DOI:
10.1039/D1NR04762K
(Paper)
Nanoscale, 2021,
13, 18451-18457
Incorporating highly basic polyoxometalate anions comprising Nb or Ta into nanoscale reaction fields of porous ionic crystals†
Received
22nd July 2021
, Accepted 1st October 2021
First published on 1st October 2021
Abstract
Polyoxometalates (POMs) are oxide cluster anions composed of high-valence early transition metals and are widely used as catalysts. Yet base catalysis of POMs remains an ongoing challenge; group V (V, Nb, and Ta) elements form more negatively charged POMs than group VI (Mo and W) elements, and in particular, polyoxoniobates and polyoxotantalates are known to show strong basicity in solution due to the highly negative surface oxygen atoms. Herein, we report for the first time porous ionic crystals (PICs) comprising Nb or Ta. The PICs are composed of Dawson-type Nb/W or Ta/W mixed-addenda POMs with oxo-centered trinuclear CrIII carboxylates and potassium ions as counter cations to control the crystal structure. Among the PICs, those with Nb or Ta tri-substituted POMs exhibit the highest yield (78–82%) and selectivity (99%) towards the Knoevenagel condensation of benzaldehyde and ethyl cyanoacetate (353 K, 6 h), which is a typical base-catalyzed reaction, as reusable solid catalysts, and they can also catalyze the reaction of other active methylene compounds. A detailed investigation into the crystal structures together with DFT calculations and in situ IR spectroscopy with methanol as a basic probe molecule shows that the exposure of [Nb3O13] or [Ta3O13] units with highly negative surface oxygen atoms to the pore surface of PICs is crucial to the catalytic performance. These findings based on the composition–structure–function relationships show that Nb- and Ta-containing PICs can serve as platforms for rational designing of heterogeneous base catalysts.
Introduction
Polyoxometalates (POMs) are robust, discrete, and structurally well-defined oxide cluster anions mainly composed of high-valence early transition metals.1–3 POMs are widely used as acid catalysts,4 oxidation catalysts,5 and photocatalysts,6 while base catalysis of POMs remains an ongoing challenge. It has been known that structurally and electronically well-defined surface basic oxygen atoms of POMs can abstract protons (Brønsted base) and/or act as a nucleophile (Lewis base).7 In this context, [WO4]2− shows high catalytic activity in CO2 fixation,8,9 and a Keggin-type lacunary POM ([γ-H2GeW10O36]6−) catalyzes the Knoevenagel reaction,10 which forms C–C bonds via the condensation of carbonyl compounds with active methylene compounds.11 Meanwhile, highly negatively charged POMs can act as strong base catalysts because the surface oxygen atoms generally become more basic by increasing the negative charges.12,13 Notably, group V elements (V, Nb, and Ta) form more negatively charged POMs than group VI elements (Mo and W) due to the difference in their highest oxidation states. In particular, polyoxoniobates and polyoxotantalates show potential applications in base-catalyzed reactions.14,15
Counter cations are imperative to isolate and crystallize POMs,16 and the use of metal ions has enabled breakthroughs in the formation of functional POM-based solids.17–21 We have been working on the synthesis of porous ionic crystals (PICs), which are crystalline porous composites of POMs with molecular cations (macrocations) such as the oxo-centered trinuclear metal carboxylates with a general formula of [M3O(OOCR)6(L)3]+.22 These macrocations have been studied as building blocks of solid materials because a wide choice of metal ions (MIII) and ligands (R,L) leads to versatile structures and functions.23–25 Among the trivalent metal ions (MIII), the octahedral CrIII complexes are chemically inert due to the large crystal field stabilization energy of the d3 electronic configuration,26 so that [Cr3O(OOCR)6(L)3]+ is stable and would be suitable as a component of PICs with catalytically active POMs. Most importantly, short-range anisotropic interactions such as hydrogen bonds and π–π interactions between the macrocations and catalytically active POMs form unique nano-scale reaction fields in the PICs. In this context, various Keggin-type ([XM12O40]n−)24,25,27,28 and Dawson-type ([X2M18O62]n−)29,30 POMs (X = P, Si, M = W, Mo, etc.) have been incorporated into PICs, while there are no reports on Nb- or Ta-containing POMs.
Based on these considerations, in this work, we report the syntheses, crystal structures, and base catalysis of PICs composed of mono-substituted or tri-substituted Dawson-type Nb/W and Ta/W mixed-addenda POMs with [Cr3O(OOCH)6(H2O)3]+ (Fig. 1). Compared with polyoxoniobates and polyoxotantalates, Nb/W and Ta/W mixed-addenda POMs are more stable in a broad pH range,31 which enables them to coexist with the macrocation in the synthetic aqueous solutions. We have utilized POMs with peroxo groups ([P2W17(NbO2)O61]7− and [P2W15(MO2)3O59]9−, M = Nb or Ta) as starting materials (Fig. 1) because it is well known that peroxo-free Nb/W and Ta/W mixed-addenda POMs tend to aggregate and form a dimer under acidic conditions.32–34 Therefore, we can expect that the peroxo groups could inhibit dimerization so that the catalytically active Nb and Ta sites can interact with reactant molecules.
