methods, especially for the expansion of normal-sized rings into medium-sized rings. This Article is predominantly focused on methods developed in our own laboratory, with selected works by other groups also discussed. Thermodynamic considerations, mechanism, reaction design, route planning and future perspective for this field are all covered.
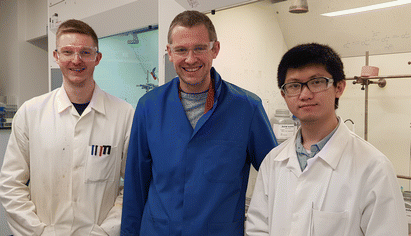
Jack M. Wootton, William P. Unsworth and Jerry K. F. Tam
| Jack M. Wootton completed his undergraduate degree at the University of York, before starting a PhD in the same institution to work with Dr Unsworth and GSK. Jack's PhD research is focused on the development of new ring expansion methods for large ring synthesis, the study of atroposelective acyl transfer reactions and computational chemistry. |
| Jerry K. F. Tam completed his undergraduate degree at the University of Cambridge, before moving to the University of York to complete a PhD with Dr Unsworth. Jerry's PhD research is focused on the development of new ring expansion methods for large ring synthesis, natural product synthesis and computational chemistry. |
| After a PhD in Oxford, William P Unsworth moved to the University of York for a PDRA in the group of Prof. Richard Taylor. In 2016 he started his independent career in York, where he is now a Senior Lecturer in Organic Chemistry. His current research interests include ring expansion approaches for the synthesis of medium-sized rings and macrocycles, catalyst selective synthesis and biocatalysis. Will also has won several prizes for his research, most notably the RSC Hickinbottom Award for his work on the synthesis of spirocycles and macrocycles. |
Introduction
Ring expansion reactions have enthralled synthetic organic chemists for decades. The eponymous ring expansion methods developed in the groups of Grob1 and Eschenmoser2 are arguably the most well-known, and these methods, alongside seminal studies by Hesse,3 Cookson4 and many others5 occupy prominent places in the annals of organic chemistry. One of the major appeals of ring expansion methods is their ability to enable non-obvious retrosynthetic disconnections through controlled rearrangement reactions. Such transformations are especially useful if they enable access to useful ring systems that are challenging to make by more conventional methods. Medium-sized rings (8–11-membered rings) and macrocycles (12+ membered rings) fall firmly into this category, as they are usually difficult to synthesise via classical end-to-end cyclisation methods; this is primarily due to competing intermolecular reactions, which often necessitates the use of impractical high dilution conditions.6 Large rings are important motifs in medicinal chemistry, where there is much current activity, hence improved methods to make them are of much interest.7,8
This Feature Article discusses recent advances in the development of synthetic strategies based on cascade ring expansion reactions. Particular emphasis is placed on methods developed in our own laboratory, in which the discovery of new cascade ring expansion methods for the synthesis of medium-sized rings and macrocycles is a major focus.9 We also cover selected works by other groups, especially when the work has helped to inspire the design of our own methods. Throughout the Feature Article, we tried to highlight the thermodynamic driving forces at play that help facilitate ring expansion; careful consideration of the driving force for rearrangement is important when designing any new cascade ring expansion reaction, and is especially important when targeting medium-sized ring products.10,11
Cascade ring expansion
Defining a ‘Cascade Ring Expansion’ reaction is not straightforward. We write this despite having used the term ourselves in published work,9e and the same phrase (or variants of it) used by several other groups to describe a range of ring expansion methods.12 Cascade reactions more broadly (also known as domino or tandem reactions) can be defined as ‘processes involving two or more consecutive reactions in which subsequent reactions result as a consequence of the functionality formed by bond formation or fragmentation in the previous step.13,14 As most ring expansion reactions involve a molecular rearrangement reaction of some kind, a reasonable argument can be made that almost all ring expansion reactions are also cascade processes! Agreement with this statement depends on your definition of what constitutes a new reaction within the cascade; for example, whether simple deprotonation reactions or protecting group cleavage steps that precede ring expansion should be classified as cascade processes.
We see little value in labouring exactly what constitutes a cascade reaction. However, we do think it is instructive to outline the most important reaction design features in which operation by a cascade helps to promote efficient rearrangement via ring expansion. Three strategies for cascade ring expansion are summarised generically in Scheme 1. Side chain insertion is the most common (Scheme 1A),15 with such reactions utilising cyclic starting materials, substituted with a linear side chain of general form 1. Ring expansion is typically enabled by activation of 1 by a chemical transformation to form a reactive intermediate (A) that is primed to undergo ring expansion, usually via nucleophilic attack of a reactive group on the side chain onto the ring, followed by fragmentation of the bridging bond highlighted in red. Alternatively, a bicyclic reactive intermediate (B) can be accessed by a cycloaddition reaction, before fragmenting via cleavage of the bridging bond (in red) to form a ring expanded product (3a + 3b → 4, Scheme 1B).16 Reactions in which overall cycloaddition is achieved asynchronously (i.e. non pericyclic reactions) are also known, with such reactions sharing features of both methods A and B.17 Finally, cyclisation/ring expansion cascades can be performed in which a linear precursor 5 undergoes cyclisation to form a cyclic reactive intermediate C, that goes on to rearrange via ring expansion (5 → 6, Scheme 1C).18 The key to achieving a successful ring expansion cascade by any of these general methods is to devise an efficient way to generate the reactive intermediate, and to design a system in which the fragmentation step is likely to be exergonic. This can be done by designing reactions that operate via high energy reactive intermediates (A, B or C), form stable products (2, 4, or 6), or both. Examples of each strategy are described in the following discussion, with the thermodynamic features that support ring expansion highlighted in all cases.
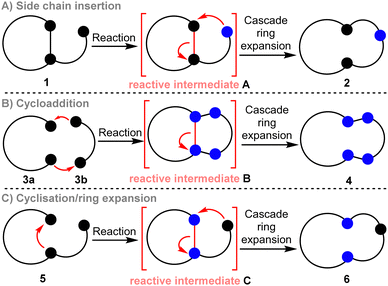 |
| Scheme 1 General strategies for cascade ring expansion. | |
Ring strain assisted cascade ring expansion
Relief of ring strain is a common strategy used to promote ring expansion. This is typically done through rearrangement reactions that exploit the chemical energy locked into strained 3- and 4-membered ring systems. For example, Donohoe and coworkers reported an ingenious 3- to 5-membered ring expansion of vinyl cyclopropanes 10b, with the approach summarised in Scheme 2.12b This method makes use of an Ir-catalysed hydrogen borrowing18 cascade, with radical fragmentation (10c → 10d) a central step in the ring expansion cascade.19 The end result is a powerful method for the synthesis of substituted cyclopentanes. Approaches such as this are less well-suited for the synthesis of medium-sized rings and macrocycles however; this is because the overall ring expansion operates by ring opening and subsequent ring closure (10d → 10e),20 and end-to-end cyclisation reactions tend to be inefficient for >7-membered ring formation, as outlined in the introduction.6
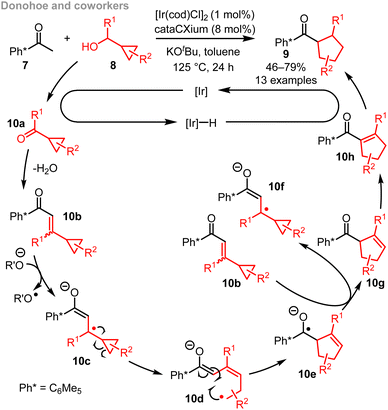 |
| Scheme 2 Vinyl cyclopropane ring expansion and hydrogen borrowing cascade. | |
There are several other ring expansion methods known in which fragmentation of strained 3-membered rings plays a central role, either by radical or 2-electron pathways.21,22 An instructive recent example is the method developed by Meng, Cao and co-workers, summarised in Scheme 3A.23a In this work, a gold(I) catalyst and triflic acid are used to co-catalyse a [4+2] cycloaddition reaction between alkynylbenzaldehydes 11 and strained cyclopropenes 12. The resulting reactive intermediate 14a is then very well set up to undergo ring expansion, considering that it is charged and contains a strained cyclopropane ring. Both of these features help to promote fragmentation via14b to form 7-membered ring products of the form 13, with charge stabilisation23 and strain relief both providing a driving force for ring expansion in this case.
