DOI:
10.1039/C9QM00154A
(Research Article)
Mater. Chem. Front., 2019,
3, 1823-1832
A novel family of AIE-active meso-2-ketopyrrolyl BODIPYs: bright solid-state red fluorescence, morphological properties and application as viscosimeters in live cells†
Received
13th March 2019
, Accepted 25th June 2019
First published on 26th June 2019
Abstract
Fluorescent probes towards viscosity determination can be used to report on variations in local molecular viscosity and have become valuable tools for the study of intracellular microenvironments. Herein, we develop a family of remarkable meso-2-ketopyrrolyl-derived BODIPY rotors and report a new strategy for building them via simple condensation reactions between oxalyl chloride and substituted pyrroles. They are well characterized by NMR, HRMS, crystal structure, spectroscopic and morphological studies. These uncommon meso-2-ketopyrrolyl-derived BODIPYs are weakly fluorescent in their organic solutions but exhibit bright solid-state red fluorescence from 620 to 661 nm with a maximum fluorescence quantum yield of 25%. All of them also exhibit notable aggregation-induced emission (AIE)-active features. Interestingly, the formed nanoaggregates were observed to exhibit morphological distinctions owing to different substituents. The fluorescence enhancement towards the increased viscosity of the environment might be mainly attributed to the restriction of their intramolecular rotations of the meso-ketopyrrolyl groups. More interestingly, the increase in the fluorescence lifetime of these representative rotors appears to perfectly correlate with the increase in the viscosity of the media, and they have been used as viscosimeters for real-time quantitative determination of the variation of the intracellular viscosity in live cells. The easy synthetic strategy and real-time quantitative determination of the variation pave a new way for creating fluorescent molecular rotor materials.
1. Introduction
Intracellular micro-viscosity has a very important role in the study of multifarious signal transportation and cellular processes such as reticulophagy and division/death of cells.1 Among various strategies for measuring viscosity directly, membrane permeable fluorescent molecular rotors (FMRs) that are sensitive to the viscosity of the surrounding microenvironments have been regarded as a class of the most successful probes for intracellular viscosity.2,3 The developed rotors suitable for fluorescence intensity or fluorescence lifetime imaging techniques usually undergo molecular rotation and further effectively dissipate their excitation energies. The corresponding fluorescence signals could be used to directly report the variations in local molecular viscosity, especially for some abnormal changes, which are closely associated with many diseases and pathologies including diabetes and Alzheimer's disease.4
Recently, some elegant FMRs based on fluorescent BODIPY (boron dipyrromethene)5 segments have been developed due to their high fluorescence quantum yields and the high rotational ability of the substituents at the meso-position, giving a linear correlation between their fluorescence lifetime and viscosity, and widely applied as viscosity sensors in intracellular microenvironments in fluorescence lifetime imaging microscopy (FLIM) techniques.1d,6–11 However, most of the recently developed BODIPY-based FMRs almost have similar cores of meso-phenyl substituted BODIPYs.1b,6–10 The efficient strategy for these BODIPY FMRs is based on the rotation of the meso-phenyl group around a C–C single bond, which causes a non-radiative decay in the excited state in non-viscous media.9 An increase of viscosity makes it more difficult to rotate the meso-phenyl group. This inhibits the non-radiative decay process and brings an increase of fluorescence lifetime. Using this strategy, one group of us previously developed a BODIPY-based FMR for high temporal and spatial determination of lysosomal viscosity in living cells through FLIM.10 Different from conventional BODIPY-based FMRs, only one example of BODIPY B distorted from internal steric hindrance between the 1,7-dimethyl group and the meso-CHO was found as an impactful viscosimeter in 2014 by Fan and Peng.11 Nevertheless, despite some advantages, BODIPY dyes exhibit some drawbacks such as low fluorescence quantum yields in the solid state and relatively complicated syntheses. Based on the above studies, we rationalized that a less bulky group such as 2-ketopyrrolyl (than phenyl) at the meso-position of a BODIPY at the same time together with the 1,7-dimethyl group may allow its rotation in non-viscous media. This kind of BODIPY dye might show bright solid-state emission and provide an alternative strategy for synthesizing novel unconventional and efficient viscosity probes.
Herein, we develop a new strategy for building uncommon meso-2-ketopyrrolyl BODIPY derivatives containing the 1,7-dimethyl group (Fig. 1), which exhibit great performance comparable or even superior to those of conventional BODIPY-based FMRs. Firstly, the easy synthetic method of the two meso-2-ketopyrrole derived BODIPYs has been reported for the first time via simple condensation between commercial oxalyl chloride and pyrrole derivatives. Secondly, these rotors unexpectedly showed notable aggregation-induced emission (AIE) features and bright red fluorescence in the solid state. Thirdly, their fluorescence intensity and lifetime were observed to perfectly correlate with the viscosity of the medium. The real-time determination of the variation of the viscosity in live cells by using FLIM has been successfully demonstrated, which qualifies them as a novel class of BODIPY-derived molecular rotors.
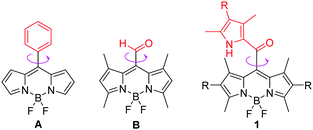 |
| Fig. 1 The chemical structures of FMRs: conventional meso-phenyl derived BODIPY A, meso-aldehyde derived BODIPY B and our designed meso-2-ketopyrrolyl BODIPYs 1 in this contribution. | |
2. Results and discussion
2.1 Synthesis and characterization
Initially, the desired meso-2-ketopyrrolyl BODIPY 1a was synthesized by the condensation of oxalyl chloride with a stoichiometric amount of 2,4-dimethyl-3-ethylpyrrole, and a subsequent BF2 complexation under refluxing conditions (Scheme 1), which was confirmed by single crystal X-ray crystallography. Interestingly, similar condensation of oxalyl chloride with 2 eq. of 2,4-dimethyl-3-ethylpyrrole at room temperature by Akkaya and coworkers gave unexpected two difluoroboron chelated oxalyl-tethered pyrroles.12 The reaction conditions were then optimized and we found that 3.5 eq. of 2,4-dimethyl-3-ethylpyrrole was enough for obtaining the targeted meso-2-ketopyrrolyl derived BODIPY 1a. Under the optimized conditions, the desired meso-2-ketopyrrolyl substituted BODIPYs 1a and 1b were synthesized from the corresponding pyrroles in one pot in 18% and 23% yields, respectively. Dipyrrolyldiketones 2a–b prepared from oxalyl chloride and substituted pyrroles13 were then found to succeed in condensing with another pyrrole molecule under BF3·OEt2, respectively, followed by the complexation with BF3·OEt2 in the presence of triethylamine (Scheme 1), from which the targeted meso-2-ketopyrrolyl BODIPYs 1a–d were prepared in 32–45% yields. The chemical shifts δ of meso-uncoordinated pyrrolic β-H at 5.84 ppm for 1b and 1d, respectively, revealed much higher fields than those of coordinated pyrrolic β-H. These meso-2-ketopyrrolyl BODIPYs were well characterized by 1H and 13C NMR and HRMS. Their structures were further confirmed by single crystal X-ray crystallography. Several meso-ester BODIPYs have been reported;14 however, the synthesis of meso-ketone derived BODIPYs has not been previously developed. Our method developed here provides a facile access to these novel BODIPYs of this kind for the first time. More interestingly, Bae and Kim demonstrated a fluorogenic J-aggregating meso-ester BODIPY recently, which could selectively monitor and image eosinophil peroxidase activity.14e
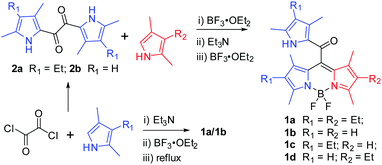 |
| Scheme 1 Syntheses of meso-2-ketopyrrolyl BODIPYs 1a–d. | |
2.2 X-ray crystallography
The crystal structure plots for the meso-2-ketopyrrolyl derived BODIPYs 1a–c are given in Fig. 2 and Fig. S1–S3 in the ESI† and the crystal data are listed in Tables S1 and S2 (ESI†). They all belong to the monoclinic space group C2/c and their boron atoms show a tetrahedral structure. The average B–N distances for 1a–b are almost the same (1.54 Å for 1a and 1.55 Å for 1b), supporting their symmetrical structures. In sharp contrast to them, the average B–N distances of 1c are 1.53 and 1.55 Å, confirming the unsymmetrical structures of 1c and 1d. What deserves to be mentioned is that the dipyrrin cores of these novel meso-2-ketopyrrolyl BODIPYs are planar, which is similar to previously reported BF2 complexes,15 and the dihedral angles defined by two coordinated pyrrolic rings are 5.0°, 8.8° and 1.5° (Table S1, ESI†) for 1a–c, respectively. This indicates that the installation of a steric meso-2-ketopyrrolyl substituent into the BODIPY core causes negligible structural distortion of the planarity of the BODIPY core structure. The carbonyl group as a linker avoided internal steric hindrance between the BODIPY core and the uncoordinated pyrrole ring at the meso-position. The results are quite different from the “distorted BODIPY” B, although they both have the same 1,7-dimethyl group and the carbonyl group. The carbonyl group and the uncoordinated pyrrole ring in their crystals are coplanar. In addition, the dihedral angles defined by the meso-2-ketopyrrolyl moiety and the BODIPY core are 88.8°, 72.9° and 81.4°, respectively, for 1a–c (Table S1, ESI†), which are almost vertical.
