Antiviral-nanoparticle interactions and reactions
Received
25th September 2020
, Accepted 3rd December 2020
First published on 7th December 2020
Abstract
From the current pandemic has blossomed a considerable range of virus and antiviral research including, but not limited to, surface disinfection, fluid filtration, vaccines, and therapeutics. Over the last decade, nanoparticle-microbe and antimicrobial nanomaterial research has flourished with a vast number of publications. In comparison, nanoparticle-virus and antiviral nanomaterial research has been relatively quiet and understudied, in particular considering the present need. Here, we present a brief review on the virus-nanoparticle literature focusing on antiviral potential of nanoparticle-virus interactions and nanoparticle-driven viral reactions. We then propose nanomaterial design parameters to optimize for viral interactions to reduce infectivity and to ultimately inactivate the virus. Future directions for antiviral nanomaterial research will be discussed.
Environmental significance
The emergence of novel pathogenic viruses is a grand challenge of our time that is generally unheeded due to the low pandemic frequency. During a pandemic event such as the present, viral research rapidly permeates into all areas of science and engineering and broad collaborative efforts are made to gain a better understanding of the challenge and to evaluate all potential solutions. A virus can be considered an evolving nanobiomachine, thus the environmental nanoscience community has an opportunity to boldly contribute to progress in areas such as virus fate, transport, and detection and antiviral nanotechnology. This paper through the review of antiviral nanomaterials attempts to support and invigorate this research progress.
|
Introduction
As humanity ventures deeper into a coexistence with COVID-19, the scientific community's interest in this novel coronavirus has concomitantly grown at an extremely rapid pace (Web of Science indicates >50
000 ‘covid-19’ papers from Jan to Dec 2020). Transmission prevention via use of personal protective equipment has emerged as a critical aspect to slow spread of the airborne virus during vaccine development.1–4 Filtration and disinfection technologies are key to transmission prevention and expertise in both is found in the environmental sciences.
Over the past decade, environmental nanotechnology has emerged as a broad area of research interest in regard to environmental monitoring and control.5,6 The unique properties of nanomaterials may offer novel solutions to classical environmental problems such as the control of pathogen transmission. For example, a material surface is inherently more reactive than its bulk due to a higher energy state and, hence, interacts more directly/intimately with its environment. Thus, nanoscale materials with fractional (>5%) surface atoms to total atoms will have both a higher reactivity and interaction area with their environment. Nanomaterials also have distinct electronic and mechanical properties due to quantum-size effects and/or lower dimensional (0D, 1D, 2D) morphologies. The nanoenabled hybridization of exceptional reactivity and functionality has stimulated research in the field of environmental nanotechnology.
Environmental control of biofilms and pathogen transmission is critical to human health as recognized by drinking and wastewater treatment being a major component of urban infrastructure.7–9 The focus has been predominantly placed on bacterial (and protozoan) pathogens for a variety of reasons such as ease of cultivation, detection, and characterization. A similar trend is observed for environmental nanotechnology research where focus has been placed on nanomaterial effects on microbial transport and metabolic processes, biofilm formation and growth, bacterial inactivation, and microbial processing of nanomaterials. For example, of the nearly three hundred 2019 articles in Environmental Science: Nano, only few were directly related to virus.10,11 Paradoxical since viruses are nature's nanobiomaterials.
In this review, we will give a brief overview of the current state of antiviral nanomaterial research with a focus on properties beneficial for virus-nanoparticle interactions and localized reactions. The antiviral properties of well-studied environmental nanoparticles such as nanosilver, nanoTiO2, and carbon nanotubes will be used to exemplify antiviral mechanisms such as cell binding inhibition and capsid degradation. The reviewed antiviral nanoparticle literature will cover a range of environments including air, water, and in vitro. Antiviral nanoparticle design principles will be discussed to promote future research in this promising field.
Antiviral nanoparticle design
A virus is a biological entity that is capable of assisted proliferation within active cells and transmission between cells and organisms.12 A virus consists of a protein shell or capsid that contains genetic material, i.e. DNA or RNA. The protein capsid is a multipurpose nanomachine that simultaneously provides stability to protect the viral genome against the external environment as well as a dynamic nanostructure that will release the genome after cellular infection.13 The capsid is composed of tens to hundreds of protein oligomer copies assembled into a symmetric metastable structure and is typically icosahedral (spherical) or helical (tubular) in shape with a diameter in the range of 20–200 nm.14 The capsid metastability arises from the hydrogen bonds, electrostatic interactions, and weak covalent bonds, e.g. disulfides, that bind the oligomers together. The virus may be enveloped or non-enveloped by a lipid bilayer. Biologically, the virus first specifically binds to a receptor that is typically embedded in the cell membrane such as a glycoprotein and enables the active transfer of the viral components into the cell.15 Physicochemically, the virus surface tends to be negatively-charged and interacts predominantly through electrostatic and hydrophobic forces.16 This basic physical virus description will guide development of fundamental engineered nanomaterial (ENM) design principles for viral removal and inactivation.
The protein capsid that protects the genetic material and enables specific interaction with and transport through a cell membrane should be the initial primary target of any virus inactivation strategy. A capsid structure and/or function disruption strategy should take advantage of its metastable nature to degrade the bonds holding the oligomer units together or to disable the function of surface receptor proteins.17 For example, acid–base or redox chemistry could be used to transform the hydrogen-bonding or oxidatively-labile thiols18 and subsequently weaken or break inter-oligomer bonds. Breakdown of the viral capsid may also release the contained genetic material leaving it unprotected from the environment. Thus, the ultimate viral inactivation strategy would include degradation of the capsid structure, function, and DNA/RNA to eliminate any chance of replication.19
Environmental engineers and scientists have a vast toolbox of methods that can alter the proton or redox potential among many other conditions of the fluid surrounding a virus.20,21 However, viral concentrations are typically quite low as only a single virus may be necessary for infection. In particular, relative to common environmental matrix components, virus concentrations would be considered extremely low, thus homogeneous generation of highly reactive species may be futile as they will be scavenged prior to a viral encounter. Techniques to generate localized reactive species or processes near the virus are needed, which is where nanomaterials may find utility. Antiviral nanoparticle design will require consideration of nanoparticle-virus interactions to ensure direct contact and subsequent localized reactions for viral inactivation.
