DOI:
10.1039/D4SU00099D
(Critical Review)
RSC Sustain., 2024, Advance Article
A comprehensive pre-treatment strategy evaluation of ligno-hemicellulosic biomass to enhance biogas potential in the anaerobic digestion process
Received
28th February 2024
, Accepted 24th July 2024
First published on 9th August 2024
Abstract
Effective pretreatment of ligno-hemicellulosic biomass has emerged as a pre-requisite for its efficient conversion into biogas through the anaerobic digestion (AD) process. Assessment of various pre-treatment methods shows microbial pretreatment to be the most promising, economically viable, and environment-friendly option. Microbial pretreatment offers the advantages of low energy consumption and minimal pollution generation, thus making it a promising avenue for enhancing biogas yields from biomass. Fungi and bacteria, along with their enzymes, play pivotal roles in this method. Fungal pretreatment, involving cellulose and lignin-degrading species like brown-rot and white-rot fungi, have shown improved biogas yield. Bacterial and enzymatic pretreatments offer quicker results, making them attractive options for shortening the reaction time. Microbial consortia have shown remarkable efficiency in biomass degradation and its anaerobic digestion under thermophilic conditions. Physical pretreatment methods, such as mechanical size reduction, have shown potential to increase biomass accessibility and enhance biogas production. However, due to its energy-intensive nature and for improving biogas yields, further research is needed to develop more cost-effective approaches. The combination of physical and biological pretreatment methods offers a promising approach to effectively pretreat ligno-hemicellulosic biomass for improved biogas production.
| Rajesh Kumar Prasad Rajesh Kumar Prasad is currently working on experimental and technical points of R&D programs related to bioenergy, pre-treatment technologies related to biomass, STP, and ETP plants. |
| Anjali Sharma Anjali Sharma’s interests lie in waste to energy solutions, green energy and the development of a circular economy. |
| Pranab Behari Mazumder Prof. Mazumder has immense and vast expertise in genetics, molecular mechanism of induced systemic tolerance in plants, PGPR, profiling of stress resistance gene expression in plants and bacteria and plant biotechnology, and has also worked on the conversion of biomass to biofuels. |
| Anil Dhussa Anil Dhussa has over 40 years of ground breaking work in biogas and waste to energy domains including national programmes, policies, research and development and execution of pioneering projects. |
Sustainability spotlight
This review article focuses on the potential transition of lignocellulosic biomass into treasured sustainable & clean energy, i.e. biogas. First, it uncovers various relevant feedstock possibilities for the production of biogas. Secondly, it provides in-depth knowledge and recent developments on different pretreatment approaches for enhanced biogas yield. Furthermore, it delivers an insight into effective and sustainable pretreatment methods. The sustainability spotlight focuses on the UN Sustainable Development Goals (SDGs), including SDG 12: responsible consumption and production, SDG 9: industry, innovation, and infrastructure, and SDG 7: affordable and clean energy. It also highlights waste-to-energy initiatives and sustainable and clean energy ideologies. The advent of pretreatment strategies for the production of sustainable and clean energy is the emphasis herein.
|
1. Introduction
The renewable energy imperative and opportunities are growing as fossil fuels deplete and climate change accelerates. Biomass-based energy offers a crucial solution, utilizing organic wastes and residues to produce sustainable power, reduce greenhouse gas emissions, and mitigate our dependence on finite resources. According to the famous quote of Lao Tzu (the father of Taoism), “If you don't change direction, you will end up where you are heading,” Where are we heading? Daily human activities and the advancement of industry have led to an astonishing amount of waste production. One striking illustration of this is the staggering quantity of municipal organic waste generated (∼2.2 billion tons by the year 2025) as forecasted by the World bank.1 When raw materials and treatment technologies and ultimately production technologies are carefully chosen, biomass energy can be generated in a financially sustainable manner. Furthermore, by effectively managing carbon emissions and ensuring economic efficiency, biomass energy supply chains can be established as sustainable solutions. At present, one such technology that has already proven itself is anaerobic digestion (AD) technology for biomass utilization to produce biomethane, looking promising even for bio-hydrogen.2,3 Anaerobic digestion is a widely adopted technology for conversion of organic waste into biogas, a renewable energy source comprising mainly methane and carbon dioxide. AD breaks down organic matter into biogas and a stabilized organic effluent through metabolic reactions involving a complex community of microorganisms (both facultative and strict anaerobes). These reactions occur under non-toxic conditions as highlighted by various researchers.4–8 As a first step, complex organic substances that cannot be directly utilized by bacteria are broken down into soluble monomers through the action of extracellular hydrolytic enzymes produced by acidogens. This process, known as acidification or fermentation, serves as an intermediate step in substrate metabolism, acting as an electron acceptor. During this stage, acidogenic fermentation bacteria convert the soluble monomers into terminal products, such as volatile fatty acids (VFAs), along with the generation of cellular materials. In the third step, hydrogen-producing acetogens utilize the hydrolytic products to produce acetic acid while generating hydrogen and carbohydrates. A small population of homo-acetogenic bacteria also utilize CO2/H2 as substrates to produce acetic acid. Finally, strictly anaerobic methanogens transform the acidification products (such as acetic acid, formic acid, CO2/H2, and others) into methane through a complex process involving various bacterial species working synergistically.2,6,9 Biomass, derived from plants, animals, and microorganisms, is a rational carbon-based feedstock that has gained prominence as a sustainable alternative to non-renewable energy sources. Its immense potential has led scientists, economists, and policymakers to envision a parallel economy known as a bio-based economy or circular bio-economy, highlighting its renewable nature.3 Among the various biomass sources, lignocellulosic feedstocks (LCF) stand out with an annual production of 200 billion tons, offering abundant availability.5,10 AD can be observed naturally in environments such as wetlands, swamps, and the digestive systems of various animals; its study has gained significant momentum due to the escalating energy crisis and mounting environmental concerns. Efforts to investigate this biochemical process have intensified, with a focus on utilizing effluents and residual substrates from diverse production chains. Notable examples include agriculture, livestock, agro-industries, municipal organic waste, and sewage sludge, as discussed in ref. 4. Apart from producing valuable high-energy biogas, AD also helps control the release of odours, reduces pathogens present in residual raw materials, and generates a stabilized liquid compound with beneficial properties suitable for use as a biofertilizer.5,7
There are three principal components in lignocellulosic biomass (LCB): cellulose, hemicellulose and lignin. These biopolymers are interlinked with each other in a hetero-matrix and at varying relative compositions depending on the type, species and even the origin of the LCB.5,11,12 The relative abundance of cellulose, hemicellulose and lignin is a key factor in determining the optimum energy derivable from LCB. However, the efficient breakdown of complex organic compounds and the subsequent biogas production from lignocellulosic biomass can often be challenging due to its recalcitrant nature.5,8,13
The inherent characteristics of lignocellulosic biomass materials render them less suitable for anaerobic digestion as substrates. These biomass materials exhibit diverse shapes, sizes, moisture contents, and varying levels of lignin, cellulose, hemicellulose, and fixed solids.14,15 Furthermore, due to the complex composition of lignocellulosic biomass, the enzymatic degradation of the biomass by hydrolytic microbes requires significant time due to its intricate properties.15,16 In light of these challenges, it is necessary to pretreat lignocellulosic biomass before introducing it into an anaerobic digester. This pretreatment is essential to overcome operational difficulties that commonly arise, including clogging, the formation of floating layers, and the recalcitrance of solids to enzymatic breakdown.16 Various pretreatment methods (physical, chemical, physiochemical, biological, enzymatic, etc.) have been explored to enhance the biodegradability and increase biogas yields. Among these, enzymatic pretreatment has gained considerable attention as a promising approach to overcome the limitations associated with biomass degradation.1,3 Enzymatic pretreatment involves the application of specific enzymes to the biomass to break down complex carbohydrates, such as cellulose and hemicellulose, into simpler fermentable sugars.3,5 This process aims to improve the accessibility of the substrate to microbial degradation during anaerobic digestion, thereby enhancing biogas production.
The microbial pretreatment approach is primarily centered around the utilization of either a single microbe or a consortium of microbes to treat the feedstock before it undergoes the AD process. This method offers several notable advantages, including minimal energy requirements and a low production of hazardous chemicals. However, a significant drawback of microbial pretreatment is the extended incubation time necessary to achieve substantial microbial growth, as well as the prolonged duration of the pretreatment process itself.17,18 However, several studies have demonstrated the positive effects of microbial pretreatment from a variety of microorganisms on biomass degradation and biogas yields. Microbial pretreatment offers several advantages over other pretreatment methods. Firstly, it is a mild and environmentally friendly process, as it operates under mild conditions and does not require harsh chemicals. Moreover, microbial pretreatment can be tailored to target specific biomass types and compositions, allowing for customization based on the feedstock characteristics.11,19,20 There has been extensive discussion on lignocellulosic biomass by several researchers in the past related to pre-treatment approaches and steps that can be followed for efficient bio-gas yields. However, this review is extensively focused on a hypothetic model of combined mechanical and biological treatment for practically applicable biomasses that should be followed for effective biogas/bio-CNG generation.
2. Lignocellulosic biomass: a potential source of biogas/bio-CNG
Lignocellulosic biomass encompasses different categories, including energy crops (such as perennial grasses), forest materials (like hard and softwood, sawdust, pruning, and bark residues), agricultural residues (including cereal straws, bagasse, and stovers), aquatic plants (like water hyacinth), and the organic portion of municipal solid waste.5,14,21 The compositional contents of various LCB can vary in their quantity; however generally, the primary components of lignocellulosic biomass are cellulose (35–50%), hemicelluloses (20–35%), lignin (5–30%), and other extracted substances (1–10%).21–23 The composition of lignocellulosic biomass varies depending on the substrate type and also within the same genera. Commonly utilized agricultural residues include waste materials like wheat straw, barley hull, barley straw, rice straw, oat straw, rice husks, sugarcane bagasse, corn cobs, corn stalks, and sorghum straw.
Cellulose fragments are composed of a linear chain of glucose units connected by 1,4-β-glycosidic bonds.5,14,21,24 The cellulose molecules form a crystalline network through cross-linking of various hydroxyl groups within hydrogen bonds. Hemicellulose, on the other hand, is a heteropolymer consisting of different monomers, including hexoses (β-D-glucose, α-D-galactose, and β-D-mannose) and pentoses (β-D-xylose and α-L-arabinose).24,25 It is attached to functional groups such as acetyl, methyl, glucuronic, galacturonic, and cinnamic acids. Lignin, which is insoluble in water, serves as a structural reinforcement and imparts resilience to plant tissues. The monomers of the lignin polymer consist of three phenolic compounds: coumaryl, coniferyl, and sinapyl alcohol.16,21,26–28 These three prime components make the cell wall of any type of biomass very rigid in nature and subsequently hard to break.