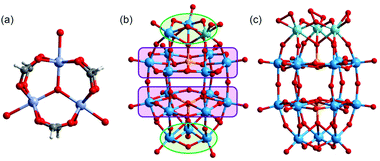 |
| Fig. 1 Molecular structures of (a) [Cr3O(OOCH)6(H2O)3]+, (b) [P2W17(NbO2)O61]7− and (c) [P2W15(MO2)3O59]9− (M = Nb or Ta). Color codes: W: blue; Nb or Ta: cyan; P: orange; O: red; Cr: silver; C: grey, and H: light grey. W atoms at the polar and equatorial sites of the Dawson-type POMs are surrounded by the green ovals and purple rectangles, respectively. W atoms at the polar sites are substituted with Nb or Ta atoms. | |
Results and discussion
Syntheses
PIC 1 (see below for the formula) was synthesized using the following procedure. [Cr3O(OOCH)6(H2O)3](OOCH)·nH2O35 was stirred in dilute aqueous nitric acid (pH = 2) at room temperature until it was fully dissolved. Then, K7[P2W17(NbO2)O61]·8H2O36 and KCl were added and dissolved in the solution. The solution was maintained at 277 K for 3 days and green crystals of 1 were obtained. PIC 2 (see below for the formula) was synthesized using a similar procedure using hydrochloric acid instead of nitric acid. The use of the Nb tri-substituted Dawson-type POMs K8H[P2W15(NbO2)3O59]·12H2O36 and K5Na4[P2W15(TaO2)3O59]·17H2O37 instead of K7[P2W17(NbO2)O61]·8H2O gives PIC 3_Nb and 3_Ta, respectively (see below for the formulae). PIC 3_Nb and 3_Ta crystallized from both hydrochloric acid and nitric acid solutions. IR spectra of the PICs showed characteristic peaks of the macrocation and POMs (Fig. S1–S3†). Elemental analysis and thermogravimetry (Fig. S4–S7†) showed that all PICs contained a macrocation and a POM in a 2
:
1 ratio and K+ ions compensate the surplus negative charge of the POM. The chemical formulae will be discussed in detail below.
Solid state 31P-MASNMR spectroscopy was utilized to characterize the states of Nb/W and Ta/W mixed-addenda POMs in the PICs (Fig. S8 and S9†). The NMR signals of 1 and 2 can be deconvoluted into two peaks with a 1
:
1 ratio. The peaks at the lower field can be assigned to the P in the half-anion containing Nb, and those at the higher field can be assigned to the other P.38 In contrast, the NMR signals of 3_Nb and 3_Ta can be deconvoluted into two pairs of peaks (Fig. S9a and S9c†), suggesting that the peroxo groups have partially converted to oxo groups in the synthesis (see ESI† for the details).
Crystal structures of the PICs
The crystallographic data of 1, 2 and 3_Nb are summarized in Table S1.† PIC 1 crystallized in the monoclinic space group P21/n. The substituted Nb atom exists at the polar sites of Dawson-type POMs, while the actual position could not be determined due to the Nb/W substitutional disorder. Single crystal X-ray diffraction (SXRD) analysis of 1 suggests that the peroxo group of the starting material has completely converted to an oxo group, and [P2W17NbO62]7− is formed, which agrees with the result of solid-state 31P-MASNMR (Fig. S8†). Therefore, 1 can be formulated as K5H[Cr3O(OOCH)6(H2O)3]2[P2W17NbO62] (NO3)·34H2O. As shown in Fig. 2a, POMs are arranged at equal intervals forming a triangular lattice in the ab-plane. Three K+ ions are bridged by triangular NO3− with K–O distances of 2.79–2.92 Å, and this complex is located on every other centroid of the triangular lattice. The K+ ions are interlinked by the POMs with K–O distances of 2.66–2.78 Å, resulting in the formation of a 2D-network structure in the ab-plane. Fig. 2b shows a column composed of a (A) POM, (B) macrocation, and (C) NO3− with an ABCBA packing sequence along the c-axis. A K+ ion bridges (B) and (A) so that the columns are connected to each other via K+ ions in the ab-plane forming a honeycomb-like lattice, and 1D-channels with a diameter of ca. 13 Å exist along the c-axis (Fig. 2c). The void analysis by CSD Mercury39 indicates that the void volume of I is 27.8% of the unit cell (Fig. 2d).