Nanomaterials benefit from having a dimension that is similar to or lesser than the target virus allowing for intimate interaction. However, for a nanoparticle to retain this advantageous characteristic, it must be designed not only to attract virus through electrostatic or vdW interactions,16 but also prevent self-aggregation. Thus, a highly positively-charged nanomaterial that will electrostatically attract virus while concomitantly repelling itself would find utility. One design would be to utilize a nanoparticle with a high isoelectric point such that it would be positively-charged at neutral pH22,23 although inorganic particles tend to have complications in the presence of inorganic ions.23,24 A more versatile design would be to apply a positively-charged surface coating such as an organic amine to any base nanomaterial to yield viral attraction properties.25–27 If a more targeted interaction is desired, then the nanomaterial could be coated with a cellular receptor mimic such as an oligosaccharide recognized by the virus.28,29 In summary, nanomaterial surface chemistry design and manipulation via surface coatings offers a rational method to enhance nanoparticle-virus interactions.
Common viral disinfection strategies include UVC, dehydration, heat, and chemical oxidation that operate by a range of protein and genome degradation mechanisms.17–19,21 The most widely-studied nanoparticle disinfection method is UV-vis photocatalytic generation of reactive oxygen species including O2˙−, 1O2, and ˙OH,30 and when combined with a NP-virus interaction strategy can be effective and efficient.27 Near-infrared to radio frequency stimulation of single-walled carbon nanotubes has been reported to result in localized heating and thermal cytotoxicity of targeted cells.31,32 If the nanoparticles are formed into an electrical percolation network, then electric potential stimulation becomes viable. For example, virus adhered to a carbon nanotube network could be inactivated by application of an electrical potential to stimulate direct hole oxidation,33 free chlorine production, or localized resistive heating.34 In summary, external nanoparticle stimulation can yield localized regions of high reactivity toward viral inactivation that becomes significantly more effective when hybridized with a nanoparticle-virus interaction strategy.
Antiviral 3D nanoparticles
Silver has a long history of antimicrobial applications and nanosilver fits the general description desired for viral interaction and disruption as it is positively-charged, interacts specifically with sulfides, and can initiate surface redox chemistry. Accordingly, nanosilver studies have indicated it is a broad spectrum antiviral35 observed to inhibit in vitro replication of a number of viruses including HIV,36,37 hepatitis,38 monkeypox,39 and norovirus40 while exerting minimal toxicity on the cellular matrix. Smaller diameter (<20 nm) Ag has been observed to inhibit virus to a greater extent than both larger particles and dissolved Ag+.36,40 Nanosilver has been observed to bind individual virus and viral DNA38 likely via electrostatic interactions and is hypothesized to interact specifically with oligomer disulfides due to observed viral surface deposition patterns.36 Viral replication inhibition is due to a combination of the adhered nanoparticle facilitating capsid degradation,40–42 and more importantly blocking of cell attachment, cell entry, and protein/genome injection.43–45 Interference with cellular recognition can be enhanced by nanoparticle coatings that stabilize dispersions increasing viral availability and specifically interact with viral envelope glycoprotein cell receptor binding domains.28,43,45 Similar antiviral mechanisms have been observed for non-silver nanoparticles such zero-valent iron and iron oxide,41,46 copper oxide,44 metal alloys,47 and glycosylated C60.28
The nanosilver–virus interactions and reactions can be utilized as practical guidelines for antiviral nanoparticle design as depicted in Scheme 1. The nanoparticle should be of similar or lesser size than the virus to maximize the physicochemical interactions between NP and viruses, and the NP surface should be kinetically accessible (e.g. well-dispersed). The nanoparticle surface or surface coating should have strong physicochemical interactions with the viral protein capsid such as general attractive (e.g. electrostatic or hydrophobic) or specific chemical (e.g. glycoprotein or disulfide). After the nanoparticle is adhered to the viral envelope, there are two primary antiviral mechanisms. The first is that the adhered nanoparticle sterically hinders and physically blocks the virus from recognizing cellular receptors and entering the cell, thus preventing infection and inhibiting replication. This may provide a therapeutic solution that would require application or injection of the antiviral nanoparticle to deter replication and propagation. The second is that the nanoparticle can produce localized reactive species post-adhesion resulting in degradation of the viral capsid receptors, stability, and genetic material. This may provide a permanent viral inactivation solution that would require the proper environment or external stimulation.
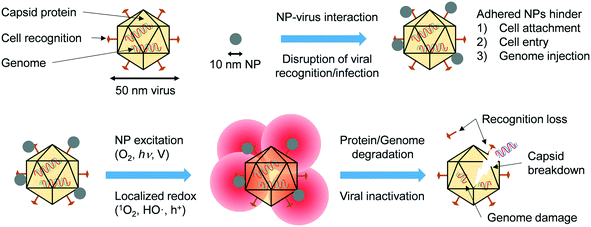 |
| Scheme 1 Antiviral mechanisms of a 3D nanoparticle. A generalized schematic of antiviral-nanoparticle interactions and reactions. The initial state is a well-dispersed virus and smaller dimension nanoparticle. In the first step, the NP and virus aggregate due to attractive electrostatic, vdW, or specific chemical interactions. The adhered NP can reduce virulence by inhibiting recognition and/or replication. In the second step, the adhered nanoparticle can be externally stimulated by light (hv) or an electric potential (V) to produce localized reactive species (1O2, HO·, h+) or heat represented by the red regions around the NP. In the final step, the reactive species may then degrade the capsid proteins and/or encapsulated genome resulting in viral inactivation. | |
The latter method is applicable to environmental pathogen control in air and water to prevent human exposure and subsequent infection. In the presence of oxygen, silver, iron, and copper nanoparticles will slowly oxidize to form reactive metal ions and reactive oxygen species that can lead to adhered viral degradation.40,41,47 However, use of reactive processes that involve nanoparticle oxidative dissolution such as antiviral nAg during ultrafiltration tend to be limited due to rapid nanomaterial loss.42 Processes driven by external stimulation may provide a kinetically-faster and longer-lived reactant source. Photocatalytic processes have been widely-studied due to the potential of utilizing the abundant and freely available solar spectrum. TiO2 photocatalysis has been the most widely studied because of its favorable band gap, band energy levels, and stability.48 However, nTiO2 may suffer in regard to viral inactivation since the particles typically have a slight negative surface charge at neutral pH that would electrostatically repel the similarly charged virus.49,50 Thus, nTiO2 is the converse of nAg in terms of viral adhesion and reactivity i.e. lesser interaction and stronger reaction, and previous research on photocatalytic viral disinfection will exemplify the importance of strong nanoparticle-viral interactions on subsequent inactivation.