3. Applicable feedstock for bio-gas/bio-CNG production
Biogas and bio-CNG (compressed natural gas) are renewable energy sources that have gained significant attention due to their potential to reduce greenhouse gas emissions and provide a sustainable alternative to fossil fuels.29 These biofuels are produced through the anaerobic digestion of organic materials, commonly known as feedstock. A study was carried out by researchers to evaluate the annual energy potential associated with biomass waste (like animal manure, crop residues, logging residues, and municipal waste).30 The findings revealed that the technical bioenergy potential of these biomass resources amounts to 1.29 × 103 petajoules (PJ) in 2.31 × 104 cubic megameters (Mm3) of biogas and 7.79 × 102 PJ in 3.49 × 104 million liters (ML) of cellulosic ethanol. The selection of suitable feedstock plays a crucial role in the efficiency, profitability, and environmental sustainability of biogas and bio-CNG production systems.3,4 This section aims to explore various applicable feedstock options for the production of biogas and bio-CNG.
3.1 Agricultural residues
Agricultural wastes refer to waste materials generated from diverse agricultural activities. According to the UN, these wastes typically encompass manure and other byproducts from farms, poultry houses, and slaughterhouses, as well as waste from the harvesting process, fertilizer runoff from fields, and pesticides that find their way into water, air, or soils.31,32 Additionally, according to the world energy council, spoiled food waste can also be considered a part of agricultural waste. Crop residue, commonly known as harvest waste, consists of both field residues, which are the remains left in the agricultural field or orchard after harvesting, and process residues, which are the leftovers from the processing of crops into usable resources. Examples of field residues include stalks, stubble, leaves, and seed pods, while process residues include materials like sugarcane bagasse and molasses.32,33
Agricultural residues are one of the primary feedstock sources for biogas and bio-CNG production. These residues include crop residues such as straw, husks, stalks, and cobs.21,28 The residues from crops like wheat, rice, maize, and sugarcane are particularly suitable for anaerobic digestion.22,28 These feedstock materials are abundantly available, making them cost-effective and environmentally friendly options for bio-gas/bio-CNG production. Additionally, their utilization helps to manage agricultural waste and reduces the need for traditional disposal methods such as open burning as commonly practiced across the world, especially in developing countries like India. In India, a diverse array of crops is cultivated, accompanied by the generation of substantial amounts of crop residues. Statistics indicate that India produces over 500 MT of residues each year from its primary crops. Notably, approximately 65% of these residues find purposeful applications such as animal feed, bio-manuring, soil mulching, temporary shelters, and fuel for both domestic and industrial consumption.34 Additionally, each year, a staggering 140 billion tons of biomass are generated from diverse agricultural residues.35 Insufficient implementation of sustainable management practices has resulted in the burning of a substantial amount of crop waste in India each year, estimated to be about 92 metric tons.32 This practice leads to the release of excessive particulate matter emissions and contributes significantly to air pollution.
3.2 Animal manure
Animal manure is another significant feedstock for biogas and bio-CNG production. Livestock operations generate substantial amounts of organic waste, which can be efficiently converted into biogas through anaerobic digestion. Manure from cattle, pigs, poultry, and other livestock contains high levels of organic matter, making it an excellent source of methane production. Biogas production from animal manure not only helps in waste management but also reduces odors and potential water pollution risks associated with manure storage.36,37 According to a source from Pashudhan Praharee, India is home to approximately 535.78 million livestock, contributing to an annual production of around 3 million tonnes of livestock waste. This waste primarily consists of various materials such as dung, urine, placenta, bedding, feed wastage, and milk-house wastes, among others.38 According to the 20th livestock census conducted in 2019, India boasts one of the largest livestock populations globally, with a total count of 536.76 million animals. This population includes 193.46 million cattle, 148.89 million goats, 109.85 million buffalo, 74.26 million sheep, 0.06 million yaks, 0.39 million mithun, 9.06 million pigs, 0.34 million horses and ponies, 0.08 million mules, 0.12 million donkeys, and 0.25 million camels,39 as shown in Fig. 1. Thus, livestock manure plays an important role in economies, and its sensible and proper utilization through the adoption of contemporary processing technologies has the potential to greatly enhance earnings (especially in rural areas) by harnessing these enormous quantities of biomass for power generation and for organic recycling in general. India with its 60% agricultural sector has huge potential for biogas/bio-CNG generation from the abundant and wide range of feedstock materials. According to the Government of India (GoI), a total of 44 bio-CNG projects have been installed in the country during the last five years and the current year as of 30-11-2022 with a total capacity of 218 tonnes per day, for this renewable fuel40 as shown in Fig. 2.
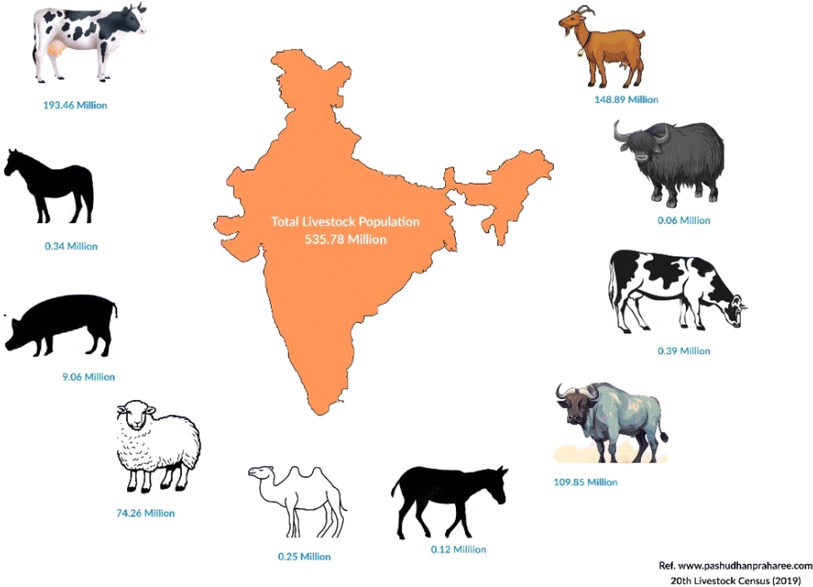 |
| Fig. 1 Pictorial diagram representation of livestock availability of India.38 | |
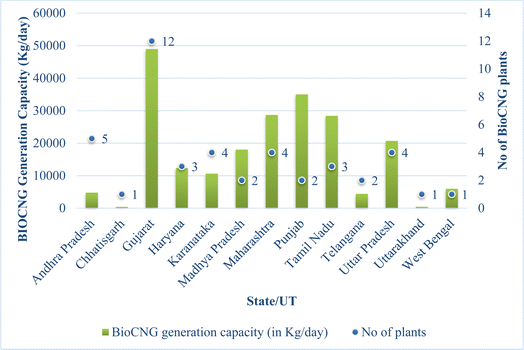 |
| Fig. 2 Figure representing bio-CNG plants operational in various districts of India (updated data as on 6 January 2023).41 | |
3.3 Energy crops
Energy crops specifically grown for biogas production are becoming increasingly popular as feedstock sources. Perennial grasses such as switchgrass, miscanthus, and reed canary grass are considered ideal energy crops due to their high biomass yield and ability to grow under diverse climatic conditions.16,30,42 These crops require minimal fertilizers, pesticides, and water, making them environmentally sustainable choices. Energy crops provide a consistent and reliable feedstock supply for biogas plants, ensuring a steady production of biogas or bio-CNG throughout the year.11,43 In India, the production of crop residues from the 10 major Indian crops amounts to approximately 683 million tonnes annually, as reported in ref. 42 and 44, which also highlighted the use of Opuntia ficus-indica (L) Mill. and Euphorbia tirucalli L. as energy crops for anaerobic digestion. Over a period of four months, the plantations with the highest density, specifically Euphorbia tirucalli at 266
667 plants per hectare and Opuntia ficus-indica (L) Mill. at 20
000 plants per hectare, produced approximately 1791 m3 of methane for Euphorbia tirucalli L. and 1860 m3 for Opuntia ficus-indica (L) Mill. from one hectare of marginal land. However, according to ref. 41 and 45, typically, the methane yield obtained from anaerobic digestion of energy crops is lower compared to that from crop residues such as corn stover and wheat straw. Nevertheless, it is possible to improve the yield by pre-treating energy crops, which involves breaking down the cell-wall structure to facilitate cellulose hydrolysis.28,45
3.4 Organic fraction of municipal solid waste (OF-MSW)
The effective management of Municipal Solid Waste (MSW) also referred to as the organic fraction of municipal solid waste (OF-MSW) remains a persistent challenge across all nations. It serves as a crucial component in the journey towards achieving a circular economy. According to ref. 46, the United States, China, and India rank as the top three contributors to municipal solid waste globally. Research indicates that developed countries generate approximately 107 kg of food waste per capita per year, while developing countries produce around 56 kg of food waste per capita per year. These figures clearly demonstrate that higher living standards are associated with increased waste generation.47 Furthermore, the composition of solid waste exhibits variations based on income levels. Adhering to the principles of the circular economy, it becomes imperative to generate high-quality digestate suitable for agricultural applications. Achieving this outcome hinges upon the initial quality of the organic fraction of municipal solid waste (OF-MSW). Consequently, it becomes crucial to delve into the factors that influence the characteristics of the feedstock. In low-to-middle-income populations, the predominant type of waste generated is organic waste. Conversely, high-income populations tend to produce larger amounts of waste paper, metals, and glass. According to ref. 48 and 49, MSW is composed of various types of waste. These include organic waste, which consists of food scraps, yard leaves, grass, brush, wood, process residue paper, and similar materials. Paper waste, on the other hand, encompasses paper scraps, cardboard, newspapers, magazines, bags, boxes, wrapping paper, telephone books, shredded paper, and paper beverage cups. Although paper is technically organic, it is not classified as organic unless it is contaminated by food residue. Another category is plastic waste (PW), which includes bottles, packaging, containers, bags, lids, and cups. Glass waste consists of bottles, broken glassware, light bulbs, and colored glass. Metal waste encompasses cans, foil, tins, non-hazardous aerosol cans, railings, bicycles, and similar items. Lastly, there is the category of other waste, which includes textiles, leather, rubber, multi-laminates, e-waste, appliances, ash, and other inert materials. MSW can also be further divided into different streams. Recyclables encompass paper, glass, plastic, metals, and other materials that can be recycled. Compostable organic matter consists of food waste, fruit and vegetable waste, and similar organic materials suitable for composting. Toxic substances include paints, pesticides, medicines, used batteries, and other hazardous materials. Lastly, hazardous solid waste includes items like blood-stained cotton, disposable syringes, sanitary napkins, and other potentially harmful waste materials.