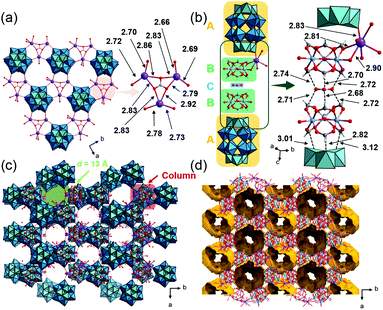 |
| Fig. 2 Crystal structure of K5H[Cr3O(OOCH)6(H2O)3]2[P2W17NbO62](NO3)·34H2O (1). Color codes: W: blue; W/Nb: cyan; P: orange; O: red; Cr: silver; K: violet; C: grey; N: light silver; H: light grey; [WO6]: blue octahedra; [W/NbO6]: cyan octahedra; and [PO4]: orange tetrahedra. (a) Arrangement of a POM, macrocation, and NO3− in the ab-plane, and a local view of three K+ ions bridged by a NO3− ion. (b) Arrangement of a POM, K+ ion, and NO3− along the c-axis. (c and d) Honeycomb-like lattice and 1D channels; void space (in yellow) is depicted with a probe radius of 1.2 Å. | |
PIC 2 is isostructural with the reported PIC composed of [P2W18O62]6− with a similar hexagonal arrangement K4[Cr3O(H2O)3(OOCH)6]2[P2W18O62]·9.5H2O (Cr-H-P2W18);29,40 the substitution of W with Nb at the polar sites of the POM leads to a decrease in symmetry, which causes the alteration of the space group from P63/m to P63/mmc. As in the case of 1, the actual position of Nb could not be determined due to the Nb/W substitutional disorder. The peroxo group of the starting material has completely converted to an oxo group, and [P2W17NbO62]7− is formed. Therefore, 2 can be formulated as K5[Cr3O(OOCH)6(H2O)3]2[P2W17NbO62]·18H2O. The crystal structure of 2 can be described by the assembly of two types of columns I and II. Column I is composed of the (A) POM and (B) macrocation with an ABAB packing sequence along the c-axis (Fig. 3a). Column II is composed only of the macrocation, which is positionally disordered with 0.5 occupancy (Fig. 3b). In the ab-plane, a POM is surrounded by three macrocations from column I and three macrocations from column II (Fig. 3c). K+ ions, which are positionally disordered with 1/3 occupancy, bridge the POM and the macrocation with K–O distances of 2.96 and 2.88 Å, respectively. As shown in Fig. 3d, the structure of 2 can be viewed as a honeycomb lattice composed of column I, and column II situates inside the honeycomb.
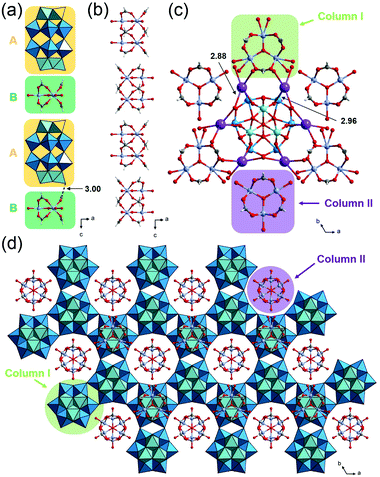 |
| Fig. 3 Crystal structure of K5[Cr3O(OOCH)6(H2O)3]2[P2W17NbO62]·18H2O (2). Color codes are the same as Fig. 2. (a) Arrangement of a POM and macrocation in column I. (b) Arrangement of a macrocation in column II. (c) Local view of a POM and the surrounding K+ ions and macrocations. (d) Honeycomb-like lattice. | |
PIC 3_Nb crystallized in the trigonal space group P
m1. Tri-substituted Nb atoms exist at either the top or the bottom polar site of the POM with a ratio of 0.28
:
0.72. In addition, SXRD analysis suggests that about half of the peroxo group of the starting material has converted to an oxo group, which agrees with the results of solid-state 31P-MASNMR (Fig. S9a†). Therefore, 3_Nb can be formulated as K6H[Cr3O(OOCH)6(H2O)3]2 [P2W15(NbO2)3O59]0.5[P2W15Nb3O62]0.5·17H2O. As shown in Fig. 4a, six POMs are interlinked by K+ ions with K–O distances of 2.94 Å and 2.95 Å, and a six-membered ring is formed. In the six-membered ring, two K+ ions coordinate to a POM, and these K+ ions are bridged by a water molecule with a K–O distance of 2.83 Å (Fig. 4a). Macrocations are located at the center of the 6-membered ring and are arrayed along the c-axis, and the distances between the μ3–O of adjacent macrocations are 6.13 and 6.49 Å (Fig. 4b and c). As a result, closed pores, which are surrounded by six macrocations and four POMs, are formed (Fig. 4d and e). The diameter of the closed pore is ca. 8 Å and the void analysis indicates that the volume is 8.3% of the unit cell.