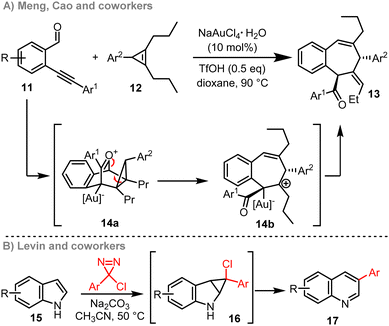 |
| Scheme 3 (A) Gold and TfOH co-catalyzed tandem [4+2] cycloaddition/ring expansion. (B) Carbon atom insertion into indoles promoted by chlorodiazirines. | |
Strain relief also plays an important role in enabling cascade ring expansion processes recently developed for molecular/skeletal editing.24 The work of Levin and co-workers to convert indoles 15 into quinolines 17 is a powerful example (Scheme 3B), with similar reactivity also able to convert pyrroles into pyridines (not shown).25 Both methods, which are inspired by the classical Ciamician–Dennstedt rearrangement,26 involve formation of a strained bicyclic reactive intermediate (16), with relief of ring-strain again providing a driving force for ring expansion, alongside re-aromatisation.27 As interest in molecular/skeletal editing methods continues to grow, cascade ring expansion reactions are very likely to play a key role in the development of this field.
Ring expansion cascade reactions can also be promoted by ring-opening of 4-membered ring reactive intermediates, with representative examples summarised in Scheme 4. For example, Carreira and coworkers reported an interesting cascade based on alkyne formation, [2+2] cycloaddition and electrocyclic ring opening (20c → 20d) to form 7–10-membered enones 21. Here, the chemical energy in cyclic alkyne 20b enables formation of the key 4-membered ring reactive intermediate 20c, which undergoes ring expansion in situ (Scheme 4A).12c In a recent report by Zhao and coworkers, an interesting ring expansion cascade that proceeds via Michael addition (to form 24a), cyclisation (24a → 24b) and fragmentation (24b → 25) is described (Scheme 4B).12e Another recent contribution was reported by Tu and co-workers, who describe an inventive double ring expansion cascade based on a Nazarov cyclisation (26 → 27a), followed by consecutive 1-atom ring expansions and tautomerisation to form tricyclic scaffold 28 (Scheme 4C).12f In all three cases, relief of ring-strain upon forming the ring expanded products is central to the success of the cascade, with strain-relief providing a thermodynamic driving force for rearrangement in all cases, either by fragmentation or migration.28
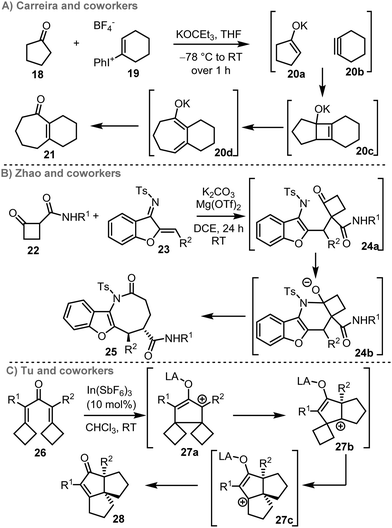 |
| Scheme 4 Ring expansion cascade reactions involving 4-membered ring fragmentation. LA – Lewis acid. | |
Successive ring expansion (SuRE) reactions
Some of the previous studies that helped inspire the cascade ring expansion reactions developed in our own laboratory are much older than those described in this Feature Article so far. As noted above in Scheme 1, side chain insertion is the most commonly used cascade ring expansion strategy, with carbonyl-containing substrates of the form 29 (Scheme 5A) finding the widest use. Such processes are typically achieved by conversion of a synthetic handle (Y) on the side chain of starting material 29 to a more reactive group (Z) to promote ring expansion (29a → 30). The side chain activation step can be done in several ways, including simple deprotonation,29 protecting group cleavage,30 enamine formation,31 other functional group interconversions32 and coupling reactions.33 The key fragmentation step requires cleavage of the C–X bond of 29a, with ‘X’ generally being a nitrogen atom,34 or a carbon able to stabilise negative charge.35 Two instructive examples from Hesse and coworkers are summarised in Schemes 5B and C.5a,36 Diketone 31 is converted into ring expanded lactone 33 by reduction and subsequent treatment with fluoride; in this case, ring expansion is driven by conversion of the cyclic ketone into a comparatively stable lactone group, in addition to formation of a stable α-nitro carbanion in the fragmentation step.36 The overall conversion of 6-membered ring 34 into 14-membered 36via an impressive double ring expansion cascade was especially informative in terms of our group's work, as it demonstrated the power of consecutive ring expansion reactions in forming larger ring products, without having to resort to end-to-end macrocyclisation at high dilution.37
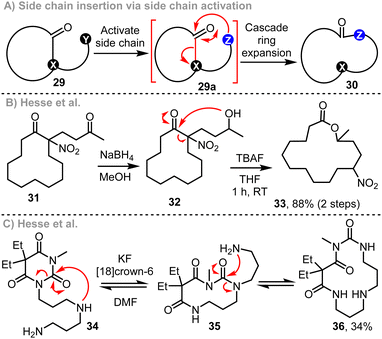 |
| Scheme 5 Side chain insertion cascade ring expansion reactions. | |
A representative example of the first cascade ring reaction expansion sequence developed in our lab is summarised in Scheme 6.9a The design of this reaction drew inspiration from both of the Hesse works in Scheme 5. Thus, starting from simple 12-membered ring cyclic β-ketoester 37, a side chain was added by a C-acylation reaction, using acid chloride Fmoc-β-ala-Cl to form 38. Treatment with piperidine then initiated a cascade reaction, starting with cleavage of the Fmoc protecting group to form amine 39a, followed by spontaneous cyclisation and fragmentation to form ring expanded product 40 in good overall yield. The formation of a stable amide bond, and ejection of a stabilised β-ketoester carbanion during the fragmentation step (39b → 40) are both important features of the reaction, designed to provide a strong thermodynamic driving force for ring expansion. This is especially important when using smaller cyclic starting materials, and means that the same method can be used to expand the analogous 5–8-membered cyclic β-ketoesters into medium-sized ring products; this is a more challenging transformation, in the view of the relatively high strain in medium-sized rings.10 Another integral design feature of the method is that the β-ketoester motif in the starting material 37 is regenerated in the product 40 (highlighted in yellow). This enables the product to participate in further ring expansion reactions following further iterations of the same method (40 → 41 → 42) – hence the ‘Successive Ring Expansion’ (SuRE) moniker used to describe this chemistry.