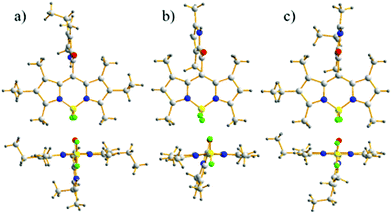 |
| Fig. 2 Front and top views of the X-ray structures for meso-2-ketopyrrolyl BODIPYs 1a–c. C, light gray; H, gray; N, blue; F, bright green; B, yellow; O, red. | |
2.3 Photophysical studies
The absorption and emission spectra (Fig. 3 and Fig. S4–S9, ESI†) of these meso-2-ketopyrrolyl BODIPYs 1a–d were measured in various common organic solvents with different polarities such as cyclohexane, toluene, dichloromethane, tetrahydrofuran, acetonitrile and methanol (Table S3, ESI†). They all exhibit a strong absorption band in the 450–550 nm region. For example, 1a exhibits an intense absorption band centred at 534 nm with a high extinction coefficient of 4.81 × 104 M−1 cm−1 (Fig. 3a and Fig. S4, Table S3, ESI†). With respect to 1b in dichloromethane, obvious bathochromic shifts were observed for 1a and 1c (Fig. 3a and Fig. S4 and S8, ESI†). It is worth mentioning that 1c and 1d with the same unsymmetrical BODIPY core exhibit almost the same absorption and emission (Fig. S8 and S9 and Table S3, ESI†). The results indicate that the enhancement of the intramolecular charge transfer (ICT) effect of alkyl substituents on the coordinated pyrrole ring might mainly contribute to the observed bathochromic shifts. Besides, these meso-2-ketopyrrolyl BODIPYs exhibit relatively large Stokes shifts ranging from 653 to 986 cm−1. In addition, no significant variations of the absorption and emission maxima were observed with the variation of the polarity of the organic solvents for them although a slight variation of the fluorescence intensity was observed (Table S3, ESI†).
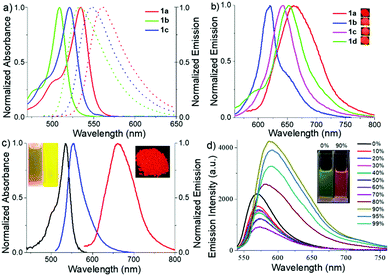 |
| Fig. 3 (a) Overlaid normalized absorption and emission spectra of 1a–c in dichloromethane, excited at 480 nm for 1a and 460 nm for 1b and c. (b) Normalized emission spectra of 1a–d in the powder state, excited at 550 nm for 1a and 500 nm for 1b–d. Photographs of their powders under 365 nm UV lamp irradiation. (c) Normalized absorption (black) and emission (blue) spectra in glycerol, and the emission spectrum in the powder state (red) of 1a. Photographs of the samples in daylight and under 365 nm UV lamp irradiation. (d) Emission spectra of 1a in acetonitrile/water with different water fractions, excited at 500 nm. | |
In comparison with most BODIPY dyes,15 most of these meso-2-ketopyrrolyl BODIPYs are poorly emissive in organic solvents except for 1a, which exhibit relatively much higher fluorescence quantum yields (ϕ) of 0.10–0.21 (Table S3, ESI†). For example, the fluorescence quantum yields were evaluated to be 0.03 and 0.01, respectively, for 1b and 1c in acetonitrile. The possible rotations of the meso-2-ketopyrrolyl group might result in the internal conversion through a non-radiative process. As expected, 1a and 1b indeed show bright fluorescence emission in glycerol, with fluorescence quantum yields of 0.43 and 0.15, respectively (Fig. 3c and Fig. S5 and S7, Table S3, ESI†). In their powder state, they exhibit relatively strong fluorescence as well. Besides, red-shifted fluorescence emissions were observed with maxima centered at 662, 620, 653 and 644 nm, respectively, for 1a–d (Fig. 3b) and their fluorescence quantum yields range from 0.13 to 0.25 as summarized in Table S3 (ESI†). The bright solid-state red-emissive properties are similar to those in a recent paper published by Niu and co-workers.15f1a–c exhibit characteristic J-aggregating packing as previously reported.14b,16a Their packing patterns are observed to adopt a coplanar inclined arrangement of the transition dipoles, respectively, with slip angles of 23.2°, 30.7°, and 18.5°, and adjacent interlayer distances of 4.12 Å, 3.38 Å, and 3.88 Å for 1a–c (Fig. S1–S3, ESI†). The head-to-head or head-to-tail dimer and J-aggregating crystal packing might inhibit the free rotation of the meso-2-ketopyrrolyl group, reduce the nonradiative rate of energy loss, and further enhance their solid-state red emissive quantum yields.
To further understand the electronic properties of the meso-2-ketopyrrolyl BODIPYs, the density functional theory (DFT) and time-dependent DFT (TDDFT) calculations were performed using a B3LYP/6-311++G* basis set. As can be deduced from Fig. 4, the HOMO−1 electronic distribution of 1a is mainly localized on the 2-ketopyrrolyl group segment, while the LUMO is delocalized over the BODIPY core, indicating an obvious ICT character. The TDDFT calculated parameter (Table S4, ESI†) of BODIPY 1a shows S0 → S1 with an excitation wavelength of 468 nm, oscillator strength f = 0.2725; and S0 → S2 with an excitation wavelength of 449 nm, oscillator strength f = 0.3296. These two excited states may all contribute to the maximum absorption. The S0 → S1 energy state is fully contributed by HOMO−1 → LUMO and HOMO → LUMO (Table S4, ESI†).