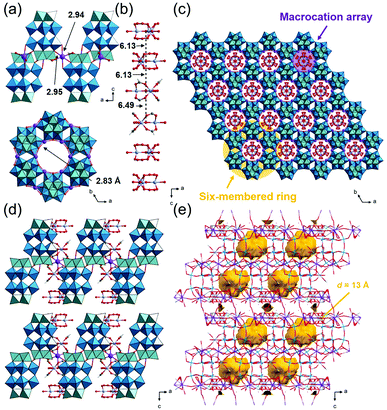 |
| Fig. 4 Crystal structure of K6H[Cr3O(OOCH)6(H2O)3]2[P2W15(NbO2)3O59]0.5 [P2W15Nb3O62]0.5·17H2O (3_Nb). Color codes are the same as Fig. 2. (a) Local views of the 6-membered ring; (b) side view of the macrocation array; arrangements of the ionic components (c) in the ab-plane and (d) along the c-axis; (e) void space (in yellow) is depicted with a probe radius of 1.2 Å. | |
The bulk purities of 1–3_Nb were confirmed by powder X-ray diffraction (PXRD) patterns (Fig. S10–S12†); the experimental PXRD patterns were in accordance with those calculated from the crystallographic data. The PXRD pattern of 3_Ta was analogous to that calculated from the CIF file of 3_Nb, showing that the two PICs are isostructural (Fig. S13†). In addition, the cell parameters of 3_Ta were estimated from the PXRD pattern by the Pawley method:41a = 16.113(1) Å and c = 25.269(5) Å. The slightly longer c-axis may be ascribed to the larger atomic radius of Ta than Nb.
Catalytic performances in the Knoevenagel condensation
The Knoevenagel condensation is a classic base-catalyzed reaction, which includes nucleophilic addition of an active hydrogen compound to a carbonyl group followed by a dehydration process. We carried out the Knoevenagel condensation of benzaldehyde with ethyl cyanoacetate to investigate the effect of Nb and Ta sites in the PICs on the base catalysis (Scheme 1). The reaction conditions (solvent, temperature, time, and mole ratio) were optimized (Table S2†), and the results are summarized in Table 1 (see Table S3† for full results). The product (ethyl α-cyanocinnamate) yield in the absence of a catalyst was 9% (entry 1). PICs 1 and 2 exhibited product yields of 18% and 29% after 6 h (entries 2 and 3, Fig. 5). However, the PXRD patterns of 1 and 2 indicated that amorphous phases and/or different structures are generated under reaction conditions, which is a drawback as a solid catalyst (Fig. S10 and S11†). As expected, 3_Nb showed a product yield of 40% after 1 h, which reached 78% after 6 h (entry 4, Fig. 5); the product yield is comparable to those of highly basic POMs as solid catalysts and higher than some typical metal oxides and zeolites (Table S4†). The product selectivity was maintained at 99% throughout the reaction with no obvious by-products (Fig. S14 (GC chart) and Fig. S15 (1H-NMR)†). As shown in Fig. 5, the reaction completely stopped upon the removal of 3_Nb, and no obvious leaching in the filtrate was detected by AAS (less than 1% based on Cr). These results show that the observed catalysis is truly heterogeneous. Moreover, 3_Nb is easily recovered from the reaction mixture by simple filtration and washing with ethanol and can be reused at least twice without a significant loss in the yield or selectivity (entries 5 and 6). The PXRD patterns and cell parameters of 3_Nb did not show obvious changes under the reaction conditions (Fig. S12c and S12d†), which confirms the durability as a solid catalyst. PIC 3_Ta exhibited a product yield of 29% after 1 h, which reached 82% after 6 h (entry 7, Fig. 5). The product selectivity was maintained at 99% (Fig. S14 (GC) and Fig. S15 (1H-NMR)†). PIC 3_Ta showed a catalytic activity similar to that of 3_Nb (Fig. 5), can be reused at least twice without significant changes in the structure (entries 8 and 9, Fig. S13c and S13d†), and showed no leaching. As a reference, the reaction was carried out with the non-substituted PIC (Cr-H-P2W18, entry 10), which showed a low product yield, indicating that the incorporation of Nb or Ta is crucial to the base catalysis. Note that the catalytic performances of physical mixtures of the macrocation and POM (entries 6–8 in Table S3†) were much inferior, suggesting the importance of the nanoscale reaction field. PIC 3_Nb and 3_Ta can also catalyze the Knoevenagel condensation of benzaldehyde and malononitrile (Table S5†), and both reached high yields (99% for 3_Nb and 96% for 3_Ta), which are comparable to those of highly basic POMs as catalysts.10,12,13,42–44
 |
| Scheme 1 Knoevenagel condensation of benzaldehyde and ethyl cyanoacetate. | |
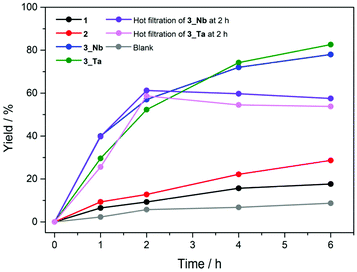 |
| Fig. 5 Time courses of the Knoevenagel condensation catalyzed by 1 (black), 2 (red), 3_Nb (blue), and 3_Ta (green), and the blank test (gray) and that after removal of 3_Nb (purple) or 3_Ta (pink). | |
Table 1 Catalytic results of various catalysts in the Knoevenagel condensation reactiona
Entry |
Catalyst |
Conv./% |
Yieldb/% |
Selec.c/% |
Reaction conditions: 0.01 mmol catalyst, 1.0 mmol benzaldehyde, 1.0 mmol ethyl cyanoacetate, 10 mg biphenyl (internal standard), and 1 mL ethanol.