Semiconductor photocatalysis (e.g. TiO2) occurs when a photon of energy similar to or greater than the band gap excites an electron to the conduction band of the particle leaving a valence band hole. The separated electron–hole pair can then drive subsequent surface or homogeneous reduction and oxidation chemistry, respectively. In regard to viral inactivation, surficial holes or free hydroxyl radicals are the predominant oxidant,30,51 reinforcing the advantage of direct viral-photocatalyst contact52 to enable near-surface HO˙ utilization prior to matrix scavenging.53 A number of methods have been employed to overcome the disadvantage of non-attractive virus-TiO2 interactions such as addition of ferrous ion to promote Fenton chemistry,49 application of electric potential or surface coating to increase TiO2 positive surface charge,54,55 and optimization of reactor configuration to improve mixing.50 In many cases, although the base component is nTiO2 particles, they are ultimately used as a larger aggregate such as the standard Degussa P25.30 Fullerene photosensitizers that produce singlet oxygen and are well-dispersed may be more representative of the antiviral nanoprocess described in Scheme 1. A variety of aqueous fullerene derivatives have been reported to rapidly photosensitize viral inactivation with evidence of viral capsid protein oxidation.56 Both the fullerene photoactivity and viral interaction were surface functionalization dependent, and the most interactive (closest viral association) C60(NH2)6 and photoactive (highest local 1O2) was observed to rupture the capsid structure. The viral photoinactivation was significantly improved by C60 derivatization with quaternary amines of permanent positive charge to optimize viral C60 interactions resulting in multi-log inactivation at nanomolar fullerene concentrations over a matter of minutes.27
The quaternary aminated fullerene viral photoinactivation process is similar to that depicted in Scheme 1 for a batch process where the nanoparticle first strongly adheres to the virus then is externally stimulated to drive localized viral inactivation. As expected, the reported process is rapid and effective for viral inactivation and capsid degradation. The batch configuration would be viable for disinfection of discrete fluid volumes for personal use, but may not be amenable to high volumes and continuous flows required for community level water systems or even personal level air systems. Therefore, filtration techniques that incorporate nanomaterials of similar surface and reactive properties into their hierarchal design may be a solution.
Antiviral 1D nanofibers
Viral removal from a flowing fluid will be dependent on its physicochemical properties such as size, shape, surface charge, and surface functionalization. Membrane sieving has potential for viral rejection; however, the pore sizes (<20 nm) and tight size distributions (no macro-defects) necessary to achieve >4
log removal and the corresponding applied pressures may restrict use to niche applications.57 Nanomaterials may offer a solution as their nanosize will allow them to be embedded into polymeric membranes with minimal performance loss due to pore blocking. Viral–surface interactions are predominantly mediated by electrostatic and hydrophobic forces,16 and the external virus surface is typically negatively-charged under environmental conditions. Thus, addition of an appropriately charged nanomaterial to a membrane could increase viral retention. For example, addition of nano-silver oxide,42 magnetite (iron oxide),58 and titania59 to ultrafiltration membrane surfaces resulted in multi-log increases in virus removal due to increased electrostatic adsorption. However, the enhanced removal was limited by accessible nanoparticle surface area for viral adsorption.
A design to enhance virus-nanoparticle adsorptive removal would be to generate a 1D nanomaterial fibrous filter with an exemplary positively charged surface area to volume ratio (>5 m2 mL−1).60 A nanofiber filter (dpore ∼ 8dfiber) would also be expected to have a relatively low pressure drop. For this process, the primary depth filtration mechanisms for individual virus and virus containing aggregates or aerosols would be diffusion, electromigration, interception, and inertial impaction.61 Alumina is a common metal oxide with a zero-point charge of 8–9.5 and can be formed into nanofibers using facile methods. For instance, an alumina nanofiber filter (dfiber = 2–4 nm) was reported to remove viral aerosols (10–400 nm) with both a lower removal efficiency and pressure drop than HEPA filters resulting in a slightly improved filter quality factor.62 The alumina nanofilter was also minimally affected by relative humidity and more importantly resulted in a multi-log reduction in extracted virus viability as compared to conventional glass fiber filters. An electropositive alumina nanofiber filter was also reported to be effective for aqueous viral adsorption at higher flow rates as compared to classical virus membrane filters without the need for pre-filtration, acidification, or multi-valent cation addition.63 Similar multi-log viral removal performance was observed for nanohematite coated glass fibers64 and quaternary amine functionalized chitosan nanofibers.65
In summary, positively-charged nanofiber filters perform well toward virus removal due to large surface area to volume ratios, relatively low pressure drop, and high surface charge densities. However, since these filters operate by depth filtration, the viral deposition sites will eventually be consumed58 necessitating periodic filter regeneration. Environmental matrix effects such as competitive adsorption of negatively-charged natural organic matter are also expected to interfere with virus removal performance.64 Therefore, hybridization of filtration and viral inactivation processes should be considered and again engineered nanomaterials may offer potential solutions. Photocatalytic TiO2 filters have been reported to be effective for viral removal and inactivation from both water and air.66,67 However, the incorporation of a photon source into a filtration device can be complex, although low-cost UV-LED development may offer novel configurations.68 Thus, an alternative external stimulus may be necessary such as application of an electric potential, which would be viable if the nanofiber filter material was conductive. Engineered carbon nanomaterials69 such as carbon nanotubes (CNT) meet this requirement due to their unique set of properties: 1D morphology, electrical conductivity, mechanical stability, ease of surface/structure manipulation, and ability to form fibrous network. The inherent CNT antimicrobial activity resulted in a vast amount of work over the past decade on CNT germicidal mechanisms and structure–activity relationships.69–74 However, similar antiviral studies have been buried by the CNT–microbial interaction research. For example, a thin CNT filter was not only effective for microbe removal, but also quite effective for multi-log viral removal.75–77 Even though the CNT have a slight negative charge, the strong CNT–virus vdW attractive force overcomes the electrostatic repulsion resulting in effective viral filter attachment.78
Initial studies on CNT filters supported by porous polymer substrates indicated that MWNT had optimal performance toward aqueous viral removal due to the uniformity of the vacuum filtered CNT network.78,79 The thin (∼20 μm) CNT (d = 20–40 nm diameter; l = 50–100 μm) filter (10
000 LMH bar) removed >4
log (WHO recommended goal) of the influent MS2 virus (d = 25 nm vs. 100 nm for COVID-19) by depth filtration over a range of aqueous conditions representative of typical waters.80 As expected, increased concentrations of natural organic matter reduced the MWNT viral removal efficacy (<2
log) likely due to steric repulsion and competition for deposition sites. The few subsequent CNT filtration works have focused on material development: reduction of CNT mass in filter,81 addition of germicidal Ag for inactivation,82 and addition of metal oxide coating to alter surface charge for increased removal.83 However, none of these improvements have led to a technological breakthrough or a resurgence in CNT–virus interaction research.