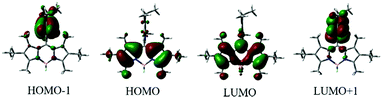 |
| Fig. 4 Molecular frontier orbital plots of meso-2-ketopyrrolyl BODIPY 1a calculated with DFT at the B3LYP/6-31+G(d,p) level. | |
2.4 AIE studies
Encouraged by their photophysical properties as observed above, we studied their AIE behaviour by gradually increasing the water fraction from 0 to 99% in the acetonitrile–water system and measuring the variation of their absorption and fluorescence emission. With an increasing water content of over 80%, their absorption spectra exhibited more broad and dual peaks (Fig. S10a, S11a, S12a and S13a, ESI†), which are characteristic of the J-type packing14b,16a and possibly formed nanoparticles. As shown in Fig. 3d and Fig. S10b (ESI†), 1a exhibits relatively weak emission in pure acetonitrile. However, by increasing the water fraction to 90%, its fluorescence intensity increases instantaneously with the redshifted emission maximum at 591 nm, indicating that 1a is a new AIEgen. Similarly, 1b–d also exhibited excellent AIE-activity in the aforesaid solvent system (Fig. S11b, S12b and S13b, ESI†). It is worth mentioning that a further increase of the water fraction up to 99% for 1b and 1c (Fig. S11b and S12b, ESI†) leads to an increase of the fluorescence intensity, while maintaining the position of their emission maxima. Their red-shifted and enhanced emissions in the aggregated state might be ascribed to their rigidified molecular structures as well as strong dipole–dipole interactions. Their fluorescence activities above revealed that they exhibit notable AIE-activity. The AIE phenomenon16–20 is very unusual for the members of the BODIPY family which normally exhibit a very strong tendency of ACQ. To our best knowledge, only very limited AIE-active BODIPYs have been reported.16–18 Some of them were elegantly designed through conjugation of BODIPY derivatives with AIE-active tetraphenylethene moieties developed by Tang.17
To make clear their aggregation behaviours, 1a–d with a water fraction of 90% in the acetonitrile–water system were, respectively, subjected to scanning electron microscopy (SEM) and transmission electron microscopy (TEM). Very interestingly, different types of nanoaggregates were formed within their solutions. As shown in Fig. 5a and Fig. S14a and b (ESI†), nanoball aggregates of relatively uniform size were created for 1a, 1c and 1d, respectively. In sharp contrast to them, 1b gave cuboid nanoaggregates about 200 nm wide (Fig. 5b). The shape and size of these aggregates are further confirmed by the corresponding TEM plots (Fig. 5c and d and Fig. S14c and d, ESI†), which are in good agreement with SEM. Dynamic light scattering (DLS) measurements of 1a and 1b with the water fraction equal to 99% in the acetonitrile–water system (Fig. S15 and S16, ESI†) revealed the average diameter sizes of 200 and 348 nm and the corresponding PDI values of 0.098 and 0.283, respectively. Among the formed nanoaggregates, the size of the aggregates of 1a is smaller, which may be ascribed to its greater hydrophobicity due to its possession of more alkyl substituents. The obviously morphological distinction between nanoballs for 1a and nanocuboids for 1b indicates that their aggregate morphologies could be tunable. The observed phenomenon was similar to AIE luminogens based on quinoline-BODIPYs developed by Pandey.18a
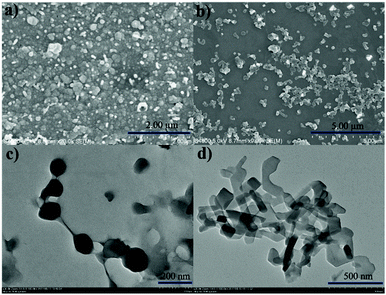 |
| Fig. 5 SEM (a and b) and TEM (c and d) images of nanoballs for 1a (50 μM) and nanocuboids for 1b (30 μM) in the acetonitrile–water system containing a water fraction equal to 90%. The insets show the corresponding magnified images. | |
2.5 Viscosity studies
To decrease the rapid non-radiative decay and restrict their rotations, we tested viscosity (η) sensitivity for these two novel meso-2-ketopyrrolyl BODIPYs as FMRs by increasing the volume ratio of glycerol to methanol. The gradually increased viscosity restricts the motions of the meso-2-ketopyrrolyl groups, decreases their nonradiative rate of energy loss, and naturally enhances their fluorescence for 1a–d (Fig. 6a and Fig. S17–S19, ESI†). The gradual increase of the viscosity from 0.6 to 360 cP brings a continuous enhancement of the fluorescence emission intensity (F) of 1a and 1b at 560 and 530 nm, respectively (Fig. 6a and Fig. S17, ESI†). More importantly, we were fortunate to find that both dyes exhibited quite perfect linear relationships between their log(F/F′) and log
η (Pearson's r = 0.996 for 1a, Fig. 6b; and Pearson's r = 0.990 for 1b, Fig. S20, ESI†). In comparison with that of the fluorescence intensity, the detection of the fluorescence lifetime has more intrinsic advantages for practical applications in imaging, because the fluorescence lifetime is independent of the sample concentration and does not depend on the intensity of the laser.6–8 Thus, the fluorescence decays of these meso-2-ketopyrrolyl BODIPYs 1a and 1b in methanol/glycerol mixtures with different viscosities were studied (Fig. 6c and Fig. S21, ESI†). Similar to that observed in the increase of the fluorescence intensity of 1a, a remarkable increase of the fluorescence lifetime (τ) at 560 nm (from 0.8 ns to 3.9 ns) was observed for 1a with the increase of the viscosity from 0.6 cP to 360 cP in methanol/glycerol solutions. In particular, a greatly perfect linear relationship was also observed between log
τ and log
η with Pearson's r of 0.994 (Fig. 6d), indicating that meso-2-ketopyrrolyl BODIPY 1a we have developed in this contribution could be used to construct calibration curves to quantitatively detect viscosity in vitro. Similar linear correlations of the fluorescence lifetimes (Fig. S22, ESI†) for 1b were also determined as a function of viscosity with Pearson's r of 0.997, respectively, indicating that 1b might also be used as a viscosity mapping rotor for real-time tracking viscosity changes during cell apoptosis events.
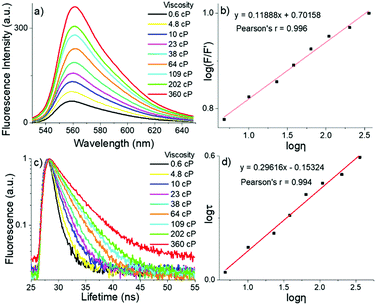 |
| Fig. 6 (a) Fluorescence intensity of 1a (5 μM, excited at 500 nm) with the variation of the viscosity of the system in the methanol–glycerol system. (b) Linear working curve between log(F/F′) and log η of 1a (560 nm). (c) Fluorescence lifetime for 1a with the variation of the viscosity collected at 560 nm. (d) Linear working curve between log τ and log η for 1a. | |
2.6 Cell culture
Before they were used for MTT and cellular uptake, the morphology of 1a (5 μM) was studied in phosphate-buffered saline (PBS, pH = 7.4). In distinct contrast to those at a concentration of 50 μM, no nanoparticles were observed for 5 μM 1a (Fig. S23a, ESI†). As confirmed by energy dispersive X-ray spectrometer (EDS, Fig. S23, ESI†) mapping, 1a was found to be dispersed in PBS. Besides, the solubility of 1a (5 μM) was studied in Dulbecco's modified Eagle's medium (DMEM). As shown in Fig. S24 (ESI†), 1a in DMEM exhibits similar absorbances at different concentrations with the absorption wavelength maximum of 540 nm, and the absorbance complies with the Lambert–Beer law, with lg
εabs of 4.61. The results indicated that aggregates were not formed for 5 μM 1a in DMEM. The results further confirmed that these dyes were not in the aggregated-form when used for cellular uptake, and intracellular micro-viscosity might play a critical role. Membrane permeation is the most likely way for small molecule fluorescent dyes to enter the cells. To make clear if our dyes could localize subcellular organelles, the co-localization experiments were carried out. The co-localization imaging studies (Fig. S25 and S26, ESI†) show that the positions of different subcellular organelles with different viscosities (like cytoplasm, lysosome and the ER) are all distributed for 1a and 1b. It is precisely because these dyes are dispersed in the cytoplasm, so they can be used to detect the overall level of intracytoplasmic viscosity.