Yield of ethyl α-cyanocinnamate.
Selectivity to ethyl α-cyanocinnamate.
Since the particle size of solid catalysts may affect the catalysis, the solids were ground and passed through a 150-mesh sieve.
Catalyst dissolved in the reaction solution.
|
1 |
None |
35 |
9 |
25 |
2 |
1
|
46 |
18 |
39 |
3 |
2
|
51 |
29 |
57 |
4 |
3_Nb
|
79 |
78 |
99 |
5 |
3_Nb (2nd run) |
79 |
78 |
99 |
6 |
3_Nb (3rd run) |
75 |
70 |
93 |
7 |
3_Ta
|
83 |
82 |
99 |
8 |
3_Ta (2nd run) |
83 |
82 |
99 |
9 |
3_Ta (3rd run) |
81 |
77 |
95 |
10 |
Cr-H-P2W18
|
45 |
21 |
47 |
Among the PICs, the product yields decreased in the order of 3_Ta (82%) ≈ 3_Nb (78%) > 2 (29%) > 1 (18%). It is generally understood that the void space or porosity of solid catalysts, where the reaction takes place, is one of the important keys in heterogeneous reactions. However, the porosity decreases in the order of 1 (28.9%) > 2 (ca. 11%)‡ ≈ 3_Nb, 3_Ta (8.7%), which shows no relationship with the catalysis. The water adsorption isotherms of 1–3 at 298 K (Fig. S16†) show that the amounts of water adsorbed at 2.85 kPa or P/P0 = 0.9 decrease in the order of 1 (33 mol mol−1, i.e., the amount of water in mol per mol of PIC) > 2 (13) ≈ 3_Nb (12) ≈ 3_Ta (10), which is in line with the porosity judged from the crystal structures. The porosity is further characterized by CO2 adsorption isotherms at 268 K (Fig. S17†), which show the same order of 1 (1.3) > 2 (0.6) ≈ 3_Nb (0.7) ≈ 3_Ta (0.6) at 90 kPa.§ These results suggest that the local structure of PICs rather than porosity may be affecting the catalytic activity. In fact, in the crystal structure of 3_Nb, the terminal oxygen atoms of the Nb sites are exposed to the voids (Fig. 6), which allows the reactants to directly contact the active sites. On the other hand, in the crystal structures of 1 and 2, the polar sites of the POM are in close contact with the macrocation, so that the active sites are probably blocked (Fig. 6). This kind of steric effect has been also observed in homogeneous Knoevenagel reactions.45
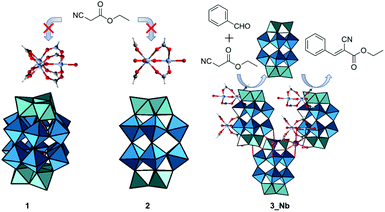 |
| Fig. 6 Schematic illustration of the possible steric effects in 1, 2, and 3_Nb. | |
Basicity of the oxygen atoms of [NbO6] and [TaO6] sites
Previous studies indicate that the basic oxygen (O) atoms of a POM serve as active-sites for the Knoevenagel reaction and that the basicity of the O atom strongly correlates with the negative charge.12,13 In order to study the basicity of the Nb- and Ta-substituted POMs, theoretical calculations were performed by the density functional theory (DFT) method (Tables S6–S9†). Fig. 7 shows the optimized molecular structures and natural bond orbital (NBO) charges of the O atoms in [P2W17NbO62]7−, [P2W15Nb3O62]9−, and [P2W15Ta3O62]9−. The terminal (Ot(W)) and bridging (W–Ob–W) O atoms of [WO6] in [P2W17NbO62]7− have NBO charges ranging from −0.553 to −0.568 and −0.700 to −0.719, respectively. The terminal O atom of [NbO6] in [P2W17NbO62]7− (Ot(Nb)) has an NBO charge of −0.658, and those of the bridging O atoms between [WO6] and [NbO6] (Ob(W–Ob–Nb)) range from −0.715 to −0.730. For [P2W15Nb3O62]9−, the O atoms of [NbO6] show higher negative NBO charges (–0.704 for Ot(Nb) and −0.785 for Ob(Nb–Ob–Nb)) than those of [WO6] (the highest negative values are −0.616 and −0.738 for Ot(W) and Ob(W–Ob–W), respectively). The negative NBO charges of O atoms of [TaO6] in [P2W15Ta3O62]9− (−0.809 for Ot(Ta) and −0.884 for Ob(Ta–Ob–Ta)) are higher than those of [NbO6] in [P2W15Nb3O62]9−. In addition, DFT calculations