The ultimate potential of the CNT filter for virus control may be discovered in simultaneous utilization of the multitude of CNT properties. For example, the CNT electrical conductivity would allow for concurrent or post CNT network filtration viral disinfection via electrochemistry,33 electroporation,84 or resistive heating.85 An electroactive MWNT filter was reported to enhance viral removal from 4
log in the absence of applied potential to >6
log (effluent virus < LODPFU) at low potentials of 2–3 V.33 Not only was the viral removal enhanced, the virus extracted from the filter post-filtration were observed to be electrochemically inactivated at >99% at 2 V and >99.99% at 3 V (<LODPFU).33,86 The electroactive CNT filter also overcame the negative effects of natural organic matter on viral removal efficacy maintaining high performance over a wide range of aquatic conditions.86 The electro-enhanced viral removal was due to both increased viral electromigration transport to the anodic CNT filter surface and increased attractive electrostatic CNT–virus interactions. However, even though there have been general advances in electroactive CNT membrane research and technology87,88 such as potential for wastewater effluent polishing,89 there has been limited progress in the area of CNT–virus interactions and control.
Although the initial environmental work on CNT filtration for aqueous viral removal and inactivation was promising, the research has not developed much in recent years. This statement resounds even stronger for CNT air filtration due to the potential of an ultrathin CNT filter to operate in the free molecular flow regime and overcome the classical air filtration efficiency—pressure drop balance.90,91 For example, an early CNT air filter study reported that a micron-thin (1–2 μm) CNT (d = 20–50 nm) filter (dpore = 600 nm; >90% porosity) deposited on a cellulose acetate fiber filter (d = 20–50 μm; 200 μm thick) had a >99.9% efficacy for removal of 300 nm aerosols at a negligible pressure drop (<3 kPa).92 A similar result was observed for an aligned cross-plied CNT filter that met HEPA requirements.93 One of the only studies on airborne viral CNT filtration, indicated that >90% of the MS2 deposited onto a CNT coated glass fiber filter were also inactivated.94 Thus, CNT composite filters may have potential for airborne virus control in terms of both removal and inactivation.
The potential implications of utilizing CNT for air filtration must first be addressed. A primary concern would be the potential for release of CNT from the filter and subsequent inhalation. A number of methods have been reported to enhance stability of CNT polymer composites such as covalent bonding,95 mechanical compression,96 and physical incorporation97 such that CNT are not released during physical filtration. Thus, similar methods could be utilized here. Another concern would be dynamic performance loss (fouling) during CNT aerosol filtration due to the extremely thin nature of the filter. A range of electroactive fouling reduction and recovery techniques have been reported in the aqueous filtration literature.98,99 Similar methods could be utilized during air filtration, for example, a recent study on a CNT coated stainless steel mesh air filter reported it could be regenerated by resistive heating (4 V; 3.1 A; 3 h; ∼450 °C) that burned off >98% of the deposited PM2.5 particles.34 Therefore, although the investigations of nanofiber filters for airborne virus removal have been limited, there is potential, in particular for reactive nanofiber filters, to provide innovative solutions.
Outlook and future directions
Nanoparticles' compatible size and adaptable morphology, surface chemistry, and reactivity offer great potential for engineered nanomaterial utility as an antiviral for disinfection and filtration, and even therapeutics. Advancement has been hindered by a general lack of research in this area, which may arise from difficulties of cultivating, characterizing, and inactivating the viral nanobiomachine (especially if pathogenic). Thus, interdisciplinary collaboration between viral microbiologists and nanomaterial scientists will be key to future developments.
The general design parameters discussed in this review can give initial guidance on engineering antiviral nanomaterials by determining if the issue better addressed with batch (3D NP) or flow (1D nanofiber) reactor, if inactivation necessary, and if so what stimulation is most amenable. However, the antiviral nanomaterial toolbox is still relatively limited as compared to other nanomaterial applications given the near infinite combinations of nanomaterial surface chemistry and morphology. A similar but more impactful statement can be made about potential nanomaterial utility in regard to virus type since the current range of viruses evaluated has been extremely narrow. As the investigation of all virus-nanomaterial pairs is untenable, fundamental bio-physical–chemical studies of the NP-virus binding sites and energies, reaction mechanisms and kinetics, and subsequent effects on bioactivity and function are needed. An improved fundamental understanding of virus-nanoparticles interactions and reactions will allow for rational design of antiviral nanomaterials.
As with any environmental nanotechnology, the environmental aspects of antiviral nanomaterial use must also be considered. Most studies to date have been completed in relatively ‘clean’ synthetic environments, thus matrix impacts on antiviral efficacy should be evaluated. For example, similar to viruses, most environmental colloids are negatively-charged and may interfere with the viral-nanomaterial interactions indicating nanomaterials with viral surface specificity such as glycoprotein mimics may be needed. Another consideration would be the nanomaterial environmental stability due to their reactive and large specific surface area that has high passivation/dissolution potential. There should also be concern for nanomaterial release, in particular for 3D NP, as this will have environmental implications, at least the high cost of a single use nanomaterial.
Conflicts of interest
There are no conflicts to declare.
References
- T. M. Cook, Personal protective equipment and concerns over airborne transmission of COVID-19: A reply, Anaesthesia, 2020, 75(8), 1117–1118 CrossRef CAS
.
- B. E. Howard, High-risk aerosol-generating procedures in COVID-19: Respiratory protective equipment considerations, Otolaryngol.–Head Neck Surg., 2020, 163(1), 98–103 CrossRef
.
