DOI:
10.1039/D3BM01934A
(Review Article)
Biomater. Sci., 2024,
12, 1425-1448
Applications, advancements, and challenges of 3D bioprinting in organ transplantation
Received
27th November 2023
, Accepted 6th February 2024
First published on 20th February 2024
Abstract
To date, organ transplantation remains an effective method for treating end-stage diseases of various organs. In recent years, despite the continuous development of organ transplantation technology, a variety of problems restricting its progress have emerged one after another, and the shortage of donors is at the top of the list. Bioprinting is a very useful tool that has huge application potential in many fields of life science and biotechnology, among which its use in medicine occupies a large area. With the development of bioprinting, advances in medicine have focused on printing cells and tissues for tissue regeneration and reconstruction of viable human organs, such as the heart, kidneys, and bones. In recent years, with the development of organ transplantation, three-dimensional (3D) bioprinting has played an increasingly important role in this field, giving rise to many unsolved problems, including a shortage of organ donors. This review respectively introduces the development of 3D bioprinting as well as its working principles and main applications in the medical field, especially in the applications, and advancements and challenges of 3D bioprinting in organ transplantation. With the continuous update and progress of printing technology and its deeper integration with the medical field, many obstacles will have new solutions, including tissue repair and regeneration, organ reconstruction, etc., especially in the field of organ transplantation. 3D printing technology will provide a better solution to the problem of donor shortage.
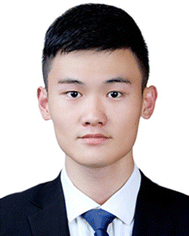 Guobin Huang | Guobin Huang is studying for his master's degree at the Institute of Organ Transplantation, Tongji Hospital, Tongji Medical College, Huazhong University of Science and Technology. The main topic of his research is the combination of biomaterials and organ/tissue regeneration. His aim is to develop advanced biomaterials for stem cell based clinical applications in tissue engineering and regenerative medicine. |
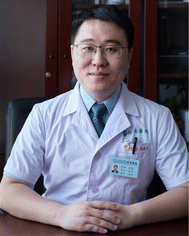 Bo Yang | Bo Yang received his PhD from the Institute of Organ Transplantation, Tongji Hospital, Tongji Medical College, Huazhong University of Science and Technology. He was a visiting scholar at the University of Pittsburgh from 2015 to 2016. He is now the doctor-in-charge of the Institute of Organ Transplantation, Tongji Hospital. Tissue/organ regeneration of post-transplantation injuries based on stem cell technology and the mechanism of the pathogenesis of liver transplantation complications are his main basic research interests and he aims to find out more effective methods for tissue/organ regeneration. |
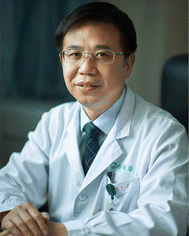 Zhishui Chen | Zhishui Chen received his PhD from the Institute of Organ Transplantation, Tongji Hospital, Tongji Medical College, Huazhong University of Science and Technology. As a chief physician and professor specializing in organ transplantation, he has been performing scientific research on the topics of liver transplantation complications and transplantation immunity, and the combination of biomaterials and organ/tissue regeneration. He aims to find out the mechanism of pathogenesis of liver transplantation complications and develop a more effective approach to improve tissue/organ regeneration based on stem cell based technology and tissue engineering. |
1. Introduction
Human organ transplantation is a type of medical procedure in which healthy organs of donors are implanted into the patient to replace the damaged ones in order to save the patient who has exhausted the function of organs or has serious diseases and prolong the life of the patient.1 With the development of immune preparations, surgical techniques, and equipment, organ transplantation has progressed remarkably worldwide. In 2018, a total of 146
840 organ transplants were performed worldwide, with an average of 16.76 transplants performed per hour; however, more than 90% of patients are still waiting for organ transplants. In 2019, 153
863 organ transplants were performed globally. There was an increase of 4.8% compared with the previous year, but it still failed to meet the demand.2 The organs available for transplantation are too insufficient to meet the demand of patients on the waiting list, and this enormous gap has become a difficult problem worldwide. Efforts have been made to alleviate the donor shortage problem, ranging from the use of marginal donors to attempts at pig-to-human xenotransplantation. In recent years, with the rise of bioengineering technology, three-dimensional (3D) printing technology has been introduced into the field of organ transplantation,3 opening up a new research direction for alleviating the shortage of donors.
As a manufacturing technology, 3D printing technology is based on path planning parameters after a 3D digital model is sliced, and the adhesive material is printed layer-by-layer using a 3D printer according to the planned path to produce 3D products.4 Previously, 3D printing technology was mainly used to make models and various instruments, and with the continuous improvement of technology as well as continuous integration with the medical field, 3D printing technology has become a hot spot in medical research,5 one of the most important of which is exploration in the field of organ transplantation. Patients who are in their end-stage period may die if they do not receive organ transplantation, which is currently the most effective treatment.
3D printing is an additive manufacturing (AM) technology that creates patient-specific 3D structures based on the results of patient examinations, such as computed axial tomography (CAT) and magnetic resonance imaging (MRI).5 Characterized by the ability to fabricate highly complicated custom patterns and patient-specific structures that are not possible with traditional assembly techniques such as compression/injection molding, melt/solvent casting, pore-forming agent leaching, and electrospinning, 3D printing can fabricate medical products on demand, which also makes it potentially more attractive in the medical field.6 Since its introduction in the 1980s, 3D printing has become one of the most efficient ways to manufacture custom products using a variety of materials.7 Compared to other sections of the AM industry, the medical industry is the third largest market, accounting for about 16% of total revenue.8 With the increasing demand for customized and patient-specific medical structures, the application of 3D printing in the medical field continues to expand. In addition, as key patents for 3D printing expire, 3D printers have become cheaper and more accessible. Therefore, a new wave of open-source 3D printers is being created to boost research and manufacturing development while reducing production costs.
3D printing technology is currently used in various medical fields, including the oral industry, anatomical models, medical devices, tissue regeneration, physical or pathological models, and pharmaceutical preparations.9 Medical applications are shown in Fig. 1. To date, the dental and hearing aid industries have witnessed the highest degree of application of 3D printing. This may be because end products (such as stomatology products, prosthetics, surgical guidance kits, and hearing aid outer shells) are small and need to be designed for patients, rendering these industries more compatible with 3D printing technology. The applications in the medical field consist of medical devices such as orthotics/prostheses (O&P) (including implantable substances and exterior O&P), surgical instruments, and anatomical models. Patient-specific surgical devices are necessary to enable higher precision and to improve the efficiency. This anatomical model is helpful for pre-operative protocol development and pre-operative patient education. 3D printing technology is also being applied in the pharmaceutical field, and there is a growing focus on personalized formulations for drug use. Tissue and organ bioprinting is an emerging field as 3D printing is becoming more and more important in the tissue engineering and regeneration field, and there is growing interest in 3D printing in both academia and industry.1 Apart from that, 3D bioprinting of preclinical, patient-derived or personalized tissue and pathological models for high-throughput screening of drug testing is emerging. With high potentiality for manufacturing patient-customized drugs and cutting down animal model-based experiments,6 3D bioprinting is showing its significant position in the medical field.
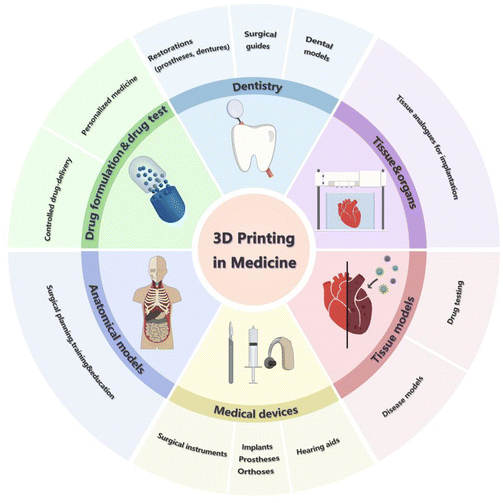 |
| Fig. 1 Applications of 3D printing in different fields. 3D printing has been used in many fields, including dentistry, printed tissues or organs for tissue restoration or transplantation, printed tissues or cells for the establishment of pathological models, medical devices, anatomical models for surgical planning, training or education, drug testing, and high-throughput drug screening. | |
This review, combined with the development history of 3D printing technology, principles of different 3D printing technologies, and their applications in the medical field, introduces the application status, challenges, and future trends of 3D printing in the field of organ transplantation. First, we begin with the development of 3D printing technology, introducing the principles of different 3D printing technologies and their corresponding advantages and disadvantages, and explaining their related applications in the medical field. Then, based on the important limiting factor in the development of organ transplantation, namely the shortage of donors, we discuss the current research progress and introduce the application and promotion of 3D printing in the development of organ transplantation. Finally, combined with the related development of stem cells and organoids, we briefly introduce how 3D printing technology in combination with stem cell technology can promote the development of organ transplantation-related research, and anticipate that the application of 3D printing technology will push the research and development of organ transplantation to a new level.
2. The development, working principles and applications of 3D bioprinting in medicine
3D bioprinting uses cells or other bio-materials as printing inks to print complex 3D biological structures with biological functions according to the requirements of the bionic morphology, biological function, and cellular microenvironment through additive manufacturing methods.10
Since 1995, 3D bioprinting has undergone four stages of development and is becoming increasingly mature. The developmental timeline of 3D printing in medicine is shown in Fig. 2. The first development stage can be called the primary stage of 3D bioprinting technology. The materials used at this stage of 3D bioprinting technology are not biocompatible and cannot be directly used for disease treatment or organ replacement in the human body. Instead, they can be used only to manufacture medical devices and surgical assistance models. In the second stage, biocompatible but non-degradable materials are selected to produce permanent medical implants such as artificial limbs, prosthetic ear grafts, and heart stents. For the first time in the world, the amorphous bone of the scapula was successfully reconstructed using titanium alloy as the “ink” and 3D printing of a prosthesis that was completely consistent with the patient's clavicle and scapula lesions. Compared with the second stage, the materials selected in the third stage not only consider biocompatibility and degradability but also improve safety. Bone, skin, and other tissue engineering scaffolds can be printed to promote the regeneration and repair of human tissues. The absorbable dural patch developed by Guangzhou Maipu Company is a notable success. The fourth stage of the 3D bioprinting technology is a milestone. During this period, 3D bioprinting technology uses living cells, proteins, and other extracellular matrices as materials, and the printed products can be biologically active, which is why the fourth stage of 3D bioprinting technology has become the most historic.11 In other words, in the near future, modern 3D printing can print biologically active in vitro bionic structures that can be used to manufacture human tissues, organs, and tumor models, which is of great significance for organ transplantation and body rehabilitation.
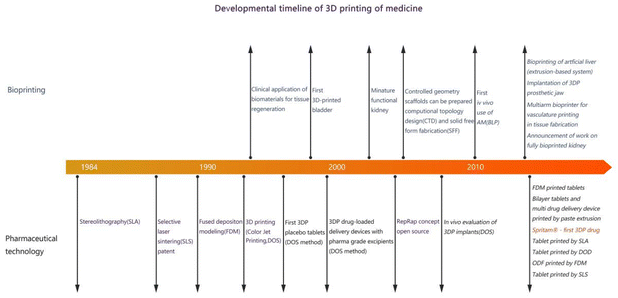 |
| Fig. 2 The developmental history of 3D printing in medicine. SLA: stereolithography; 3DP: three-dimensional printing; SLS: selective laser sintering; FDM: fused deposition modeling; DOS: drop on solid; DOD: drop on drop; CT: computed tomography design; SFF: solid free-form fabrication. | |
Based on the fundamental operating principles for establishing functional tissue constructs and ASTM standards, there are various strategies for 3D bioprinting, including extrusion-based (mechanical/pneumatic), jetting-based (inkjet/microvalve/laser-assisted bioprinting or laser induced forward transfer, acoustic, etc.), and vat photopolymerization-based (stereolithography, digital light processing, and two photon polymerization) bioprinting and magnetic bioprinting.12 The different strategies for 3D bioprinting are shown in Fig. 3. Comparisons among the different printing strategies are listed in Table 1.