According to ref. 50, the generation of municipal solid waste is projected to increase to 3.4 billion tonnes by 2050. Additionally, there is a concerning prediction that the number of individuals lacking adequate access to essential waste management services may rise to 5.6 billion by the same year. This alarming situation needs to be managed effectively. Organic waste from municipal sources, including food waste and green waste, can be effectively utilized as feedstock for biogas and bio-CNG production.51,52 The organic fraction of municipal solid waste contains significant amounts of organic matter that can be anaerobically digested to produce biogas.45,53 Overall, methane emissions from municipal solid waste (MSW) landfills are recognized as a significant contributor. Currently, unsegregated MSW is indiscriminately dumped in these landfills, posing grave environmental and groundwater risks.52 By diverting organic waste from landfills, the production of biogas from municipal waste helps in reducing methane emissions, minimizing landfill space requirements, and promoting a circular economy approach. With the projected increase in population and economic growth, conversion of bio-waste can offer multiple co-benefits, in addition to mitigating greenhouse gas emissions. These benefits include (i) utilizing biogas as a substitute for fossil energy, thereby reducing direct methane emissions from landfills, (ii) freeing up valuable land occupied by landfills for other productive activities, (iii) reducing health hazards associated with landfills, and (iv) recovering organic fertilizer through improved waste and slurry management.49,53,54 Accurately estimating future waste generation in urban areas and determining the potential energy that can be derived from these waste resources through anaerobic digestion are crucial steps towards building sustainable cities in developing countries like India, which are undergoing significant rural to urban transitions.
3.5 Industrial waste
Certain industrial wastes can also serve as feedstock for biogas and bio-CNG production. For example, wastewater from food processing facilities, breweries, and distilleries often contains high organic content, which can be anaerobically digested to generate biogas.47,55 This offers a dual benefit of waste treatment and renewable energy generation, contributing to the sustainability goals of industries. For example, the largest proportion of waste generated in the brewing industry is spent grain, also known as exhausted malt. It accounts for approximately 85% of the total waste produced during the brewing process. Spent grain has a composition that is rich in carbohydrates, cellulose (15–25%), hemicellulose (28–35%), lignin (28%), proteins (15 to 26.2%), free amino acids, lipids (10%), phenolic compounds, vitamins, and minerals such as calcium, selenium, phosphorus, and magnesium.47,56 It also contains a high moisture content ranging from 75% to 80%. The specific composition of spent grain can vary depending on the type of barley used and the conditions during the technological process. Ref. 57 and 58 highlight that anaerobic digestion (AD) is commonly employed for the treatment of both liquid and solid waste streams. These include industrial wastewater with a significant organic content, the organic fraction of municipal solid waste (OFMSW), and sewage sludge.
The selection of suitable feedstock is vital for the successful implementation of biogas and bio-CNG production systems. Agricultural residues, animal manure, energy crops, organic municipal waste, and industrial wastes offer diverse and abundant feedstock options. Utilizing these organic materials through anaerobic digestion helps in waste management, reduces greenhouse gas emissions, and provides a renewable energy source. Furthermore, the production of biogas and bio-CNG from these feedstock sources promotes a circular economy by transforming organic waste into valuable energy resources. Continued research and technological advancements in feedstock treatment will lead to enhancement of bioenergy generation.
4. Microbial approach towards a high BMP yield
The breakdown and conversion of biomass into biogas/bio-CNG can be enhanced through various processes, including physical, physio-chemical, and biological methods. Each approach has its own set of advantages and disadvantages. However, among these methods, the biological process has emerged as the most economically viable and feasible option.5,59,60 Biological pretreatment offers unique advantages, such as low energy consumption and a high level of environmental friendliness. Studies have shown that environmentally friendly pretreatment methods do not generate significant pollution, particularly in terms of water, air, and soil, following the pretreatment process.59 Cellulose, a major component of biomass, is relatively resistant to microbial degradation compared to hemicelluloses, which are more easily broken down. Lignin, another component, is highly resistant and requires specialized fungi for its degradation. Different types of fungi, such as brown rot, white rot, and soft rot fungi, are commonly utilized to break down these biomass components, each employing unique mechanisms to do so.61 As a result of the lower energy consumption, biological processes are always under research and areas of focus. Processing of the feedstock requires a high level of residence time, and the activity of the reaction also decreases with increasing temperature, since microorganisms are unable to withstand high temperatures.62 The utilization of fungal and bacterial strains or their enzymes is a prominent approach in this method. It is gaining increasing attention owing to its remarkable ability to operate within a relatively short reaction time while requiring minimal nutritional resources for enzymatic reactions.43,63 It is to be noted that fungal pretreatment necessitates an extended incubation period, ranging from weeks to months, whereas bacterial and enzymatic pretreatments can be accomplished within a few hours.64 In the biological route, anaerobic digestion and fermentation are the two main categories. In anaerobic digestion, microorganisms break down biomass and lignocellulosic materials in an oxygen-free environment, resulting in the production of biogas, also known as biomethanation. Anaerobic digestion consists of four steps mainly (i) hydrolysis, where the initial breaking of polysaccharide molecules takes place, (ii) acidogenesis, where the products of hydrolysis are converted into volatile fatty acids, alcohols, hydrogen, and carbon dioxide, (iii) acetogenesis, where the volatile fatty acids and alcohols are further broken down into acetic acid, hydrogen, and carbon dioxide, and (iv) methanogenesis, where the acetic acid, hydrogen, and carbon dioxide are converted into methane and water by methanogenic archaea.60,64 This process mimics the natural decomposition of organic matter, leading to the creation of a valuable fossil fuel alternative. By leveraging the potential of biological pretreatment methods and utilizing anaerobic digestion or fermentation, the biomass–bioCNG sector can effectively convert biomass resources into biogas, contributing to a sustainable and eco-friendly energy solution.
Furthermore, fungal pretreatment is extensively used as a pretreatment approach for lignocellulosic biomass.61,65 The fungi may be categorized as cellulose or lignin degrading species, where cellulolytic fungi are mainly brown-rot-fungi61 and for delignification, white rot fungi65 are used during the pre-treatment process. For better CH4 yield, several researchers have used fungi during their pre-treatment process. In a study conducted on corn silage, pretreatment with Trametes versicolor showed an improved production of biogas. Total solids (TS) of corn silage estimated were 35.75%. Corn silage was co-digested with cow manure along with corn grits under mesophilic conditions, whereas the methane production rate improved up to 0.236 m3 CH4 per kg VS from 0.167 m3 CH4 per kg VS of untreated corn silage.66 Ref. 67 showed the impact of a combination of metals (MnSO4; CuSO4 and FeSO4) along with the fungus Polyporus brumalis BRFM985 on wheat straw. They found an improved biogas production of 52%. V. Wyman et al.68 reported and screened different fungi (Pleurotus eryngii, Pleurotus ostreatus, and Trametes versicolor) for their pretreatment productivity towards ligno-hemicellulosic biomass. According to the study the production of ligninolytic enzymes is high in Pleurotus eryngii resulting in a 19% increase in biogas yield. Several studies were reported on the disintegration of lignin and hemicellulose by different fungal species. The effects of moisture content on the pre conditioning of Agropyron elongatum with Flammulina velutipes were assessed, and at 65% optimum moisture content, a remarkably 120% higher biogas production was observed.69 After 48 days of pretreatment of Albizia chips with Ceriporiopsis subvermispora, lignin removal from biomass reached a maximum of 24% and methane production increased by 3.7 times to approximately 123.9 L per kg VS.70 The fungal pretreatment on rice straw for biogas production was explored using two species namely Pleurotus ostreatus and Trichoderma reesei by solid state anaerobic digestion. After the pre-treatment process, a methane yield of 263 L per kg VS achieved which was 120% higher than that of the untreated rice straw. Whereas, in another study over rice straw, pretreatment with Trichoderma reesei resulted in a methane yield of 214 L per kg VS.71 Hence, fungal treatment can be an effective choice for disintegration of biomass and a subsequently high biogas yield.
Pretreatment of ligno-hemicellulosic biomass with microbial consortia is an efficient approach to improve biomass degradation. A consortium is a group of two or more diversified microorganisms living symbiotically. Cellulomonas and Cytophaga groups of bacteria and various fungi such as Humicola, Penicillium, Aspergillus and Trichoderma are studied mostly for their ability to secrete extracellular proteins.72 In a study conducted in ref. 73, microbial consortia WSD-5 was used for pretreatment of Napier grass which improved the methane yield by 49% during the AD process. Consortia WSD-5 is a combination of fungal and bacterial communities, the main fungus is Coprinus cinereus and bacterium is a Gram negative, Ochrobactrum sp. Along with these, two more microbial consortia were studied for the pretreatment of Napier grass, XDC-2 and MC1. XDC-2 was primarily composed of genera Clostridium, Bacteroides, Alcaligenes and Pseudomonas and MC1 was composed of thermophilic bacteria, majorly cellulose degrading bacteria such as as Clostridium straminisolvens. The pretreatment of rice straw was performed with rumen fluid, which showed the improved biogas production with an 82.6% higher methane yield.74 Also, C. Zhong et al.75 studied pretreatment of wheat straw with a microbial consortium during the AD process and obtained an 80.34% higher methane yield. A degradation study was reported on catalpa saw dust (cellulose 45.89%, hemicellulose 18.11%, and lignin 21.75%) with the help of two consortia such as an aerobic consortium (CS-5) and an anaerobic consortium (BC-4) to enhance biogas production.76 The CS-5 aerobic consortium consists of individual species such as Micrococcus luteus SR-1, Citrobacter freundii SR-3, Exiguobacterium acetylicum SR-5, Acidisoma tundrae strain SR-14 and Dyella sp. Strain SR-16 whereas BC-4 has Thermoanaerobacterium aciditolerans strain SR-4, Ruminococcus flavefaciens strain SR-7, Caproiciproducens galcititolivorans strain SR-8 and Methanobrevibacter thaueri stain SR-13. The synergistic effect of both the consortia CS-5 and BC-4 resulted in a 113.7% higher methane yield. Under thermophilic conditions due to high lignocellulolytic enzyme activities, anaerobic digestion of ligno-hemicellulosic biomass was advantageous for its degradation and subsequent high yield of CH4 production.77
The microbial pretreatment approach has been of utmost priority among the scientific community, and is basically centered around the use of either a single microbe or a consortium of microbes to treat the feedstock before it undergoes the AD process. For instance, S. O. Thong et al.78 investigated the enzymatic hydrolysis of cassava starch waste water using a thermophilic mixed culture (natural microbial consortia of samples of a hot spring located in Southern Thailand) and observed a significant increase in biohydrogen production. Similarly, fermentation of cassava wastewater by using Clostridium acetobutylicum resulted in up to 42% COD (chemical oxygen demand) removal and COD concentrations of 10.7, 7.5, and 5 g L−1 provided 1.34, 1.2 and 2.41 mol H2 per mol of glucose.79 Again, according to ref. 80 rumen bacteria isolated from a barn for the treatment of cow manure feedstock resulted in a CH4 enhancement of 103.3% (CH4 yield: 138 mL per g VS). The effectiveness of the xylanase enzyme isolated from Aspergillus niger LC1 in saccharifying various feedstocks was investigated. Feedstocks including barley husk, groundnut shell, pearl millet husk, rice husk, rice straw, sugarcane bagasse, wheat bran, and wheat straw were studied and, the highest saccharification yield of up to 34.5% was achieved with rice straw.6 K. Sophanodorn et al.81 used 2% (2% v/v) of cellulase enzyme with 2398 units per g, β-glucosidase 577 units per g, and pH 4 (Union Science Company, Chiang Mai Thailand) over hydrothermally pretreated dried tobacco stalks and on tobacco stalk residue, which resulted in total and reducing sugar concentrations of dried tobacco stalks of 27.97 g L−1 and 5.43 g L−1, respectively. Also, it was observed that when hydrothermal pretreatment was applied, the total sugar (complex sugar) concentration increased. Meanwhile, the reducing sugar (simple sugars) was observed to be lower compared to that in the untreated biomass with values ranging from 4.07 to 4.55 g L−1 according to the study.