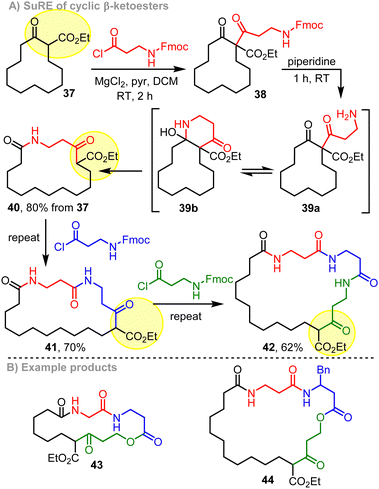 |
| Scheme 6 SuRE reactions of cyclic β-keto esters. | |
The same general method can also be used to prepare ring-expanded lactone products, by replacing the Fmoc-protected amine with a benzyl-protected alcohol, revealing the alcohol using hydrogenoloysis (not shown).9a Macrocyclic products 43 and 44 (Scheme 6B) illustrate the types of molecules that are accessible by mixing the lactam- and lactone-forming SuRE variants; both products were formed via 3 consecutive SuRE reaction iterations. The value of this method in making lead-like libraries for medicinal chemistry has also been demonstrated in our laboratory,9b and its use in the synthesis of natural product scaffold libraries was later reported in an excellent study by Tang and coworkers.38
Another instructive example of a side-chain insertion ring expansion cascade, developed by Houk, Duan, Guo and coworkers, is summarised in Scheme 7.12g In this reaction, cyclisation and activation of the starting material hydroxy ketone 45 is done using hydrogen peroxide under acidic conditions to form bicyclic intermediates 46. These bicyclic species are isolable, but the energy added to the system through the formation of this peroxide intermediate means that they can also be reacted further in a telescoped process. Thus, single electron reduction using a Cu(I) catalyst enables the formation of a reactive oxygen-centred radical 48b with sufficient energy to undergo β-scission to form radical 48c. This radical species can then take part in coupling in situ, and diverse functional groups (group ‘X’ in 47) can be installed by a radical relay strategy. The cascade is thought to operate via an unusual Cu(I)/Cu(II)/Cu(III) catalytic cycle, with the proposed mechanism supported by experimental and computational data.
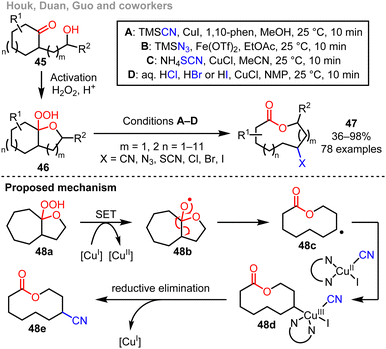 |
| Scheme 7 Radical-based side chain insertion ring expansion/functionalisation cascade. | |
In terms of our own work, a second generation SuRE approach was later developed based on the use of lactam starting materials in place of cyclic β-ketoesters.9c,d An example lactam SuRE sequence is shown in Scheme 8A. In this reaction, N-acylation of lactam starting material 49 affords an imide intermediate 51, that is primed to undergo cascade ring expansion following Fmoc protecting group cleavage to form 53. In this case, the rearrangement results in the overall functional group conversion of an imide and an amine into two amide bonds. The formation of two more stable functional groups in this way provides a clear thermodynamic driving force for ring expansion.
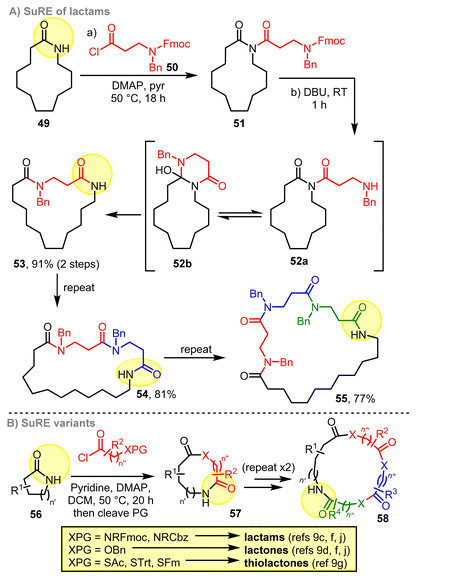 |
| Scheme 8 SuRE reactions of lactams. | |
The ‘successive’ element of this SuRE variant is enabled by the NH lactam group (highlighted in yellow) being regenerated during the ring expansion. The new NH lactam in the product can be used to perform further iterations of N-acylation and ring expansion (e.g.53 → 54 → 55). Around 100 examples of this class of ring expansion have been reported by our lab (Scheme 8B). Variants based on protected amines (to form lactams),9c alcohols9d and thiols9g have been developed, and used to effect 3- and 4-atom ring expansion of a range of lactam precursors. Computational methods have also been developed that enable reaction outcomes to be reliably predicted, based on the calculated ground state Gibbs free energies of the isomeric species in the ring expansion step (i.e.52a, 52b and 53 in the example in Scheme 8A).9f Lactam- and lactone-forming SuRE reactions have also been used to prepare analogues of the macrocyclic natural product solomonamide (not shown)9j and successfully applied to iminosugar derivatives.9n
Extensions to the SuRE method
More recent work in our group has resulted in the discovery of a series of extensions to the SuRE method that operate by similar principles, but allow access to different product classes and/or allow products to be made more efficiently.9h–lScheme 9A summarises our conjugate addition/ring expansion (CARE) cascade method.9h As with the SuRE method above, this approach starts with N-acylation of a lactam staring material, but in this case, using acryloyl chloride 60 (Scheme 9A). The resulting imide 61 can then react with various primary amines, to undergo efficient conjugation addition39 and form an intermediate amine 62a, that is primed to undergo ring expansion in situ, by the same rearrangement seen in the SuRE method (62a → 62b → 63). The scope of the method is similarly broad as it is for SuRE, with >50 examples of CARE reactions featuring in the first publication.9h The method is especially broad with respect to the primary amine reaction partner, with excellent functional group compatibility demonstrated. Another advantage of the CARE approach compared with SuRE is that it is protecting group-free, thus enabling large libraries of diversely functionalised medium-sized rings to be made quickly from commercially available amines. The reaction conditions are mild (RT in methanol) and the reaction is insensitive to air and moisture; indeed, the reaction even works using water as the solvent. As for SuRE, CARE reactions can be performed successively to allow access to larger ring products, with macrocycles 64a–c all having been prepared from Valero lactam following three iterations of the CARE sequence, using different amines for each iteration (Scheme 9B).
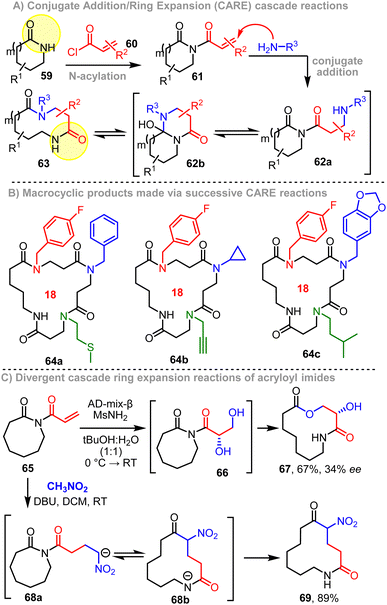 |
| Scheme 9 Cascade ring expansion reactions of acryloyl imides. | |
Two more reaction classes were later developed that further highlight the value of acryloyl imides as versatile substrates for use in ring expansion cascade reactions.9l In addition to undergoing CARE with amines to form lactams using the method summarised in Scheme 9A, lactones (e.g.67) and cyclic ketones (e.g.69) can also be prepared from common acryloyl imide precursors. For example, the reactions of acryloyl imide 65 with AD-mix-β using standard conditions40 enables smooth dihydroxylation (65 → 66) and ring expansion via a cascade process, to afford lactone 67 in good yield and with modest enantioselectivity. The same imide 65 can also be converted into cyclic ketone 69, via a CARE process using deprotonated nitromethane as the nucleophile. In this CARE variant, ring expansion is achieved with concomitant formation of two C–C bonds.