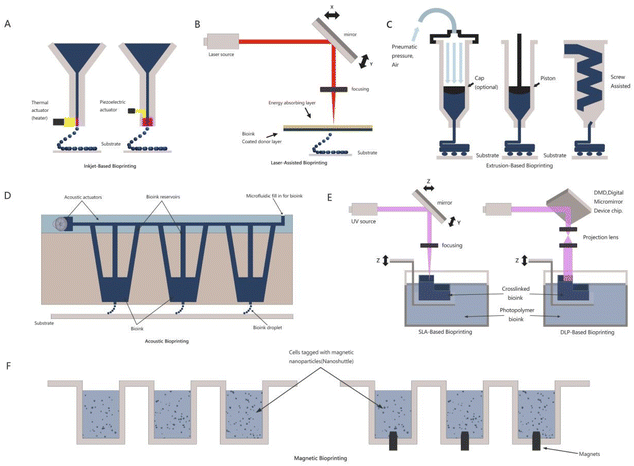 |
| Fig. 3 Schematic figures showing different strategies for fabricating functional tissue constructs. (A) Inkjet bioprinting with thermal and piezoelectric actuators based on drop-on-demand (DOD). (B) Laser-assisted bioprinting; LIFT: laser-induced forward transfer. (C) Extrusion-based bioprinting with pneumatic pressure, pistons, and screw. (D) Acoustic bioprinting. (E) Stereolithography bioprinting including SLA and DLP (digital light processing) laser-based. (F) Magnetic bioprinting: printed cells are shown in the culture media. | |
Table 1 The comparison of several printing strategies for 3D printing
|
Operating principles |
Advantages |
Disadvantages |
Applications |
ECM: extracellular matrix. |
Inkjet bioprinting |
Utilizes a piezoelectric or thermally driven printhead, the bio-ink (a mixture of hydrogel and cells) is divided into a series of micro-droplets, which are later printed in layers. |
• Low cost |
• Unable to print materials with high viscosity and high concentration of cells |
Suitable for printing biological entities |
• Could print different cell types at the same time |
• Potential mechanical or heat injury to cells |
• Fast |
Laser-assisted bioprinting |
Uses a droplet as the basic forming unit, laser-absorbing materials are used to generate microbubbles, which are expanded to drive biomaterials and cells off the substrate and onto the forming platform, which is then fabricated into shape. |
• No mechanical shearing damage to cells |
• High cost |
Mainly in laboratory stage |
• Higher viscosity biomaterials can be printed |
• Not mature enough, lack of commercially available printing devices |
• Wider range of materials available |
• Coating bio-inks on laser-absorbing materials is time-consuming layer by layer |
Extrusion-based bioprinting |
Uses a pneumatic or mechanically driven nozzle to eject the bio-ink in a controllable manner and it deposits onto the forming substrate to form a two-dimensional structure, which is then formed to be a three-dimensional structure. |
• Can be used to print biomaterials with high viscosity |
• Relatively low printing speed |
Suited for the fabrication of scaffolds or implanted prostheses for tissue engineering |
• Can print biomaterials with different viscosities and different concentrations of cells |
• Low-to-medium resolution |
• The range of material applicability is wider |
• Moderate cell viability (40–80) |
• Allowing for the creation of tissues with better structural strength |
Acoustic bioprinting |
Works by generating and depositing cell-encapsulated picolitres of liquid droplets from a bio-ink pool in the presence of a mild sound field |
• Avoid the problem of clogging |
• High cost |
Used for paper printing or distributing organic polymers in semiconductor production or automated liquid managing solutions for high throughput screening, and printing requiring high cell viability |
• Prevent the battery from harmful shear stress, heat, and pressure |
• Relatively complex system |
Vatphotopoly-merization-based bioprinting |
Uses a UV laser as a light source, the laser beam will first draw an object shape on the surface of the liquid resin, and then move with the printing platform so that the platform is immersed in the liquid resin, and so on and so forth, to form a solid print. |
• High flexibility |
• Cell damage caused by UV light and its initiators |
Corneal stromal tissue reconstruction, insulin convey; tissue engineering; scaffold manufacturing |
• High resolution |
• Requirements for lamp wavelength, print size, and “bio-ink” viscosity, which is difficult to standardize |
• Fast manufacturing |
• High cost |
• The devices are easy to control |
Magnetic bioprinting |
Cells are gently magnetized and assembled with magnetic forces |
• Fine spatial control |
• High cost |
Assemble 3D multi-type co-cultures in the laboratory, used for high throughput screening |
• Ability to synthesize endogenous ECM without any man-made protein substrate |
• Relatively complex system |
• Ability to quickly print multiple tissue-like structures |
• Potential to influence cells or cause injury because of the magnets |
2.1 Extrusion-based bioprinting
Extrusion-based or pressure-assisted bioprinting is widely used in scientific research and other fields for creating 3D cell-loaded structures. Conventional thermal inkjet bio-printers can only process low-viscosity bio-inks with bubbles of less than 10 mPa s,13 while high-viscosity bio-inks cannot be ejected from the nozzle spout. By placing the bioink in a disposable medical-grade plastic syringe, extrusion bioprinting can dispense the bioink onto sterile biomaterials in a pneumatic or mechanical manner (piston or rotating screw). The piston-driven deposition device limits the bioink from spilling out, whereas the screw-driven system is good at handling viscous bioinks as well as achieving a suitable position in space. The pneumatic-driven system can adjust pressure as well as valve time in order to facilitate the deposition of bioinks of different types and viscosities.14 Under different pressures, viscous bioinks outflow just like cylindrical filaments (∼150–350 microns in diameter).15 Right after that, those thin long strip bioinks are interconnected with the help of light (mainly ultraviolet light), enzymes, biochemicals and so on, and then highly resilient and long-lasting constructs will be formed. The temperature of the ink reservoir and collecting substrate should be accurately controlled to achieve appropriate bio-ink viscosity and avoidance of gelatinization for the purpose of distributing the thermal and photosensitive polymers.16 Simultaneously, in order to achieve higher precision and resolution, other regulatory factors such as the air pressure and extrusion speed, can be adjusted. However, high pressures and high velocities would augment shear forces, leading to reduced cell viability, which is an undesirable outcome. Therefore, the printing parameters were optimized to maintain a stable printing structure without affecting cell viability so that the advantages of extrusion printing could be fully realized. This printing method has been applied to bioprint many biopolymers such as DNA, RNA, and peptide fibers.17,27 Being widely used, extrusion-based bioprinting has been regarded as an applicable approach for the fabrication of scaffolds or implanted prostheses for tissue reconstruction.
2.2 Jetting-based bioprinting
2.2.1 Inkjet-based bioprinting.
Inkjet-based printing technology is one of the most well-known printing methods and is a continuation of traditional 2D inkjet printers. Without direct contact, image reconstruction during this process is based on the creation and precise positioning of “bioink” droplets of picoliter volume (1–100 pl) on the substrate controlled by a computer.18 Ink droplets prepared for bioprinting can be formed in two ways: (1) continuous inkjet printing (CIJ) and (2) on-demand droplet printing (DOD).19 In short, CIJ printing will produce continuous discrete droplets of ink based on the inherent tendency of liquid flow accompanied by morphological changes. With the help of an electric or magnetic field, droplets become electrified and can get to their own position. In contrast, DOD printing produces biological ink droplets on a substrate when required. In contrast, the CIJ-based bioprinter produces droplets much faster than the DOD system. Nevertheless, the need for becoming conductive and the risk of pollution during the liquid process impede their application in the medical field. In contrast, DOD-based bioprinting is more suitable for material sedimentation and composition due to its accuracy and least bioink waste. A DOD bioprinter contains temperature controlling, piezoelectric, or acoustic units.20 Usually, inkjet bioprinters use thermal or physical compression to generate and shoot off droplets. According to Saunders et al., they can achieve a survival rate of more than 90% for human fibroblasts by piezoelectric-based bioprinting, because it can vibrate more properly to reduce the disruption of molecules and bio-membranes.21–23 In general, temperature controlled inkjet bioprinting is well suited for the printing of bio-entities.
2.2.2 Laser-assisted bioprinting.
This method obtains a micron level resolution based on the laser-induced forward transfer (LIFT) effect, in order to cater to various living cells and biomaterial printing needs.24 LIFT was originally created for metal use, and with advancements, it can now be used for biological material printing using cells, nucleic acids (DNA), and peptides as bioinks.25–27 In 2004, Barron and his team developed a bio-laser printer (BioLP) with a spatial accuracy of >5 μm, which can transfer biological patterns to biological materials.28 A LIFT-based bioprinter or LAB is generally composed of (1) an excited pulsed laser (mainly infrared laser), (2) a simple target or belt used as a biomaterial donor membrane, and (3) a collecting substrate for the produced materials. The belt consists of a laser-transmittable substrate, such as quartz or glass, with a thin layer of metal on the top, such as gold (Au) or titanium (Ti), which can absorb laser light. A metal membrane carrier is then covered with the bioink containing cells or molecules which are immersed in a liquid or gel-like solution such as a culture medium or collagen. At the time the metal film is vaporized by the laser, the bio-ink droplets are subsequently ejected and absorbed by the opposite substrate.29–31 During this period, the interaction between the laser and cells or matrix and cells may affect cell integrity.30,32 Using its nonporous technology, LABs are highly versatile and can handle bioinks with tolerable viscosities that range from 1 to 300 mPa s and cell concentrations of approximately 108 cells per mL. Thus, tissues with a high level of cell density, resolution of 10–100 μm, and different sizes can be assembled to fit for the physiological structure, and based on that, LABs will become applicable for 3D tissue fabrication. However, the selection of biological materials, gravity settlement, and long manufacturing time of the battery in the solution are the main problems for application.
2.2.3 Acoustic bioprinting.
The acoustic bioprinter generates and deposits cell-encapsulated picolitres of liquid droplets from a bio-ink pool in the presence of a mild sound field. With the help of surface acoustic techniques, single-cell controlled and 3D structure construction open a new gate to a higher level of bioprinting.33 Influenced by sound waves, cells can adopt different orientations for complex 3D structure creation. Owing to the absence of nozzles, it avoids clogging problems and insulates the battery from harmful shear stress, heat, and pressure, similar to DOD printers.19 More than a decade ago, researchers created an acoustic bioprinter that could seal and print various types of cells such as hepatocytes, cardiomyocytes, stem cells, etc. The bioink maintained cell viability (>85%) at high-throughput levels. It consists of a single set of 2D microfluidic channels that keep the bioink in place. The sound projector was composed of a piezoelectric substrate made of lithium niobate/lithium tantalate topped with a gold ring to establish a surface acoustic wave. Those sound waves are focused at the air–liquid interface. Acoustic droplets are produced when the force of acoustic vibration exceeds the surface tension of the bioink. The diameter of a droplet varies with the frequency of an acoustic wave.34,35 Recently, researchers have reported the application of some types of 3D acoustic tweezers that use standing surface acoustic wave (SSAW) technology for printing cells, which are characterized by precision, non-invasiveness, and non-contact.33 However, other applications, such as acoustic bioblotting combining multiple types of cells and other molecules, so as to establish bionic cell-loaded scaffolds, still need to be further explored.
2.3 Vat photopolymerization
2.3.1 Stereolithography bioprinting.
Photocurable molding technology (SLA bioprinting) is another efficient approach for constructing three-dimensional patterned scaffolds with microscale or nanoscale structures.36,37 The porosity, accuracy, and physical properties of three-dimensional scaffold structures constructed using ordinary printing methods are typically uncontrollable. SLA bioprinting is an approach using light as a supplement in which photocurable bioinks can be connected one by one in a plane using a light projector.38,39 With the help of SLA, three-dimensional cell-loaded structures can be printed in less than 30 minutes even with resolutions as low as 100 μm while maintaining high cell viability (>90%).40 Some researchers have reported that bioink of polyethylene glycol diacrylate (PEG-DA) and gelatin methacrylate (GelMA) hydrogel which combine with fibroblasts (NIH 3T3) may be cross-linked by visible light to execute SLA printing with a resolution of 50 μm and a cell survival rate of 85%.41 Recently, it is reported that SLA bioprinting was used in neural tissue bioengineering with the application of electrospinning. The combination of directional electrified fibers of polycaprolactone (PCL)/gelatin composite with SLA printed scaffolds with low microporosity, along with polyethylene glycol DA with 66% porosity can improve the behavior and mechanical properties of nerve cells.42
2.3.2 Digital light processing bioprinting.
DLP, as one type of 3D printing technology based on digital light processing is highly controllable with high resolution. Using a digital micromirror device (DMD), ultraviolet (UV) light can be used to project a 2D model onto the target surface and activate localized photopolymerization.43 By moving vertically, its basic layer will repeat back and forth layer-by-layer for a 3D structure. A suitable DMD having an adjusted optical lens can achieve high resolution projection which enables DLP printing to reach micron-level resolution. Although DLP is also one type of VP (vat-polymerization) printing, there are still several differences between those two systems. By contrast with the raster laser scanning of SLA, DLP is characterized by the two-dimensional (2D) photopolymerization on the basis of DMD or a liquid crystal display (LCD) pattern.44 DMD is a special instrument composed of plenty of micromirrors changing positions between ‘on’ and ‘off’ and rays will only be reflected under the “on” condition. As a result of photocrosslinking the ink using the light projected from the digital platform mentioned, such as DLP, with a complete 2D layer, higher efficiency can be achieved compared to SLA printing. On this basis, Fiona Verisqa et al. successfully printed gyroid scaffolds in which human adipose-derived stem cells can maintain viability for about 21 days as well as express osteogenic markers like RUNX2, OCN, etc.45 They can also find a physiological level of calcium deposition from the cells. Sushma Kumari et al. demonstrated their success in printing hydrogels with different concentrations of MA-κ-CA encapsulating NIH-3T3 cells.46 According to their report, their printed hydrogel containing MA-κ-CA showed excellent biocompatibility and recapitulation of biomechanical properties of human tissues as well as high level of cell viability and proliferation. Also, the vascularization of the hydrogel has made progress. Van Thuy Duong et al. reported that they applied the thiol-norbornene cross-linked GelNB hydrogel designed and synthesized by them for encapsulating cells for vascular formation.47 The special hydrogel has several advantages such as lower initial stiffness (G′ ∼ 100–4000 Pa) for better cell viability, physiological status perfusable channels and conjugation of various angiogenic peptides to improve vascularization.
2.3.3 Two photon polymerization.
The past few years had witnessed the emergence of two-photon polymerization (TPP) printing acting effectively for the fabrication of 3D micro-structures for the purpose of cell–material interaction applications due to its micro or even nano-scale resolution accompanied by design flexibility despite of geometrical constraints. Its working principles involve two-photon absorption. Accompanied by a scanning laser, it may enable the fabrication of microscale 3D printing structures by applying two-photon fluorescence microscopy to the initiating of a photoinduced polymerization reaction.48,49 Accordingly, it has been widely applied in the biomedical field. Amedeo Ruggiero and his team took advantage of TPP, combining it with poly(3,4-ethylenedioxythiophene):polystyrene sulfonate (PEDOT:PSS) to fabricate PEDOT:PSS-coated micropillars.48 The complicated 3D structures can cater to any geometrical needs, paving the road to develop 3D electrodes interfacing with biological systems for applications in biomedical science. Directed by a femtosecond laser, two-photon polymerization (TPP) acts as a microscale writing technique which may allow rapid polymerization process from transparent polymers into high-resolution 3D microscale structures, and it has been applied in the field of nanophotonics, microfluidics, tissue regeneration and drug research. On the basis of this advantage, Teng Li et al. used glycidyl methacrylate (GMA) to modify BSA molecules for various BSA-GMA materials, for the purpose of two-photon polymerization with one type of water-soluble free radical type I photoinitiator.50 This so-called BSA-GMA hydrogel structure showed high level of autofluorescence imaging, pH responsiveness, and biocompatibility, which show potential in the biomedical field like tissue engineering to cater to many specific needs. The ability to tailor and strategically design biomaterials of TPP can make a difference in the biomedical field, even in cell culture. Kristan S. Worthington et al. used TPP to print topological patterns with different feature sizes of human-induced pluripotent stem cells (iPSCs) to demonstrate the potential to moderately control stem cell fate, allowing substrate interactions to specifically design biomaterials in a more accurate and efficient way.51
2.4 Magnetic bioprinting
On the basis of the magnetic levitation principle, magnetic 3D bioprinting (M3DB) enables the assembly of three-dimensional multi-type co-cultures in the lab.52 This technology has many advantages, such as fine spatial control, ability to synthesize endogenous ECM without any man-made protein substrate, and ability to quickly print various tissue-like structures.53,54 It primarily uses a contact-free approach to guide cells to assemble into a fixed-shape structure using two different methods.55 The first method was label-free inverse magnetic permeation printing. After mixing the cell medium with paramagnetic buffer, it was exposed to a circumjacent magnetic field to establish cell aggregates.56 In the second approach, cells are assembled with nanoparticles composed of poly-L-lysine, magnetic iron oxide (Fe3O4, magnetite), and gold nanoparticles (nano-shuttle-PL), which are prone to gel formation owing to electrostatic interactions. The cells can be magnetized while absorbing the gel for easy control. Cells can float on the surface of the plate and enter the medium, forming aggregates.54 Under the slight magnetic force generated by the prefabricated magnetic plate, the magnetized cell aggregates can form 3D structures. The spatial structure of cell aggregates can be changed by changing the shape of the magnetic plates applied.57 Adipose tissue, lung tissue, aortic valve tissue, blood vessel tissue, glioblastoma, and breast tumor tissue can be prepared and analyzed for active protein expression and extracellular matrix using this method.58 Based on the M3DB technique, Tseng and his team verified spheroid shrinkage as a biological endpoint for cytotoxicity detection using the response of 3T3 cells and mouse embryonic fibroblasts (MEFs) to 5 different toxic compounds. This study shows that the detection method developed based on the property that M3DB spheres can measure cytotoxicity in 3D microenvironments overcomes the limitations of other three-dimensional cell culture approaches in terms of manipulation, imaging, etc.58 In addition, they presented a 3D in vitro model that could be used to evaluate the physiology of uterine contractions simulated using human myometrium cells. Patient-derived myometrium cells are used to form a hollow ring using magnetic printing technology, which can be used to analyze the changes in uterine contractility over time and provide many parameters for its clinical application. These ring-like uterine 3D-printed products are of different origins and differ in their contraction forms and responses to the uterine contraction suppressors nifedipine and indomethacin. This study addresses the urgent necessity for high-throughput assessment of different drugs and uterine contraction status in clinical studies.59 Hou and his team used M3DB and cell repulsion to establish pancreatic cancer organoids in ordinary flat-bottomed cell culture plates and examined the inhibitory effects of approximately 3300 drugs under clinical trials on cells derived from pancreatic cancer patients, and cancer-related fibroblasts were also included.60 Furthermore, Baillargeon and his team used a similar technique to expand the density of traditional 96-well and 384-well plates to 1536-well plates to confirm that this method can be used for the large-scale production of cell spheroids and organoids for high-throughput screening.61
2.5 Factors affecting the cell viability and printability in each of the bioprinting technique
As mentioned before, there are different printing technologies different from each other, all of which can play a significant role in the biomedical field. Despite the working principles, there are still many factors that may influence the efficiency and effect of printing, among which cell viability, printability, and types of bioinks play an important role.