Inspired by the excellent viscosity sensitivity above for these novel meso-2-ketopyrrolyl BODIPYs, we then studied their cell imaging. After incubation with 1a and 1b for only 10 minutes (Fig. 7 and Fig. S27, ESI†), their green fluorescence was observed in the cytoplasm of MCF-7. Both 1a and 1b quickly entered cells and exhibited unexpectedly biocompatible capability. MTT assay as a standard cell viability protocol was then used to study their cytotoxic effects on MCF-7 cells (Fig. S28 and S29, ESI†). A high cell viability was observed with a survival rate of above 85% (in 1.0 × 104 cells per well) after treating MCF-7 cells with dye 1a or 1b (0–10 μM) for 24 h. This low cytotoxicity makes these novel dyes applicable for in vitro intracellular imaging.
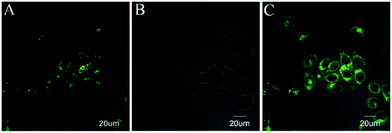 |
| Fig. 7 Imaging of 1a in MCF-7 cells. (A) MCF-7 cells stained with 1a (5 μM, λex = 515 nm, λem = 520–600 nm); (B) DIC image; and (C) merge of A and B. | |
2.7 Viscosity determination in real-time during apoptosis
Considering the perfect correlation of the fluorescence lifetimes of these meso-2-ketopyrrolyl BODIPYs with the viscosity of media, we further investigated the performance of 1a and 1b as FMRs in monitoring the viscosity changes during the pathological processes such as cell apoptosis events. Therefore, etoposide, a clinical antitumor drug that induces cell apoptosis by inhibiting the repair of damaged DNA, was used to stimulate MCF-7 cells. The FLIM image of these cells revealed that the fluorescence lifetime of 1a in cytoplasm changed from 2.45 ns to 3.8 ns during the 60 minutes of etoposide treatment (Fig. 8 and Fig. S30, ESI†). The average viscosity of the cytoplasm around 1a significantly increased from 70 cP to 300 cP and a quite similar linear lifetime–viscosity relationship during cell apoptosis (Fig. 8c) was obtained. The linear correlation of 1b (Fig. S31, ESI†) seems to be slightly lost. This might be ascribed to the relatively weak lipotropism and poor solubility compared to those of 1a.
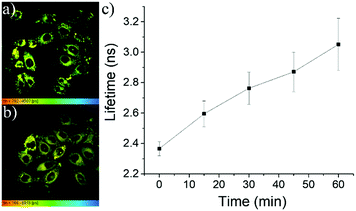 |
| Fig. 8 (a and b) FLIM images of MCF-7 cells incubated with etoposide for 0 and 45 min, respectively. (c) Variation of the fluorescence lifetimes of 1a with the extension of the cell apoptosis time. | |
In combination with FLIM, 1a is a reliable tool for the determination of the local intracellular viscosity. As shown in the FLIM images of MCF-7 cells (Fig. 9a), the fluorescence lifetime of 1a within cells can be clearly mapped from its fluorescence lifetime distribution histogram. For example, the average fluorescence lifetime was established to be 2.4 ns for 1a (Fig. 9b). This indicates that inside cytoplasm, the average viscosity around 1a is about 60 cP as shown in the calibration graph (Fig. 6d). The high spatial resolution of these FLIM images was found to be compatible with their corresponding confocal images. This makes it feasible to indicate the local viscosities in different areas of cytoplasm based on the determination of their fluorescence lifetimes. For example, within different randomly chosen intracellular regions, the precision of the resultant fluorescence decay curves is almost identical to those obtained in the solution state. As shown in Fig. 9c and d, the different regions (Fig. 9a) of cells have different lifetimes of 2.25 ns and 2.11 ns, with respect to 50.9 and 41.0 cP, respectively, indicating that the change in intracellular viscosity could be facilely detected by our meso-2-ketopyrrolyl derived BODIPY 1a.
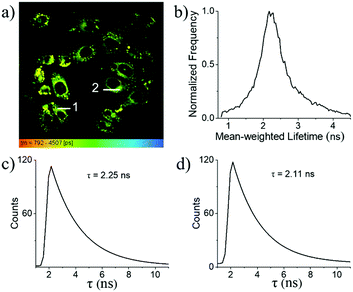 |
| Fig. 9 (a) Intracellular FLIM of 1a (MCF-7 cells, the mean-weighted lifetime range is 0.80–4.50 ns). (b) Histogram showing the fluorescence lifetime distribution (x axis: the lifetime and y axis: normalized fequency of the black cross point). (c) and (d) Fluorescence lifetimes of regions 1 (c) and 2 (d) marked in (a). | |
3. Conclusions
In summary, we have first synthesized a novel family of unconventional meso-2-ketopyrrolyl BODIPYs via the efficient condensation reactions between oxalyl chloride and substituted pyrrolic derivatives. The meso-2-ketopyrrolyl BODIPYs exhibited high red fluorescence quantum yields of up to 25% from 620 to 661 nm, and notable AIE-active and viscosity-sensitive characteristics. The formed nanoaggregates were observed to exhibit morphological distinctions with average diameters owing to different substituents. In addition, the restriction of the rotation of the meso-ketopyrrolyl group in viscous media resulted in large fluorescence enhancement and lengthened the fluorescence lifetime from 0.8 to 3.9 ns, which are in good correlation with the variation of the viscosity in the media. More importantly, as novel mapping viscosity BODIPY indicators, they show excellent applicability for real-time tracking viscosity changes during cell apoptosis events, and for monitoring normal and abnormal cell viscosities and for further quantifying the local viscosity within the living cell. This easy strategy and a perfect linear relationship between the fluorescence lifetime and viscosity might indicate that the developed meso-2-ketopyrrolyl derived BODIPYs here are suitable viscosimeters as designed.
4. Experimental
General methods
Reagents and solvents were used as received from commercial suppliers unless noted otherwise. All reactions were performed in oven-dried or flame-dried glassware, and monitored by TLC using 0.25 mm silica gel plates with a UV indicator. 1H and 13C NMR were recorded on a 300 MHz NMR spectrometer at room temperature. Chemical shifts (δ) are given relative to CDCl3 (7.26 ppm for 1H and 77 ppm for 13C) or internal tetramethylsilane (abbreviated as TMS). High-resolution mass spectra (HRMS) were obtained using APCI-TOF in a positive mode.
Syntheses of meso-2-ketopyrrolyl BODIPYs 1a and b
Synthesis of 1a.