Our group has also developed related approaches to prepare medium-sized and macrocyclic sulfonamides,9i phosphonate esters and phosphonamidates.9k For example, a CARE approach similar to that described in Scheme 9A, can be used to convert cyclic vinyl sulfonamides 70 into medium-sized and macrocyclic sulfonamides 72via a conjugate addition and 4-atom ring expansion cascade (Scheme 10A).9i Alternatively, ring expanded cyclic sulfonamides can also be accessed by a cascade ring expansion process initiated by nitro reduction; for example, nosylation of lactam 73 followed by nitro reduction promotes ring expansion in situ to form 75 (Scheme 10B).9i Transformation of a comparatively reactive amine into a more stable amide group is thought to be the driving force for ring expansion in each case.
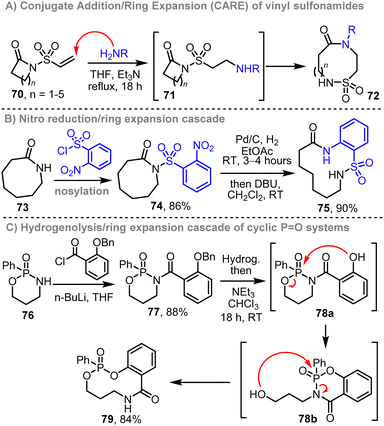 |
| Scheme 10 Cascade ring expansion reactions of SO2 and P O containing systems. | |
Similar logic can also be applied to the cascade ring expansion of P
O-containing systems.9k For example, N-acylation of phosphonamidate 76, followed by benzyl group cleavage by hydrogenolysis initiates a cascade ring expansion, in which the rearrangement of 78a into 78b was observed to take place ahead of ring expansion into 79. Accompanying computational studies support the notion that formation of a strong P–O bond at the expense of weaker P–N bond provides a driving force for ring expansion in this case. The synthesis of various other P
O containing rings is also reported as part of the same study (not shown).9k
Cyclisation/ring expansion cascade reactions of linear starting materials
This subsection relates to cascade ring expansion reactions of the type ‘C’ described in the introduction (Scheme 1C). Starting from a linear molecule and forming a ring that undergoes ring expansion in situ can bring notable advantages, especially if the initial cyclisation generates a cyclic reactive intermediate predisposed to undergo the desired rearrangement.41 An interesting example of such reactivity was reported by Zhou and Yeung, who have developed an innovative approach to make azepanes 82 from linear olefinic aziridines 80.12h This cascade is initiated by reaction of linear starting material 80 with NBS, to form a reactive bromonium ion 81a, which rearranges in situ via a cyclisation to form cyclic aziridinium ion 81b. This charged and strained reactive intermediate is then well set up to undergo a stereoselective ring opening to complete the cascade, with relief of ring strain and quenching of the positively charged nitrogen both likely to play an important role in enabling the key ring expansion step (Scheme 11).
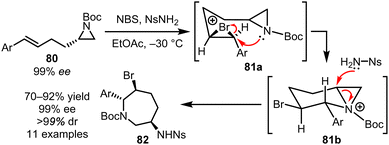 |
| Scheme 11 NBS induced cyclisation/ring expansion cascade of linear olefinic aziridines. | |
This method shares design features with a concept developed more recently in our own laboratory, based on a system of cyclisation/ring expansion (CRE) cascade reactions.9e,l At face value, the overall transformation depicted in Scheme 12A is very simple; i.e. a linear alcohol (81, X = O) or amine (81, X = NR/NH) is converted into a lactone or lactam (83) respectively by cyclisation. However, whilst for normal-sized ring synthesis such reactions are usually straightforward, this is rarely the case for medium-sized ring synthesis, where competing intermolecular reactions (dimerisation/polymerisation) often out-compete the desired cyclisation reaction. The main design feature of this cascade ring expansion reaction was to enable overall cyclisation to form medium-sized rings by a cascade reaction that avoids direct end-to-end cyclisation, and instead operates exclusively through normal-sized ring (5–7-membered) cyclisation reactions. This can be achieved via the strategic placement of pyridine/tertiary amine groups in the linear starting material that can act as internal nucleophilic catalysts. Thus, a linear carboxylic acid of the form 81 can be reacted with T3P to form an activated derivative 82a, that is primed to react with the internal pyridine/amine group highlighted in green, to form in this case a 6-membered ring intermediate 82b. This positively charged intermediate can then undergo ring expansion in situ following nucleophilic attack from a tethered alcohol or amine group (highlighted in blue). In this system, ring expansion is likely driven by neutralisation of the positive charge in reactive intermediate 82b.
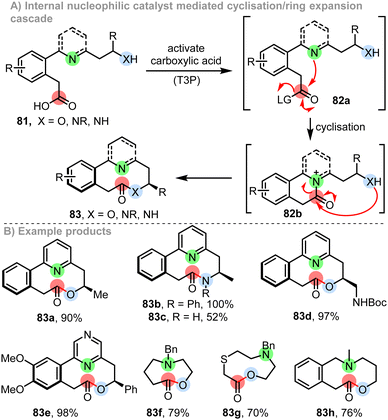 |
| Scheme 12 Internal nucleophilic catalyst mediated cyclisation/ring expansion cascades for the synthesis of medium-sized lactones and lactams. Coloured highlighting has been used to highlight the electrophilic component (pink), internal nucleophile (green) and terminal nucleophile (blue), with the new bond formed in the product drawn in red. | |
Various medium-sized ring lactones (e.g.83a, 83d–h) and lactams (e.g.83b, c) can be prepared in high yields using this method. The reactions are performed at RT under ambient conditions, are insensitive to air and moisture and do not require high dilution to proceed efficiently (≈0.1 M reaction concentration is typical). In terms of the biaryl products (83a–e), operation by a cascade brings an additional benefit of enabling a single atropisomer to be generated in the reaction; the point stereogenic centre in 82b imparts complete facial selectivity during the acyl transfer step, which manifests in the isolation of products 83a–e as the single atropisomers shown.9e
More recently, the CRE concept was extended to encompass a much wider range of reaction modes, with 9 distinct CRE reactions having now been demonstrated.9l For example, diamino alcohol 84 can be converted into thiocarbamate 86 in 83% yield upon reaction with thiophosgene at RT at 0.1 M reaction concentration (Scheme 13A). The reaction is thought to proceed by a CRE cascade, by initial cyclisation to form 5-membered ring cationic intermediate 84a, which expands in situ to afford the 9-membered ring product 86. Support for reaction via this intermediate is found in the fact that amino alcohol 85 – the analogous substrate but lacking the internal amine nucleophile – afforded none of the equivalent thiocarbamate 87 under the same conditions. Similarly, diol 88 can be converted smoothly into cyclic sulfite 100 upon reaction with thionyl chloride via cationic intermediate 88a, while diamine 102 can be converted into cyclic urea 104via cationic intermediate 102a; in the latter case, a reaction based on CO2 trapping and activation in situ was used. For all of these CRE modes (and indeed all new CRE modes published) control reactions have been performed that support operation by a cyclisation and subsequent ring expansion mechanism as proposed (Scheme 13A). The synthesis of ≈100 diversely functionalised medium-sized rings have been reported using CRE approaches to date,9e,l a selection of which are shown in Scheme 13B (106a–h).