2.5.1 Cell viability.
As we all know, during different bioprinting processes, living cells from different sources will suffer from different magnitudes of stresses which results in different cell viability.62 Maintaining high cell viability is the key point to retain normal cellular behaviors, such as the proliferation and differentiation capability. In this section, the common factors affecting cell viability of each bioprinting technique will be discussed.
2.5.1.1 Extrusion-based bioprinting.
Extrusion-based bioprinting is a nozzle-based bioprinting technique where its cell viability is largely influenced by the shear stress as well as thermal and radiative stresses when photocrosslinkable materials are selected.63 Viscosity of bioink projected by the nozzle ranges from 30 to 6 × 108 mPa s and with the concentration and viscosity increasing, the cell viability decreases, giving rise to a low cell viability ranging from 40 to 80%.62 Nevertheless, with the development of bioprinting, bioprinting performance with better cell viability can also be achieved via optimizing the original design and operation conditions. Rashik Chand et al.64 created a method using the wall shear stress to analyze cell viability, taking dispensing pressure, nozzle diameter, and nozzle geometry into account, they indicated that the cells will experience higher shear stress depending on the nozzle length in the cylindrical nozzle, and emphasized that the flow rate of bioink is crucial in relation to shear stress. Müller et al.65 demonstrated that the bioink containing alginate sulfate, nanocellulose, and chondrocytes was projected with different air pressures for the purpose of fabrication of complicated structures such as a mini-ear. Cell viability under different conditions was maintained at a rate of more than 85% during a 14-day culture and steady at 88% after a 28-day culture. Gao et al.66 creatively combined the bioink comprising alginate and fibroblasts with bioink containing alginate and smooth muscle cells and bioprinted them into vascular-like 3D constructs filled with fluid channels. After a 7-day culture, 91% cell viability was reached.
2.5.1.2 Jetting-based bioprinting.
Jetting-based bioprinting comprises inkjet/microvalve/laser-assisted bioprinting or laser induced forward transfer, acoustic bioprinting, etc. Cell viability during jetting-based bioprinting is mainly influenced by the shear stress, thermal stress, droplet impact velocity, droplet volume, etc. In the process of printing, due to the limited nozzle size, bioink was required with low viscosity and concentration for the purpose of reducing the shear stress and protecting cells from significant injuries.62 It is reported that a bioink with a viscosity ranging from 3 to 30 mPa s is suitable for jetting-based bioprinting67 and a cell viability rate of more than 85% can be obtained.68 Wei Long Ng et al.69 reported that cell-encapsulating bioinks with higher viscosity may reach higher average cell viability (%), as the containing of polymer within the printed droplets can provide a cushioning effect (higher energy dissipation) for the cells, protecting them even at higher droplet impact velocity. Apart from that, Wei Long Ng et al.70 also demonstrate that via a sub-nanoliter scale control of the droplet impact velocity and droplet volume during bioprinting, viability and proliferation of printed human sourced cells will be protected at a higher level. Xu et al.71 produced zigzag tubes encapsulating 3T3 fibroblasts through inkjet-based bioprinting. During the process, the cell viability maintained at about 82% after 72 hours of culture. Then, using a bioink composed of sodium alginate and 3T3 fibroblasts cells, vascular-like 3D structures were fabricated by inkjet printing,72 and a 90% cell viability rate was achieved after a 24-hour culture.
2.5.1.3 Vat photopolymerization bioprinting.
Vat photopolymerization, including stereolithography (SLA) and recently popularized digital light processing (DLP), is developing rapidly. It holds promise for the fabrication of biomedical scaffolds for applications in drug discovery and regenerative medicine.73 To date, the development of biomaterials enables the combination of living cells and bioactive agents. Although VP-based bioprinting holds high-resolution photopolymerization catering for the creation of 3D structures, cell viability is mainly affected by the thermal and radiative stresses and sometimes, the mechanical strength and porosity of the materials.62 It is reported that cell viability of VP-based bioprinting can exceed 90% while the resolution may reach 10 μm.74 Therefore, this type of bioprinting has been favored in the field of tissue engineering. Wang et al.75 constructed 3D structures using SLA-based bioprinting using the bioink containing polyethylene glycol diacrylate (PEGDA), GelMA, eosin Y (EY) and 3T3 fibroblast cells. They reported a minimum resolution of 50 μm and a cell viability rate of more than 85% maintained for at least 5 days. Interestingly, the wavelength of lasers can also affect cell viability. It has been reported that shorter excitation wavelengths of the VP-based bioprinting system may cause harmful effects on the cell viability during printing.76 Recently, Zhang et al.77 used a bioink composed of GelMA, mouse bone marrow mesenchymal stem cells (mBMSCs), etc. to develop a SLA-based bioprinter in order to create natural tissue-like 3D structures. As they reported, the constructs they obtained can reach a high cell survival rate of 95%.
2.5.2 Printability & bioinks.
2.5.2.1 Extrusion-based printing.
Extrusion-based bioprinting is one of the widely used 3D printing techniques, characterized by the use of a syringe, a nozzle and a pressure system. Many factors affect its printing effect.78 (a) Material properties: the properties of the filament material used can significantly affect printability. Factors such as the melting temperature, flow characteristics, viscosity, and adhesion of the material can influence how well it extrudes and adheres to the build plate and previously printed layers. (b) Nozzle temperature: nozzle temperature also matters. If the nozzle temperature is too low, the filament may not melt adequately, resulting in poor layer adhesion and weak prints. On the other hand, if the temperature is too high, it can cause issues like filament oozing, stringing, or even clogging of the nozzle. (c) Bed adhesion: appropriate adhesion of the first printed layer to the build plate is essential for successful printing. Factors such as the bed surface material, leveling, and the use of adhesives or coatings (e.g., hairspray, glue stick, etc.) can affect the adhesion between the print and the build plate. (d) Layer height and print speed: the layer height and print speed settings impact the overall print quality and printability, which is based on the printer's capabilities and the desired level of detail and strength is crucial. Higher layer heights and faster print speeds may result in reduced print quality, while lower layer heights and slower speeds can improve detail but increase printing time. (e) Support structures: for complex or overhanging geometries, support structures are often required to prevent sagging or collapse during printing. Appropriate design and placement of support structures, as well as their ease of removal after printing, can affect the printability of intricate models. (f) Printer calibration and maintenance: appropriate leveling of the print bed, calibration of the extruder, and checking the belt tension ensure accurate printing. Keeping the printer clean and lubricated, and regularly replacing worn-out or damaged parts, such as the nozzle or filament drive gear, help maintain reliable and high-quality prints.
Apart from this, different bioinks79 and the applied concentration may also affect the printing results. Here are some general concentration ranges for commonly used bioinks: (a) alginate-based bioinks: alginate concentrations typically range from 1% to 4% (w/v). Lower concentrations provide higher printability due to lower viscosity, while higher concentrations offer better structural support. Alginate concentrations can be adjusted by altering the ratio of alginate powder to the solvent. (b) Gelatin-based bioinks:80 gelatin concentrations typically range from 5% to 15% (w/v). Lower concentrations offer better printability and lower viscosity, while higher concentrations provide increased mechanical strength. The gelatin concentration can be adjusted by varying the ratio of gelatin powder to the solvent, such as water or cell culture media. (c) Fibrin-based bioinks: fibrin concentrations usually range from 2 mg mL−1 to 10 mg mL−1. The concentration can be adjusted by varying the ratio of fibrinogen to thrombin during the crosslinking process. Lower fibrin concentrations result in better printability, while higher concentrations offer improved mechanical stability. (d) Collagen-based bioinks: collagen concentrations typically range from 1 mg mL−1 to 10 mg mL−1. The concentration can be adjusted by varying the ratio of collagen powder to the solvent, such as acetic acid or cell culture media. Lower collagen concentrations provide better printability, while higher concentrations offer enhanced mechanical strength and support cell attachment. (e) Hyaluronic acid (HA)-based bioinks: hyaluronic acid concentrations usually range from 0.1% to 2% (w/v). The concentration can be adjusted by varying the ratio of HA powder to the solvent, such as phosphate-buffered saline (PBS) or cell culture media. Lower HA concentrations provide better printability, while higher concentrations offer increased viscosity and better tissue-mimicking properties.
2.5.2.2 Jetting-based printing.
Jetting-based 3D printing, such as inkjet or drop-on-demand printing, involves the precise deposition of small droplets of bioink onto a substrate to create the desired structure.81 (a) Material properties: the properties of the jetting materials, such as photopolymers or resins, significantly impact printability. The viscosity, flow characteristics, curing behavior, and adhesion properties of the materials can affect how well they are jetted and solidify during the printing process.
(b) Print head configuration: the number and arrangement of print heads, the size of the nozzles, and the precision of droplet deposition determine the resolution and accuracy of the printed objects. (c) Print resolution and layer thickness: the chosen print resolution and layer thickness impact the level of detail and the overall print quality. Higher resolutions and thinner layers generally result in finer details but may also increase printing time. (d) Support material and removal: jetting-based 3D printers often employ support materials to provide stability for overhanging or complex structures during printing. The support materials should be compatible with the main printing material and be easily removable without damaging the printed object. (e) Print environment and chamber conditions: factors such as temperature, humidity, and air circulation can influence the curing or drying process of the printed materials, affecting their adhesion, dimensional accuracy, and overall print quality. (f) Material handling and storage: some materials may be sensitive to light, heat, or moisture and require specific storage conditions. Following the manufacturer's guidelines for material handling, storage temperature, and shelf life can help preserve the quality and printability of the materials. (g) Printer software and settings: appropriate configuration of printing parameters such as layer thickness, print speed, support generation, and curing options is essential for successful prints. Understanding the capabilities and limitations of the printer and software and fine-tuning the settings accordingly can improve printability.
The suitable concentration of bioinks in jetting-based 3D printing can vary based on the specific bioink formulation, printer specifications, droplet size, and the requirements of the target tissue or organ. Here are some general concentration ranges for bioinks used in jetting-based 3D printing:82 (a) alginate-based bioinks: alginate concentrations for jetting-based printing typically range from 1% to 3% (w/v). Lower concentrations improve jetting ability and prevent nozzle clogging, while higher concentrations provide better structural support and gelation. (b) Gelatin-based bioinks: gelatin concentrations for jetting-based printing generally range from 5% to 10% (w/v). Lower concentrations offer better jetting properties and reduce the risk of nozzle clogging, while higher concentrations provide increased mechanical strength. (c) Fibrin-based bioinks: fibrin concentrations in jetting-based printing typically range from 5 mg mL−1 to 10 mg mL−1. Lower fibrin concentrations improve the jetting ability, while higher concentrations offer improved mechanical stability. (d) Collagen-based bioinks: collagen concentrations for jetting-based printing generally range from 1 mg mL−1 to 5 mg mL−1. Lower collagen concentrations improve jetting properties, while higher concentrations offer increased mechanical strength and support cell attachment. (e) Hyaluronic acid (HA)-based bioinks: hyaluronic acid concentrations for jetting-based printing typically range from 0.1% to 2% (w/v). Lower HA concentrations improve the jetting ability, while higher concentrations offer increased viscosity and better tissue-mimicking properties.
2.5.2.3 Vat photopolymerization.
Vat photopolymerization-based 3D printing, such as stereolithography (SLA) or digital light processing (DLP), utilizes photopolymer resins that solidify when exposed to light.83 During this process, resin properties, exposure settings, build platform and adhesion, etc. may somewhat influence the printability. (a) Resin properties: factors such as viscosity, curing behavior, shrinkage, and adhesion properties of the resin can impact how well it spreads, solidifies, and adheres to the build platform and previously cured layers. (b) Exposure settings: these settings determine how much light energy is delivered to the resin to initiate the curing process. Finding the right exposure settings for a specific resin is essential to achieve appropriate layer curing, adequate detail resolution, and overall print quality. (c) Build platform and adhesion: the build platform or build plate serves as the foundation for the printed object. Ensuring appropriate adhesion between the first cured layer and the build platform is crucial for successful printing. Factors such as the build platform material, surface treatment, leveling, and the use of adhesive layers or coatings can impact the adhesion and stability of the printed object during the printing process. (d) Support structures: for designs with overhangs or complex geometries, support structures may be necessary to prevent sagging or collapse during printing. (e) Resin handling and storage: resins can be sensitive to light, heat, or moisture, and may require specific storage conditions. Following the manufacturer's guidelines for resin handling, storage temperature, and shelf life can help preserve the quality and printability of the resin. (f) Printer calibration and maintenance: appropriate leveling of the build platform, calibration of the light source, and ensuring the integrity of optical components contribute to achieving accurate and reliable prints. Regular cleaning and maintenance of the vat and optical surfaces also help maintain print quality. (g) Post-processing and curing: after printing, post-processing steps such as removing the printed object from the build platform, cleaning excess resin, and properly curing the object can impact the final print quality and dimensional accuracy. Curing ensures the final strength and stability of the printed part. The suitable concentration of bioinks in vat photopolymerization-based 3D printing can vary based on the specific bioink formulation, printer specifications, desired print resolution, and the requirements of the target tissue or organ. Here are some general concentration ranges for bioinks used in vat photopolymerization-based 3D printing:84
(a) Photopolymerizable hydrogel-based bioinks: photopolymerizable hydrogel-based bioinks often consist of a combination of a photocrosslinkable polymer, such as polyethylene glycol (PEG) or poly(ethylene glycol) diacrylate (PEGDA), and bioactive components like cells or growth factors. The concentration of the photocrosslinkable polymer can range from 5% to 20% (w/v), depending on the desired mechanical properties and print resolution. Higher concentrations generally provide better structural integrity, while lower concentrations may offer improved printability and resolution. (b) Gelatin methacryloyl (GelMA)-based bioinks: gelatin methacryloyl (GelMA) is a modified form of gelatin that can be crosslinked using photopolymerization. GelMA-based bioinks typically have a GelMA concentration ranging from 5% to 15% (w/v). Higher concentrations offer better mechanical stability, while lower concentrations can improve printability and cell response. (c) Methacrylated hyaluronic acid (HAMA)-based bioinks: methacrylated hyaluronic acid (HAMA) is a modified form of hyaluronic acid that can undergo photopolymerization. HAMA-based bioinks usually have a HAMA concentration ranging from 1% to 4% (w/v). Higher concentrations provide better structural stability and increased viscosity, while lower concentrations offer improved printability and cell response. (d) Other photopolymerizable bioinks: there are various other photopolymerizable bioinks available, such as poly(caprolactone) diacrylate (PCL-DA) or poly(ethylene glycol) dimethacrylate (PEGDMA). The concentration of these bioinks can vary depending on the specific formulation and requirements of the application. Typically, concentrations ranging from 5% to 20% (w/v) are used, but it's important to determine the most suitable concentration depending on the specific situation.