3-Ethyl-2,4-methylpyrrole (0.95 mL, 7 mmol) was dissolved into 60 mL freshly distilled 1,2-dichloroethane under argon. To this solution was dropwise added oxalyl chloride (0.17 mL, 2 mmol) in 1,2-dichloroethane (10 mL) via a pressure-equalizing dropping funnel under ice-bath conditions. By increasing the reaction temperature to 35 °C, the reaction mixture was then allowed to stir at this temperature for 3 h. To this reaction mixture was added Et3N (2 mL) under ice-bath conditions. The mixture was further stirred for 10 minutes before subsequent addition of BF3·OEt2 (3 mL) at this temperature via a syringe. The reaction mixture was left stirring overnight under refluxing conditions. The reaction mixture was cooled down to room temperature, poured into water (150 mL) and extracted with dichloromethane (3 × 40 mL). Organic layers were combined, and subsequently washed with water. The resultant organic layers were dried over anhydrous sodium sulfate and filtered, and the solvent was evaporated under vacuum. The crude product was then purified by silica gel chromatography (petroleum/ethyl acetate = 4/1, v/v). Target 1a was obtained as a reddish powder in 18% yield (163 mg). 1H NMR (300 MHz, CDCl3) δ: 9.49 (brs, 1H; NH), 2.52 (s, 6H; CH3), 2.36–2.28 (m, 9H; CH2, CH3), 1.86 (s, 9H; CH3), 1.00 (t, J = 7.2 Hz, 9H; CH3). 13C NMR (75 MHz, CDCl3) δ: 179.2, 154.8, 136.9, 136.7, 136.3, 132.7, 130.5, 128.4, 128.2, 126.1, 17.1, 15.2, 14.7, 12.7, 11.9, 11.1, 11.0, 10.6. HRMS (APCI): m/z = 454.2831 [M + H]+.
Synthesis of 1b.
As described in the procedure for 1a above, BODIPY 1b was prepared as a reddish powder in 23% yield (169 mg) from 2,4-methylpyrrole (0.73 mL, 7 mmol) and oxalyl chloride (0.17 mL, 2 mmol). 1H NMR (300 MHz, CDCl3) δ: 9.39 (brs, 1H; NH), 6.01 (s, 2H), 5.84 (s, 1H), 2.54 (s, 6H; CH3), 2.32 (s, 3H; CH3), 1.95 (s, 6H; CH3), 1.93 (s, 3H; CH3). 13C NMR (75 MHz, CDCl3) δ: 178.9, 156.8, 141.6, 138.7, 137.8, 133.3, 129.1, 128.7, 121.0, 113.8, 14.8, 13.6, 13.4, 13.0. HRMS (APCI): m/z = 370.1907 [M + H]+.
General synthetic procedure for 1a–d
To a dried flask containing dipyrrolediketone 2 (1 mmol) in dichloromethane (60 mL) was added 0.2 mL BF3·OEt2. The solution turned brown quickly. Then 2,4-dimethylpyrrole or 2,4-dimethyl-3-ethylpyrrole (1.2 mmol, 1.2 equivalents) in 10 mL dichloromethane was dropwise added slowly. The mixture was stirred for 3 hours at room temperature (35 °C). When dipyrrolediketone 2 was consumed completely, 3 mL of Et3N was added and the mixture was stirred for 10 minutes before subsequent addition of BF3·OEt2 (3.5 mL) through a syringe. The reaction mixture was left stirring for 30 min. Then the reaction mixture was extracted with EtOAc (30 mL × 3). The organic layers were combined, dried over anhydrous Na2SO4, filtered, and evaporated to dryness under vacuum. The crude product was purified by column chromatography on silica gel (hexane/EtOAc = 4/1, v/v) and was further recrystallized from hexane to give 1a–d as reddish powders.
1a
.
It was prepared using the above procedure from 2a (240 mg, 0.8 mmol) and 2,4-dimethyl-3-ethylpyrrole (1.2 eq.), affording a reddish powder in 45% yield (164 mg). 1H NMR (300 MHz, CDCl3) δ: 9.49 (brs, 1H; NH), 2.52 (s, 6H; CH3), 2.36–2.28 (m, 9H; CH2, CH3), 1.86 (s, 9H; CH3), 1.00 (t, J = 7.2 Hz, 9H; CH3). 13C NMR (75 MHz, CDCl3) δ: 179.2, 154.8, 136.9, 136.7, 136.3, 132.7, 130.5, 128.4, 128.2, 126.1, 17.1, 15.2, 14.7, 12.7, 11.9, 11.1, 11.0, 10.6. HRMS (APCI): m/z = 454.2831 [M + H]+.
1b
.
It was prepared using the above procedure from 2b (195 mg, 0.8 mmol) and 2,4-dimethylpyrrole (1.2 eq.), affording a reddish powder in 32% yield (94 mg). 1H NMR (300 MHz, CDCl3) δ: 9.39 (brs, 1H; NH), 6.01 (s, 2H), 5.84 (s, 1H), 2.54 (s, 6H; CH3), 2.32 (s, 3H; CH3), 1.95 (s, 6H; CH3), 1.93 (s, 3H; CH3). 13C NMR (75 MHz, CDCl3) δ: 178.9, 156.8, 141.6, 138.7, 137.8, 133.3, 129.1, 128.7, 121.0, 113.8, 14.8, 13.6, 13.4, 13.0. HRMS (APCI): m/z = 370.1907 [M + H]+.
1c
.
It was prepared using the above procedure from 2a (240 mg, 0.8 mmol) and 2,4-dimethylpyrrole (1.2 eq.), affording a reddish powder in 45% yield (153 mg). 1H NMR (300 MHz, CDCl3) δ: 9.36 (s, 1H), 5.96 (s, 1H), 2.53 (s, 6H), 2.32–2.35 (m, 4H), 2.28 (s, 3H), 1.92 (s, 3H), 1.88 (s, 3H), 1.88 (s, 3H), 0.98–1.03 (m, 6H). 13C NMR (75 MHz, CDCl3) δ: 178.6, 156.7, 154.9, 140.4, 137.9, 137.5, 136.9, 133.5, 130.6, 129.0, 128.6, 128.0, 126.2, 120.2, 17.1, 16.9, 15.2, 14.6, 13.6, 12.9, 11.9, 11.1, 10.6. HRMS (APCI): m/z = 426.2522 [M + H]+.
1d
.
It was prepared using the above procedure from 2b (195 mg, 0.8 mmol) and 2,4-dimethyl-3-ethylpyrrole (1.2 eq.), affording a reddish powder in 41% yield (130 mg). 1H NMR (300 MHz, CDCl3) δ: 9.76 (s, 1H), 5.96 (s, 1H), 5.84 (s, 1H), 2.53 (s, 6H), 2.32 (s, 5H), 1.93 (s, 6H), 1.89 (s, 3H), 1.00 (s, 3H). 13C NMR (75 MHz, CDCl3) δ: 179.2, 156.8, 155.0, 140.3, 139.7, 137.7, 137.1, 133.7, 133.6, 129.0, 128.8, 120.3, 113.8, 113.6, 17.1, 14.6, 13.5, 13.4, 13.2, 12.9, 11.0. HRMS (APCI): m/z = 398.2208 [M + H]+.