The microbial community residing in the rumen, comprising bacteria such as Fibrobacter succinogenes, Ruminococcus flavefaciens, Roseovarius albus, and others, possesses the ability to attach to lignocellulosic materials. These microorganisms produce enzymes that effectively remove the waxy layer and lignin, facilitating the hydrolysis of approximately 65% of cellulose within a span of 48 hours.11,82 Also, the pretreatment of unsterilized yard trimming by C. subvermispora followed by anaerobic solid-state digestion yielded 44 L kg−1 of methane. T. Rangseesuriyachai et al.43 studied anaerobic co-digestion of elephant dung (ED) and Napier grass (NG) with and without the biological pretreatment approach at various mixing ratios. The biological pretreatment of Napier grass was performed using the microbial activator super LDD1 (mixed culture microbial sludge) enzyme before further biogas production. The results confirmed that LDD1 enzymatic microbes increased methane production capacity by 1.95 times compared to untreated napier grass. Furthermore, the findings from the data analysis demonstrated that employing a NG/ED ratio of 1
:
1 for a 14-day pretreatment period resulted in the highest cumulative methane production, reaching 234.8 ± 5.9 mL CH4 per g VS. This represents a substantial increase of 99.2% compared to the baseline. In a comprehensive investigation, L. A. Fdez-Guelfo et al.83 explored the composting pretreatment of the organic fraction of municipal solid waste (OF-MSW) within a thermophilic dry batch anaerobic digestion (AD) system. Their findings indicated a substantial enhancement in the specific microbial growth rate, ranging from 160% to 205%. This suggests that the composting pretreatment can significantly promote the microbial activity in the subsequent AD process. In contrast, previous studies, such as that conducted in ref. 84, reported a reduction in volatile solids by 19.5% during composting, alongside a 40% loss of methane, emphasizing the complexity and variability of these processes. Y. Ueno et al.85 furthered the investigation by examining synthetic OF-MSW subjected to pre-hydrolysis pretreatments under both mesophilic and thermophilic conditions. Their results revealed that mesophilic pre-hydrolysis was more efficient in hydrogen production, whereas thermophilic pre-hydrolysis led to better solubilization. Additionally, a continuous two-stage AD system achieved the highest methane production, reaching 341 mL CH4 per g VS. Furthermore, during thermophilic pre-hydrolysis of OF-MSW in a continuous two-stage AD system, C. Escamilla-Alvarado et al.86 reported an impressive 81.5% COD removal with 95.7% utilization of volatile suspended solids (VSS). This process resulted in twice the biogas production compared to the untreated sample, as demonstrated in ref. 85.
Numerous aerophilic bacterial species have been studied and stated to have high degrading potential towards ligno-hemicellulosic biomass with the foremost advantage of a faster growth rate than fungi.87 V. B. Barua et al.88 reported a bacterial strain isolated from the gut of silverfish such as Citrobacter werkmanii VKVVG4 which enhanced the solubilization of water hyacinth during anaerobic digestion and also showed a cumulative biogas production of 3737 ± 21 mL for pretreated water hyacinth on the 50th day. The co-culture of Bacillus sp. with rice straw showed a substantial reduction in lignin content and 76% rise in methane production.89 Along with aerophilic bacterial treatment, microaerobic pretreatment also exists. Commonly microaerobic refers to giving little oxygen to the digestion system to boost the rate of hydrolysis and acidification.90 It is being recommended to execute the microaerobic treatment at the pretreatment stage to avoid the risk of mixing of oxygen with biogas during the AD process. Some of the biogas ventures have implemented microaerobic technology to achieve the best methane yields.91 During these large-scale biogas projects, livestock manure and biogas slurry can be chosen as a microaerobic inoculum.92 Studies showed that on utilization of cow manure as a microbial additive during microaerobic pretreatment, the rate of hydrolysis of ligno-hemicellulosic biomass increased by two times and estimated VFAs were 6–7 g L−1 resulting in 419 mL per g VS at 5%.93 The utilization of microaerobic technology at the pretreatment stage is a simple and user-friendly approach. Table 1 represents all types of biological pretreatments mainly used for disintegration of biomasses used and their CH4 yield capacity.
Table 1 Biological pre-treatment effects on biogas/methane yield from available recent data in the literature
Pre-treatment methods |
Microorganism used/inoculum |
Biomass |
AD conditions |
Effect on methane or biogas production |
References |
Fungal pre-treatment |
Polyporus brumalis and Trametes versicolor |
Wheat straw |
Batch, 36 °C, 57 days |
52% higher methane yield |
66 and 67 |
Corn silage |
Semi-continuous, 37 °C, 21 days |
Methane generation rate 0.236 m3 CH4 per kg VS (control 0.167 m3 CH4 per kg VS) |
Pleurotus eryngii |
Corn stover |
Batch, mesophilic, 40 days |
19% higher biogas production |
68 |
Flammulina velutipes |
Agropyron elongatum (tall wheat grass) |
Batch, 37 °C, 24 days |
120% higher biogas production |
69 |
Pleurotus ostreatus |
Rice straw |
Batch (SS), 37 °C, 45 days |
120% higher methane yield |
71 |
Trichoderma reesei |
Rice straw |
Batch (SS), 37 °C, 45 days |
78.3% higher methane yield |
71 |
Ceriporiopsis subvermispora |
Albizia chips |
Batch (SS), 37 °C, 58 days |
3.7-fold higher methane yield |
70 |
P. flavido-alb |
Grass of verge |
|
No effect |
94 |
Ceriporiopsis subvermispora |
Yard trimmings |
Batch (SS), 37 °C, 45 days |
106% higher methane yield |
95 |
Bacterial pre-treatment |
Composting |
OF-MSW |
Mesophilic dry batch |
19.55 VS loss, 40% loss of methane |
84 |
Composting |
OF-MSW |
Thermophilic dry batch |
160–205% higher specific microbial growth rate |
83 |
Mesophilic and thermophilic pre-hydrolysis |
OF-MSW (synthetic) |
Mesophilic and thermophilic (continuous 2-stage) |
Mesophilic resulted in better hydrogen production, while thermophilic resulted in better solubilization |
86 |
Thermophilic pre-hydrolysis |
OF-MSW (synthetic) |
Thermophilic (continuous 2-stage) |
81.5% COD removal with 95.7% VSS destruction and 2 times higher biogas production |
85 |
Thermophilic pre-hydrolysis |
Food waste |
Mesophilic AD |
61.3% VS destruction, 280 mL per g VS methane yield |
96 |
Mesophilic culture pre-hydrolysis |
Food waste |
Mesophilic continuous (2 stage system) |
9% and 13% higher biogas production than mesophilic and thermophilic AD |
97 |
Bacillus sp. |
Rice straw |
Batch (SS), 37 °C, 50 days |
76% higher biogas production |
89 |
Bacillus subtilis |
Corn straw |
Batch (SS), 37 °C, 50 days |
17.35% higher methane yield |
87 |
Citrobacter werkmanii VKVVG4 |
Water hyacinth |
Batch (SS), mesophilic, 80 days |
3.07 times higher biogas production |
88 |
Anaerobic inocula (manure & crops) |
Grass silage |
55 °C, 63 days |
405 mLCH4 per g VS |
98 |
Anaerobic sludge |
Typha latifolia |
Batch assay, 37 °C, 60 days |
151 mL CH4 per g VS |
99 |
Cattle dung |
Duckweed (aquatic plant) : cattle dung in a 1 : 1 ratio |
Batch assay, 38 °C, pH 7.2 |
580 mL per days |
100 |
Biogas slurry |
Rice straw |
35 ± 1 °C |
233.3 mL methane per g VS |
101 |
|
Anaerobic sludge |
Potamogeton maackianus |
Semi continuous operation |
255.9 mL CH4 per g VS |
102 |
|
Bioaugmentation with Piromyces rhizinflata YM 600 |
Corn silage and cattail |
60 days |
No effect on the improvement of methane production, improved the VFA degradation rate |
99 |
Microbial consortium |
Microbial consortium TC-5 |
Wheat straw |
Batch, 45 °C, 35 days |
36.6% higher methane yield |
77 |
Microbial consortium |
Saw dust |
Batch, mesophilic, 28 days |
25.6% higher biogas production |
103 |
Rumen fluid |
Rice straw |
Batch, 35 °C, 30 days |
82.6% higher methane yield |
74 |
Microbial consortium MC1 |
Napier grass |
NA |
39% higher methane yield |
104 |
Microbial consortium WSD-5 |
Napier grass |
NA |
49% higher methane yield |
Microbial consortium XDC-2 |
Napier grass |
NA |
32% higher methane yield |
Microbial consortium CS-5 & BC-4 |
Catalpa sawdust |
50 °C |
113.7% improved methane |
103 |
Enzyme pre-treatment |
Microbial consortium |
Wheat straw |
Batch, 37 °C, 20 days |
80.34% high methane yield |
75 |
Cellulase |
Corn stover |
Batch, 37 °C, 18 days |
36.9% higher biogas production |
105 |
Endoglucanase + xylanase + pectinase |
Spent hops |
Semi-batch, 37 °C |
13% higher biogas production |
106 |
Cellulase + cellobiase |
Switch grass |
Batch, 50 °C, 30 days |
Methane yields 274.28 mL per g (VS), (control 197.39 mL per g (VS)) |
107 |
Endoglucanase + xylanase + pectinase |
Sugar beet pulp silage |
Batch, 37 °C, 30 days |
27.9% higher biogas production |
108 |
Laccase (Trametes versicolor) |
Willow |
NA |
33% higher methane yield |
109 |
Endoglucanase/laccase (Pleurotus ostreatus) |
Pulp & paper sludge |
37 °C, 50 days |
34% higher methane yield |
110 |
Endoglucanase + exoglucanase + xylanase |
Sorghum forage |
Batch, 35 °C, 30 days |
15% higher methane yield |
111 |
Lipase (Candida rugosa) |
Butter |
NA |
84% methane yield improved |
112 |
Laccase |
Corn stover |
Batch, 37 °C, 30 days |
25% higher methane yield |
113 |
Cellulolytic enzymatic cocktail (novozymes) |
Corn cob |
Mesophilic |
14% higher methane yield |
104 |
Mn peroxidase + versetile peroxidase |
Corn stover |
Batch, 37 °C, 30 days |
17% higher methane yield |
113 |
It is well known that enzyme pretreatment has a quick rate of reaction and very slight loss of sugars during digestion due to which it becomes more and more appealing. Before anaerobic digestion, exogenous application of enzymes of the hydrolytic or oxidative classes can hasten the degradation of ligno-hemicellulosic biomass under anaerobic conditions.114 The biological breakdown of cellulose takes place in the presence of enzymes with exoglucanase, endoglucanase and β-glucosidase activities whereas hemicellulose breakdown involves a lot of enzymes such as endo-xylanase, endo-mannanase and α-glucuronidase.5,115 There are various factors on which efficiency of enzyme pretreatment is based on such as the activity of the enzyme, enzyme specificity towards the substrate, tolerance of the enzyme to various inhibitors, amount of enzyme used for the treatment, incubation time, the anaerobic digestion system, enzymatic stability at several temperatures and pH.116,117 The enzyme pretreatment approach can enhance biogas production from recalcitrant ligno-hemicellulosic biomass.114 Y. Lin et al.110 studied pulp & paper sludge, and observed the effect of endoglucanase and laccase enzymes isolated from Pleurotus ostreatus, and obtained 34% higher methane yield. In another pretreatment study reported on corn stover, cellulase improved the rate of substrate breakdown and better biogas was produced by up to 36.9% after 24 h of incubation time.105 K. Ziemiński et al.106 investigated the utilization of spent hops pretreated with a combination of enzymes endoglucanase, xylanase and pectinase (volume ratio of 3