After the rapid development of bio-3D printing over the last decade, many milestones have been achieved. 3D printing technology enables the use of bioactive implants to manufacture artificial tissue, facilitating the repair tissue trauma and restore tissue function; however, this approach is currently in the laboratory research stage, and only a few studies have progressed to the clinical trial stage. Currently, biological materials, stem cells, and growth factors are usually combined to produce tissue-engineered products, and the role of 3D printing technology is to build personalized bionic structures.
3. The advancement of 3D bioprinting in organ transplantation
Currently, organ failure still poses a huge threat to the patient's life and organ transplantation is still the first choice for organ failure patients. But the shortage of donors is what hinders the development of organ transplantation and a variety of patients on the waiting list for transplantation often die due to the scarcity of suitable donors, and sometimes they should withstand the risk of immunosuppression. Conventionally, tissue engineering depends on the application of suitable scaffolds for the purpose of cell adhesion, proliferation and so on, which have the advantages of optimal scaffold's mechanical properties as well as degradation kinetics and meanwhile, the biomolecules and growth factors could be delivered by being embedded in the scaffolds.85 But this also results in low density of cell seeding and insufficient homogeneity within the porous scaffolds and therefore tissue engineering has been impeded. But the application of 3D printing in the fabrication of patient-specific tissue and organs has offered a promising settlement.
The development goal of 3D printing technology in the field of organ reconstruction is mainly to build artificial organs with morphological bionics and functional bionics, and to develop in the direction of multi-organ reconstruction. Important progress has been made in morphological bionics in recent years. Three dimensional bioprinting has already been applied for the regeneration and transplantation of several tissues, such as multilayered skin, vascular grafts, bone, heart tissue, tracheal splints, cartilaginous structures, etc.86 However, many challenges remain to be overcome in solid organs. A summary of the bioink and printing strategies for several 3D printed tissues and organs for tissue regeneration and organ transplantation is listed in Table 2. Some developments in solid organ 3D bioprinting are presented in the following.
Table 2 Summary of bioink and printing strategies of several 3D printed tissues and organs for tissue regeneration and organ transplantation
|
Bioink |
Printing strategies |
Current achievements |
PGC: polyelectrolyte gelatin–chitosan; GelMA: gelatin methacrylate; LIFT: laser-induced forward transfer; LAB: laser-assisted bioprinting; HUVEC: human umbilical vein endothelial cells; hMSC: human mesenchymal stem cells; hCMPCs: human cardiac derived cardiomyocyte progenitor cells; iPSC-CM: induced pluripotent stem cell derived cardiomyocyte; PLGA: poly DL-lactic-co-glycolic acid; TCP: tricalcium phosphate; hASCs: human adipose derived stem cells; H3PO4: phosphoric acid; CNF: nanocellulose; BaG: bioactive glass; SLA: stereolithography; FDM: fused deposition model; PCL: polycaprolactone; HA: hyaluronic acid; cECM: cartilage extracellular matrix; hiPSCs: human induced pluripotent stem cells; hESCs: human embryonic stem cells; HCs: primary hepatocytes; HLFs: human lung fibroblasts; VEGF: vascular endothelial growth factor; NSCs: neural stem cells; BMSCs: human bone marrow stromal cells; SC: Schwann cells; RGD-GG: arginine–glycine–aspartic acid peptide-modified gellan gum; CNS: central nervous system; RDFs: rat dermal fibroblasts; PCL: polycaprolactone; pdECM: pancreatic tissue derived extracellular matrix. |
Skin |
• Keratinocytes |
• Extrusion-based bioprinting |
Wound healing, burned skin restoration; |
• Fibroblasts |
• LIFT bioprinting |
In vitro human skin models for product and drug testing with at least about 90% cell survival. |
• hMSC |
• LAB |
• HFF-1 cells |
• Collagen-based hydrogel |
• PGC hydrogel |
• GelMA/Col-Ty |
Cardiac |
• Primary feline adult cardiomyocytes and HL-1 |
• Inkjet bioprinting |
“Half-heart” models (1 cm inner diameter with two connected ventricles and rectangular sheet measuring 3 × 0.8 × 0.5 cm); |
• Cardiac muscle cells |
• LIFT bioprinting |
Regenerated cardiac patches and models of vascularized cardiac tissue for myocardial infarction with a cell viability of 80–90%. |
• Alginate/gelatin gels |
• Extrusion-based bioprinting |
• HUVEC |
• hMSC |
• hCMPCs |
• Pre-vascularized stem cells |
• iPSC-CM |
Bone |
• Human osteoblast cells |
• Micro SLA technology |
Bone regeneration in rat cranial defects or femoral defects; |
• PLGA microspheres |
• Microwave sintering 3D printing |
Synthetic bone graft substitutes with a cell viability of about 92.4%. |
• TCP |
• Laser/metal printing |
• hASCs |
• FDM |
• H3PO4 |
• Inkjet printing |
• Type I & II collagen |
• GelMa hydrogels |
• CNF |
• BaG |
Cartilage |
• PCL |
• Extrusion-based bioprinting |
Joint regeneration and arthritis treatment of rabbits with a cell viability of more than 80%. |
• HA |
• Electrospinning |
• Fibrin-collagen hydrogel, alginate hydrogel |
• Inkjet bioprinting |
• cECM-functionalized |
• Alginate bioinks |
• Chondrocytes |
Liver |
• hiPSCs |
• Valve-based inkjet bioprinting |
Three-dimensional microfluidic microanalytic micro-organ device; |
• hESCs |
• Extrusion-based bioprinting |
Mini livers as drug testing models. |
• HCs |
• HUVECs |
• HLFs |
Kidneys |
• Primary kidney cells |
• Extrusion-based 3D bioprinting |
Analysis of drug metabolism, aid in extracorporeal dialysis; |
• Tubular epithelial cells |
• Inkjet-based bioprinting |
Monitoring endothelial cell dysfunction; |
• Primary mouse renal tubules |
Constructing disease models and pharmacological applications. |
• Fibroblasts |
• Gelatin |
• Hyaluronic acid |
• Glycerol |
• Gelatin–fibronectin |
Lungs |
• Alveolar epithelial type II cells (A549) |
• Valve-based inkjet bioprinting |
Bioprinted lung tissue for assessments in toxicology and drug screening. |
• Endothelial (EA.hy926) cells |
Neural |
• Collagen hydrogel |
• Inkjet bioprinting |
Bioengineered nerve implantation into the Sprague Dawley rats with sciatic nerve injury with cell viability of about 70–92%; |
• VEGF-releasing fibrin gel |
• Extrusion-based bioprinting |
Three-dimensional bioprinted hydrogel could effectively repair the damaged CNS in zebrafish embryos; |
• Polysaccharide hydrogel mixed with alginate, carboxymethyl chitosan, and agarose |
|
Potential to directly treat patients with a variety of neurological disorders; |
• Murine NSCs C17.2 |
• Laser-assisted bioprinting |
Disease modeling and regenerative engineering and nerve tissue repair. |
• Mouse BMSCs |
• SC |
• RGD-GG hydrogels |
• Primary cortical neuronal cells |
• HA |
• Pluronic F-127 bioink |
Pancreas |
• RDFs |
• Extrusion-based bioprinting |
Pancreatic tissue regeneration. |
• Mouse insulinoma beta TC3 (βTC-3) cells |
Controllable release of anticancer drugs to restrain pancreatic cancer growth with rare side effects for >4 weeks; |
• PLGA |
3D structures could improve pancreatic functions such as regulation of insulin secretion and maturation of insulin-producing cells differentiated from hiPSCs. |
• PCL |
• pdECM |
3.1 Skin
As the largest organ of the human body, skin is the first barrier between the inner part and the outside world, which makes skin exposed to so many injury risks. Although the regeneration ability of skin is higher than other organs or tissues, the repair of such injuries as large-scale deep injuries like deep burns mainly depend on scar repair which leads to damage to normal functions. Since the primary cellular environment will be hard to recover, the hair follicles and sweat glands will be unable to regenerate and complications like hyperthermia and heat stroke will appear which will greatly impair the life quality of patients. Until now, autologous skin transplantation is still the most common treatment.87 But its applications are still limited due to the shortage of donor sites, secondary impairments, and possibilities of infections. The involvement of tissue engineering provides a new choice for the treatment of skin defects. A variety of tissue-engineered skin (TES) tissues have been produced such as Integra, Dermagraf, etc.,87 which may completely cover the wounds and accelerate healing with less scar, and even with the vascularization of skin substitutes. With a solid freeform fabrication (one kind of extrusion-based bioprinting) technique, Lee et al.88 reported the first fabrication of keratinocytes and fibroblasts in stratified arrangements that resembled dermal/epidermal tissues. The collagen-based hydrogel bioink was printed in the manner of LbL, that is, collagen–fibroblast–collagen–keratinocyte–collagen. Although a high cell viability was observed, cell proliferation was still influenced by the resolution. The LIFT bioprinting employed by Koch et al.89 to print NIH 3T3 (fibroblasts)/HaCaT (keratinocytes) and human mesenchymal stem cells (hMSC) demonstrated that stem cells are more likely to reduce cell viability. In order to investigate the native tissue formation, Koch et al.90 also designed experiments to print cells in the collagen matrix via LAB printing, which demonstrated that cells could still proliferate in the printed structure while retaining vitality and printing pattern.
Recently, Albanna et al.91 reported their proof-of-concept validation of a clinical skin bioprinter which printed autologous skin cells in situ catering to the purpose of healing full-thickness wounds. They combined dermal fibroblasts and epidermal keratinocyte cells with a fibrin/collagen hydrogel carrier which can maintain their viability and accurate deposition for multicellular, multi-layered skin structure formation and they found that 3–6 weeks after printing, the survival of human fibroblasts, keratinocytes and endogenous cells can still be observed and they surprisingly recognize that re-epithelialization in bioprinted wounds has been reached and after 6 weeks, the wound was completely healed.
Skin appendages, which include sweat glands, hair follicles and sebaceous glands, are known for the ability to maintain skin barrier function, as well as to regulate body temperature and so on.87 A variety of studies demonstrate that microenvironment matters when it comes to the regulation of skin appendages. The directive differentiation in vitro of skin appendages and incubation have succeeded, which means various bio-engineering skin equivalents derived from induced stem cells or spheroids have proven to be workable to promote skin tissue regeneration for wound healing in which 3D bioprinting plays a significant role.92,93
Existing in the dermal layer of the skin, hair follicles are composed of hair papillae, hair matrix, root sheath, and hair bulges, in which papilla plays an important part because of its key effect in the length, hardness, and growth cycle of hair. Wu et al.94 reported that they combined hair follicle epithelial cells, dermal papilla cells (DPCs), and dermal sheath cells with collagen and transplanted them into the dorsal skin of nude mice. They demonstrated that hair fibers were formed. The human HPCs cultured in vitro can regenerate intact hair follicles because human HPCs quickly lose their induction ability in vitro. As a result of that, maintaining the induction capability of dermal papilla in vitro is still challenging. Higgins et al.95 demonstrated that using a 3D spheroid culture, the dermal papilla could partly maintain the intact transcriptional signature. The induction ability could be partially restored by translating from the signature and could in situ induce hair follicles in human skin. Miao et al.96 also reported that they prepared 3D-constructed HPC spheres based on a Matrigel scaffold. The loss of those hair papilla marker gene expression like NCAM, α-smooth muscle actin (α-SMA), etc., in 2D culture could be maintained in HPC spheres.
Sweat glands contain eccrine sweat glands and apocrine sweat glands, of which the former controls thermoregulation and sweating.97 When injured with extensive trauma and deep burn, patients will usually suffer from the disorder of sweat gland regeneration and the pain of inability to sweat and dissipate heat. Regarding the reconstruction of the sweat glands, Huang et al.98,99 primarily fabricated ECM containing mouse PD homogenate via 3D bioprinting to create a microenvironment for the differentiation of epidermal progenitor cells. At the same time, the in vivo transplantation of the 3D bioprinted structures leads to the maintenance of sweat glands of mice paws injured from burn. Yao et al.100 used extrusion-based bioprinting to fabricate a microenvironment of sweat glands for the purpose of inducing mesenchymal stem cell (MSCs) differentiate into functional sweat gland. During this process, biochemical and structural cues were essential for MSCs fate decision into the glandular lineage. Apart from that, they also demonstrated that collagen triple helix repeat containing 1 (CTHRC1), and hemeoxygenase 1 (Hmox1), acted as two key factors that increased the profile of sweat gland gene expression.