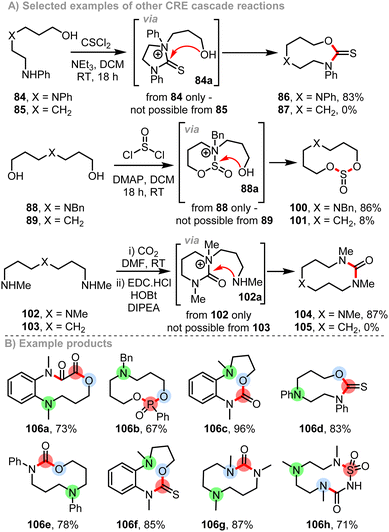 |
| Scheme 13 Alternative CRE reaction modes (A) and products accessible (B). Coloured highlighting has been used to highlight the electrophilic component (pink), internal nucleophile (green) and terminal nucleophile (blue), with the new bond formed in the product drawn in red. | |
To enable larger macrocyclic ring systems to be prepared using the same CRE concept, a further extension is summarised in Scheme 14. Through the incorporation of more than one internal nucleophile, larger rings can be made by adding additional ring expansion steps to the cascade, while still maintaining reaction solely via normal-sized ring cyclisation steps (Scheme 14A). The extended CRE method is exemplified by the reaction of linear starting material 113; in this case, activation of the carboxylic acid is proposed to initiate cyclisation (113 → 113a), followed by two successive ring expansion steps (113a → 113b → 114), to afford 14-membered ring lactone 114 in 73% yield, as the single atropisomer shown (Scheme 14B). Control reactions were again performed to validate the proposed CRE mechanism. An additional benefit to this approach is that in general, the linear starting materials can be prepared easily from simple building blocks; for example, 113 was prepared in 50% yield over 4 steps from simple starting materials 109–112 on gram-scale. The synthesis of 16 macrocyclic products has been demonstrated using extended CRE reactions to date, including the synthesis lactone 115, which operates via three consecutive ring expansion steps, to afford 17-membered ring macrocycle 115 in which 4 different heteroatoms have been incorporated within the ring.
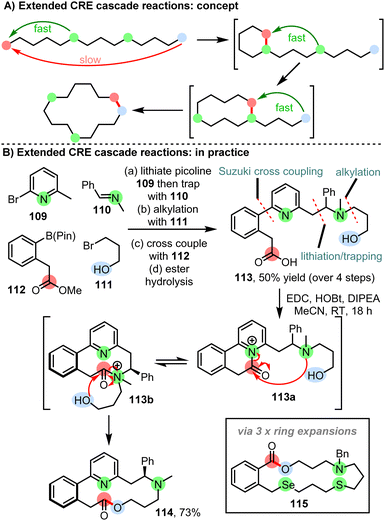 |
| Scheme 14 Extended CRE reactions. Coloured highlighting has been used to highlight the electrophilic component (pink), internal nucleophile (green) and terminal nucleophile (blue), with the new bond formed in the product drawn in red. | |
Conclusions
The wide array of methods described in this Feature article clearly demonstrate the utility of cascade ring expansion reactions for the preparation of diversely functionalised medium-sized rings and macrocycles. In the majority of cases, operation by a ring expansion enables the large ring products to be synthesised at standard reaction concentrations, thus avoiding high dilution – one of the big drawbacks of classical end-to-end macrocyclisation methods.
Ensuring that there is adequate thermodynamic driving force for ring expansion is a key challenge when designing new cascade ring expansion methods, especially for those in which the expansion of normal-sized rings into medium-sized rings is required. Another challenge that should not be overlooked is synthetic route design. When designing a synthetic route towards a medium-sized ring or macrocyclic target, typical retrosynthetic analysis using a disconnection approach naturally tends towards routes involving direct end-to-end cyclisation, the problems associated with which have been discussed above. Cascade ring expansion methods in contrast typically arise from non-obvious retrosynthetic disconnections. Thus, one of our major goals in writing this feature article is to encourage researchers involved in the synthesis of medium-sized rings and macrocycles to actively consider cascade ring expansion methods in their synthetic route planning.
In terms of future direction for this field, as the number of established methods grows, opportunities will inevitably arise in which different classes of cascade ring expansions can be combined and/or performed sequentially. For example, the two-step sequence summarised in Scheme 15, shows how linear starting material 116 was first converted into 9-membered ring 117 using our group's CRE method,9m and then expanded further by a conjugate addition/ring expansion cascade9i to afford macrocyclic sulfonamide 119 in 57% overall yield from 116. Combining compatible ring expansion approaches in this way will expand the range of accessible products even further, and exploring such possibilities will likely be a major focus in our laboratory in the future, based on methods developed both in our lab and others.
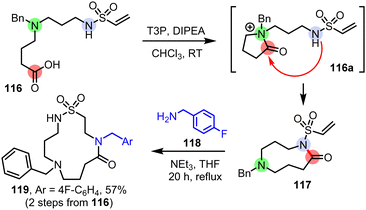 |
| Scheme 15 A sequential cascade ring expansion sequence combining two methods. | |
Author contributions
This Feature Article was researched, written and prepared though contributions from all authors, led by WPU.
Conflicts of interest
There are no conflicts to declare.
Acknowledgements
The authors would like to thank the University of York and the Wild Fund for supporting the PhD studentships of J. M. W. and J. K. F. T. We are also grateful to GSK for supporting the PhD studentship of J. M. W.
Notes and references
-
(a) C. A. Grob and W. Baumann, Helv. Chim. Acta, 1955, 38, 594 CrossRef CAS
;
(b) P. S. Wharton, J. Org. Chem., 1961, 26, 4781 CrossRef
.
- A. Eschenmoser and A. Frey, Helv. Chim. Acta, 1952, 35, 1660 CrossRef CAS
.
-
(a) U. Kramer, A. Guggisberg, M. Hesse and H. Schmid, Angew. Chem., Int. Ed. Engl., 1977, 16, 861 CrossRef
;
(b) U. Kramer, A. Guggisberg, M. Hesse and H. Schmid, Helv. Chim. Acta, 1978, 61, 1342 CrossRef CAS
;
(c) R. Wälchli and M. Hesse, Helv. Chim. Acta, 1982, 65, 2299 CrossRef
;
(d) R. Wälchli, A. Guggisberg and M. Hesse, Helv. Chim. Acta, 1984, 67, 2178 CrossRef
.
- R. C. Cookson and P. S. Ray, Tetrahedron Lett., 1982, 23, 3521 CrossRef CAS
.
- For reviews of ring expansion reactions, see:
(a)
M. Hesse, Ring Enlargement in Organic Chemistry, Wiley-VCH, Weinheim, 1991 Search PubMed
;
(b) W. P. Unsworth and J. R. Donald, Chem. – Eur. J., 2017, 23, 8780 CrossRef PubMed
;
(c) K. Prantz and J. Mulzer, Chem. Rev., 2010, 110, 3741 CrossRef CAS
;
(d) T. C. Stephens and W. P. Unsworth, Synlett, 2020, 133 CAS
;
(e) B. Biletskyi, P. Colonna, K. Masson, J.-L. Parrain, L. Commeiras and G. Chouraqui, Chem. Soc. Rev., 2021, 50, 7513–7538 RSC
.