:
1 respectively) which aids in an improved biogas production of 13%. Lignin was a hindrance to improve pretreatment efficacy when ligno-hemicellulosic biomass was pretreated by laccases and versatile peroxidases. Willow was pretreated with laccase isolated from Trametes versicolor which showed a 33% higher methane yield.109 The ideal incubation conditions for these enzymes are 37–50 °C and 2–72 h. Limited use and high cost of commercial enzymes is the major reason for inadequate applications during digestion of ligno-hemicellulosic biomass. Lately, the solid-state digestion method, which relied on agricultural and industrial waste as the primary medium components to produce affordable enzymes was proposed.68
5. Physical pretreatment towards effective digestion
The physical pretreatments are those methods that do not use external compounds like chemicals or microbes during the process. This approach can increase the surface area of ligno-hemicellulosic biomass by reducing the particle size.6 Size reduction can encourage the digestion of biomass during the AD process by making it more accessible to microbial and enzymatic exposure. Prominently, physical pretreatments do not produce any noxious compounds, which constrain the AD process.118,119 Physical pretreatment includes mechanical, irradiation and microwave-based methods.6,120 Mechanical pretreatment methods have also gained attention in OF-MSW processing. Researchers have employed techniques such as rotary drums, disc screens, screw presses, and shredders with magnetic separation. B. Zhu et al.121 reported methane yields ranging from 457 to 557 mL CH4 per g VS and methane content between 57.3% and 60.6% through rotary drum pretreatment, sometimes combined with semi-composting. Similarly, T. Subramani et al.122 observed 18–36% higher biogas production with the same approach. T. L. Hansen et al.123 explored the use of screw presses, disc screens, and shredders with magnetic separation for mechanical pretreatment of sorted OF-MSW under thermophilic batch conditions, achieving methane yields of 461 mL CH4 per g VS, 428 mL CH4 per g VS, and 487 mL CH4 per g VS, respectively. Mechanical pretreatment is a familiar method to improve biogas production, though it is still considered to be an expensive method, due to its high energy requirements.124 There are widely used mechanical techniques for pretreatment which are chipping, grinding, milling, knife milling, hammer mill, extrusion, etc.
Several studies were reported on the effect of particle size of different feedstocks. S. K. Sharma et al.125 reported and studied the effect of different particle sizes 0.088, 0.40, 1.0 and 6.0 mm of numerous agricultural residues like wheat straw, rice straw, cauliflower leaves, Ipomoea fistulosa leaves, banana peel, etc. and obtained the highest biogas yield with a particle size of 0.88 mm with Ipomoea fistulosa leaves followed by cauliflower leaves. M. A. De la Rubia et al.126 carried out a study on sunflower oil cake feedstock, and reported the maximum methane yield of 17% at a particle size of 1.4–2.0 mm. Some of the reports are on the different mechanical pretreatments which tested the particle size in the range of 0 to >20 cm.127 The study indicates no significant increase in methane yield against the untreated biomass. Correspondingly, C. Herrmann et al.128 compared various particle lengths of the same feedstock and discovered a strong link between particle length and methane yield. N. Pérez-Rodríguez et al.104 compared 30 and 60 min duration of pretreatment, and found a 21% rise in methane yield for 60 min whereas no effect was recorded for 30 min. S. Sumardiono et al.129 studied the effect of bagasse size reduction up to 0.85 mm (22 mesh) with the use of cow dung as an inoculum. The study resulted in the highest biogas yield of 51.04 L kg−1 substrate from a combination bagasse treated with 2% NaOH solution for 24 hours and as much as 20% cow's rumen. The difference between experimental and control groups can be summarized as, the experimental groups involving longer pretreatment durations and specific size reduction and treatment methods showed significant improvements in methane and biogas yields, whereas the control groups showed little to no improvement. According to ref. 127, importantly, the equipment's speed must be taken into account in order to reduce the cost of energy used during physical pretreatments. S. Menardo et al.130 carried out a study on barley straw and reported an increase of 54% CH4 yield at 5 mm particle size and 41% methane yield at 20 mm particle size separately. Additionally, it was found that methane yield decreased with particle sizes greater and smaller than the range of 2–5 mm.130–132 The methane yield with several feedstocks pretreated mechanically is presented in Table 2.
Table 2 Physical pre-treatment effects on biogas/methane yield from available recent data in the literature
Substrates |
Particle size |
Methane yield (untreated) |
Methane yield (after treatment) |
Reaction system |
References |
Barley straw |
5 mm |
240 mL per g VS |
370 mL per g VS |
Glass reactor 2 L |
130 |
20 mm |
240 mL per g VS |
339 mL per g VS |
50 mm |
240 mL per g VS |
286 mL per g VS |
Crop feedstocks (winter rye, sorghum, forage rye, maize, and triticale) |
6–33 mm |
278 mL per g ODM |
403 mL per g ODM |
Stirred tank reactor 3 L |
128 |
Maize stalks |
2 mm |
246 mL per g VS |
272 mL per g VS |
Glass reactor 2 L |
130 |
20 mm |
246 mL per g VS |
254 mL per g VS |
Glass reactor 2 L |
2 mm |
297 mL per g VS |
376 mL per g VS |
Bottle 0.5 L |
133 |
OF-MSW |
16 mm (shredder with magnetic separation) |
487 mL CH4 per g VS |
NA |
Thermophilic batch |
123 |
16 mm (use of disc screen) |
428 mL CH4 per g VS |
NA |
Thermophilic batch |
16 mm (use of screw press) |
461 mL CH4 per g VS |
NA |
Thermophilic batch |
Meadow grass |
<200 mm |
— |
347 mL per g VS (increase 20%) |
Bottle 0.5 L |
11 |
0–200 mm |
303 mL per g VS |
372 mL per g VS |
Bottle 0.5 L |
134 |
0.3 mm |
58.1 mL per g VS |
62.7 mL per g VS |
— |
131 |
Rice straw |
0.75 mm |
58.1 mL per g VS |
65.7 mL per g VS |
— |
50 mm |
197 mL per g VS |
203 mL per g VS |
Glass reactor 2 L |
130 |
Switch grass |
2–10 mm |
127.4 mL per g VS |
170.7 mL per g VS |
Bottle 0.5 L |
135 |
0.001 mm |
— |
Increase 20% (from 50 to 70%) |
Digester 0.45 L |
136 |
Water hyacinth |
0.05 mm |
— |
Increase 16% (from 50 to 66%) |
Digester 0.45 L |
136 |
1.0 mm |
— |
Increase 10% (from 50 to 60%) |
Digester 0.45 L |
2.5 mm |
— |
Increase 5% (from 50 to 55%) |
Digester 0.45 L |
0.3 mm |
167.8 mL per g VS |
245.6 mL per g VS |
Reactor 2 L |
132 |
1.2 mm |
167.8 mL per g VS |
264.7 mL per g VS |
Reactor 2 L |
0.3 mm |
67.1 mL per g VS |
70.3 mL per g VS |
— |
131 |
Wheat straw |
0.75 mm |
67.1 mL per g VS |
93.1 mL per g VS |
— |
0.088–0.759 mm |
183.4 mL per g VS |
252.8 mL per g VS |
Lab flask |
137 |
2 mm |
182 mL per g VS |
334 mL per g VS |
Glass reactor 2 L |
130 |
50 mm |
182 mL per g VS |
285 mL per g VS |
Glass reactor 2 L |
138 |
Wastepaper |
60 min of beating time |
132 mL per g VS |
215 mL per g VS |
Flask 0.5 L |
104 |
6. Chemical approach as a pre-treatment approach
The most preferred approach is chemical pretreatment of ligno-hemicellulosic biomass with chemicals like acids, alkalis and solvents because they can be very effective at degrading complex substrates.139,140 Acids and alkalis are commonly used to solubilize the hemicellulose and lignin present in biomass. Several studies demonstrated that the most promising method for treating ligno-hemi-cellulose is the dilute acid treatment.45,141 M. Germec et al.140 discovered that 1.58% v/v of dilute sulphuric acid (DSA) was the ideal acid concentration for the pretreatment of spent tea leaves. Furthermore, in the study, it was also observed that 131 °C for 20 min is more effective than 121 °C for 1 h in terms of temperature and reaction time respectively which was also suggested in ref. 142. I. Syaichurrozi et al.143 suggested dilute acid pretreatment under room temperature conditions for a duration of 2 days. Besides acid treatment, alkaline pre-treatment is also commonly used to disrupt the biomass surface layer. During delignification of biomass, alkaline pretreatment causes the cell wall to swell, increasing the internal surface area while simultaneously reducing the degree of polymerization and cellulose crystallinity.10,22 To boost the methane yield, researchers in ref. 144 and 145 studied and optimized the NaOH loading rate. During the pretreatment of giant reed with varied NaOH concentrations between 0.5% and 2% w/v an increase in methane yield at 2% NaOH from 217 mL per g VS to 355 mL per g VS was observed. M. S. Romero-Güiza et al.146 reported that 0.7% w/w KOH pretreated wheat straw produced 128% more methane than untreated wheat straw. Other studies were investigated with different concentrations of Ca(OH)2 at 6%, 8%, 10%, 12% and 15% out of which the maximum biogas production was found for 8% and 10% pretreated rice straw which also recorded the biogas production value of 34.3% and 36.7% higher than that of the control.147 Numerous studies have also explored the effects of chemical pretreatment on OF-MSW. M. Lopez Torres et al.148 investigated alkaline [Ca(OH)2] pretreatment and observed an 11.5% higher COD solubilization. They achieved a methane yield of 0.15 m3 CH4 per kg VS, which was 172% higher than that of the untreated sample. Conversely, another study used HCl addition at pH 2 under room temperature conditions and achieved a 13 ± 7% higher COD solubilization and a 48% increase in biogas production. Water hyacinth was tested in ref. 149 and reported to have a 97.6% higher methane yield when pretreated with 1-N-butyl-3-methyimidazolium chloride/dimethyl sulfoxide (DMSO) at 120 °C for 120 min. N. R. Katukuri et al.150 considered the pretreatment of Miscanthus floridulus by using 0.8% of H2O2 and recorded 49% increment in methane yield over the untreated substrate. Similarly, B. R. A. Alencar et al.151 explored a method to recover H2O2 which was used during the pretreatment, and also tested the efficacy of recovered H2O2 for reusing it. Methane yields from different residues after chemical pretreatment are presented in Table 3 and Fig. 3.