Photophysical characterization
UV-visible absorption and fluorescence emission spectra were recorded on commercial spectrophotometers (190–870 nm scan range, Shimadzu UV-2450 and Hitachi F-4500) at room temperature (1 cm quartz cuvette). The absolute fluorescence quantum efficiencies in different organic solvents (excited at 500 nm for 1a, excited at 480 nm for 1b–d) and the powder state (excited at 550 nm for 1a and 500 nm for 1b–d) of the meso-2-ketopyrrolyl derived BODIPYs 1a–d were obtained using an Edinburgh FLS 920 fluorescence spectrometer by comparing the areas under the corrected emission spectrum of the test sample using an integrating sphere according to the definition of fluorescence efficiency,21 using eqn (1) given below: | 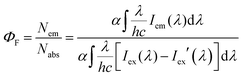 | (1) |
where Nem and Nabs are the numbers of emitted and absorbed photons, respectively, α is the calibration factor for the measurement setup, λ is the wavelength, h is Planck's constant, c is the speed of light, Iem(λ) is the emission intensity at λ, and Iex(λ) and Iex′(λ) are the intensities of the excitation laser beam with λ in the absence and presence of the sample, respectively. The measured ΦF value is independent of the shape and thickness of the sample and the power of the excitation laser.
The fluorescence lifetime (τ) was measured by a time-correlated single photon counting method using an Edinburgh FLS 980 fluorescence spectrometer and emission was monitored at the peak maximum (excited at 470 nm). The fluorescence lifetime values were obtained from deconvolution and distribution lifetime analysis. The solvents were obtained by mixing methanol and glycerol in different proportions. The viscosity values were referenced to previously reported articles.10
Crystals suitable for X-ray crystallographic analysis
CCDC 1541729 (1a), CCDC 1541728 (1b) and CCDC 1901461 (1c)† contain the supplementary crystallographic data for this paper. Diffraction data were collected using a Bruker APEXII CCD area detector diffractometer using graphite-monochromated Mo-Kα radiation (λ = 0.71073 Å) at 293(2) K, with ϕ and ω scan techniques. An empirical absorption correction was applied with the SADABS program. Using Olex222a or SHELXL-97,22b the structure was solved with the ShelXT23 structure solution program using direct methods and refined with the ShelXL24 refinement package using least squares minimisation. The hydrogen atom coordinates were calculated with SHELXL by using an appropriate riding model with varied thermal parameters. The residual electron densities were of no chemical significance.
DFT computations
The ground state geometry was optimized by using the DFT method at the B3LYP/6-31+G(d,p) level. The same method was used for vibrational analysis to verify that the optimized structures correspond to local minima on the energy surface. TD-DFT computations were used to obtain the vertical excitation energies and oscillator strengths for the optimized ground state equilibrium geometries at the B3LYP/6-31+G(d, p) theoretical level. TD-DFT calculations of 1a in dichloromethane were done using the Self-Consistent Reaction Field method and Polarizable Continuum Model.
Cell culture
MCF-7 cells were obtained from the Institute of Basic Medical Sciences (IBMS) of the Chinese Academy of Medical Sciences (CAMS). All cell lines were maintained under standard culture conditions (an atmosphere of 5% CO2 and 95% air at 37 °C) in DMEM, supplemented with 10% FBS (fetal bovine serum). The cells were used in the exponential phase of growth on 35 mm glass bottom culture dishes for 24 h. The cells were treated with 5 μM of 1a and 1b in culture media for 10 min at 37 °C with 5% CO2 in a humidified incubator. Fluorescence imaging was then carried out after washing the cells with PBS. For the control experiment, the cells without treatment of dyes did not show any noticeable fluorescence under the same conditions.
Cytotoxicity study
MCF-7 cells (106 cells mL−1) were dispersed within replicate 96-well microtiter plates to a total volume of 200 μL well−1. Plates were maintained at 37 °C in a 5% CO2 and 95% air incubator for 4–6 h. Then MCF-7 cells were incubated for 24 h with different concentrations of 1a and 1b. MTT solution was then added to each well. After 4 h, the remaining MTT solution was removed, and 150 μL of DMSO was added to each well to dissolve formazan crystals. Absorbance was measured at 490 nm in a Triturus microplate reader.
Viscosity determination in real-time during apoptosis
The fluorescence lifetimes of 1a under different conditions were obtained with an excitation wavelength of 515 nm and collected at 520–600 nm. The fluorescence lifetimes of 1b under different conditions were obtained with an excitation wavelength of 488 nm and collected at 510–600 nm. MCF-7 cells internalized with 1a and 1b were washed with PBS and then etoposide was added.11 Fluorescence images were obtained at different time points: 0, 5, 15, 30, 45, and 60 min.
Conflicts of interest
There are no conflicts to declare.
Acknowledgements
This work was supported by the National Natural Science Foundation of China (Grant No. 21672006, 21672007, 21402001 and 21871006), Hong Kong Polytechnic University (1-YW2T and 1-ZE1C), the Hong Kong Research Grants Council (C6009-17G), the Open Funds of Nanjing University State Key Laboratory of Coordination Chemistry (SKLCC1911), and the Doctoral up Starting Foundation of Anhui Normal University (2017XJJ28).
References
-
(a) S. J. Singer and G. L. Nicolson, Science, 1972, 175, 720–731 CrossRef CAS;
(b) S. Chen, Y. Hong, Y. Zeng, Q. Sun, Y. Liu, E. Zhao, G. Bai, J. Qu, J. Hao and B. Z. Tang, Chem. – Eur. J., 2015, 21, 4315–4320 CrossRef CAS;
(c) P. Ning, P. Dong, Q. Geng, L. Bai, X. Tian, R. Shao, L. Li and X. Meng, J. Mater. Chem. B, 2017, 5, 2743–2749 RSC;
(d) Y. He, J. Shin, W. Gong, P. Das, J. Qu, Z. Yang, W. Liu, C. Kang, J. Qu and J. S. Kim, Chem. Commun., 2019, 55, 2453–2456 RSC.
-
(a) M. K. Kuimova, S. W. Botchway, A. W. Parker, M. Balaz, H. A. Collins, H. L. Anderson, K. Suhling and P. R. Ogilby, Nat. Chem., 2009, 1, 69–73 CrossRef CAS PubMed;
(b) M. K. Kuimova, Phys. Chem. Chem. Phys., 2012, 14, 12671–12686 RSC;
(c) X. Qian and Z. Xu, Chem. Soc. Rev., 2015, 44, 4487–4493 RSC;
(d) F. Liu, T. Wu, J. Cao, S. Cui, Z. Yang, X. Qiang, S. Sun, F. Song, J. Fan, J. Wang and X. Peng, Chem. – Eur. J., 2013, 19, 1548–1553 CrossRef CAS;
(e) H. Doan, S. L. Raut, D. Yale, M. Balaz, S. V. Dzyuba and Z. Gryczynski, Chem. Commun., 2016, 52, 9510–9513 RSC;
(f) W. Chen, C. Gao, X. Liu, F. Liu, F. Wang, L. Tang and J.-H. Jiang, Anal. Chem., 2018, 90, 8736–8741 CrossRef CAS.
-
(a) M. R. Dent, I. Lopez-Duarte, C. J. Dickson, P. Chairatana, H. L. Anderson, I. R. Gould, D. Wylie, A. Vyšniauskas, N. J. Brooks and M. K. Kuimova, Chem. Commun., 2016, 52, 13269–13272 RSC;
(b) S.-C. Lee, J. Heo, J.-W. Ryu, C.-L. Lee, S. Kim, J.-S. Tae, B.-O. Rhee, S.-W. Kim and O.-P. Kwon, Chem. Commun., 2016, 52, 13695–13698 RSC;
(c) T. Luo, Y. Lu, S. Liu, D. Lin and J. Qu, Anal. Chem., 2017, 89, 8104–8111 CrossRef CAS;
(d) M. Ren, B. Deng, K. Zhou, X. Kong, J.-Y. Wang and W. Lin, Anal. Chem., 2017, 89, 552–555 CrossRef CAS;
(e) L. Hou, P. Ning, Y. Feng, Y. Ding, L. Bai, L. Li, H. Yu and X. Meng, Anal. Chem., 2018, 90, 7122–7126 CrossRef CAS.