Sebaceous glands comprise various acini branching away from a central duct,101 and their regeneration ability has also drawn attention recently. As for the reconstruction of the sebaceous glands in vitro, Chen et al. reported that with the help of 3D bioprinting, they fabricated tarsal plate scaffolds using polycaprolactone, which was covered with decellularized matrix of adipose-derived stem cells (ADSCs). The scaffolds, which are biocompatible, were filled with human SZ95 sebocytes. Excellent sebocyte viability and lipogenesis were observed on the scaffolds, and based on that, tarsal plate regeneration will be available.102
3.2 Heart
Noor et al. used induced pluripotent stem cells constructed from patients’ adipocytes, and mixed extracellular matrix, collagen, and other components to prepare a bio-ink and constructed a miniature heart with atria, ventricles, and major blood vessels.103 Because the main components of the bio-ink are derived from the patient's own cells, the artificial organ can match the patient's immune, cellular, and biochemical characteristics. The functional bionics of biological 3D printing artificial organs have also made important breakthroughs. In terms of heart bioprinting, Jallerat et al. constructed a new bioprinting method that printed collagen inner and outer walls and cardiomyocytes in the middle, and constructed left ventricle and heart valve models.104 After in vitro culture, this tissue model can produce spontaneous beating. A team of researchers at the University of Erlangen-Nuremberg in Germany made remarkable achievements in the field of 3D printing technology.105 They successfully created a miniature heart chamber (the chamber at the bottom of the heart) that could beat autonomously for at least three months. Although there have been many breakthroughs in 3D printing in recent years, the fine structure and complex function of the myocardium have not been fully simulated. In the future, this 3D-printed artificial ventricle could potentially be used to treat patients with heart disease, especially those awaiting a heart transplant. However, there are many challenges that need to be overcome with this technology. How to ensure that the body can accept the 3D printed heart, how to keep the ventricle alive for a longer period of time, and how to make it effectively connect with other organ systems in the body are topics that need to be studied by scientists.
3.3 Ear
The New York Times reported that the US regenerative medicine implant technology company 3DBio Therapeutics announced that it had successfully completed the first implantation of AuriNovo, a 3D printed ear implant grown from human cells, to reconstruct a patient's missing outer ear. In 2018, the Ninth People's Hospital Affiliated to Shanghai Jiao Tong University School of Medicine, Shanghai Key Laboratory of Tissue Research, China National Center for Tissue Engineering, Weifang Medical University, and Xinhua Hospital of Dalian University joined hands to cultivate “ear implants” through chondrocyte culture and 3D printing, and successfully implanted them into the ears of five children aged 6–9 years with unilateral microtia.106 The technology of ear cartilage culture through tissue engineering is becoming increasingly mature, but there are still many challenges in its clinical application. One of them is how to improve the mechanical and anti-wear properties of the ear cartilage to maintain its shape for a long time. In addition, coordination of the regeneration and degradation rate of chondrocytes in vivo is crucial to avoid excessive degradation leading to structural loss.
3.4 Lungs
The interwoven vascular network in the human body is physiologically and biochemically interconnected and its structure is closely related to its tissue function. Reconstruction of the structure and function of solid organ pipelines has always been a difficult problem for scientists engaged in organ 3D printing. For example, the reconstruction of the blood vessels and trachea of the lungs is a key point in research on lung 3D printing. In terms of bio-3D printing of alveoli, Grigoryan et al. built 3D printed lungs with the same network structure as human blood vessels and trachea structures, which can transport oxygen like normal lungs and carry out the physiological process of “breathing”.107 Using projection stereolithography 3D printing technology, with food dye additives as biocompatible light absorbers, a vascular system with a 3D internal functional structure can be prepared in a transparent photopolymerized hydrogel in just a few minutes. They designed and fabricated intertwined vascular networks based on a space-filling mathematical topology, and simulated the alveolar structure to realize oxygen exchange between red blood cells in flowing human blood. To investigate how COVID-19 affects patients who recover from it, Axial3D and the respiratory team at the Health and Social Insurance Foundation in Belfast have used 3D printing technology to replicate 3D scale lung models of COVID-19 patients.108 The 3D printed model was based on CT scans taken on day 14 of the patient's infection, which clearly showed that their lungs were affected by the virus. 3D printed lung models can be used to visualize the lungs, providing researchers with a completely new way to view and understand the long-term effects of infection.
3.5 Liver
The liver plays a very important role in the human body, and it is an important organ responsible for metabolism, detoxification, and bile secretion. Owing to its complex structure and function, the reconstruction of the liver is a major problem in the application of 3D printing technology in the field of organ transplantation.109 In order to overcome these obstacles, various liver-on-a-chip models have been approved for in vitro liver drug testing.110 With the help of bioprinting, Lee et al. developed a three-dimensional liver-on-a-chip model, including different types of liver cells, liver ECM microenvironments, and vascular/biliary fluid tubes.111 They used the model to facilitate co-culture of hepatocytes, produce a liver decellularized ECM bioink for the microenvironment, and create vascular and biliary systems. The different hepatocyte sources were well deposited and showed their respective characteristics on a 3D liver chip. The chip equipped with biliary fluidic tubes could enhance biliary system construction and improve liver-specific gene expression as well as demonstrate hepatic functions compared to a chip lacking bile duct channels, and evaluation using Acetaminophen showed that there was an effective response.111 Faulkner-Jones and his team made use of inkjet bioprinting technology to successfully get hepatocyte-like cells (HLCs) differentiated from human induced pluripotent stem cells (HiPSCs) and human embryonic stem cells (HESCs)112 based on cell printing. They found that the cells they got could express nuclear factor 4α(4α) and secrete albumin. Based on this, those cells could be applied to construct mini livers for drug testing.112 For the first time, it has been demonstrated that HiPSCs have the possibility to be bio-printed without negatively affecting normal functions, such as cell viability and pluripotency. Lei and Wang et al. used a 3D bioprinter based on multi-nozzle extrusion to establish complex organ precursors with branched vascular systems, ADSCs, and primary hepatocytes. On the basis of their findings, they demonstrated that the four-nozzle cryodeposition fabrication technique can be used to generate liver-like organ structures.113 This study illustrates that this combinatorial technique can be used to fabricate vascularized constructs with predefined internal channels.
The significance of liver reconstruction using 3D printing as a complex and multifunctional substantive organ is self-evident. However, microfluidic organ chips or small liver lobes can only be manufactured using 3D printing technology, which can be used for high-throughput drug screening or model construction, and it is still in the laboratory research stage. Considerable effort and time are still required for precipitation to produce a fully functional liver with a solid structure.
3.6 Kidneys
Kidneys are an important organ of the body to filter metabolic waste and maintain water–electrolyte balance and acid–base balance. At present, patients with end-stage renal disease still rely on dialysis treatment in addition to waiting for kidney transplantation. Therefore, providing a functional and biocompatible artificial kidney for these patients is challenging but worthwhile.
Based on the extruded 3D printing technology, Ali et al. mixed gelatin, hyaluronic acid, glycerol and extracellular matrix of normal decellularized renal cells to prepare photocrosslinked bioink for 3D printing.114 Then, the primary kidney cells were used to print an in vitro kidney model. The cells in the model had good cellular activity and had the structural and functional characteristics of natural kidney tissue.
The kidney has an extremely complex vascular structure to participate in its normal physiological function. Suhun Chae et al. used vascular corrosion casting technology to develop a bionic renal vascular stent,115 which can effectively promote blood vessel formation.
The proximal tubules are involved in the absorption and transport of 65% to 80% of the nutrients in the glomerular filtrate into the blood, including the reabsorption of salt, water, glucose, and amino acids and the secretion of metabolites. Its abnormality can lead to renal Fanconi syndrome with hydrocapnia, amino aciduria, phosphoruria and uricuria.116 Kimberly A. Homan et al. successfully constructed kidney proximal tubules in vitro using 3D printing technology.117 These tubules are composed of numerous proximal tubular epithelial cells encasing open luminal structures. The results showed that these artificial proximal tubules had normal physiological functions and could be used for the analysis of drug metabolism and the aid extracorporeal dialysis. Lin et al. successfully constructed vascularized proximal tubules with a diameter of 250 μm using an extrusion based 3D printing technique using a gelatin–fibronectin bioink.118 Co-culture of the proximal tubular cells with endothelial cells revealed their active albumin and glucose reabsorption abilities. In addition, hyperglycemia experiments show that it can be involved in monitoring endothelial cellular dysfunction, which provides different ways for studying renal function in vitro by constructing disease models and pharmacological applications. Addario et al. isolated primary mouse renal tubules (PmTECs), endothelial cells and fibroblasts, and embedded them in alginate bioink to establish a bionic proximal tubular core–shell tube with a diameter of 450 μm in vitro.119 Its function is maintained for nearly a month and it exhibits functions that support cell survival and metabolic activity.
Although bioengineered kidneys are still far from applications such as clinical organ transplantation based on current research on 3D bioprinted kidney models, recent developments have demonstrated their potential in kidney tissue engineering.
At present, bio-3D printing for organ reconstruction has evolved from single-component printing to multi-component printing. From single organization to multi-organization collaborative printing development, progression from unresponsiveness to stimulus responsiveness. It is believed that in the near future, printing of complete organs will also become a reality.
4. Recent advances and challenges of bio-engineering and cell therapy interacting with 3D bioprinting and related technologies
In the past few years, the intercrossing of 3D bioprinting technology and other technologies has also produced a variety of new devices and applications. For instance, the combination of electrospinning technology and bio-3D printing technology helps to construct tissue repair scaffolds on micro- and nanosurfaces to improve the bionic effect and biocompatibility of scaffolds. Combined with 3D bioprinting technology, cell-loaded injectable microspheres have been applied to construct high-cell loads and modular bio-inks to prepare functional tissue repair scaffolds.
4.1 Organs-on-chips and biological micromaterials
In terms of organ reconstruction, with the continuous deepening of basic research on the complex regulation of organ development, basic research combining organoid models, organ-on-a-chip technology, and bio-3D printing technology is becoming a hotspot. The continuous development of microfluidic chip technology has indicated an influential trend in the construction of bionic microorgans on chips to improve drug evaluation and other biomedical research. The integration of 3D printing technology in microtissue construction and organ reconstruction with microfluidic chip technology has presented a huge advantage in research on organ disease mechanisms and the development and evaluation of related drugs.120 The dynamic perfusion characteristics of organ chips make them different from traditional cultures. Zohar et al. and their team designed a high-throughput vascular engineering chip to study the relationship between the liquid perfusion environment, epithelial cell migration, and vascular network generation.121 They noted that under appropriate perfusion conditions, the formation rate of blood vessels was twice that under static conditions, which proved the importance of dynamic perfusion conditions in organ model culture. Apart from that, for the purpose of carrying out some micro-level research, catering to some specific needs of certain cells, the structure of the tiny organ-on-a-chip shows another advantage. Yamamoto et al. with their team members developed a mutually separated dual-chamber culture system (divided into nerve and muscle culture chambers) and a micron-level flow channel connecting the two chambers,122 through which nerve axons can be guided to grow from the nerve culture chamber into the muscle culture chamber to form a neuromuscular unit. By virtue of the independent cultivation of nerves and muscles, it has the disadvantage that under the traditional muscle and nerve co-culture system, it is difficult to separate and control these two parts, which also confirms that the tiny neuromuscular junction unit can be used to realize the need for drug testing related to myasthenia gravis, and devise a new method for drug screening. With the help of 3D printing technology, Lind et al. built a resistance sensor on a chip by mixing various materials as ink, which can calculate the contractility of myocardial tissue according to the change in resistance and constantly collect related data on myocardial tissues in a visual, non-invasive, and convenient way, and it can be used to study the effects of myocardial drugs.123
With the rapid development of organ chips, microfluidic platforms provide good culture conditions for organoids with high precision, dynamic perfusion, small size, high throughput, and can also be more sophisticated. Nonetheless, the “organ” part, which is the main body of the organ chip, remains in the 2D or 2.5D stage, and single-layer cell culture of most organ chips still hinders the development of the intersected field of 3D printing and medicine.4 How to reestablish the natural complex 3D organ structure is still a key factor restricting the development of organ chips, but this may be well solved by bioprinting technology.
4.2 Stem cell and organoid technology
With the continuous improvement and expansion of treatment methods, cell therapy has gradually emerged. Cells are the basic structural and functional unit of the organism, and parenchymal organs are composed of cells, which are the main functional units. The liver is an important organ. Most studies have shown that impaired liver function can be corrected by the infusion of normal liver cells. The New England Journal reported a case124 that the injection of normal primary human liver cells into a 10-year-old girl presented with Crigler-Najjar syndrome type I not only corrected hyperbilirubinemia for 11 months but also had no significant adverse effects on her metabolism. Since then, various hepatocellular therapies based on cell infusion have been developed.
The use of primary cells, which are undoubtedly the first choice of therapy, has recently achieved remarkable results. Some researchers have injected hepatocytes into parts such as the spleen and lymph nodes, and used them as incubators for the extrahepatic growth of hepatocytes, so that they can produce functional hepatocytes in vivo, and even replace the original diseased liver to achieve an effect similar to liver transplantation. Currently, many researchers have conducted relevant experiments. In 2020, Dr. Lintao Wang and others at Nanjing University published a paper in Science Advances titled: research paper on transforming the spleen into a liver-like organ in vivo.125 The spleen was successfully engineered into a liver-like organ with typical liver function in live mice. This is the first attempt in a small mammal. Researchers from the University of Pittsburgh and West Virginia University have published a study in the journal Liver Transplantation titled Development of Ectopic Livers by Hepatocyte Transplantation into Swine Lymph Nodes. The team isolated hepatocytes from the left liver lobe of pigs with acute liver failure and transplanted them directly into the mesenteric lymph nodes via injection.126 Hepatocytes in the lymph nodes were successfully verified in all transplanted animals 30 to 60 days after transplantation, and the ectopic livers developing within the lymph nodes showed histological features characteristic of porcine liver lobules, including sinusoids and bile duct formation.126 This study shows that large animals with liver disease can grow new organs in the lymph nodes from their own liver cells, followed by human clinical trials. The corresponding author of the article, Professor Eric Lagasse and his team have successfully developed new miniature livers in mice, pigs and dogs in order to further promote the clinical translation of the results. Primary cells are convenient and functional, but the capability of expansion of the primary cells is limited, which means that they will lose their normal phenotype and markers in vitro in a short time, limiting their large-scale use. This raises the question of where the cells should originate. From the perspective of functional maintenance, primary human hepatocytes are undoubtedly the first choice, but the expansion of primary hepatocytes in vitro is limited.127 Stem cell technology is a good source of cells for cell transplantation therapy and organ reconstruction. Stem cells have self-renewal ability and the potential for multi-differentiation, which means that under certain interferences, they have potential to differentiate into various cells with their normal functions. Stem cells are divided into embryonic stem cells (ES cells) and somatic stem cells, according to their developmental stage. Stem cells can be divided into three types according to their developmental potential: potent, pluripotent, and unipotent.128
The induction of stem cells into hepatoid cells in a specific growth environment in vitro can meet the requirements of quantity and function, including cell transplantation and liver scaffold filling. At present, more research studies are focused on embryonic stem cells and induced pluripotent stem cells, and iPS cells are more patient-specific, with the same ethical concerns as embryonic stem cells, and have good genetic stability, so they are currently a high-quality source of cells for various liver studies.127,129 However, to some extent, the induced hepatocytes are not mature enough, the induction time is long, the cost is high, and the existence of tumor formation is the key to limiting further study of such cells.127 Researchers in various countries have long used human pluripotent stem cells (including embryonic stem cells and induced pluripotent stem cells) as seeds to prepare liver cells in vitro. However, the liver contains more than 1011 liver parenchymal cells and approximately 1010 bile duct epithelial cells, and a large-scale culture system capable of producing such a large number of cells has not yet been established. The expected preparation cost is staggering; only the reagents and consumables required for cell proliferation and differentiation require tens of millions of yuan, any remaining undifferentiated cells may also form tumors in the body, and the liver cells derived from pluripotent stem cells have a considerable gap with adult cells in both purity and function. In response to such problems, most scholars have continuously updated the induction program, based on the normal development process of hepatocytes, and changed the induction environment to promote the maturation of hepatoid cells and shorten the time required for induction,130 thus improving efficiency. Earlier this year, Xin Cheng of the Chinese Academy of Sciences, in collaboration with Professor Xu Chengran and Luonan Chen of the Peking University School of Biology and Professor Yin Hao of Shanghai Changzheng Hospital, established a new type of stem cell line, the human endoderm stem cell line.131 These seed cells are derived from pluripotent stem cells, but are closer to mature cells. In addition to retaining the advantages of infinite proliferation in vitro and the establishment of individual patient cell lines, the outstanding feature of these cells is that they can be efficiently transformed into liver, pancreas, small intestine, and other cells in vitro, making them an ideal seed cell for large-scale preparation of liver cells in vitro.131 Subsequent research published in Nature by Professor Ding Sheng's team at Tsinghua University also provided another way of thinking, and Professor Ding's team found a new drug combination in vitro-TTNPB, 1-azakenpaullone, and WS6, these three small molecule drug combinations (TAW).132 Mouse pluripotent stem cells (PSCs) can be induced into TotiSCs with the potential to transform into complete organisms, and the omnipotency (intra- and extra-embryonic differentiation potential) of these induced cells can be maintained in the laboratory. The team named these cells ciTotiSCs (chemically induced totipotent stem cells).132 This innovative alternative route to obtaining the earliest starting cells from more mature cells, rather than using germ cells (sperm and eggs), has broad implications. This discovery is not only conducive to the study of the origin of life but also provides a way of thinking, using the cells closer to the beginning of life as the initial stage of induction, we tested whether they can be made to fit the liver development process at an earlier stage, so that subsequent induction can obtain cells closer to mature liver cells.