Table 3 Chemical pre-treatment effects on biogas/methane yield from available recent data in the literature
Substrate |
Pre-treatment conditions |
Methane yield (untreated) |
Methane yield (after pre-treatment) |
Reaction system |
References |
Corn straw |
Sulfuric acid 2% v/v |
100.6 mL per g VS |
175.6 mL per g VS |
Flask 1 L |
152 |
Hydrochloric acid 2% v/v |
100.6 mL per g VS |
163.4 mL per g VS |
Acetic acid 4% v/v |
100.6 mL per g VS |
145.1 mL per g VS |
Hydrogen peroxide 3% v/v |
100.6 mL per g VS |
216.7 mL per g VS |
Sodium hydroxide 8% v/v |
100.6 mL per g VS |
163.5 mL per g VS |
Calcium hydroxide 8% v/v |
100.6 mL per g VS |
206.6 mL per g VS |
Ammonia 10% v/v |
100.6 mL per g VS |
168.3 mL per g VS |
Pennisetum hybrid |
4% NaOH, 37 ± 0.5 °C |
249.3 mL per g VS |
281.4 mL per g VS |
NA |
153 |
Food waste |
Addition of HCl until pH = 2 at room temperature |
13 ± 7% higher COD solubilization and 48% higher biogas production |
NA |
Thermophilic batch |
154 |
Household waste |
KOH until pH = 10, 70 °C for 60 min |
Methane yield of 500 mL CH4 per g VS, no enhancement due to pretreatment |
NA |
Thermophilic batch |
155 |
OF-MSW |
Alkaline |
11.5% higher COD solubilization, methane |
Methane yield of 0.15 m3 CH4 per kg VS (172% higher than untreated) |
1 L batch reactor |
148 |
Corn stalk |
2% NaOH, 35 ± 1 °C |
187 mL per g VS |
196 mL per g VS |
NA |
156 |
Cotton gin waste |
Citric acid 0.5 mmol per g VS substrate |
95.4 mL per g VS |
147.1 mL per g VS |
Flask 0.25 L |
157 |
|
Hydrogen peroxide 0.5 mmol per g VS substrate |
178.0 mL per g VS |
247.5 mL per g VS |
Flask 0.25 L |
|
Ethanol 0.5 mmol per g VS substrate |
172.5 mL per g VS |
241.5 mL per g VS |
Flask 0.25 L |
|
Citric acid 0.5 mmol per g VS substrate |
180.4 mL per g VS |
353.5 mL per g VS |
Flask 0.25 L |
|
Hydrogen peroxide 0.5 mmol per g VS substrate |
275.0 mL per g VS |
385.6 mL per g VS |
Flask 0.25 L |
Olive pomace |
Ethanol 0.5 mmol per g VS substrate |
214.3 mL per g VS |
332.8 mL per g VS |
Flask 0.25 L |
157 |
Citric acid 0.5 mmol per g VS substrate |
200.1 mL per g VS |
183.2 mL per g VS |
Hydrogen peroxide 0.5 mmol per g VS substrate |
178.7 mL per g VS |
172.3 mL per g VS |
Ethanol 0.5 mmol per g VS substrate |
193.0 mL per g VS |
157.5 mL per g VS |
Sulfuric acid 2% v/v |
11.2 mL per g VS |
16.6 mL per g VS |
Bottle 0.6 L |
143 |
Salvinia molesta |
Sulfuric acid 4% v/v |
11.2 mL per g VS |
17.4 mL per g VS |
Bottle 0.6 L |
Sulfuric acid 6% v/v |
11.2 mL per g VS |
17.8 mL per g VS |
Bottle 0.6 L |
Switch grass |
Sodium hydroxide 7 g L−1 |
112.4 mL per g VS |
132.5 mL per g VS |
Bottle 0.5 L |
135 |
Water hyacinth |
Sulfuric acid 5% v/v; 60 min residence time |
58 mL per g VS |
64 mL per g VS |
Flask 1 L |
158 |
NMMO 85% 120 °C; 3 h |
274 mL per g VS |
304 mL per g VS |
Serum bottles 0.125 L |
159 |
Wheat straw |
Ethanol 50% 180 °C; 1 h |
274 mL per g VS |
316 mL per g VS |
Serum bottles 0.125 L |
159 |
Sodium hydroxide 1.6% w/w 30 °C; 24 h |
274 mL per g VS |
315 mL per g VS |
Serum bottles 0.125 L |
Citric acid 0.5 mmol per g VS substrate |
159.1 mL per g VS |
163.7 mL per g VS |
Flask 0.25 L |
157 |
Winery waste |
Hydrogen 0.5 mmol per g VS substrate peroxide |
171.3 mL per g VS |
190.2 mL per g VS |
Flask 0.25 L |
157 |
Ethanol 0.5 mmol per g VS substrate |
190.7 mL per g VS |
239.5 mL per g VS |
Flask 0.25 L |
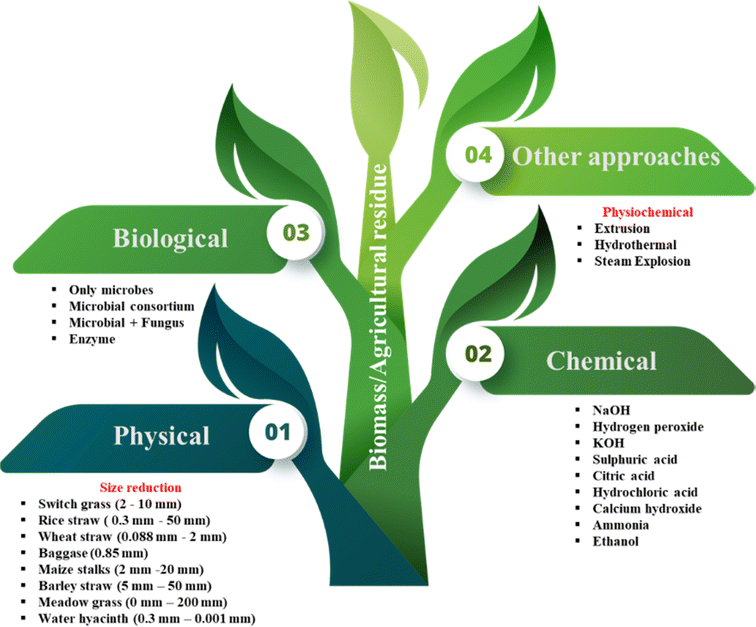 |
| Fig. 3 Preferred pre-treatment process for efficient biogas yield as per literature studies cited in this article. | |
7. Hypothetical combined physical and biological strategy for efficient biogas yield
Biogas production from biomass is a sustainable and renewable energy solution that holds immense potential to address global energy and environmental challenges. Combining physical and biological methods in the biogas production process can enhance efficiency, optimize yields, and ensure a more sustainable and economically viable approach for harnessing this valuable energy source. In this hypothetical scenario, a cutting-edge biogas production system is being considered that integrates physical and biological techniques to maximize the decomposition of organic matter and subsequent biogas yield. This innovative approach seeks to overcome the limitations of conventional anaerobic digestion systems and significantly improve the conversion of biomass into biogas.
The physical aspect of the proposed system focuses on preprocessing biomass before its introduction into the anaerobic digestion process. According to several studies mentioned, advanced mechanical techniques, such as hydrothermal pretreatment after mechanical milling through use of a shredder (one way or through use of two shredders, primary and secondary) that can convert the biomass up to average 2–5 mm size, can effectively disrupt the complex lignocellulosic structures of biomass. Furthermore, using hydrothermal treatment at temperatures up to 90 °C for several hours to a day, followed by cooling the pulpy slurry to the mesophilic range in a mixing tank, increases the surface area and creates a pulpy biomass. This process enhances the accessibility of substrates for microbial degradation within the mixing tank. Optimizing the pulpy material after hydrothermal treatment will also help to maintain the temperature inside the reactor, which subsequently maintains the growth of the population for anaerobic microorganisms (especially methanogens). Additional pre-treatment such as the use of an optimized microbial consortium may be applied inside the mixing tank that will enhance the biogas yield. This approach may be more suitable for complex lignocellulosic materials such as rice straw, sorghum grass, etc. However, from an economical point of view large plants with this approach still need to be addressed properly. But, in the successful longer run of the plants, this technology seems more suitable as compared to existing processes.
Consequently, the breakdown of complex organic compounds becomes more efficient, accelerating the overall biogas production process. To complement the physical approach, the hypothetical system incorporates a tailored consortium of specialized microorganisms (Bacillus, Pseudomonas, and Citrobacter are the most commonly preferred) during the biological (hydrolytic) phase. The microbial community is carefully selected and optimized (with the same feedstock on which the methanogens are going to act) to efficiently decompose different types of biomasses, ensuring a diverse and robust population. Genetic engineering and synthetic biology techniques could also be employed to enhance the metabolic capabilities of the microorganisms, making them more efficient in converting specific organic compounds into biogas. Additionally, a carefully balanced nutrient (macro, micro and optimized vitamin solution) supply and pH control system would be implemented to maintain favorable conditions for the microbial community throughout the anaerobic digestion process. By closely monitoring and adjusting these parameters, the hypothetical system would create an environment conducive to increased biogas production while minimizing the risk of process inhibition. Moreover, the integration of sensors and real-time monitoring systems would enable precise control and optimization of the process. These sensors would provide valuable data on key parameters like temperature, pH, and biogas composition. Artificial intelligence algorithms could be employed to analyze this data and fine-tune the operation of the system, leading to continuous improvements in biogas yield and system efficiency. Furthermore, the hypothetical approach emphasizes the use of a diverse range of biomass feedstocks (like herbs, shrubs, etc.). This ensures a constant supply of organic material to the system and reduces dependence on specific feedstock sources. By incorporating agricultural residues, food waste, and organic by-products from various industries, the system enhances the circular economy and contributes to waste reduction.