- A. Konda, A. Prakash, G. A. Moss, M. Schmoldt, G. D. Grant and S. Guha, Aerosol filtration efficiency of common fabrics used in respiratory cloth masks, ACS Nano, 2020, 14, 6339–6347 CrossRef CAS
.
- W. W.-F. Leung and Q. Sun, Charged PVDF multilayer nanofiber filter in filtering simulated airborne novel coronavirus (COVID-19) using ambient nano-aerosols, Sep. Purif. Technol., 2020, 245, 116887 CrossRef CAS
.
- R. Das, C. D. Vecitis, A. Schulze, B. Cao, A. F. Ismail, X. Lu, J. Chen and S. Ramakrishna, Recent advances in nanomaterials for water protection and monitoring, Chem. Soc. Rev., 2017, 46, 6946–7020 RSC
.
- X. Qu, J. Brame, Q. Li and P. J. J. Alvarez, Nanotechnology for a safe and sustainable water supply: Enabling integrated water treatment and reuse, Acc. Chem. Res., 2013, 46, 834–843 CrossRef CAS
.
- K. Bibby, R. J. Fischer, L. W. Casson, E. Stachler, C. N. Haas and V. J. Munster, Persistence of Ebola virus in sterilized wastewater, Environ. Sci. Technol., 2015, 2, 245–249 CAS
.
- Y. Ye, R. M. Ellenberg, K. E. Graham and K. R. Wigginton, Survivability, partitioning, and recovery of enveloped viruses in untreated municipal wastewater, Environ. Sci. Technol., 2016, 50, 5077–5085 CrossRef CAS
.
- K. Lin and L. C. Marr, Aerosolization of Ebola virus surrogates in wastewater systems, Environ. Sci. Technol., 2017, 51, 2669–2675 CrossRef CAS
.
- L. Cai, C. Liu, G. Fan, C. Liu and X. Sun, Preventing viral disease by ZnONPs through directly deactivating TMV and activating plant immunity in Nicotiana benthamiana, Environ. Sci.: Nano, 2019, 6, 3653–3669 RSC
.
- P. Yu,
et al., Bottom-up biofilm eradication using bacteriophage-loaded magnetic nanocomposites: A computational and experimental study, Environ. Sci.: Nano, 2019, 6, 3539–3550 RSC
.
-
M. G. Mateu, The structural basis of virus function, in Structure and Physics of Viruses, ed. M. G. Mateu, Springer, Netherlands, 2013, pp. 3–52 Search PubMed
.
-
J. R. Caston and J. L. Carrascosa, The basic architecture of viruses, in Structure and Physics of Viruses, ed. M. G. Mateu, Springer, Netherlands, 2013, pp. 53–77 Search PubMed
.
-
C. San Martin, Structure and assembly of complex viruses, in Structure and Physics of Viruses, ed. M. G. Mateu, Springer, Netherlands, 2013, pp. 329–360 Search PubMed
.
-
J. M. Casasnovas, Virus-receptor interactions and receptor-mediated virus entry into host cells, in Structure and Physics of Viruses, ed. M. G. Mateu, Springer, Netherlands, 2013, pp. 441–466 Search PubMed
.
- A. Armanious, M. Aeppli, R. Jacak, D. Refardt, T. Sigstam, T. Kohn and M. Sander, Viruses at solid water interfaces: A systematic assessment of interactions driving adsorption, Environ. Sci. Technol., 2016, 50, 732–743 CrossRef CAS
.
- K. R. Wigginton, L. Menin, J. P. Montoya and T. Kohn, Oxidation of virus proteins during UV254 and singlet oxygen mediated inactivation, Environ. Sci. Technol., 2010, 44, 5437–5443 CrossRef
.
- Y. Ye, P. H. Chang, J. Hartert and K. R. Wigginton, Reactivity of enveloped virus genome, proteins, and lipids with free chlorine and UV254, Environ. Sci. Technol., 2018, 52, 7698–7708 CrossRef CAS
.
- K. R. Wigginton, B. M. Pecson, T. Sigstam, F. Bosshard and T. Kohn, Virus inactivation mechanisms: Impact of disinfectants on virus function and structural integrity, Environ. Sci. Technol., 2012, 46, 12069–12078 CrossRef CAS
.
- K. R. Wigginton and A. B. Boehm, Environmental engineers and scientists have important roles to play in stemming outbreaks and pandemics caused by enveloped viruses, Environ. Sci. Technol., 2020, 54, 3736–3739 CrossRef CAS
.
- K. Lin and L. C. Marr, Humidity-dependent decay of viruses, but not bacteria, in aerosols and droplets follows disinfection kinetics, Environ. Sci. Technol., 2020, 54, 1024–1032 CrossRef CAS
.
- J. I. Nieto-Juarez and T. Kohn, Virus removal and inactivation by iron (hydr)oxide-mediated Fenton-like processes under sunlight and in the dark, Photochem. Photobiol. Sci., 2013, 12, 1596–1605 RSC
.
- S. Jin, P. H. Fallgren, J. M. Morris and Q. Chen, Removal of bacteria and viruses from waters using layered double hydroxide nanocomposites, Sci. Technol. Adv. Mater., 2007, 8(1–2), 67–70 CrossRef CAS
.
- K. Wong, B. Mukherjee, A. M. Kahler, R. Zepp and M. Molina, Influence of inorganic ions on aggregation and adsorption behaviors of human adenovirus, Environ. Sci. Technol., 2012, 46(20), 11145–11153 CrossRef CAS
.
- X. Mi and C. L. Heldt, Adsorption of a non-enveloped mammalian virus to functionalized nanofibers, Colloids Surf., B, 2014, 121, 319–324 CrossRef CAS
.
- S. H. Zhan, Y. Yang, Z. Q. Shen, J. J. Shan, Y. Li, S. S. Yang and D. D. Zhu, Efficient removal of pathogenic bacteria and viruses by multifunctional amine-modified magnetic nanoparticles, J. Hazard. Mater., 2014, 274, 115–123 CrossRef CAS
.
- S. D. Snow, K. Park and J.-H. Kim, Cationic fullerene aggregates with unprecedented virus photoinactivation efficiencies in water, Environ. Sci. Technol., 2014, 1, 290–294 CAS
.