When discussing the development of stem cells, we must mention the emergence of organoids. Organoids are “similar” organ-like organs produced by 3D culture of stem cells in vitro, which have the ability to undergo self-renewal and self-organization, and their structure and function are highly similar to the source tissues or organs. The most important characteristic of organoids is that stem cells have high tissue memory and self-assembly ability in vitro, can grow into structures similar to in vivo tissues, highly restore the cell composition and function of internal organs, and maintain genetic and phenotypic stability during long-term expansion.133 The development of organoid technology has facilitated in-depth research on disease modeling, drug development, precision medicine, developmental biology, pathogenic microbiome host interactions, toxicology, gene editing, and regenerative medicine.
As the field of 3D organ bioprinting continues to advance, though promising solutions for organ transplantation and regenerative medicine can be offered, it is accompanied by a variety of regulatory challenges. To begin with, safety and efficacy standards, one of the primary concerns surrounding 3D organ bioprinting, requires developing a regulatory framework that ensures the quality and reliability of bioprinted organs, which is crucial to protect patients and promote public trust. This involves determining appropriate testing methodologies, establishing criteria for validating the bioprinted organs’ functionality and durability, and defining acceptable levels of risk. Secondly, bioprinting involves complex manufacturing processes, including the selection of suitable bioinks, cell sources, and scaffold materials, as well as the optimization of printing parameters. Developing standardized protocols and guidelines while accommodating technological advancements can help streamline the regulatory assessment of bioprinting procedures. Thirdly, the intersection of bioprinting, stem cell research, and tissue engineering raises complex intellectual property (IP) issues. Clear guidelines and policies on IP protection specific to bioprinting need to be defined to encourage innovation while preventing undue monopolization and fostering collaboration among researchers. Apart from that, ethical considerations of the bioprinting process are also a key point to regulate. Bioprinting raises ethical questions related to the use of human cells, animal testing, and the creation of hybrid or chimeric organs. Researchers must ensure that the sourcing of cells and materials adheres to ethical guidelines, protect animal welfare during experimentation, and assess the implications of creating novel biological entities. Public engagement and interdisciplinary discussions involving scientists, ethicists, and policymakers are essential to strike a balance between scientific progress and ethical boundaries. Last but not least., the development and commercialization of bioprinted organs may involve multiple regulatory agencies, including those responsible for medical devices, pharmaceuticals, and tissue engineering. Achieving regulatory harmonization between these agencies is crucial to avoid duplication, streamline the approval process, and ensure consistent oversight. Collaborative efforts at the national and international levels are necessary to establish unified regulatory frameworks that address the unique challenges of bioprinting.
4.3 Challenges and future outlook
Due to its controllable and reproducible manner, 3D bioprinting can accurately manipulate bio-materials or bioinks for the fabrication of microstructures, which can be used for in vitro studies in the field of cell biology, tissue engineering and drug testing. The increasing number of bio-materials and bioprinting technologies can not only promote the fabrication of microstructures, but also produce an enormous workload for researchers studying the optimization of biomaterials and process parameters as well as the evaluation of the effects they produce during the printing process. For instance, during the process of bioprinting, the materials or bioinks should be calculated according to the printing purpose and the whole process should be supervised about the qualification and so on.134 Accordingly, as mentioned before, the mathematical models or scientific research equations are inefficient. In order to work out this insufficiency, deep learning (DL)/machine learning (ML) methods provide a promising solution. Deep learning is a new research direction in the field of machine learning. The motivation is to build a model that simulates the neural connection structure of the human brain with the goal of making machines able to analyze and learn like humans, able to recognize data such as text, images and sound, that is, artificial intelligence. In terms of the applications in the biomedical field, DL/ML has already cut a striking figure, mainly from the following aspects:
4.3.1 Bioink development.
(a) Material characterization: deep learning algorithms can analyze large datasets of material properties to identify patterns and correlations that contribute to the desired characteristics of bioinks. This can aid in the formulation of bioinks with optimized mechanical, rheological, and biological properties.
(b) Predictive modeling: machine learning models can be trained to predict the behavior and properties of bioinks based on their composition, facilitating the design of bioinks tailored for specific printing applications.134
4.3.2 Printing optimization.
(a) Print path planning: deep learning algorithms can optimize the printing process by learning from large datasets of printing parameters and outcomes to generate efficient print paths. This can improve printing speed, accuracy, and structural integrity.
(b) Parameter optimization: machine learning techniques can analyze data from previous printing experiments to identify optimal printing parameters, such as the nozzle temperature, printing speed, and layer thickness, leading to improved print quality and reproducibility.
4.3.3 Tissue modeling and design.
(a) Tissue structure prediction: deep learning models can analyze medical imaging data (e.g., MRI or CT scans) to predict and reconstruct the 3D structure of tissues or organs. This information can guide the design and printing of complex tissue constructs.
(b) Scaffold design: machine learning algorithms can assist in the design of scaffolds by learning from the existing tissue-engineering literature and databases, enabling the generation of optimized scaffold geometries that promote cell viability, proliferation, and tissue integration.135
4.3.4 Quality control and process monitoring.
(a) Defect detection: deep learning algorithms can analyze high-resolution images of bioprinted constructs to identify defects, such as structural inconsistencies or cell distribution irregularities. This enables real-time quality control during the printing process.
(b) Process monitoring: machine learning models can monitor process parameters and sensor data in real-time to detect deviations or anomalies, allowing for timely adjustments and ensuring consistent print quality.136
4.3.5 Data analysis and prediction.
(a) Biomaterial–cell interactions: deep learning techniques can analyze large-scale omics data (e.g., genomics, proteomics) to elucidate the complex interactions between biomaterials and cells, helping to optimize bioink compositions and improve tissue integration.
(b) Tissue function assessment: machine learning algorithms can analyze functional data, such as electromechanical or biochemical responses, to predict the functionality, maturity, and long-term behavior of bioprinted tissues.137
5. Conclusion
3D bioprinting, as a product of the integration of material engineering, biomedicine and even clinical medicine, shines brightly in the medical field. From biological scaffolds to cell therapy, organ chip research and development, and 3D printed organ reconstruction, 3D bioprinting has gradually emerged in the field of organ transplantation. The emergence of 3D printed ears, miniature hearts, artificial lungs, miniature livers, etc. not only confirms the possibility of 3D printed organs, but also makes a good start for the further development of 3D bioprinting. At present, the reconstruction of most organs, especially solid organs, is still in the laboratory stage, and it is still faced with many problems such as immune response, vascularization, multi-tissue printing, and bionic structures, and there is still a long way to go to truly make 3D printed products that can replace existing organs. However, these are both challenges and opportunities. We believe that in the near future, bioprinting can lead to breakthroughs in artificial organ preparation and promote the development of organ transplantation and personalized medicine.
Author contributions
Guobin Huang and Bo Yang jointly conceptualized the focus of this review, conducted literature research and synthesis, and reviewed the final manuscript. Dong Chen and Lai Wei contributed their expertise in specific fields and authored relevant sections. Yuanyuan Zhao and Zhiping Hu conducted statistical analyses of the data presented in the review and created data visualizations. Junbo Li and Xi Zhou primarily organized and ordered the literature. Zhishui Chen oversaw the peer-review and revision process. All authors have read and approved the final version of the manuscript, contributing collaboratively to this research.
Conflicts of interest
The authors declare no competing interests.
Acknowledgements
The authors acknowledge the research community for their contributions to the field, which have provided valuable insights for this study. This study was supported by grants from the Health Commission of Hubei Province (funder: ZS.C; Grant No. WJ2021C001), Key Research and Development Plan of Hubei Province (funder: ZS.C; Grant No. 2022BCA015), National Natural Science Foundation of China (funder: B.Y; Grant No. 82000602) and Knowledge Innovation Program of Wuhan-Shuguang Project (funder: B.Y; Grant No. 2022020801020448).
References
- H. G. Yi, H. Kim, J. Kwon, Y. J. Choi, J. Jang and D. W. Cho, Application of 3D bioprinting in the prevention and the therapy for human diseases, Signal Transduction Targeted Ther., 2021, 6(1), 177, DOI:10.1038/s41392-021-00566-8.
- N. Goldaracena, J. M. Cullen, D. S. Kim, B. Ekser and K. J. Halazun, Expanding the donor pool for liver transplantation with marginal donors, Int. J. Surg., 2020, 82S, 30–35, DOI:10.1016/j.ijsu.2020.05.024.
- T. Ikegami and Y. Maehara, Transplantation: 3D printing of the liver in living donor liver transplantation, Nat. Rev. Gastroenterol. Hepatol., 2013, 10(12), 697–698, DOI:10.1038/nrgastro.2013.195.
- W. Huang, Frontier hotspots and research progress on 3D bioprinting in organ reconstruction, Organ Transplant., 2022, 13(2), 161–168 Search PubMed.
- C. Y. Liaw and M. Guvendiren, Current and emerging applications of 3D printing in medicine, Biofabrication, 2017, 9(2), 024102, DOI:10.1088/1758-5090/aa7279.
- H. Q. Xu, J. C. Liu, Z. Y. Zhang and C. X. Xu, A review on cell damage, viability, and functionality during 3D bioprinting, Mil. Med. Res., 2022, 9(1), 70, DOI:10.1186/s40779-022-00429-5.
- M. Dey and I. T. Ozbolat, 3D bioprinting of cells, tissues and organs, Sci. Rep., 2020, 10(1), 14023, DOI:10.1038/s41598-020-70086-y.
- G. Pennarossa, S. Arcuri and T. De Iorio,
et al., Current Advances in 3D Tissue and Organ Reconstruction, Int. J. Mol. Sci., 2021, 22(2), 830, DOI:10.3390/ijms22020830.
- W. Zhu, X. Ma and M. Gou,
et al., 3D printing of functional biomaterials for tissue engineering, Curr. Opin. Biotechnol., 2016, 40, 103–112, DOI:10.1016/j.copbio.2016.03.014.
- S. Vijayavenkataraman, W. C. Yan and W. F. Lu,
et al., 3D bioprinting of tissues and organs for regenerative medicine, Adv. Drug Delivery Rev., 2018, 132, 296–332, DOI:10.1016/j.addr.2018.07.004.
- J. Zhang, E. Wehrle and M. Rubert,
et al., 3D Bioprinting of Human Tissues: Biofabrication, Bioinks, and Bioreactors, Int. J. Mol. Sci., 2021, 22(8), 3971, DOI:10.3390/ijms22083971.
- I. Matai, G. Kaur and A. Seyedsalehi,
et al., Progress in 3D bioprinting technology for tissue/organ regenerative engineering, Biomaterials, 2020, 226, 119536, DOI:10.1016/j.biomaterials.2019.119536.
- P. Calvert, Inkjet printing for materials and devices, Chem. Mater., 2001, 13(10), 3299–3305, DOI:10.1021/cm0101632.
- V. Mironov, Printing technology to produce living tissue, Expert Opin. Biol. Ther., 2003, 3(5), 701–704, DOI:10.1517/14712598.3.5.701.
- R. F. Pereira and P. J. Bártolo, 3D bioprinting of photocrosslinkable hydrogel constructs, J. Appl. Polym. Sci., 2015, 132(48), 15, DOI:10.1002/APP.42458.
- S. Wüst, M. E. Godla and R. Müller,
et al., Tunable hydrogel composite with two-step processing in combination with innovative hardware upgrade for cell-based three-dimensional bioprinting, Acta Biomater., 2014, 10(2), 630–640, DOI:10.1016/j.actbio.2013.10.016.
- H. N. Chia and B. M. Wu, Recent advances in 3D printing of biomaterials, J. Biol. Eng., 2015, 9, 4, DOI:10.1186/s13036-015-0001-4.
- R. E. Saunders and B. Derby, Inkjet printing biomaterials for tissue engineering: bioprinting, Int. Mater. Rev., 2014, 59(8), 430–448, DOI:10.1179/1743280414Y.0000000040.
- T. Boland, T. Xu and B. Damon,
et al., Application of inkjet printing to tissue engineering, Biotechnol. J., 2006, 1(9), 910–917, DOI:10.1002/biot.200600081.
- A. N. Leberfinger, D. J. Ravnic and A. Dhawan,
et al., Concise Review: Bioprinting of Stem Cells for Transplantable Tissue Fabrication, Stem Cells Transl. Med., 2017, 6(10), 1940–1948, DOI:10.1002/sctm.17-0148.
- P. Ihalainen, A. Määttänen and N. Sandler, Printing technologies for biomolecule and cell-based applications, Int. J. Pharm., 2015, 494(2), 585–592, DOI:10.1016/j.ijpharm.2015.02.033.