Biological additives play a vital role in scrubbing hydrogen sulfide (H2S) and carbon dioxide (CO2) during anaerobic digestion processes. One such example is the use of sulfur-oxidizing bacteria, like Thiobacillus, which convert H2S into elemental sulfur or sulfate, reducing the odorous and corrosive effects of H2S.160,161 Additionally, methanotrophic bacteria, such as Methylococcus capsulatus, consume methane and CO2, preventing methane loss and mitigating greenhouse gas emissions.162,163 These biological additives enhance the overall efficiency of anaerobic digestion by reducing environmental hazards, ensuring stable biogas production, and promoting a sustainable waste-to-energy conversion process.
Another approach can be pretreatment of chopped biomass with boiling water followed by the addition of a hydrolytic culture and incubation, which has been expected to be a method to enhance biogas production in anaerobic digestion. The proposed pretreatment approach is relatively simple and can be implemented using conventional equipment. Boiling water is readily available and easy to use, making it feasible for small-scale biogas production units (not sure about big plants). Additionally, the optimized hydrolytic culture developed in the laboratory, provides flexibility in its application. This approach can be insightful in a number of ways such as, boiling water pretreatment can break down the lignocellulosic structure of the biomass, increasing its surface area and making it more accessible to hydrolytic enzymes. This results in higher efficiency during the subsequent hydrolysis step. By introducing an optimized hydrolytic culture, the breakdown of complex organic compounds into simpler sugars is accelerated. This improves the availability of substrates for anaerobic microorganisms, leading to increased biogas production.
Boiling water is a low-cost pretreatment method, and the hydrolytic culture can be obtained at a relatively low cost or even cultured in-house, making it economically viable. The use of an optimized hydrolytic culture and boiling water pretreatment can help in the efficient digestion of a wider range of biomass feedstocks, including agricultural residues and organic waste, thereby contributing to waste reduction and management (Fig. 4). However, there are some cons as well with this approach, such as, boiling water pretreatment requires a significant amount of energy, especially when dealing with large quantities of biomass. This could increase the overall operational cost and environmental impact of the process. The addition of a hydrolytic culture may introduce the need for additional nutrients to support microbial growth and activity. Ensuring a proper balance of nutrients is essential for optimal performance. A very important factor is that the pretreatment process and subsequent hydrolysis phase might take several days to complete. This extended time frame could affect the overall biogas production rate and may not be suitable for applications with a demand for rapid energy generation. Furthermore, managing the process requires careful control of temperature, pH, and nutrient levels to maintain favorable conditions for the hydrolytic culture. Deviations from optimal conditions could lead to reduced performance. Also, the introduction of a hydrolytic culture might alter the microbial community structure within the anaerobic digester. If not carefully managed, this could lead to potential competition among different microbial groups, impacting overall process stability. Overall, the pretreatment of chopped biomass with boiling water followed by the addition of a hydrolytic culture has both advantages and disadvantages. While it can improve biogas production efficiency and expand feedstock options, the approach's energy intensity, time consumption, and potential changes in microbial dynamics need to be carefully considered during the design and operation of the anaerobic digestion system. Based on the literature cited and analyzing the positive and negative sides of biomass pre-treatment technologies, a concept of low to high factor of characters is presented in Fig. 5.138,164,165 Comparatively, the most economical and easier method among the given options for biomass conversion depends on the specific criteria. For economic feasibility (EF) and energy consumption (EC), alkaline treatment scores high, offering efficient lignocellulosic breakdown with lower costs. However, for gas yield (GY) and an eco-friendly approach (EfA), steam explosion may be preferred, delivering a good balance between economic feasibility and environmental sustainability. This also minimizes carbon loss (CL) and has moderate maintenance costs (MC). Dilute or strong acid treatment may be ideal in a surface to volume ratio increase (ISVR) and establishment of R&D facilities (ERD), making it a viable option for specific research-focused applications. This concept of characteristic scaling may help in the selection of pre-treatment technologies. Additionally, it's crucial to conduct thorough research and optimization to assess the feasibility and benefits of this method for specific applications.
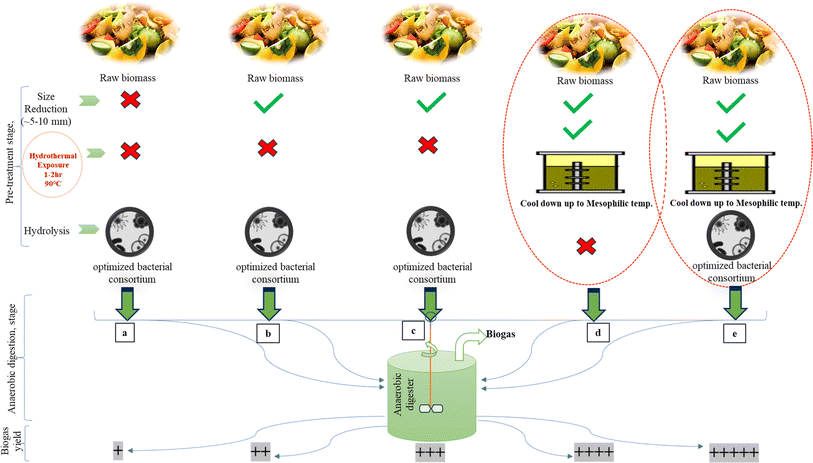 |
| Fig. 4 Hypothetical pre-treatment process for efficient biogas yield, where steam exposure can be an effective method (+ sign denotes increasing efficiency). (a) No use of mechanical and hydrothermal pre-treatment technology; (b) use of mechanical pretreatment and microbial pretreatment technology but not hydrothermal technology; (c) no use of mechanical pretreatment but use of hydrothermal and microbial treatment approach; (d) use of mechanical, hydrothermal but not microbial pre-treatement; (e) use of mechanical, hydrothermal and microbial pretreatment technology. | |
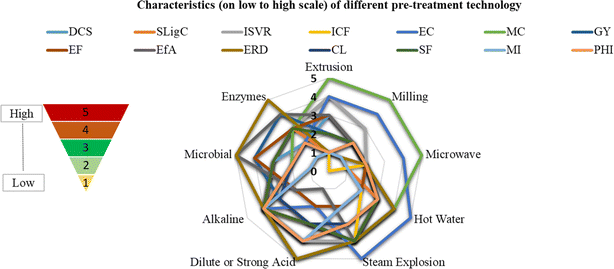 |
| Fig. 5 Conceptualization based on literature studies of different characteristics (low to high scale) in pre-treatment technologies, where DCS = destruction of cellulose; SLigC = dolubilization of ligno-hemicellulosic components; ISVR = increase in the surface to volume ratio; ICF = inhibitor compound formation; EC = energy consumption; MC = maintenance cost; GY = gas yield; EF = economical feasibility; EfA = eco-friendly approach; ERD = establishment of R&D facilities; CL = carbon loss; SF = slow/fast process; MI = methanogen inhibition; PHI = pH imbalance. | |
The benefits of this combined physical and biological approach are manifold. Firstly, it would lead to a substantial increase in biogas production efficiency compared to conventional anaerobic digestion systems. This would, in turn, reduce the land and resource requirements for biogas plants, making them more economically viable and scalable. Secondly, the approach would contribute to waste management by utilizing various organic residues that would otherwise end up in landfills, mitigating their environmental impact and reducing greenhouse gas emissions. The hypothetical combined physical and biological approach for efficient biogas yield from biomass presents a promising avenue for sustainable energy production. By integrating advanced physical preprocessing techniques with tailored microbial communities and advanced monitoring systems, this approach holds the potential to revolutionize biogas production and contribute significantly to a cleaner and more sustainable future. However, it is essential to recognize that such an approach is theoretical and would require extensive research, development, and testing before it could be practically implemented on a large scale.
8. Conclusion
The successful conversion of lignocellulosic biomass into valuable biogas and bio-CNG through anaerobic digestion (AD) requires efficient pretreatment methods. Among the various approaches, the biological pretreatment method stands out as the most economically viable and environmentally friendly option. Microbial pretreatment offers advantages such as low energy consumption and minimal pollution generation, making it a promising avenue for enhancing biomass yield suitable for the AD industry. Fungi and bacteria, along with their enzymes, play pivotal roles in this method. Fungal pretreatment, involving cellulose and lignin-degrading species like brown-rot and white-rot fungi, has shown great promise in improving biogas yield. Studies have demonstrated significant increases in methane production after fungal pretreatment of various biomass sources, such as corn silage, wheat straw, and rice straw. While fungal pretreatment may require an extended incubation period, bacterial and enzymatic pretreatments offer quicker results, making them attractive options for shortening the reaction time. Moreover, microbial consortia, consisting of diverse microorganisms living symbiotically, have shown remarkable efficiency in biomass degradation. Co-culturing different bacteria and fungi has led to higher methane yields, enabling effective ligno-hemicellulosic biomass disintegration and AD under thermophilic conditions. Chemical pretreatment using acids, alkalis, and solvents has also proven effective in disrupting the biomass structure, particularly with dilute acid treatment and alkaline pretreatment. Optimizing the concentration and duration of chemical pretreatment has led to significant increases in methane production from various feedstocks like spent tea leaves, giant reed, wheat straw, and rice straw. Physical pretreatment methods, such as mechanical size reduction, have shown potential in increasing biomass accessibility to microorganisms and enzymes, enhancing biogas production. However, due to its energy-intensive nature, further research is needed to develop more cost-effective approaches.
In conclusion, the combination of physical and biological pretreatment methods offers a promising approach to effectively pretreat ligno-hemicellulosic biomass for improved biogas production. By leveraging the potential of these pretreatment methods and utilizing anaerobic digestion or fermentation, the AD industry can contribute to a sustainable and eco-friendly energy solution while effectively converting biomass resources into valuable biogas. Continued research and development in this field will drive the advancement of pretreatment technologies and enhance the feasibility of biogas production from various ligno-hemicellulosic biomass sources.
Data availability
No primary research results, software or code have been included and no new data were generated or analysed as part of this review.
Author contributions
Rajesh Kumar Prasad: conceptualization, methodology, writing – original draft preparation, visualization. Anjali Sharma: assisting in draft preparation, and sorting tables and figures. PB Mazumder: reviewing. Anil Dhussa: supervision, reviewing and editing.
Conflicts of interest
No conflict of interest exists.
Acknowledgements
The authors humbly acknowledge Mr Utkarsh Gupta for his kind support in motivating them towards preparation of a hypothetical model for efficient biogas yield from ligno-hemicellulosic waste.
References
- Y. Li, Y. Chen and J. Wu, Enhancement of methane production in anaerobic digestion process: A review, Appl. Energy, 2019, 240, 120–137, DOI:10.1016/j.apenergy.2019.01.243.
- Y. Ulusoy, A. H. Ulukardesler, R. Arslan and Y. Tekin, Energy and emission benefits of chicken manure biogas production: a case study, Environ. Sci. Pollut. Res., 2018 DOI:10.1007/s11356-018-3466-0.