- B. M. Illescas, J. Rojo, R. Delgado and N. Martin, Multivalent glycosylated nanostructures to inhibit Ebola virus infection, J. Am. Chem. Soc., 2017, 139, 6018–6025 CrossRef CAS
.
- M. F. Gholami,
et al., Functionalized graphene as extracellular matrix mimics: Toward well-defined 2D nanomaterials for multivalent virus interactions, Adv. Funct. Mater., 2017, 27(15), 1606477 CrossRef
.
- C. Zhang, Y. Li, D. Shuai, Y. Shen and D. Wang, Progress and challenges in photocatalytic disinfection of waterborne viruses: A review to fill current knowledge gaps, Chem. Eng. J., 2019, 355, 399–415 CrossRef CAS
.
- C. J. Gannon,
et al., Carbon nanotube-enhanced thermal destruction of cancer cells in a noninvasive radiofrequency field, Cancer, 2007, 110, 2654–2665 CrossRef CAS
.
- N. W. S. Kam, M. O'Connell, J. A. Wisdom and H. J. Dai, Carbon nanotubes as multifunctional biological transporters and near-infrared agents for selective cancer cell destruction, Proc. Natl. Acad. Sci. U. S. A., 2005, 102, 11600–11605 CrossRef CAS
.
- C. D. Vecitis, M. H. Schnoor, M. S. Rahaman, J. D. Schiffman and M. Elimelech, Electrochemical multiwalled carbon nanotube filter for viral and bacterial removal and inactivation, Environ. Sci. Technol., 2011, 45, 3672–3679 CrossRef CAS
.
- K. Yang, Z. Yu, C. Yu, H. Chen and F. Pan, An electrically renewable air filter with integrated 3D nanowire networks, Adv. Mater. Technol., 2019, 4(7), 1900101 CrossRef CAS
.
- H. H. Lara, E. N. Garza-Trevino, L. Ixtepan-Turrent and D. K. Singh, Silver nanoparticles are broad-spectrum bactericidal and virucidal compounds, J. Nanobiotechnol., 2011, 9, 8 CrossRef
.
- J. L. Elechiguerra, J. L. Burt, J. R. Morones, A. Camacho-Bragado, X. Gao, H. H. Lara and M. J. Yacaman, Interaction of silver nanoparticles with HIV-1, J. Nanobiotechnol., 2005, 3, 6 CrossRef
.
- R. W. Y. Sun, R. Chen, N. P. Y. Chung, C. M. Ho, C. L. S. Lin and C. M. Che, Silver nanoparticles fabricated in Hepes buffer exhibit cytoprotective activities toward HIV-1 infected cells, Chem. Commun., 2005, 5059–5061, 10.1039/b510984a
.
- L. Lu, R. W. Y. Sun, R. Chen, C. K. Hui, C. M. Ho, J. M. Luk, G. K. K. Lau and C. M. Che, Silver nanoparticles inhibit hepatitis b virus replication, Antiviral Ther., 2008, 13, 253–262 CAS
.
- J. V. Rogers, C. V. Parkinson, Y. W. Choi, J. L. Speshock and S. M. Hussain, A preliminary assessment of silver nanoparticle inhibition of monkeypox virus plaque formation, Nanoscale Res. Lett., 2008, 3, 129–133 CrossRef
.
- B. De Gusseme, L. Sintubin, L. Baert, E. Thibo, T. Hennebel, G. Vermeulen, M. Uyttendaele, W. Verstraete and N. Boon, Biogenic silver for disinfection of water contaminated with viruses, Appl. Environ. Microbiol., 2010, 76, 1082–1087 CrossRef CAS
.
- J. Y. Kim, C. Lee, D. C. Love, D. L. Sedlak, J. Yoon and K. L. Nelson, Inactivation of MS2 coliphage by ferrous ion and zero-valent iron nanoparticles, Environ. Sci. Technol., 2011, 45, 6978–6984 CrossRef CAS
.
- K. Zodrow, L. Brunet, S. Mahendra, D. Li, A. Zhang, Q. Li and P. J. J. Alvarez, Polysulfone ultrafiltration membranes impregnated with silver nanoparticles show improved biofouling resistance and virus removal, Water Res., 2009, 43, 715–723 CrossRef CAS
.
- P. Orlowski,
et al., Tannic acid modified silver nanoparticles show antiviral activity in herpes simplex virus type 2 infection, PLoS One, 2014, 9(8), e104113 CrossRef
.
- X. F. Hang, H. R. Peng, H. Y. Song, Z. T. Qi, X. H. Miao and W. S. Xu, Antiviral activity of cuprous oxide nanoparticles against hepatitis C virus in vitro, J. Virol. Methods, 2015, 222, 150–157 CrossRef CAS
.
- X. X. Yang, C. M. Li and C. Z. Huang, Curcumin modified silver nanoparticles for highly efficient inhibition of respiratory syncytial virus infection, Nanoscale, 2016, 8, 3040–3048 RSC
.
- Y. Hao, W. Yuan, C. X. Ma, J. C. White, Z. T. Zhang, M. Adeel, T. Zhou, Y. K. Rui and B. S. Xing, Engineered nanomaterials suppress Turnip mosaic virus infection in tobacco (Nicotiana benthamiana), Environ. Sci.: Nano, 2018, 5, 1685–1693 RSC
.
- H. E. Kim, H. J. Lee, M. S. Kim, T. Kim, H. Lee, H. H. Kim, M. Cho, S. W. Hong and C. Lee, Differential microbicidal effects of bimetallic iron-copper nanoparticles on Escherichia coli and MS2 coliphage, Environ. Sci. Technol., 2019, 53, 2679–2687 CrossRef CAS
.
- M. R. Hoffmann, S. T. Martin, W. Y. Choi and D. W. Bahnemann, Environmental applications of semiconductor photocatalysis, Chem. Rev., 1995, 95, 69–96 CrossRef CAS
.
- J. C. Sjogren and R. A. Sierka, Inactivation of phage MS2 by iron-aided titanium-dioxide photocatalysis, Appl. Environ. Microbiol., 1994, 60, 344–347 CrossRef CAS
.
- D. Gerrity, H. Ryu, J. Crittenden and M. Abbaszadegan, Photocatalytic inactivation of viruses using titanium dioxide nanoparticles and low-pressure UV light, J. Environ. Sci. Health, Part A: Toxic/Hazard. Subst. Environ. Eng., 2008, 43, 1261–1270 CrossRef CAS
.