-
(a) G. S. Zubenko, U. Kopp, T. Seto and L. L. Firestone, Psychopharmacology, 1999, 145, 175–180 CrossRef CAS;
(b) H. Tan, Y. Qiu, H. Sun, J. Yan and L. Zhang, Chem. Commun., 2019, 55, 2688–2691 RSC.
-
(a) A. Loudet and K. Burgess, Chem. Rev., 2007, 107, 4891–4932 CrossRef CAS;
(b) G. Ulrich, R. Ziessel and A. Harriman, Angew. Chem., Int. Ed., 2008, 47, 1184–1201 CrossRef CAS PubMed;
(c) N. Boens, V. Leen and W. Dehaen, Chem. Soc. Rev., 2012, 41, 1130–1172 RSC;
(d) H. Lu, J. Mack, Y. Yang and Z. Shen, Chem. Soc. Rev., 2014, 43, 4778–4823 RSC;
(e) Y. Ni and J. Wu, Org. Biomol. Chem., 2014, 12, 3774–3791 RSC;
(f) N. Boens, B. Verbelen and W. Dehaen, Eur. J. Org. Chem., 2015, 6577–6595 CrossRef CAS.
-
(a) M. K. Kuimova, G. Yahioglu, J. A. Levitt and K. Suhling, J. Am. Chem. Soc., 2008, 130, 6672–6673 CrossRef CAS PubMed;
(b) N. A. Hosny, G. Mohamedi, P. Rademeyer, J. Owen, Y. L. Wu, M. X. Tang, R. J. Eckersley, E. Stride and M. K. Kuimova, Proc. Natl. Acad. Sci. U. S. A., 2013, 110, 9225–9230 CrossRef CAS;
(c) I. López-Duarte, T. T. Vu, M. A. Izquierdo, J. A. Bull and M. K. Kuimova, Chem. Commun., 2014, 50, 5282–5284 RSC;
(d) A. Vyšniauskas, M. Qurashi, N. Gallop, M. Balaz, H. L. Andersonb and M. K. Kuimova, Chem. Sci., 2015, 6, 5773–5778 RSC;
(e) T. T. Vu, R. Méallet-Renaul, G. Clavier, B. A. Trofimov and M. K. Kuimova, J. Mater. Chem. C, 2016, 4, 2828–2833 RSC;
(f) P. S. Sherin, I. López-Duarte, M. R. Dent, M. Kubánková, A. Vyšniauskas, J. A. Bull, E. S. Reshetnikova, A. S. Klymchenko, Y. P. Tsentalovich and M. K. Kuimova, Chem. Sci., 2017, 8, 3523–3528 RSC;
(g) J. E. Chambers, M. Kubánková, R. G. Huber, I. López-Duarte, E. Avezov, P. J. Bond, S. J. Marciniak and M. K. Kuimova, ACS Nano, 2018, 12, 4398–4407 CrossRef CAS.
-
(a) M. A. H. Alamiry, A. C. Benniston, G. Copley, K. J. Elliott, A. Harriman, B. Stewart and Y.-G. Zhi, Chem. Mater., 2008, 20, 4024–4032 CrossRef CAS;
(b) S. Raut, J. Kimball, R. Fudala, H. Doan, B. Maliwal, N. Sabnis, A. Lacko, I. Gryczynski, S. V. Dzyuba and Z. Gryczynski, Phys. Chem. Chem. Phys., 2014, 16, 27037–27042 RSC;
(c) S. L. Raut, J. D. Kimball, R. Fudala, I. Bora, R. Chib, H. Jaafari, M. K. Castillo, N. W. Smith, I. Gryczynski, S. V. Dzyuba and Z. Gryczynski, Phys. Chem. Chem. Phys., 2016, 18, 4535–4540 RSC;
(d) L.-L. Li, K. Li, M.-Y. Li, L. Shi, Y.-H. Liu, H. Zhang, S.-L. Pan, N. Wang, Q. Zhou and X.-Q. Yu, Anal. Chem., 2018, 90, 5873–5878 CrossRef CAS PubMed.
-
(a) Z. Yang, Y. He, J.-H. Lee, N. Park, M. Suh, W.-S. Chae, J. Cao, X. Peng, H. Jung, C. Kang and J. S. Kim, J. Am. Chem. Soc., 2013, 135, 9181–9185 CrossRef CAS;
(b) Z. Yang, Y. He, J. H. Lee, W.-S. Chae, W. X. Ren, J. H. Lee, C. Kang and J. S. Kim, Chem. Commun., 2014, 50, 11672–11675 RSC;
(c) H. Lee, Z. Yang, Y. Wi, T. W. Kim, P. Verwilst, Y. H. Lee, G. Han, C. Kang and J. S. Kim, Bioconjugate Chem., 2015, 26, 2474–2480 CrossRef CAS;
(d) D. Dziuba, P. Jurkiewicz, M. Cebecauer, M. Hof and M. Hocek, Angew. Chem., Int. Ed., 2016, 55, 174–178 CrossRef CAS;
(e) W. Zhang, W. Sheng, C. Yu, Y. Wei, H. Wang, E. Hao and L. Jiao, Chem. Commun., 2017, 53, 5318–5321 RSC.
-
(a) H. L. Kee, C. Kirmaier, L. Yu, P. Thamyongkit, W. J. Youngblood, M. E. Calder, L. Ramos, B. C. Noll, D. F. Bocian, W. R. Scheidt, R. R. Birge, J. S. Lindsey and D. Holten, J. Phys. Chem. B, 2005, 109, 20433–20443 CrossRef CAS;
(b) E. Bahaidarah, A. Harriman, P. Stachelek, S. Rihn, E. Heyer and R. Ziessel, Photochem. Photobiol. Sci., 2014, 13, 1397–1401 RSC.
- L. Wang, Y. Xiao, W. Tian and L. Deng, J. Am. Chem. Soc., 2013, 135, 2903–2906 CrossRef CAS.
- H. Zhu, J. Fan, M. Li, J. Cao, J. Wang and X. Peng, Chem. – Eur. J., 2014, 20, 4691–4696 CrossRef CAS.
- R. Guliyev, S. Ozturk, E. Sahin and E. U. Akkaya, Org. Lett., 2012, 14, 1528–1531 CrossRef CAS.
- C. Yu, E. Hao, T. Li, J. Wang, W. Sheng, Y. Wei, X. Mu and L. Jiao, Dalton Trans., 2015, 44, 13897–13905 RSC.
-
(a) Y. Ni, L. Zeng, N.-Y. Kang, K.-W. Huang, L. Wang, Z. Zeng, Y.-T. Chang and J. Wu, Chem. – Eur. J., 2014, 20, 2301–2310 CrossRef CAS;
(b) S. Kim, J. Bouffard and Y. Kim, Chem. – Eur. J., 2015, 21, 17459–17465 CrossRef CAS;
(c) Y. Ni, R. K. Kannadorai, S. W.-K. Yu, Y.-T. Chang and J. Wu, Org. Biomol. Chem., 2017, 15, 4531–4535 RSC;
(d) T.-I. Kim, H. Kim, Y. Choi and Y. Kim, Sens. Actuators, B, 2017, 249, 229–234 CrossRef CAS;
(e) T.-I. Kim, B. Hwang, B. Lee, J. Bae and Y. Kim, J. Am. Chem. Soc., 2018, 140, 11771–11776 CrossRef CAS;
(f) J. A. Peterson, C. Wijesooriya, E. J. Gehrmann, K. M. Mahoney, P. P. Goswami, T. R. Albright, A. Syed, A. S. Dutton, E. A. Smith and A. H. Winter, J. Am. Chem. Soc., 2018, 140, 7343–7346 CrossRef CAS.