-
(a) M. A. Casadei, C. Galli and L. Mandolini, J. Am. Chem. Soc., 1984, 106, 1051 CrossRef CAS
;
(b) A. Sharma, P. Appukkuttan and E. Van der Eycken, Chem. Commun., 2012, 48, 1623 RSC
;
(c) J. Fastrez, J. Phys. Chem., 1989, 93, 2635 CrossRef CAS
;
(d) J. C. Collins and K. James, Med. Chem. Commun., 2012, 3, 1489 RSC
;
(e) H. Kurouchi and T. Ohwada, J. Org. Chem., 2020, 85, 876 CrossRef CAS
;
(f) G. Illuminati and L. Mandolini, Acc. Chem. Res., 1981, 14, 95 CrossRef CAS
.
-
(a) E. M. Driggers, S. P. Hale, J. Lee and N. K. Terrett, Nat. Rev. Drug Discovery, 2008, 7, 608 CrossRef CAS
;
(b) E. Marsault and M. L. Peterson, J. Med. Chem., 2011, 54, 1961 CrossRef CAS
;
(c) F. Giordanetto and J. Kihlberg, J. Med. Chem., 2014, 57, 278 CrossRef CAS PubMed
;
(d) A. K. Yudin, Chem. Sci., 2015, 6, 30 RSC
;
(e) M. D. Cummings and S. Sekharan, J. Med. Chem., 2019, 62, 6843 CrossRef CAS
.
- For recent studies on the discovery of new macrocyclic antibiotics, see:
(a) I. B. Seiple, Z. Zhang, P. Jakubec, A. Langlois-Mercier, P. M. Wright, D. T. Hog, K. Yabu, S. R. Allu, T. Fukuzaki, P. N. Carlsen, Y. Kitamura, X. Zhou, M. L. Condakes, F. T. Szczypiński, W. D. Green and A. G. Myers, Nature, 2016, 533, 338 CrossRef CAS
;
(b) Q. Li and I. B. Seiple, J. Am. Chem. Soc., 2017, 139, 13304 CrossRef CAS PubMed
;
(c) M. I. McLaughlin and W. A. van der Donk, Biochem., 2020, 59, 343 CrossRef CAS PubMed
;
(d) R. Shukla, A. J. Peoples, K. C. Ludwig, S. Maity, M. G. N. Derks, S. De Benedetti, A. M. Krueger, B. J. A. Vermeulen, T. Harbig, F. Lavore, R. Kumar, R. V. Honorato, F. Grein, K. Nieselt, Y. Liu, A. M. J. J. Bonvin, M. Baldus, U. Kubitscheck, E. Breukink, C. Achorn, A. Nitti, C. J. Schwalen, A. L. Spoering, L. L. Ling, D. Hughes, M. Lelli, W. H. Roos, K. Lewis, T. Schneider and M. Weingarth, Cell, 2023, 186, 4059 CrossRef CAS PubMed
.
-
(a) C. Kitsiou, J. J. Hindes, P. I’Anson, P. Jackson, T. C. Wilson, E. K. Daly, H. R. Felstead, P. Hearnshaw and W. P. Unsworth, Angew. Chem., Int. Ed., 2015, 54, 15794 CrossRef CAS
;
(b) L. G. Baud, M. A. Manning, H. L. Arkless, T. C. Stephens and W. P. Unsworth, Chem. – Eur. J., 2017, 23, 2225 CrossRef CAS
;
(c) T. C. Stephens, M. Lodi, A. Steer, Y. Lin, M. Gill and W. P. Unsworth, Chem. – Eur. J., 2017, 23, 13314 CrossRef CAS PubMed
;
(d) T. C. Stephens, A. Lawer, T. French and W. P. Unsworth, Chem. – Eur. J., 2018, 24, 13947–13953 CrossRef CAS PubMed
;
(e) A. Lawer, J. A. Rossi-Ashton, T. C. Stephens, B. J. Challis, R. G. Epton, J. M. Lynam and W. P. Unsworth, Angew. Chem., Int. Ed., 2019, 58, 13942 CrossRef CAS
;
(f) A. Lawer, R. G. Epton, T. C. Stephens, K. Y. Palate, M. Lodi, E. Marotte, K. J. Lamb, J. K. Sangha, J. Lynam and W. P. Unsworth, Chem. – Eur. J., 2020, 26, 12674–12683 CrossRef CAS
;
(g) K. Y. Palate, R. G. Epton, A. C. Whitwood, J. M. Lynam and W. P. Unsworth, Org. Biomol. Chem., 2021, 19, 1404–1411 RSC
;
(h) K. Y. Palate, Z. Yang, A. C. Whitwood and W. P. Unsworth, RSC Chem. Biol., 2022, 3, 334–340 RSC
;
(i) Z. Yang, I. Zalessky, R. G. Epton, A. C. Whitwood, J. M. Lynam and W. P. Unsworth, Angew. Chem., Int. Ed., 2023, e202217178 CAS
;
(j) Z. Yang, C. R. B. Swanson and W. P. Unsworth, Synlett, 2023, 1694–1698 CAS
;
(k) Z. Yang, J. K. F. Tam, J. M. Wootton, J. M. Lynam and W. P. Unsworth, Chem. Commun., 2023, 59, 7927–7930 RSC
;
(l) W. E. Orukotan, K. Y. Palate, B. Pogrányi, P. Bobinski, R. G. Epton, L. Duff, A. C. Whitwood, G. Grogan, J. M. Lynam and W. P. Unsworth, Chem. – Eur. J., 2023, 29, e202303270 Search PubMed
;
(m) I. Zalessky, J. M. Wootton, J. K. F. Tam, D. E. Spurling, W. C. Glover-Humphreys, J. R. Donald, W. E. Orukotan, L. C. Duff, B. J. Knapper, A. C. Whitwood, T. F. N. Tanner, A. H. Miah, J. M. Lynam and W. P. Unsworth, J. Am. Chem. Soc., 2024, 146(8), 5702 CrossRef CAS PubMed
;
(n) Z. Yang, M. Arnoux, D. Hazelard, O. Hughes, J. Nabarro, A. C. Whitwood, M. A. Fascione, C. D. Spicer, P. Compain and W. P. Unsworth, Org. Biomol. Chem., 2024, 22, 2985–2991 RSC
.
- A. K. Clarke and W. P. Unsworth, Chem. Sci., 2020, 11, 2876 RSC
.
- K. C. Majumdar, RSC Adv., 2011, 1, 1152 RSC
.
-
(a) M. Yoshida, Y. Komatsuzaki, H. Nemoto and M. Ihara, Org. Biomol. Chem., 2004, 2, 3099 RSC
;
(b) S. Wübbolt, C. B. Cheong, J. R. Frost, K. E. Christensen and T. J. Donohoe, Angew. Chem., Int. Ed., 2020, 59, 11339 CrossRef
;
(c) C. Gampe, S. Boulos and E. Carreira, Angew. Chem., Int. Ed., 2010, 49, 4092 CrossRef CAS
;
(d) N. Arichi, K. Yamada, Y. Yamaoka and K. Takasu, J. Am. Chem. Soc., 2015, 137, 9579 CrossRef CAS PubMed
;
(e) J. Wang, J. Li and C. Zhao, Molecules, 2023, 28, 1650 CrossRef CAS
;
(f) Y. Wang, K. Fang, Y. Tu, J. Yin, Q. Zhao and T. Ke, Nat. Commun., 2022, 13, 2335 CrossRef CAS
;
(g) S. Liu, P. Ma, L. Zhang, S. Shen, H. Miao, L. Liu, K. N. Houk, X. Duan and L. Guo, Chem. Sci., 2023, 14, 5220 RSC
;
(h) J. Zhou and Y. Yeung, Org. Lett., 2014, 16, 2134 CrossRef CAS PubMed
.