were carried out for the non-substituted [P2W18O62]6−. As shown in Fig. S18,† the NBO charges of the terminal O atoms of [WO6] in [P2W18O62]6− are −0.546 and −0.538, for the polar and equatorial positions, respectively. The negative charges of the O atoms of Nb/Ta-substituted POMs (−0.658 to −0.809 for the polar positions and −0.553 to −0.591 for the equatorial positions) were much higher than those of the non-substituted POM. The results on NBO charges suggest that the basicity would be in the order of [P2W18O62]6− < [P2W17NbO62]7− < [P2W15Nb3O62]9− < [P2W15Ta3O62]9− for the POMs and 1, 2 < 3_Nb < 3_Ta for the PICs. However, the catalytic activities were in the order of 1 < 2 ≪ 3_Nb ≈ 3_Ta (Table 1). These results clearly indicate that not only basicity but also the steric effect, as illustrated in Fig. 6, affects the base catalysis.
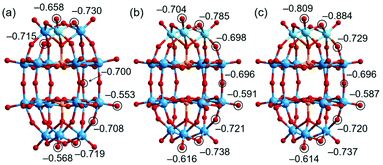 |
| Fig. 7 Calculated NBO charges of oxygen atoms in (a) [P2W17NbO62]7− in 2, (b) [P2W15Nb3O62]9− in 3_Nb, and (c) [P2W15Ta3O62]9− in 3_Ta. | |
The accessibility of the basic sites in the PICs was characterized by in situ IR spectroscopy with methanol, which is one of the smallest and simplest basic probe molecules. Fig. S19† shows the difference spectra of methanol adsorbed on the PICs at 298 K. PICs 1–3 exhibit broad bands between 3400 and 3600 cm−1, which can be assigned to the ν(OH) of methanol.46 In addition, the spectra show bands at 2945 cm−1 and 2838 cm−1, which can be assigned to the asymmetric and symmetric CH3 stretching vibration modes, respectively.42 The positions of the bands suggest that methanol is molecularly adsorbed on the PICs. In contrast, Cr-H-P2W18 shows no signals due to methanol. Therefore, methanol probably adsorbs on the [NbO6] and [TaO6] sites, and not on the [WO6] sites, highlighting the importance of accessible basic [NbO6] or [TaO6] sites to attain high catalytic activity in the Knoevenagel reaction catalyzed by PICs. Furthermore, the methanol desorption behaviors of 3_Nb and 3_Ta were almost identical (Fig. S20†), indicating that the interactions between methanol and 3_Nb or 3_Ta are alike, which is in line with the similar catalytic activities.
Conclusions
For the first time, Nb- and Ta-containing porous ionic crystals (PICs) composed of Dawson-type Nb/W mixed-addenda POMs with oxo-centered trinuclear CrIII carboxylates and potassium ions as counter cations were reported. The PICs with Nb or Ta tri-substituted POMs exhibited high yields and selectivities in the Knoevenagel condensation as reusable solid base catalysts. The high catalytic activities can be ascribed to a synergetic effect of high basicity of [Nb3O13] or [Ta3O13] and the exposure of these sites to the pore surface. These findings based on the composition–structure–function relationships show that Nb- and Ta-containing PICs can serve as platforms for rational designing of heterogeneous base catalysts. Since these PICs can adsorb CO2 under ambient conditions, our next aim is to look into CO2 fixation reactions.
Author contributions
Z.W. and N.O. performed the syntheses and characterization of the compounds; Z.W. carried out the SXRD measurements and analysis; T.K. carried out the XRF measurements; Y.K. carried out the in situ IR measurements; Y.G. and L.Y. carried out the DFT calculations; Z.W., N.O., and S.U. wrote the manuscript. S.U. conceived and designed the project. All authors discussed the results and commented on the manuscript.
Conflicts of interest
There are no conflicts to declare.