-
(a) N. Zhao, M. G. Vicente, F. R. Fronczek and K. M. Smith, Chem. – Eur. J., 2015, 21, 6181–6192 CrossRef CAS;
(b) N. Zhao, S. Xuan, Z. Zhou, F. R. Fronczek, K. M. Smith and M. G. H. Vicente, J. Org. Chem., 2017, 82, 9744–9750 CrossRef CAS;
(c) N. Zhao, T. M. Williams, Z. Zhou, F. R. Fronczek, M. Sibrian-Vazquez, S. D. Jois and M. G. H. Vicente, Bioconjugate Chem., 2017, 28, 1566–1579 CrossRef CAS;
(d) G. Zhang, N. Zhao, P. Bobadova-Parvanova, M. Wang, F. R. Fronczek, K. M. Smith and M. G. H. Vicente, J. Phys. Chem. A, 2018, 122, 6256–6265 CrossRef CAS;
(e) D. J. LaMaster, N. E. M. Kaufman, A. S. Bruner and M. G. H. Vicente, J. Phys. Chem. A, 2018, 122, 6372–6380 CrossRef CAS;
(f) C. Duan, Y. Zhou, G.-G. Shan, Y. Chen, W. Zhao, D. Yuan, L. Zeng, X. Huang and G. Niu, J. Mater. Chem. C, 2019, 7, 3471–3478 RSC.
-
(a) S. Choi, J. Bouffard and Y. Kim, Chem. Sci., 2014, 5, 751–755 RSC;
(b) H. Chua, Y. Ni, M. Garai, B. Zheng, K.-W. Huang, Q.-H. Xu, J. Xu and J. Wu, Chem. – Asian J., 2015, 10, 1631–1634 CrossRef.
-
(a) R. Hu, C. F. A. M. Gómez-Durán, J. W. Y. Lam, J. L. Belmonte-Vázquez, C. Deng, S. Chen, R. Ye, E. Peña-Cabrera, Y. Zhong, K. S. Wong and B. Z. Tang, Chem. Commun., 2012, 48, 10099–10101 RSC;
(b) Z. Zhao, B. Chen, J. Geng, Z. Chang, L. Aparicio-Ixta, H. Nie, C. C. Goh, L. G. Ng, A. Qin, G. Ramos-Ortiz, B. Liu and B. Z. Tang, Part. Part. Syst. Charact., 2014, 31, 481–491 CrossRef CAS;
(c) C. F. A. Gómez-Durán, R. Hu, G. Feng, T. Li, F. Bu, M. Arseneault, B. Liu, E. Peña-Cabrera and B. Z. Tang, ACS Appl. Mater. Interfaces, 2015, 7, 15168–15176 CrossRef;
(d) H.-T. Feng, X. Gu, J. W. Y. Lam, Y.-S. Zheng and B. Z. Tang, J. Mater. Chem. C, 2018, 6, 8934–8940 RSC.
-
(a) R. S. Singh, R. K. Gupta, R. P. Paitandi, M. Dubey, G. Sharma, B. Koch and D. S. Pandey, Chem. Commun., 2015, 51, 9125–9128 RSC;
(b) H. T. Feng, J.-B. Xiong, Y.-S. Zheng, B. Pan, C. Zhang, L. Wang and Y. Xie, Chem. Mater., 2015, 27, 7812–7819 CrossRef CAS;
(c) H. Manzano, I. Esnal, T. Marqués-Matesanz, J. Bañuelos, I. López-Arbeloa, M. J. Ortiz, L. Cerdán, A. Costela, I. García-Moreno and J. L. Chiara, Adv. Funct. Mater., 2016, 26, 2756–2769 CrossRef CAS;
(d) E. Sen, K. Meral and S. Atilgan, Chem. – Eur. J., 2016, 22, 736–745 CrossRef CAS PubMed.
-
(a) J. Mei, Y. Hong, J. W. Y. Lam, A. Qin, Y. Tang and B. Z. Tang, Adv. Mater., 2014, 26, 5429–5479 CrossRef CAS;
(b) J. Mei, N. L. Leung, R. T. Lam, J. W. Kwok and B. Z. Tang, Chem. Rev., 2015, 115, 11718–11940 CrossRef CAS;
(c) M. Chen, X. Hu, J. Liu, B. Li, N. L. C. Leung, L. Viglianti, T. S. Cheung, H. H. Y. Sung, R. T. K. Kwok, I. D. Williams, A. Qin, J. W. Y. Lam and B. Z. Tang, Chem. Sci., 2018, 9, 7829–7834 RSC;
(d) D. Wang, H. Su, R. T. K. Kwok, X. Hu, H. Zou, Q. Luo, M. M. S. Lee, W. Xu, J. W. Y. Lam and B. Z. Tang, Chem. Sci., 2018, 9, 3685–3693 RSC.
-
(a) S. Gan, J. Zhou, T. A. Smith, H. Su, W. Luo, Y. Hong, Z. Zhao and B. Z. Tang, Mater. Chem. Front., 2017, 1, 2554–2558 RSC;
(b) M. Yamaguchi, S. Ito, A. Hirose, K. Tanaka and Y. Chujo, Mater. Chem. Front., 2017, 1, 1573–1579 RSC;
(c) J. S. Ni, H. Liu, M. Jiang, Z. Zhao, Y. Chen, R. T. K. Kwok, J. W. Y. Lam, Q. Peng and B. Z. Tang, Mater. Chem. Front., 2018, 2, 1498–1507 RSC;
(d) F. Ren, P. Liu, Y. Gao, J. Shi, B. Tong, Z. Cai and Y. Dong, Mater. Chem. Front., 2019, 3, 57–63 RSC.
- Y. Kawamura, H. Sasabe and C. Adachi, Jpn. J. Appl. Phys., 2004, 43, 7729–7730 CrossRef CAS.
-
(a) O. V. Dolomanov, L. J. Bourhis, R. J. Gildea, J. A. K. Howard and H. Puschmann, J. Appl. Crystallogr., 2009, 42, 339–341 CrossRef CAS;
(b)
G. M. Sheldrick, SHELXTL 5.10 for Windows NT: Structure Determination Software Programs, Bruker Analytical X-ray Systems, Inc., Madison, WI, 1997 Search PubMed.
- G. M. Sheldrick, Acta Crystallogr., Sect. A: Found. Adv., 2015, 71, 3–8 CrossRef.
- G. M. Sheldrick, Acta Crystallogr., Sect. A: Found. Crystallogr., 2008, 64, 112–122 CrossRef CAS.
Footnotes |
† Electronic supplementary information (ESI) available: Fig. S1–S31 and Tables S1–S4. CCDC 1541729, 1541728 and 1901461 for 1a–c. For ESI and crystallographic data in CIF or other electronic format see DOI: 10.1039/c9qm00154a |
‡ C. Y. and Z. H. contributed equally. |
|
This journal is © the Partner Organisations 2019 |