- M. V. Liga, E. L. Bryant, V. L. Colvin and Q. L. Li, Virus inactivation by silver doped titanium dioxide nanoparticles for drinking water treatment, Water Res., 2011, 45, 535–544 CrossRef CAS
.
- Y. Koizumi and M. Taya, Kinetic evaluation of biocidal activity of titanium dioxide against phage MS2 considering interaction between the phage and photocatalyst particles, Biochem. Eng. J., 2002, 12, 107–116 CrossRef CAS
.
- B. Guo, S. D. Snow, B. J. Starr, I. Xagoraraki and V. V. Tarabara, Photocatalytic inactivation of human adenovirus 40: Effect of dissolved organic matter and prefiltration, Sep. Purif. Technol., 2018, 193, 193–201 CrossRef CAS
.
- M. Cho, E. L. Cates and J.-H. Kim, Inactivation and surface interactions of MS-2 bacteriophage in a TiO2 photoelectrocatalytic reactor, Water Res., 2011, 45, 2104–2110 CrossRef CAS
.
- H. R. Jafry, M. V. Liga, Q. Li and A. R. Barron, Simple route to enhanced photocatalytic activity of P25 titanium dioxide nanoparticles by silica addition, Environ. Sci. Technol., 2011, 45, 1563–1568 CrossRef CAS
.
- A. R. Badireddy, J. F. Budarz, S. Chellam and M. R. Wiesner, Bacteriophage inactivation by UV-A illuminated fullerenes: Role of nanoparticle-virus association and biological targets, Environ. Sci. Technol., 2012, 46, 5963–5970 CrossRef CAS
.
- R. van Reis and A. Zydney, Bioprocess membrane technology, J. Membr. Sci., 2007, 297, 16–50 CrossRef CAS
.
- I. Raciny, K. R. Zodrow, D. Li, Q. Li and P. J. J. Alvarez, Addition of a magnetite layer onto a polysulfone water treatment membrane to enhance virus removal, Water Sci. Technol., 2011, 63, 2346–2352 CrossRef CAS
.
- X. Zheng, D. Chen, Z. Wang, Y. Lei and R. Cheng, Nano-TiO2 membrane adsorption reactor (MAR) for virus removal in drinking water, Chem. Eng. J., 2013, 230, 180–187 CrossRef CAS
.
- H. Liu, J. Liu, Y. B. Liu, K. Bertoldi and C. D. Vecitis, Quantitative 2D electrooxidative carbon nanotube filter model: Insight into reactive sites, Carbon, 2014, 80, 651–664 CrossRef CAS
.
- M. Pan, J. A. Lednicky and C. Y. Wu, Collection, particle sizing and detection of airborne viruses, J. Appl. Microbiol., 2019, 127, 1596–1611 CrossRef CAS
.
- H. W. Li, C. Y. Wu, F. Tepper, J. H. Lee and C. N. Lee, Removal and retention of viral aerosols by a novel alumina nanofiber filter, J. Aerosol Sci., 2009, 40, 65–71 CrossRef CAS
.
- D. Li, H. C. Shi and S. C. Jiang, Concentration of viruses from environmental waters using nanoalumina fiber filters, J. Microbiol. Methods, 2010, 81, 33–38 CrossRef CAS
.
- L. Gutierrez, X. Li, J. W. Wang, G. Nangmenyi, J. Economy, T. B. Kuhlenschmidt, M. S. Kuhlenschmidt and T. H. Nguyen, Adsorption of rotavirus and bacteriophage MS2 using glass fiber coated with hematite nanoparticles, Water Res., 2009, 43, 5198–5208 CrossRef CAS
.
- X. Mi, K. S. Vijayaragavan and C. L. Heldt, Virus adsorption of water-stable quaternized chitosan nanofibers, Carbohydr. Res., 2014, 387, 24–29 CrossRef CAS
.
- T. Daikoku, M. Takemoto, Y. Yoshida, T. Okuda, Y. Takahashi, K. Ota, F. Tokuoka, A. T. Kawaguchi and K. Shiraki, Decomposition of organic chemicals in the air and inactivation of aerosol-associated influenza infectivity by photocatalysis, Aerosol Air Qual. Res., 2015, 15, 1469–1484 CrossRef CAS
.
- G. Y. Rao, K. S. Brastad, Q. Y. Zhang, R. Robinson, Z. He and Y. Li, Enhanced disinfection of Escherichia coli and bacteriophage MS2 in water using a copper and silver loaded titanium dioxide nanowire membrane, Front. Environ. Sci. Eng., 2016, 10(4), 11 CrossRef
.
- H. Amano,
et al., The 2020 UV emitter roadmap, J. Phys. D: Appl. Phys., 2020, 53, 503001 CrossRef CAS
.
- S. C. Smith and D. F. Rodrigues, Carbon-based nanomaterials for removal of chemical and biological contaminants from water: A review of mechanisms and applications, Carbon, 2015, 91, 122–143 CrossRef CAS
.
- S. Kang, M. Herzberg, D. F. Rodrigues and M. Elimelech, Antibacterial effects of carbon nanotubes: Size does matter, Langmuir, 2008, 24, 6409–6413 CrossRef CAS
.
- S. Kang, M. Pinault, L. D. Pfefferle and M. Elimelech, Single-walled carbon nanotubes exhibit strong antimicrobial activity, Langmuir, 2007, 23, 8670–8673 CrossRef CAS
.
- C. D. Vecitis, K. R. Zodrow, S. Kang and M. Elimelech, Electronic-structure-dependent bacterial cytotoxicity of single-walled carbon nanotubes, ACS Nano, 2010, 4, 5471–5479 CrossRef CAS
.
- Q. Li, S. Mahendra, D. Y. Lyon, L. Brunet, M. V. Liga, D. Li and P. J. J. Alvarez, Antimicrobial nanomaterials for water disinfection and microbial control: Potential applications and implications, Water Res., 2008, 42, 4591–4602 CrossRef CAS
.
- L. Wang, Z. Yuan, H. E. Karahan, Y. Wang, X. Sui, F. Liu and Y. Chen, Nanocarbon materials in water disinfection: State-of-the-art and future directions, Nanoscale, 2019, 11, 9819–9839 RSC
.