- L. F. Tietze and U. Beifuss, Angew. Chem., Int. Ed. Engl., 1993, 32, 131 CrossRef
.
- For perspective on cascade, domino and tandem reactions, see:
(a) R. J. K. Taylor, M. Reid, J. Foot and S. A. Raw, Acc. Chem. Res., 2005, 38, 851 CrossRef CAS PubMed
;
(b) K. C. Nicolaou and J. S. Chen, Chem. Soc. Rev., 2009, 38, 2993 RSC
;
(c) B. Prabagar, N. Ghosh and A. K. Sahoo, Synlett, 2017, 2539 CAS
;
(d) J. M. Sperl and V. Sieber, ACS Catal., 2018, 8, 2385 CrossRef CAS
;
(e) N. Inprung, M. J. James, R. J. K. Taylor and W. P. Unsworth, Org. Lett., 2021, 23, 2063 CrossRef CAS
;
(f) H.-M. Huang, M. Garduño-Castro, C. Morrill and D. J. Procter, Chem. Soc. Rev., 2019, 48, 4626 RSC
.
-
(a) E. J. Corey, D. J. Brunelle and K. C. Nicolaou, J. Am. Chem. Soc., 1977, 99, 7359 CrossRef CAS
;
(b) G. H. Posner, M. A. Hatcher and W. A. Maio, Tetrahedron, 2016, 72, 6025 CrossRef CAS
;
(c) A. Borchardt and W. C. Still, Synlett, 1995, 539 CrossRef CAS
;
(d) G. A. Molander, Y. Le Huérou and G. A. Brown, J. Org. Chem., 2001, 66, 4511 CrossRef CAS PubMed
;
(e) J. Tummatorn and G. B. Dudley, Org. Lett., 2011, 13, 1572 CrossRef CAS PubMed
.
- M. Ikeda, K. Ohno, M. Takahashi, K.-I. Homma, T. Uchino and Y. Tamura, Heterocycles, 1983, 20, 1005 CrossRef CAS
. See also ref. 24 and the associated discussion on skeletal editing reactions, several of which are promoted by cycloaddition reactions.
-
(a) H. Stach and M. Hesse, Helv. Chim. Acta, 1986, 69, 85 CrossRef CAS
;
(b) M. R. Kling, G. McNaughton-Smith and R. J. K. Taylor, J. Chem. Soc., Chem. Commun., 1993, 1593 RSC
;
(c) Y. Zou, C. Ding, L. Zhou, Z. Li, Q. Wang, F. Schoenebeck and A. Goeke, Angew. Chem., Int. Ed., 2012, 51, 5647 CrossRef CAS PubMed
;
(d) Y. Zhou, Y.-L. Wei, J. Rodriguez and Y. Coquerel, Angew. Chem., Int. Ed., 2019, 58, 456 CrossRef CAS
;
(e) Y. Shiratori, J. Jiang, K. Kubota, S. Maeda and H. Ito, J. Am. Chem. Soc., 2024, 146(3), 1765 CrossRef CAS PubMed
.
- For perspective on hydrogen borrowing reactions such as this one, see: B. G. Reed-Berendt, D. E. Latham, M. B. Dambatta and L. C. Morrill, ACS Cent. Sci., 2021, 7, 570 CrossRef CAS PubMed
.
- Radical cascade reactions have a long and important history, with several examples involving ring expansion. For review on radical ring expansion reactions, see Section 4 of reference 5b and:
(a) L. Yet, Tetrahedron, 1999, 55, 9349 CrossRef
;
(b) P. Dowd and W. Zhang, Chem. Rev., 1993, 93, 2091 CrossRef CAS
;
(c) T. Singha, A. R. S. Mondal, S. Midya and D. P. Hari, Chem. – Eur. J., 2022, 28, e202202025 CrossRef CAS PubMed
For selected recent examples see: ;
(d) Z.-L. Li, X.-H. Li, N. Wang, N.-Y. Yang and X.-Y. Liu, Angew. Chem., Int. Ed., 2016, 55, 15100 CrossRef CAS PubMed
;
(e) L. Li, Z.-L. Li, F.-L. Wang, Z. Guo, Y.-F. Cheng, N. Wang, X.-W. Dong, C. Fang, J. Liu, C. Hou, B. Tan and X.-Y. Liu, Nat. Commun., 2016, 7, 13852, DOI:10.1038/ncomms13852
;
(f) L. Li, Z.-L. Li, Q.-S. Gu, N. Wang and X.-Y. Liu, Sci. Adv., 2017, 3, e1701487 CrossRef PubMed
;
(g) N. Wang, Q.-S. Gu, Z.-L. Li, Z. Li, Y.-L. Guo, Z. Guo and X.-Y. Liu, Angew. Chem., Int. Ed., 2018, 57, 14225 CrossRef CAS PubMed
;
(h) N. Wang, J. Wang, Y.-L. Guo, L. Li, Y. Sun, Z. Li, H.-X. Zhang, Z. Guo, Z.-L. Li and X.-Y. Liu, Chem. Commun., 2018, 54, 8885 RSC
;
(i) Z. Xu, Z. Huang, Y. Li, R. Kuniyil, C. Zhang, L. Ackermann and Z. Ruan, Green Chem., 2020, 22, 1099 RSC
;
(j) T. Singha, G. A. Kadam and D. P. Hari, Chem. Sci., 2023, 14, 6930 RSC
;
(k) D. C. Harrowven, N. L’Helias, J. D. Moseley, N. J. Blumirea and S. R. Flanaganan, Chem. Commun., 2003, 2658 RSC
.
- For other ring expansion methods that operate via ring opening and subsequent re-closure to form larger rings, see:
(a) C. J. White, J. L. Hickey, C. C. G. Scully and A. K. Yudin, J. Am. Chem. Soc., 2014, 136, 3728 CrossRef CAS PubMed
;
(b) E. E. Hyland, P. Q. Kelly, A. M. McKillop, B. D. Dherange and M. D. Levin, J. Am. Chem. Soc., 2022, 144, 19528 CrossRef PubMed
.
- For perspective on ring expansion reactions enabled by 3-membered ring fragmentation, see ref. 5e, and:
(a) D. J. Mack and J. T. Njardarson, ACS Catal., 2013, 3, 272 CrossRef CAS
;
(b) T. Schleif, Chem. – Eur. J., 2022, 28, e202201775 CrossRef CAS PubMed
.
- For related perspective on homologation reactions, see:
(a) S. M. Kohlbacher, V.-S. Ionasz, L. Ielo and V. Pace, Monatsh. Chem., 2019, 150, 2011 CrossRef CAS
;
(b) N. R. Candeias, R. Paterna and P. M. P. Gois, Chem. Rev., 2016, 116, 2937 CrossRef CAS PubMed
;
(c) Chapter 19 of V. Pace (Ed.) Homologation Reactions: Reagents, Applications, and Mechanisms, Wiley-VCH, Weinheim, 2023. ISBN:9783527830237.
-
(a) X. Meng, M. Guo, J. Zhu, H. Zhu, X. Sun, L. Tian and Z. Cao, Eur. J. Org. Chem., 2019, 1952–1956 CrossRef CAS
. For other ring expansion methods in which stabilisation of charged reactive intermediates plays a role, see: ;
(b) J. E. Hall, J. V. Matlock, J. W. Ward, K. V. Gray and J. Clayden, Angew. Chem., Int. Ed., 2016, 55, 11153 CrossRef CAS PubMed
;
(c) R. Costil, Q. Lefebvre and J. Clayden, Angew. Chem., Int. Ed., 2017, 56, 14602 CrossRef CAS PubMed
.