- R. E. Saunders, J. E. Gough and B. Derby, Delivery of human fibroblast cells by piezoelectric drop-on-demand inkjet printing, Biomaterials, 2008, 29(2), 193–203, DOI:10.1016/j.biomaterials.2007.09.032.
-
R. Saunders, J. Gough and B. Derby, Symposium on Nanoscale Materials Science in Biology and Medicine held at the 2004 MRS Fall Meeting, Nov 28–Dec 02 2004: Ink jet printing of mammalian primary cells for tissue engineering applications, Materials Research Society Boston, MA, 2005, pp. 57–62.
- C. B. Arnold, P. Serra and A. Piqué, Laser direct-write techniques for printing of complex materials, MRS Bull., 2007, 32(1), 23–31, DOI:10.1557/mrs2007.11.
- D. B. Chrisey, MATERIALS PROCESSING: The Power of Direct Writing, Science, 2000, 289(5481), 879–881, DOI:10.1126/science.289.5481.879.
- M. Colina, P. Serra and J. M. Fernández-Pradas,
et al., DNA deposition through laser induced forward transfer, Biosens. Bioelectron., 2005, 20(8), 1638–1642, DOI:10.1016/j.bios.2004.08.047.
- V. Dinca, E. Kasotakis and J. Catherine,
et al., Directed three-dimensional patterning of self-assembled peptide fibrils, Nano Lett., 2008, 8(2), 538–543, DOI:10.1021/nl072798r.
- J. A. Barron, P. Wu and H. D. Ladouceur,
et al., Biological laser printing: a novel technique for creating heterogeneous 3-dimensional cell patterns, Biomed. Microdevices, 2004, 6(2), 139–147, DOI:10.1023/b:bmmd.0000031751.67267.9f.
- V. Keriquel, H. Oliveira and M. Rémy,
et al., In situ printing of mesenchymal stromal cells, by laser-assisted bioprinting, for in vivo bone regeneration applications, Sci. Rep., 2017, 7(1), 1778, DOI:10.1038/s41598-017-01914-x.
- F. Guillemot, A. Souquet and S. Catros,
et al., Laser-assisted cell printing: principle, physical parameters versus cell fate and perspectives in tissue engineering, Nanomedicine, 2010, 5(3), 507–515, DOI:10.2217/nnm.10.14.
- B. R. Ringeisen, C. M. Othon and J. A. Barron,
et al., Jet-based methods to print living cells, Biotechnol. J., 2006, 1(9), 930–948, DOI:10.1002/biot.200600058.
- S. Catros, B. Guillotin and M. Bacáková,
et al., Effect of laser energy, substrate film thickness and bioink viscosity on viability of endothelial cells printed by Laser-Assisted Bioprinting, Appl. Surf. Sci., 2011, 257(12), 5142–5147, DOI:10.1016/j.apsusc.2010.11.049.
- F. Guo, Z. Mao and Y. Chen,
et al., Three-dimensional manipulation of single cells using surface acoustic waves, Proc. Natl. Acad. Sci. U. S. A., 2016, 113(6), 1522–1527, DOI:10.1073/pnas.1524813113.
- U. Demirci, Acoustic picoliter droplets for emerging applications in semiconductor industry and biotechnology, J. Microelectromech. Syst., 2006, 15(4), 957–966, DOI:10.1109/JMEMS.2006.878879.
- U. Demirci and G. Montesano, Single cell epitaxy by acoustic picolitre droplets, Lab Chip, 2007, 7(9), 1139–1145, 10.1039/b704965j.
- V. Chan, P. Zorlutuna and J. H. Jeong,
et al., Three-dimensional photopatterning of hydrogels using stereolithography for long-term cell encapsulation, Lab Chip, 2010, 10(16), 2062–2070, 10.1039/c004285d.
- G. Mapili, Y. Lu and S. Chen,
et al., Laser-layered microfabrication of spatially patterned functionalized tissue-engineering scaffolds, J. Biomed. Mater. Res., Part B, 2005, 75(2), 414–424, DOI:10.1002/jbm.b.30325.
- K. C. Hribar, P. Soman and J. Warner,
et al., Light-assisted direct-write of 3D functional biomaterials, Lab Chip, 2014, 14(2), 268–275, 10.1039/c3lc50634g.
- V. B. Morris, S. Nimbalkar and M. Younesi,
et al., Mechanical Properties, Cytocompatibility and Manufacturability of Chitosan: PEGDA Hybrid-Gel Scaffolds by Stereolithography, Ann. Biomed. Eng., 2017, 45(1), 286–296, DOI:10.1007/s10439-016-1643-1.
- R. Gauvin, Y. C. Chen and J. W. Lee,
et al., Microfabrication of complex porous tissue engineering scaffolds using 3D projection stereolithography, Biomaterials, 2012, 33(15), 3824–3834, DOI:10.1016/j.biomaterials.2012.01.048.
- Z. Wang, R. Abdulla and B. Parker,
et al., A simple and high-resolution stereolithography-based 3D bioprinting system using visible light crosslinkable bioinks, Biofabrication, 2015, 7(4), 045009, DOI:10.1088/1758-5090/7/4/045009.
- S. J. Lee, M. Nowicki and B. Harris,
et al., Fabrication of a Highly Aligned Neural Scaffold via a Table Top Stereolithography 3D Printing and Electrospinning, Tissue Eng., Part A, 2017, 23(11–12), 491–502, DOI:10.1089/ten.TEA.2016.0353.
- Y. Wu, H. Su, M. Li and H. Xing, Digital light processing-based multi-material bioprinting: Processes, applications, and perspectives, J. Biomed. Mater. Res., Part A, 2023, 111(4), 527–542, DOI:10.1002/jbm.a.37473.
- W. Li, M. Wang and H. Ma,
et al., Stereolithography apparatus and digital light processing-based 3D bioprinting for tissue fabrication, iScience, 2023, 26(2), 106039, DOI:10.1016/j.isci.2023.106039.
- F. Verisqa, J. R. Cha and L. Nguyen,
et al., Digital Light Processing 3D Printing of Gyroid Scaffold with Isosorbide-Based Photopolymer for Bone Tissue Engineering, Biomolecules, 2022, 12(11), 1692, DOI:10.3390/biom12111692.
- S. Kumari, P. Mondal and K. Chatterjee, Digital light processing-based 3D bioprinting of κ-carrageenan hydrogels for engineering cell-loaded tissue scaffolds, Carbohydr. Polym., 2022, 290, 119508, DOI:10.1016/j.carbpol.2022.119508.
- V. T. Duong and C. C. Lin, Digital Light Processing 3D Bioprinting of Gelatin-Norbornene Hydrogel for Enhanced Vascularization, Macromol. Biosci., 2023, 23(12), e2300213, DOI:10.1002/mabi.202300213.
- A. Ruggiero, V. Criscuolo and S. Grasselli,
et al., Two-photon polymerization lithography enabling the fabrication of PEDOT:PSS 3D structures for bioelectronic applications, Chem. Commun., 2022, 58(70), 9790–9793, 10.1039/d2cc03152c.
- J. Song, C. Michas and C. S. Chen,
et al., From Simple to Architecturally Complex Hydrogel Scaffolds for Cell and Tissue Engineering Applications: Opportunities Presented by Two-Photon Polymerization, Adv. Healthc. Mater., 2020, 9(1), e1901217, DOI:10.1002/adhm.201901217.
- T. Li, J. Liu and M. Guo,
et al., Synthesis of biocompatible BSA-GMA and two-photon polymerization of 3D hydrogels with free radical type I photoinitiator, Int. J. Bioprint., 2023, 9(5), 752, DOI:10.18063/ijb.752.
- K. S. Worthington, A. V. Do and R. Smith,
et al., Two-Photon Polymerization as a Tool for Studying 3D Printed Topography-Induced Stem Cell Fate, Macromol. Biosci., 2019, 19(2), e1800370, DOI:10.1002/mabi.201800370.
- W. L. Haisler, D. M. Timm and J. A. Gage,
et al., Three-dimensional cell culturing by magnetic levitation, Nat. Protoc., 2013, 8(10), 1940–1949, DOI:10.1038/nprot.2013.125.
- H. Tseng, J. A. Gage and R. M. Raphael,
et al., Assembly of a three-dimensional multitype bronchiole coculture model using magnetic levitation, Tissue Eng., Part C, 2013, 19(9), 665–675, DOI:10.1089/ten.TEC.2012.0157.
- H. Tseng, L. R. Balaoing and B. Grigoryan,
et al., A three-dimensional co-culture model of the aortic valve using magnetic levitation, Acta Biomater., 2014, 10(1), 173–182, DOI:10.1016/j.actbio.2013.09.003.
- J. M. Lee, S. L. Sing and M. Zhou,
et al., 3D bioprinting processes: A perspective on classification and terminology, Int. J. Bioprint., 2018, 4(2), 151, DOI:10.18063/IJB.v4i2.151.
- A. R. Abdel Fattah, E. Meleca and S. Mishriki,
et al., In Situ 3D Label-Free Contactless Bioprinting of Cells through Diamagnetophoresis, ACS Biomater. Sci. Eng., 2016, 2(12), 2133–2138, DOI:10.1021/acsbiomaterials.6b00614.
- B. R. Whatley, X. Li and N. Zhang,
et al., Magnetic-directed patterning of cell spheroids, J. Biomed. Mater. Res., Part A, 2014, 102(5), 1537–1547, DOI:10.1002/jbm.a.34797.
- H. Tseng, J. A. Gage and T. Shen,
et al., A spheroid toxicity assay using magnetic 3D bioprinting and real-time mobile device-based imaging, Sci. Rep., 2015, 5, 13987, DOI:10.1038/srep13987.
- G. R. Souza, H. Tseng and J. A. Gage,
et al., Magnetically Bioprinted Human Myometrial 3D Cell Rings as A Model for Uterine Contractility, Int. J. Mol. Sci., 2017, 18(4), 683, DOI:10.3390/ijms18040683.
- S. Hou, H. Tiriac and B. P. Sridharan,
et al., Advanced Development of Primary Pancreatic Organoid Tumor Models for High-Throughput Phenotypic Drug Screening, SLAS Discovery, 2018, 23(6), 574–584, DOI:10.1177/2472555218766842.
- P. Baillargeon, J. Shumate and S. Hou,
et al., Automating a Magnetic 3D Spheroid Model Technology for High-Throughput Screening, SLAS Technol., 2019, 24(4), 420–428, DOI:10.1177/2472630319854337.
- H. Q. Xu, J. C. Liu and Z. Y. Zhang, A review on cell damage, viability, and functionality during 3D bioprinting, Mil. Med. Res., 2022, 9(1), 70, DOI:10.1186/s40779-022-00429-5.
- S. Boularaoui, G. A. Hussein and K. A. Khan,
et al., An overview of extrusion-based bioprinting with a focus on induced shear stress and its effect on cell viability, Bioprinting, 2020, 20(24), e00093, DOI:10.1016/j.bprint.2020.e00093.
- R. Chand, B. S. Muhire and S. Vijayavenkataraman, Computational Fluid Dynamics Assessment of the Effect of Bioprinting Parameters in Extrusion Bioprinting, Int. J. Bioprint., 2022, 8(2), 545, DOI:10.18063/ijb.v8i2.545.
- M. Müller, E. Öztürk and Ø. Arlov,
et al., Alginate Sulfate-Nanocellulose Bioinks for Cartilage Bioprinting Applications, Ann. Biomed. Eng., 2017, 45(1), 210–223, DOI:10.1007/s10439-016-1704-5.
- Q. Gao, Z. Liu and Z. Lin,
et al., 3D Bioprinting of Vessel-like Structures with Multilevel Fluidic Channels, ACS Biomater. Sci. Eng., 2017, 3(3), 399–408, DOI:10.1021/acsbiomaterials.6b00643.
- R. E. Saunders and B. Derby, Inkjet printing biomaterials for tissue engineering: bioprinting, Int. Mater. Rev., 2014, 59(8), 430–448, DOI:10.1179/1743280414Y.0000000040.
- S. V. Murphy and A. Atala, 3D bioprinting of tissues and organs, Nat. Biotechnol., 2014, 32(8), 773–785, DOI:10.1038/nbt.2958.
- W. L. Ng, X. Huang and V. Shkolnikov,
et al., Polyvinylpyrrolidone-based bioink: influence of bioink properties on printing performance and cell proliferation during inkjet-based bioprinting, Bio-Des. Manuf., 2023, 6(6), 676–690, DOI:10.1007/s42242-023-00245-3.
- W. L. Ng, X. Huang and V. Shkolnikov, Controlling Droplet Impact Velocity and Droplet Volume: Key Factors to Achieving High Cell Viability in Sub-Nanoliter Droplet-based Bioprinting, Int. J. Bioprint., 2021, 8(1), 424, DOI:10.18063/ijb.v8i1.424.
- C. Xu, W. Chai and Y. Huang, Scaffold-free inkjet printing of three-dimensional zigzag cellular tubes, Biotechnol. Bioeng., 2012, 109(12), 3152–3160, DOI:10.1002/bit.24591.
- K. Christensen, C. Xu and W. Chai, Freeform inkjet printing of cellular structures with bifurcations, Biotechnol. Bioeng., 2015, 112(5), 1047–1055, DOI:10.1002/bit.25501.
- N. A. Chartrain, C. B. Williams and A. R. Whittington, A review on fabricating tissue scaffolds using vat photopolymerization, Acta Biomater., 2018, 74, 90–111, DOI:10.1016/j.actbio.2018.05.010.
- L. Herschdorfer, W. M. Negreiros and G. O. Gallucci,
et al., Comparison of the accuracy of implants placed with CAD-CAM surgical templates manufactured with various 3D printers: An in vitro study, J. Prosthet. Dent., 2021, 125(6), 905–910, DOI:10.1016/j.prosdent.2020.03.017.
- Z. Wang, R. Abdulla and B. Parker,
et al., A simple and high-resolution stereolithography-based 3D bioprinting system using visible light crosslinkable bioinks, Biofabrication, 2015, 7(4), 045009, DOI:10.1088/1758-5090/7/4/045009.
- W. L. Ng, J. M. Lee and M. Zhou,
et al., Vat polymerization-based bioprinting-process, materials, applications and regulatory challenges, Biofabrication, 2020, 12(2), 022001, DOI:10.1088/1758-5090/ab6034.
- P. Zhang, H. Wang and P. Wang,
et al., Lightweight 3D bioprinting with point by point photocuring, Bioact. Mater., 2020, 6(5), 1402–1412, DOI:10.1016/j.bioactmat.2020.10.023.
- J. K. Placone and A. J. Engler, Recent Advances in Extrusion-Based 3D Printing for Biomedical Applications, Adv. Healthc. Mater., 2018, 7(8), e1701161, DOI:10.1002/adhm.201701161.