Acknowledgements
This work was supported by the JSPS Grants-in-Aid for Scientific Research from MEXT of Japan (JP20H02750 and JP21K14639), the International Network on Polyoxometalate Science at Hiroshima University, and the JSPS Core-to-Core program. Prof. T. Ito (Tokai University) is acknowledged for obtaining the SXRD data of 3_Nb. Prof. T. Uemura (Univ. of Tokyo) is acknowledged for providing access to the X-ray fluorescence instrument. Prof. M. Matsuo and Dr K. Shozugawa (Univ. of Tokyo) are acknowledged for providing access to the ICP-OES instrument.
Notes and references
- M. T. Pope and A. Müller, Angew. Chem., Int. Ed. Engl., 1991, 30, 34–48 CrossRef
.
- C. L. Hill, Chem. Rev., 1998, 98, 1–2 CrossRef CAS PubMed
.
- L. Vilà-Nadal and L. Cronin, Nat. Rev. Mater., 2017, 2, 17054 CrossRef
.
- S. Wang and G. Yang, Chem. Rev., 2015, 115, 4893–4962 CrossRef CAS PubMed
.
- Q. Y. Chen, C. R. Shen and L. He, Acta Crystallogr., Sect. C: Struct. Chem., 2018, 74, 1182–1201 CrossRef CAS PubMed
.
- J. J. Walsh, A. M. Bond, R. J. Forster and T. E. Keyes, Coord. Chem. Rev., 2016, 306, 217–234 CrossRef CAS
.
- K. Kamata and K. Sugahara, Catalysts, 2017, 7, 1–24 CrossRef
.
- T. Kimura, K. Kamata and N. Mizuno, Angew. Chem., Int. Ed., 2012, 51, 6700–6703 CrossRef CAS PubMed
.
- T. Kimura, H. Sunaba, K. Kamata and N. Mizuno, Inorg. Chem., 2012, 51, 13001–13008 CrossRef CAS PubMed
.
- K. Sugahara, T. Kimura, K. Kamata, K. Yamaguchi and N. Mizuno, Chem. Commun., 2012, 48, 8422–8424 RSC
.
-
Y. Ono and H. Hattori, Solid base catalysis, Springer-Verlag Berlin Heidelberg, 2011 Search PubMed
.
- Q. Xu, Y. Niu, G. Wang, Y. Li, Y. Zhao, V. Singh, J. Niu and J. Wang, Mol. Catal., 2018, 453, 93–99 CrossRef CAS
.
- W. Ge, X. Wang, L. Zhang, L. Du, Y. Zhou and J. Wang, Catal. Sci. Technol., 2016, 6, 460–467 RSC
.
- M. Nyman, Dalton Trans., 2011, 40, 8049–8058 RSC
.
- H. L. Wu, Z. M. Zhang, Y. G. Li, X. L. Wang and E. B. Wang, CrystEngComm, 2015, 17, 6261–6268 RSC
.
- A. Misra, K. Kozma, C. Streb and M. Nyman, Angew. Chem., Int. Ed., 2020, 59, 596–612 CrossRef CAS PubMed
.
- O. Sadeghi, L. N. Zakharov and M. Nyman, Science, 2015, 347, 1359–1362 CrossRef CAS PubMed
.
- Y. Ren, M. Wang, X. Chen, B. Yue and H. He, Materials, 2015, 8, 1545–1567 CrossRef CAS PubMed
.
- S. Uchida, Chem. Sci., 2019, 10, 7670–7679 RSC
.
- S. Uchida, T. Okunaga, Y. Harada, S. Magira, Y. Noda, T. Mizuno and T. Tachikawa, Nanoscale, 2019, 11, 5460–5466 RSC
.
- N. Ogiwara, M. Tomoda, S. Miyazaki, Z. Weng, H. Takatsu, H. Kageyama, T. Misawa, T. Ito and S. Uchida, Nanoscale, 2021, 13, 8049–8057 RSC
.
- Y. Shimoyama and S. Uchida, Chem. Lett., 2021, 50, 21–30 CrossRef CAS
.
- A. Vimont, J.-M. Goupil, J.-C. Lavalley, M. Daturi, S. Surblé, C. Serre, F. Millange, G. Férey and N. Audebrand, J. Am. Chem. Soc., 2006, 128(10), 3218–3227 CrossRef CAS PubMed
.
- R. Kawahara, K. Niinomi, J. N. Kondo, M. Hibino, N. Mizuno and S. Uchida, Dalton Trans., 2016, 45, 2805–2809 RSC
.
- T. Yamada, K. Kamata, E. Hayashi, M. Hara and S. Uchida, ChemCatChem, 2019, 11, 3743–3747 Search PubMed
.
-
M. Weller, T. Overton, J. Rourke and F. Armstrong, Shriver-Atkins Inorganic Chemistry, Oxford University Press, Oxford, 6th edn, 2014 Search PubMed
.