- For general perspective on molecular/skeletal editing, see:
(a) J. Jurczyk, J. Woo, S. F. Kim, B. D. Dherange, R. Sarpong and M. D. Levin, Nat. Synth., 2022, 1, 352 CrossRef PubMed
. For recent examples involving ring expansion, see: ;
(b) Z. Liu, P. Sivaguru, Y. Ning, Y. Wu and X. Bi, Chem. – Eur. J., 2023, 29, e202301227 CrossRef CAS PubMed
;
(c) P. Q. Kelly, A. S. Filatov and M. D. Levin, Angew. Chem., Int. Ed., 2022, 61, e202213041 CrossRef CAS PubMed
;
(d) E. Boudry, F. Bourdreux, J. Marrot, X. Moreau and C. Ghiazza, J. Am. Chem. Soc., 2024, 146(4), 2845 CrossRef CAS PubMed
;
(e) J. C. Reisenbauer, O. Green, A. Franchino, P. Finkelstein and B. Morandi, Science, 2022, 377, 1104 CrossRef CAS PubMed
;
(f) P. Finkelstein, J. C. Reisenbauer, B. B. Botlik, O. Green, A. Florin and B. Morandi, Chem. Sci., 2023, 14, 2954 RSC
;
(g) B. W. Joynson, G. R. Cumming and L. T. Ball, Angew. Chem., Int. Ed., 2023, 62, e202305081 CrossRef PubMed
;
(h) H. Wang, H. Shao, A. Das, S. Dutta, H. T. Chan, C. Daniliuc, K. N. Houk and F. Glorius, Science, 2023, 381, 75 CrossRef CAS PubMed
.
- B. D. Dherange, P. Q. Kelly, J. P. Liles, M. S. Sigman and M. D. Levin, J. Am. Chem. Soc., 2021, 143, 11337 CrossRef CAS PubMed
.
- G. L. Ciamician and M. Dennstedty, Ber. Dtsch. Chem. Ges., 1881, 14, 1153 CrossRef
.
- For other reactions in which aromatisation provides a driving force for overall ring expansion, see:
(a) R. A. Bauer, T. A. Wenderski and D. S. Tan, Nat. Chem. Biol., 2013, 9, 21 CrossRef CAS PubMed
;
(b) T. Guney, T. A. Wenderski, M. W. Boudreau and D. S. Tan, Chem. – Eur. J., 2018, 24, 13150 CrossRef CAS PubMed
.
- For other ring expansion cascade reactions based on 4-membered ring opening, see ref. 12a, 12d, 18b, 21a, 21b and: W. Zhao, H. Qian, Z. Li and J. Sun, Angew. Chem., Int. Ed., 2015, 54, 10005 CrossRef CAS PubMed
.
-
(a) E. Stephanou, A. Guggisberg and M. Hesse, Helv. Chim. Acta, 1979, 62, 1932 CrossRef CAS
;
(b) U. Kramer, A. Guggisberg, M. Hesse and H. Schmid, Angew. Chem., Int. Ed. Engl., 1978, 17, 200 CrossRef
;
(c) Y. Nakashita and M. Hesse, Angew. Chem., 1981, 93, 1077 CrossRef CAS
;
(d) Y. Nakashita and M. Hesse, Helv. Chim. Acta, 1983, 66, 845 CrossRef CAS
.
-
(a) M. M. Shemyakin, V. K. Antonov, A. M. Shkrob, V. I. Shchelokov and Z. E. Agadzhanyan, Tetrahedron, 1965, 21, 353 CrossRef
;
(b) R. Mendoza-Sanchez, V. B. Corless, Q. N. N. Nguyen, M. Bergeron-Brlek, J. Frost, S. Adachi, D. J. Tantillo and A. K. Yudin, Chem. – Eur. J., 2017, 23, 13319 CrossRef CAS PubMed
;
(c) J. Shang, V. J. Thombare, C. L. Charron, U. Wille and C. Hutton, Chem. – Eur. J., 2021, 27, 1620 CrossRef CAS PubMed
.
- S. Bienz and M. Hesse, Helv. Chim. Acta, 1988, 71, 1704 CrossRef CAS
.
- B. M. Trost and J. E. Vincent, J. Am. Chem. Soc., 1980, 102, 5680 CrossRef CAS
.
-
(a) R. Wälchli, A. Guggisberg and M. Hesse, Tetrahedron Lett., 1984, 21, 2205 CrossRef
;
(b) D. R. Loya, A. Jean, M. Cormier, C. Fressigné, S. Nejrotti, J. Blanchet, J. Maddaluno and M. De Paolis, Chem. – Eur. J., 2018, 24, 2080 CrossRef CAS PubMed
;
(c) A. Dierks, J. Tönjes, M. Schmidtmann and J. Christoffers, Chem. – Eur. J., 2019, 25, 14912 CrossRef CAS PubMed
.
-
(a) G. I. Glover, R. B. Smith and H. Rapoport, J. Am. Chem. Soc., 1965, 87, 2003 CrossRef CAS
;
(b) J. M. Muchowski, Can. J. Chem., 1970, 48, 1946 CrossRef CAS
;
(c) A. N. Chulin, I. L. Rodionov, L. K. Baidakova, L. N. Rodionova, T. A. Balashova and V. T. Ivanov, J. Peptide Sci., 2005, 11, 175 CrossRef CAS PubMed
.
- See reference 3b, 3c and:
(a) R. Wälchli, S. Bienz and M. Hesse, Helv. Chim. Acta, 1985, 68, 484 CrossRef
;
(b) S. Bienz, A. Guggisberg, R. Wälchli and M. Hesse, Helv. Chim. Acta, 1988, 71, 1708 CrossRef CAS
;
(c) V. I. Ognyanov, Helv. Chim. Acta, 1989, 72, 1522 CrossRef CAS
;
(d) T. Ohnuma, M. Nagasaki, M. Tabe and Y. Ban, Tetrahedron Lett., 1983, 24, 4253 CrossRef CAS
;
(e) K. Oda, T. Ohnuma and Y. Ban, J. Org. Chem., 1984, 49, 953 CrossRef CAS
;
(f) B. Moon, S. Han, Y. Yoon and H. Kwon, Org. Lett., 2005, 7, 1031 CrossRef CAS PubMed
.
- S. Stanchev and M. Hesse, Helv. Chim. Acta, 1989, 72, 1052 CrossRef CAS
.
- This class of reaction developed in the Hesse group was termed a ‘Zip reaction’. For other examples of Zip reactions, see ref. 3a–c and 5a.
- C. Zhao, Z. Ye, Z.-X. Ma, S. A. Wildman, S. A. Blaszczyk, L. Hu, I. A. Guizei and W. Tang, Nat. Commun., 2019, 10, 4015 CrossRef PubMed
.
- For a conceptually cascade conjugate addition/lactamisation process, see: G. J. Noordzij and C. H. R. M. Wilsens, Front. Chem., 2019, 729 CrossRef CAS PubMed
.
- H. C. Kolb, M. S. VanNieuwenhze and K. B. Sharpless, Chem. Rev., 1994, 94, 2483 CrossRef CAS
.
- B. Zhou, L. Li, X.-Q. Zhu, J.-Z. Yan, Y.-L. Guo and L.-W. Ye, Angew. Chem., Int. Ed., 2017, 56, 4015 CrossRef CAS PubMed
.
Footnote |
† These authors contributed equally to this manuscript. |
|
This journal is © The Royal Society of Chemistry 2024 |
Click here to see how this site uses Cookies. View our privacy policy here.