- A. S. Brady-Estevez, S. Kang and M. Elimelech, A single-walled-carbon-nanotube filter for removal of viral and bacterial pathogens, Small, 2008, 4, 481–484 CrossRef CAS
.
- A. Srivastava, O. N. Srivastava, S. Talapatra, R. Vajtai and P. M. Ajayan, Carbon nanotube filters, Nat. Mater., 2004, 3, 610–614 CrossRef CAS
.
- S. T. Mostafavi, M. R. Mehrnia and A. M. Rashidi, Preparation of nanofilter from carbon nanotubes for application in virus removal from water, Desalination, 2009, 238, 271–280 CrossRef CAS
.
- A. S. Brady-Estevez, M. H. Schnoor, C. D. Vecitis, N. B. Saleh and M. Ehmelech, Multiwalled carbon nanotube filter: Improving viral removal at low pressure, Langmuir, 2010, 26, 14975–14982 CrossRef CAS
.
- A. S. Brady-Estevez, M. H. Schnoor, S. Kang and M. Elimelech, SWNT-MWNT hybrid filter attains high viral removal and bacterial inactivation, Langmuir, 2010, 26, 19153–19158 CrossRef CAS
.
- A. S. Brady-Estevez, T. H. Nguyen, L. Gutierrez and M. Elimelech, Impact of solution chemistry on viral removal by a single-walled carbon nanotube filter, Water Res., 2010, 44, 3773–3780 CrossRef CAS
.
- F. Ahmed, C. M. Santos, J. Mangadlao, R. Advincula and D. F. Rodrigues, Antimicrobial PVK:SWNT nanocomposite coated membrane for water purification: Performance and toxicity testing, Water Res., 2013, 47, 3966–3975 CrossRef CAS
.
- J. P. Kim, J. H. Kim, J. Kim, S. N. Lee and H.-O. Park, A nanofilter composed of carbon nanotube-silver composites for virus removal and antibacterial activity improvement, J. Environ. Sci., 2016, 42, 275–283 CrossRef CAS
.
- Z. Nemeth, G. P. Szekeres, M. Schabikowski, K. Schrantz, J. Traber, W. Pronk, K. Hernadi and T. Graule, Enhanced virus filtration in hybrid membranes with MWCNT nanocomposite, R. Soc. Open Sci., 2019, 6(1), 181294 CrossRef CAS
.
- C. Liu, X. Xie, W. Zhao, N. Liu, P. A. Maraccini, L. M. Sassoubre, A. B. Boehm and Y. Cui, Conducting nanosponge electroporation for affordable and high-efficiency disinfection of bacteria and viruses in water, Nano Lett., 2013, 13, 4288–4293 CrossRef CAS
.
- H.-S. Jang, S. K. Jeon, K.-S. Ryu and S. H. Nahm, Removal of virus and toxin using heatable multi-walled carbon nanotube web filters, AIP Adv., 2016, 6(2), 025020 CrossRef
.
- M. S. Rahaman, C. D. Vecitis and M. Elimelech, Electrochemical carbon-nanotube filter performance toward virus removal and inactivation in the presence of natural organic matter, Environ. Sci. Technol., 2012, 46, 1556–1564 CrossRef CAS
.
- S. A. Jame and Z. Zhou, Electrochemical carbon nanotube filters for water and wastewater treatment, Nanotechnol. Rev., 2016, 5, 41–50 CAS
.
- Y. Liu, G. Gao and C. D. Vecitis, Prospects of an Electroactive Carbon Nanotube Membrane toward Environmental Applications, Acc. Chem. Res., 2020 DOI:10.1021/acs.accounts.0c00544
.
- Y. B. Liu, H. Liu, Z. Zhou, T. R. Wang, C. N. Ong and C. D. Vecitis, Degradation of the common aqueous antibiotic tetracycline using a carbon nanotube electrochemical filter, Environ. Sci. Technol., 2015, 49, 7974–7980 CrossRef CAS
.
- P. Li, C. Wang, Y. Zhang and F. Wei, Air filtration in the free molecular flow regime: A review of high-efficiency particulate air filters based on carbon nanotubes, Small, 2014, 10, 4543–4561 CrossRef CAS
.
- Z. Zou and M. Yao, Airflow resistance and bio-filtering performance of carbon nanotube filters and current facepiece respirators, J. Aerosol Sci., 2015, 79, 61–71 CrossRef CAS
.
- G. Viswanathan, D. B. Kane and P. J. Lipowicz, High efficiency fine particulate filtration using carbon nanotube coatings, Adv. Mater., 2004, 16(22), 2045–2049 CrossRef CAS
.
- O. Yildiz and P. D. Bradford, Aligned carbon nanotube sheet high efficiency particulate air filters, Carbon, 2013, 64, 295–304 CrossRef CAS
.
- K.-T. Park and J. Hwang, Filtration and inactivation of aerosolized bacteriophage MS2 by a CNT air filter fabricated using electro-aerodynamic deposition, Carbon, 2014, 75, 401–410 CrossRef CAS
.
- A. Tiraferri, C. D. Vecitis and M. Elimelech, Covalent binding of single-walled carbon nanotubes to polyamide membranes for antimicrobial surface properties, ACS Appl. Mater. Interfaces, 2011, 3, 2869–2877 CrossRef CAS
.
- Q. Zhang, P. Arribas, E. M. Remillard, M. Carmen Garcia-Payo, M. Khayet and C. D. Vecitis, Interlaced CNT electrodes for bacterial fouling reduction of microfiltration membranes, Environ. Sci. Technol., 2017, 51, 9176–9183 CrossRef CAS
.
- G. Gao, Q. Zhang and C. D. Vecitis, CNT-PVDF composite flow-through electrode for single-pass sequential reduction-oxidation, J. Mater. Chem. A, 2014, 2, 6185–6190 RSC
.
- A. Ronen, S. L. Walker and D. Jassby, Electroconductive and electroresponsive membranes for water treatment, Rev. Chem. Eng., 2016, 32, 533–550 CAS
.
- Q. Zhang and C. D. Vecitis, Conductive CNT-PVDF membrane for capacitive organic fouling reduction, J. Membr. Sci., 2014, 459, 143–156 CrossRef CAS
.
|
This journal is © The Royal Society of Chemistry 2021 |