- J. Zhang, E. Wehrle and M. Rubert, 3D Bioprinting of Human Tissues: Biofabrication, Bioinks, and Bioreactors, Int. J. Mol. Sci., 2021, 22(8), 3971, DOI:10.3390/ijms22083971.
- A. Dravid, A. McCaughey-Chapman and B. Raos,
et al., Development of agarose-gelatin bioinks for extrusion-based bioprinting and cell encapsulation, Biomed. Mater., 2022, 17(5) DOI:10.1088/1748-605X/ac759f.
- P. Suwannakot, L. Zhu and M. A. K. Tolentino,
et al., Electrostatically Cross-Linked Bioinks for Jetting-Based Bioprinting of 3D Cell Cultures, ACS Appl. Bio Mater., 2024, 7(1), 269–283, DOI:10.1021/acsabm.3c00849.
- Z. Zhang, C. Xu and R. Xiong,
et al., Effects of living cells on the bioink printability during laser printing, Biomicrofluidics, 2017, 11(3), 034120, DOI:10.1063/1.4985652.
- M. Shah, A. Ullah and K. Azher,
et al., Vat photopolymerization-based 3D printing of polymer nanocomposites: current trends and applications, RSC Adv., 2023, 13(2), 1456–1496, 10.1039/d2ra06522c.
- A. C. Fonseca, F. P. W. Melchels and M. J. S. Ferreira,
et al., Emulating Human Tissues and Organs: A Bioprinting Perspective Toward Personalized Medicine, Chem. Rev., 2020, 120(19), 11128–11174, DOI:10.1021/acs.chemrev.0c00342.
- W. L. Ng, C. K. Chua and Y. F. Shen, Print me an organ! Why we are not there yet, Prog. Polym. Sci., 2019, 97, 101145, DOI:10.1016/j.progpolymsci.2019.101145.
- S. V. Murphy and A. Atala, 3D bioprinting of tissues and organs, Nat. Biotechnol., 2014, 32(8), 773–785, DOI:10.1038/nbt.2958.
- T. Weng, W. Zhang and Y. Xia,
et al., 3D bioprinting for skin tissue engineering: Current status and perspectives, J. Tissue Eng., 2021, 12, 20417314211028574, DOI:10.1177/20417314211028574.
- W. Lee, J. C. Debasitis and V. K. Lee,
et al., Multi-layered culture of human skin fibroblasts and keratinocytes through three-dimensional freeform fabrication, Biomaterials, 2009, 30(8), 1587–1595, DOI:10.1016/j.biomaterials.2008.12.009.
- L. Koch, S. Kuhn and H. Sorg,
et al., Laser printing of skin cells and human stem cells, Tissue Eng., Part C, 2010, 16(5), 847–854, DOI:10.1089/ten.TEC.2009.0397.
- L. Koch, A. Deiwick and S. Schlie,
et al., Skin tissue generation by laser cell printing, Biotechnol. Bioeng., 2012, 109(7), 1855–1863, DOI:10.1002/bit.24455.
- M. Albanna, K. W. Binder and S. V. Murphy,
et al., In Situ Bioprinting of Autologous Skin Cells Accelerates Wound Healing of Extensive Excisional Full-Thickness Wounds, Sci. Rep., 2019, 9(1), 1856, DOI:10.1038/s41598-018-38366-w.
- Y. Shi, T. L. Xing and H. B. Zhang,
et al., Tyrosinase-doped bioink for 3D bioprinting of living skin constructs, Biomed. Mater., 2018, 13(3), 035008, DOI:10.1088/1748-605X/aaa5b6.
- A. Skardal, D. Mack and E. Kapetanovic,
et al., Bioprinted amniotic fluid-derived stem cells accelerate healing of large skin wounds, Stem Cells Transl. Med., 2012, 1(11), 792–802, DOI:10.5966/sctm.2012-0088.
- J. J. Wu, T. Y. Zhu and Y. G. Lu,
et al., Hair follicle reformation induced by dermal papilla cells from human scalp skin, Arch. Dermatol. Res., 2006, 298(4), 183–190, DOI:10.1007/s00403-006-0686-9.
- C. A. Higgins, J. C. Chen and J. E. Cerise,
et al., Microenvironmental reprogramming by three-dimensional culture enables dermal papilla cells to induce de novo human hair-follicle growth, Proc. Natl. Acad. Sci. U. S. A., 2013, 110(49), 19679–19688, DOI:10.1073/pnas.1309970110.
- Y. Miao, Y. B. Sun and B. C. Liu,
et al., Controllable production of transplantable adult human high-passage dermal papilla spheroids using 3D matrigel culture, Tissue Eng., Part A, 2014, 20(17–18), 2329–2338, DOI:10.1089/ten.TEA.2013.0547.
- M. Zhang, C. Zhang and Z. Li,
et al., Advances in 3D skin bioprinting for wound healing and disease modeling, Regener. Biomater., 2022, 10, rbac105, DOI:10.1093/rb/rbac105.
- S. Huang, B. Yao and J. Xie,
et al., 3D bioprinted extracellular matrix mimics facilitate directed differentiation of epithelial progenitors for sweat gland regeneration, Acta Biomater., 2016, 32, 170–177, DOI:10.1016/j.actbio.2015.12.039.
- N. Liu, S. Huang and B. Yao,
et al., 3D bioprinting matrices with controlled pore structure and release function guide in vitro self-organization of sweat gland, Sci. Rep., 2016, 6, 34410, DOI:10.1038/srep34410.
- B. Yao, R. Wang and Y. Wang,
et al., Biochemical and structural cues of 3D-printed matrix synergistically direct MSC differentiation for functional sweat gland regeneration, Sci. Adv., 2020, 6(10), eaaz1094, DOI:10.1126/sciadv.aaz1094.
- C. C. Zouboulis, G. J. Yoshida and Y. Wu,
et al., Sebaceous gland: Milestones of 30-year modelling research dedicated to the “brain of the skin”, Exp. Dermatol., 2020, 29(11), 1069–1079, DOI:10.1111/exd.14184.
- L. Chen, D. Yan and N. Wu,
et al., 3D-Printed Poly-Caprolactone Scaffolds Modified With Biomimetic Extracellular Matrices for Tarsal Plate Tissue Engineering, Front. Bioeng. Biotechnol., 2020, 8, 219, DOI:10.3389/fbioe.2020.00219.
- N. Noor, A. Shapira and R. Edri,
et al., 3D Printing of Personalized Thick and Perfusable Cardiac Patches and Hearts, Adv. Sci., 2019, 6(11), 1900344, DOI:10.1002/advs.201900344.
- Q. Jallerat and A. W. Feinberg, Extracellular Matrix Structure and Composition in the Early Four-Chambered Embryonic Heart, Cells, 2020, 9(2), 285, DOI:10.3390/cells9020285.
- B. Fischer, F. Gwinner and M. M. Gepp,
et al., A highly versatile biopolymer-based platform for the maturation of human pluripotent stem cell-derived cardiomyocytes enables functional analysis in vitro and 3D printing of heart patches, J. Biomed. Mater. Res., Part A, 2023, 111(10), 1600–1615, DOI:10.1002/jbm.a.37558.
- G. Zhou, H. Jiang and Z. Yin,
et al., In Vitro Regeneration of Patient-specific Ear-shaped Cartilage and Its First Clinical Application for Auricular Reconstruction, EBioMedicine, 2018, 28, 287–302, DOI:10.1016/j.ebiom.2018.01.011.
- B. Grigoryan, S. J. Paulsen and D. C. Corbett,
et al., Multivascular networks and functional intravascular topologies within biocompatible hydrogels, Science, 2019, 364(6439), 458–464, DOI:10.1126/science.aav9750.
- K. P. A. Kumar and M. Pumera,
et al., 3D-Printing to Mitigate COVID-19 Pandemic, Adv. Funct. Mater., 2021, 31(22), 2100450, DOI:10.1002/adfm.202100450.
- S. Y. Kim, G. Han and D. B. Hwang,
et al., Design and Usability Evaluations of a 3D-Printed Implantable Drug Delivery Device for Acute Liver Failure in Preclinical Settings, Adv. Healthcare Mater., 2021, 10(14), e2100497, DOI:10.1002/adhm.202100497.
- J. Christoffersson, C. Aronsson and M. Jury,
et al., Fabrication of modular hyaluronan-PEG hydrogels to support 3D cultures of hepatocytes in a perfused liver-on-a-chip device, Biofabrication, 2018, 11(1), 015013, DOI:10.1088/1758-5090/aaf657.
- H. Lee, S. Chae and J. Y. Kim,
et al., Cell-printed 3D liver-on-a-chip possessing a liver microenvironment and biliary system, Biofabrication, 2019, 11(2), 025001, DOI:10.1088/1758-5090/aaf9fa.
- A. Faulkner-Jones, C. Fyfe and D. J. Cornelissen,
et al., Bioprinting of human pluripotent stem cells and their directed differentiation into hepatocyte-like cells for the generation of mini-livers in 3D, Biofabrication, 2015, 7(4), 044102, DOI:10.1088/1758-5090/7/4/044102.
- M. Lei and X. Wang,
et al., Biodegradable Polymers and Stem Cells for Bioprinting, Molecules, 2016, 21(5), 539, DOI:10.3390/molecules21050539.
- M. Ali, A. K. Pr and J. J. Yoo,
et al., A Photo-Crosslinkable Kidney ECM-Derived Bioink Accelerates Renal Tissue Formation, Adv. Healthcare Mater., 2019, 8(7), e1800992, DOI:10.1002/adhm.201800992.
- S. Chae, J. Kim and H. G. Yi,
et al., 3D Bioprinting of an In Vitro Model of a Biomimetic Urinary Bladder with a Contract-Release System, Micromachines, 2022, 13(2), 277, DOI:10.3390/mi13020277.
- K. Xu, Y. Han and Y. Huang,
et al., The application of 3D bioprinting in urological diseases, Mater. Today Bio, 2022, 16, 100388, DOI:10.1016/j.mtbio.2022.100388.
- K. A. Homan, D. B. Kolesky and M. A. Skylar-Scott,
et al., Bioprinting of 3D Convoluted Renal Proximal Tubules on Perfusable Chips, Sci. Rep., 2016, 6, 34845, DOI:10.1038/srep34845.
- N. Y. C. Lin, K. A. Homan and S. S. Robinson,
et al., Renal reabsorption in 3D vascularized proximal tubule models, Proc. Natl. Acad. Sci. U. S. A., 2019, 116(12), 5399–5404, DOI:10.1073/pnas.1815208116.
- G. Addario, S. Djudjaj and S. Fare,
et al., Microfluidic bioprinting towards a renal in vitro model, Bioprinting, 2020, 20, e00108, DOI:10.1016/j.bprint.2020.e00108.
- V. Carvalho, I. Gonçalves and T. Lage,
et al., 3D Printing Techniques and Their Applications to Organ-on-a-Chip Platforms: A Systematic Review, Sensors, 2021, 21(9), 3304, DOI:10.3390/s21093304.
- B. Zohar, Y. Blinder and M. Epshtein,
et al., Multi-flow channel bioreactor enables real-time monitoring of cellular dynamics in 3D engineered tissue, Commun. Biol., 2019, 2, 158, DOI:10.1038/s42003-019-0400-z.
- K. Yamamoto, N. Yamaoka and Y. Imaizumi,
et al., Development of a human neuromuscular tissue-on-a-chip model on a 24-well-plate-format compartmentalized microfluidic device, Lab Chip, 2021, 21(10), 1897–1907, 10.1039/d1lc00048a.
- J. U. Lind, T. A. Busbee and A. D. Valentine,
et al., Instrumented cardiac microphysiological devices via multimaterial three-dimensional printing, Nat. Mater., 2017, 16(3), 303–308, DOI:10.1038/nmat4782.
- J. R. Lake, Hepatocyte transplantation, N. Engl. J. Med., 1998, 338(20), 1463–1465, DOI:10.1056/NEJM199805143382012.
- L. Wang, C. Wang and Z. Wang,
et al., Transforming the spleen into a liver-like organ in vivo, Sci. Adv., 2020, 6(24), eaaz9974, DOI:10.1126/sciadv.aaz9974.
- P. Fontes, J. Komori and R. Lopez,
et al., Development of Ectopic Livers by Hepatocyte Transplantation Into Swine Lymph Nodes, Liver Transplant., 2020, 26(12), 1629–1643, DOI:10.1002/lt.25872.
- A. Messina, E. Luce and M. Hussein,
et al., Pluripotent-Stem-Cell-Derived Hepatic Cells: Hepatocytes and Organoids for Liver Therapy and Regeneration, Cells, 2020, 9(2), 420, DOI:10.3390/cells9020420.
- Z. Liu, M. Tang and J. Zhao,
et al., Looking into the Future: Toward Advanced 3D Biomaterials for Stem-Cell-Based Regenerative Medicine, Adv. Mater., 2018, 30(17), e1705388, DOI:10.1002/adma.201705388.
- S. Yamanaka, Induced pluripotent stem cells: past, present, and future, Cell Stem Cell, 2012, 10(6), 678–684, DOI:10.1016/j.stem.2012.05.005.
- M. A. Mazid, C. Ward and Z. Luo,
et al., Rolling back human pluripotent stem cells to an eight-cell embryo-like stage, Nature, 2022, 605(7909), 315–324, DOI:10.1038/s41586-022-04625-0.
- S. Feng, J. Wu and W. L. Qiu,
et al., Large-scale Generation of Functional and Transplantable Hepatocytes and Cholangiocytes from Human Endoderm Stem Cells, Cell Rep., 2020, 33(10), 108455, DOI:10.1016/j.celrep.2020.108455.
- Y. Hu, Y. Yang and P. Tan,
et al., Induction of mouse totipotent stem cells by a defined
chemical cocktail, Nature, 2023, 617(7962), 792–797, DOI:10.1038/s41586-022-04967-9.
- B. Zhao, Application prospects of organoids in organ transplantation, Organ Transplant., 2022, 13(2), 169–175 Search PubMed.
- J. Sun, K. Yao and J. An,
et al., Machine learning and 3D bioprinting, Int. J. Bioprint., 2023, 9(4), 717, DOI:10.18063/ijb.717.
- J. An, C. K. Chua and V. Mironov, Application of Machine Learning in 3D Bioprinting: Focus on Development of Big Data and Digital Twin, Int. J. Bioprint., 2021, 7(1), 342, DOI:10.18063/ijb.v7i1.342.
- J. Sun, G. Hong and M. Rahman,
et al., Improved performance evaluation of tool condition identification by manufacturing loss consideration, Int. J. Prod. Res., 2005, 43(6), 1185–1204, DOI:10.1080/00207540412331299701.
- J. Sun, K. Yao and K. Huang,
et al., Machine learning applications in scaffold based bioprinting, Mater. Today: Proc., 2022, 70, 17–23 Search PubMed.
|
This journal is © The Royal Society of Chemistry 2024 |
Click here to see how this site uses Cookies. View our privacy policy here.