- S. Uchida, M. Hashimoto and N. Mizuno, Angew. Chem., Int. Ed., 2002, 41, 2814–2817 CrossRef CAS PubMed
.
- S. Uchida, R. Kawamoto and N. Mizuno, Inorg. Chem., 2006, 45, 5136–5144 CrossRef CAS PubMed
.
- S. Uchida, R. Kawamoto, T. Akatsuka, S. Hikichi and N. Mizuno, Chem. Mater., 2005, 17, 1367–1375 CrossRef CAS
.
- S. Hitose and S. Uchida, Inorg. Chem., 2018, 57, 4833–4836 CrossRef CAS PubMed
.
- D. Zhang, Z. Liang, S. Xie, P. Ma, C. Zhang, J. Wang and J. Niu, Inorg. Chem., 2014, 53, 9917–9922 CrossRef CAS PubMed
.
- G.-S. Kim, H. Zeng, W. A. Neiwert, J. J. Cowan, D. VanDerveer, C. L. Hill and I. A. Weinstock, Inorg. Chem., 2003, 42, 5537–5544 CrossRef CAS PubMed
.
- R. G. Finke and M. W. Droege, J. Am. Chem. Soc., 1984, 106, 7274–7277 CrossRef CAS
.
- K. Nomiya, M. Kaneko, N. C. Kasuga, R. G. Finke and M. Pohl, Inorg. Chem., 1994, 33, 1469–1472 CrossRef CAS
.
- M. K. Johnson, D. B. Powell and R. D. Cannon, Spectrochim. Acta, 1981, 37, 995–1006 CrossRef
.
- J. Gong, Y. Chen, L. Y. Qu and L. Qin, Polyhedron, 1996, 15, 2273–2277 CrossRef CAS
.
- S. Li, S. Liu, S. Liu, Y. Liu, Q. Tang, Z. Shi, S. Ouyang and J. Ye, J. Am. Chem. Soc., 2012, 134, 19716–19721 CrossRef CAS PubMed
.
- D. A. Judd, J. H. Nettles, N. Nevins, J. P. Snyder, D. C. Liotta, J. Tang, J. Ermolieff, R. F. Schinazi and C. L. Hill, J. Am. Chem. Soc., 2001, 123, 886–897 CrossRef CAS PubMed
.
- C. F. Macrae, I. Sovago, S. J. Cottrell, P. T. A. Galek, P. McCabe, E. Pidcock, M. Platings, G. P. Shields, J. S. Stevens, M. Towler and P. A. Wood, J. Appl. Crystallogr., 2020, 53, 226–235 CrossRef CAS PubMed
.
- X. Zhang, W. You, Z. Zhu, L. Dang, Z. Sun and X. Zheng, Chem. Res. Chin. Univ., 2007, 23, 8–13 CrossRef CAS
.
- G. S. Pawley, J. Appl. Crystallogr., 1981, 14, 357–361 CrossRef CAS
.
- A. Yoshida, S. Hikichi and N. Mizuno, J. Organomet. Chem., 2007, 692, 455–459 CrossRef CAS
.
- A. Davoodnia, Synth. React. Inorg. Met.-Org. Chem., 2012, 42, 1022–1026 CrossRef CAS
.
- S. Zhao, Y. Chen and Y.-F. Song, Appl. Catal., A, 2014, 475, 140–146 CrossRef CAS
.
- S. Hayashi, S. Yamazoe and T. Tsukuda, J. Phys. Chem. C, 2020, 124, 10975–10980 CrossRef CAS
.
- S. Kanai, I. Nagahara, Y. Kita, K. Kamata and M. Hara, Chem. Sci., 2017, 8, 3146–3153 RSC
.
Footnotes |
† Electronic supplementary information (ESI) available: Experimental section, IR, NMR, TGA, PXRD, NBO charges, catalytic results. CCDC 2057126 for 1, 2057132 for 2 and 2057141 for 3_Nb. For ESI and crystallographic data in CIF or other electronic format see DOI: 10.1039/d1nr04762k |
‡ The void volume of 2 cannot be evaluated with accuracy because of the positional disorder of the macrocation. The void volume of 2 is calculated as 0.7% with the disordered macrocation and 21.1% without the disordered macrocation. Therefore, ca. 11% is an average of the two values. |
§ There is a large discrepancy between the amounts of water and CO2 adsorption because of the difference in the saturated vapor pressure of the gaseous adsorbates at measurement temperatures (i.e., P0 = 3.167 kPa for water at 298 K and P0 = 3.03 × 103 kPa for CO2 at 268 K). |
|
This journal is © The Royal Society of Chemistry 2021 |
Click here to see how this site uses Cookies. View our privacy policy here.