- E. K. Tetteh, M. O. Amankwa, C. Yeboah and M. O. Amankwa, Emerging carbon abatement technologies to mitigate energy-carbon footprint- a review, Cleaner Mater., 2021, 2, 100020, DOI:10.1016/j.clema.2021.100020.
- N. Scarlat, J. F. Dallemand and F. Fahl, Biogas: developments and perspectives in Europe, Renewable Energy, 2018, 129, 457–472, DOI:10.1016/j.renene.2018.03.006.
- S. Prasad, A. Singh and H. C. Joshi, Biogas production potential from biomass and waste materials in India, Renewable Sustainable Energy Rev., 2019, 100, 101–113 Search PubMed.
- B. Kumar, N. Bhardwaj, K. Agrawal, V. Chaturvedi and P. Verma, Current perspective on pretreatment technologies using lignocellulosic biomass: An emerging biorefinery concept, Fuel Process. Technol., 2020, 199, 106244, DOI:10.1016/j.fuproc.2019.106244.
- Y. Xu, Y. Lu, L. Zheng, Z. Wang and X. Dai, Perspective on enhancing the anaerobic digestion of waste activated sludge, J. Hazard. Mater., 2020, 389, 121847, DOI:10.1016/j.jhazmat.2019.121847.
- P. A. Cremonez, J. G. Teleken, T. R. Weiser Meier and H. J. Alves, Two-Stage anaerobic digestion in agro industrial waste treatment: A review, J. Environ. Manage., 2021, 281, 111854, DOI:10.1016/j.jenvman.2020.111854.
- P. Wang, H. Wang, Y. Qiu, L. Ren and B. Jiang, Microbial characteristics in anaerobic digestion process of food waste for methane production-a review, Bioresour. Technol., 2018, 248, 29–36, DOI:10.1016/j.biortech.2017.06.152.
- H. Chen, J. Liu, X. Chang, D. Chen, Y. Xue, P. Liu, H. Lin and S. Han, A review on the pretreatment of lignocellulose for high-value chemicals, Fuel Process. Technol., 2017, 160, 196–206, DOI:10.1016/j.fuproc.2016.12.007.
- A. S. Nair and N. Sivakumar, Recent advancements in pretreatment technologies of biomass to produce bioenergy, Recent Developments in Bioenergy Research, 2020, ch. 16, DOI:10.1016/B978-0-12-819597-0.00016-7.
- M. O. Daramola and A. O. Ayeni, Valorization of Biomass to Value-Added Commodities, Green Energy and Technology, Springer, 2020, 978-3-030-38031-1, DOI:10.1007/978-3-030-38032-8.
- M. Chen and P. M. Smith, The US cellulosic biofuels industry: expert views on commercialization drivers and barriers, Biomass Bioenergy, 2017, 102, 52–61, DOI:10.1016/j.biombioe.2017.05.002.
- J. K. Saini, R. Saini and L. Tewari, Lignocellulosic agriculture wastes as biomass feedstocks for second-generation bioethanol production: concepts and recent developments, 3 Biotech, 2015, 5, 337–353, DOI:10.1007/s13205-014-0246-5.
- P. Tsapekos, P. G. Kougias and I. Angelidaki, Mechanical pretreatment for increased biogas production from lignocellulosic biomass; predicting the methane yield from structural plant components, Waste Manage., 2018, 78, 903–910, DOI:10.1016/j.wasman.2018.07.017.
- S. O. Dahunsi, Mechanical pretreatment of lignocelluloses for enhanced biogas production: Methane yield prediction from biomass structural components, Bioresour. Technol., 2019, 280, 118–126, DOI:10.1016/j.biortech.2019.02.006.
- D. P. Maurya, A. Singla and S. Negi, An overview of key pretreatment processes for biological conversion of lignocellulosic biomass to bioethanol, 3 Biotech, 2015, 5, 597–609, DOI:10.1007/s13205-015-0279-4.
- A. Abraham, A. K. Mathew, H. Park, O. Choi, R. Sindhu, B. Parameswaran, A. Pandey, J. H. Park and B. I. Sanga, Pretreatment strategies for enhanced biogas
production from lignocellulosic biomass, Bioresour. Technol., 2020, 301, 122725, DOI:10.1016/j.biortech.2019.122725.
- J. Hou, X. Zhang, S. Zhang, K. Wang and Q. Zhang, Enhancement of bioethanol production by a waste biomass-based adsorbent from enzymatic hydrolysis, J. Cleaner Prod., 2021, 291, 125933, DOI:10.1016/j.jclepro.2021.125933.
- H. Zhang, L. Han and H. Dong, An insight to pretreatment, enzyme adsorption and enzymatic hydrolysis of lignocellulosic biomass: Experimental and modeling studies, Renewable Sustainable Energy Rev., 2021, 140, 110758, DOI:10.1016/j.rser.2021.110758.
- A. Devi, A. Singh, S. Bajar, D. Pant and Z. Ud Din, Ethanol from lignocellulosic biomass: An in-depth analysis of pre-treatment methods, fermentation approaches and detoxification processes, J. Environ. Chem. Eng., 2021, 9, 105798, DOI:10.1016/j.jece.2021.105798.
- J. U. Hernández-Beltrán, I. O. Hernández-De Lira, M. M. Cruz-santos, A. S. Luevanos, F. Hernández-Teran and N. Balagurusamy, Insight into pretreatment methods of lignocellulosic biomass to increase biogas yield: Current state, challenges, and opportunities, Appl. Sci., 2019, 9, 3721, DOI:10.3390/app9183721.
- A. R. Mankar, A. Pandey, A. Modak and K. K. Pant, Pretreatment of lignocellulosic biomass: A review on recent advances, Bioresour. Technol., 2021, 334, 125235, DOI:10.1016/j.biortech.2021.125235.
- S. Kassaye, K. K. Pant and S. Jain, Synergistic effect of ionic liquid and dilute sulphuric acid in the hydrolysis of microcrystalline cellulose, Fuel Process. Technol., 2016, 148, 289–294, DOI:10.1016/j.fuproc.2015.12.032.
- S. Chatterjee, S. Sharma, R. K. Prasad, S. Datta, D. Dubey, M. K. Meghvansi, M. G. Vairale and V. Veer, Cellulase enzyme-based biodegradation of cellulosic materials: An overview, South Asian J. Exp. Biol., 2015, 5, 271–282, DOI:10.38150/sajeb.5(6).
- A. Limayem and S. C. Ricke, Lignocellulosic biomass for bioethanol production: Current perspectives, potential issues and future prospects, Prog. Energy Combust. Sci., 2012, 38, 449–467, DOI:10.1016/j.pecs.2012.03.002.
- K. Rajendran, D. Edward, S. Varma.V, M. Shanmugaprakash and K. Gopalakrishnan, Updates on the Pretreatment of Lignocellulosic Feedstocks for Bioenergy Production-A Review, Biomass Convers. Biorefin., 2018, 8, 471–483, DOI:10.1007/s13399-017-0269-3.
- M. Ferdes, M. N. Dincă, G. Moiceanu, B. S. Zăbavă and G. Paraschiv, Microorganisms and Enzymes Used in the Biological Pretreatment of the Substrate to Enhance Biogas Production: A Review, Sustainability, 2020, 12, 7205, DOI:10.3390/su12177205.
- C. T. Chong, Y. V. Fan, C. T. Lee and J. J. Kleme, Post COVID-19 ENERGY sustainability and carbon emissions neutrality, Energy, 2022, 241, 122801, DOI:10.1016/j.energy.2021.122801.
- A. D. Singh, B. Gajera and A. K. Sarma, Appraising the availability of biomass residues in India and their bioenergy potential, Waste Manage., 2022, 152, 38–47, DOI:10.1016/j.wasman.2022.08.001.
- United Nations Glossary of Environment Statistics, Studies in Methods, Department for Economic and Social Information and Policy Analysis, Statistics Division, New York, NY, USA, 1997, vol. 96, Series F, 67 Search PubMed.
- S. Bhuvaneshwari, H. Hettiarachchi and J. N. Meegoda, Crop Residue Burning in India: Policy Challenges and Potential Solutions, Int. J. Environ. Res. Public Health, 2019, 16(5), 832, DOI:10.3390/ijerph16050832.
- F. O. Obi, B. O. Ugwuishiwu and J. N. Nwakaire, Agricultural Waste Concept, Generation, Utilization and Management, Nijotech, 2016, 35, 957–964, DOI:10.4314/njt.v35i4.34.
- M. Nasir, D. P. Khali, M. Jawaid, P. M. Tahir, R. Siakeng, M. Asim and T. A. Khan, Recent development in binderless fiber-board fabrication from agricultural residues: A review, Constr. Build. Mater., 2019, 211, 502–516, DOI:10.1016/j.conbuildmat.2019.03.279.
- J. Y. Choi, J. Nam, B. Y. Yun, Y. Uk Kim and S. Kim, Utilization of corn cob, an essential agricultural residue difficult to disposal: Composite board manufactured improved thermal performance using microencapsulated PCM, Ind. Crops Prod., 2022, 183, 114931, DOI:10.1016/j.indcrop.2022.114931.
- R. Malolan, R. S. Jayaraman, S. Adithya, J. Arun, K. P. Gopinath, P. S. SundarRajan, O. Nasif, W. Kim and M. Govarthanan, Anaerobic digestate water for Chlorella pyrenoidosa cultivation and employed as co-substrate with cow dung and chicken manure for methane and hydrogen production: A closed loop approach, Chemosphere, 2020, 266, 128963, DOI:10.1016/j.chemosphere.2020.128963.
- C. Sasikumar, R. Sundaresan and M. Nagaraja, et al., A review on energy generation from manure biomass, Mater. Today: Proc., 2020 DOI:10.1016/j.matpr.2020.10.832.
- https://www.pashudhanpraharee.com/waste-to-wealth-patterns-in-livestock-sector-in-india/.
- M. Nehra and S. Jain, Estimation of renewable biogas energy potential from livestock manure: A case study of India, Bioresour. Technol. Rep., 2023, 22, 101432, DOI:10.1016/j.biteb.2023.101432.
- M. K. Dey, Clean Energy, Waste to bio-CNG: The role of Indian sugar mills in the transition to cleaner fuel, Mongabay Series: Clean Energy, 2023 Search PubMed.
- Y. Li, R. Zhang, Y. He, X. Liu, C. Chen and G. Liu, Thermophilic Solid-State Anaerobic Digestion of Alkaline-Pretreated Corn Stover, Energy Fuels, 2014, 28(6), 3759–3765, DOI:10.1021/ef5005495.
- S. Bhattacharjya, A. Sahu, M. C. Manna and A. K. Patra, Potential of surplus crop residues, horticultural waste and animal excreta as a nutrient source in the central and western regions of India, Curr. Sci., 2019, 116, 1314–1323, DOI:10.18520/cs/v116/i8/1314-1323.
- T. Rangseesuriyachai, J. Boonnorat, N. Glanpracha, W. Khetkorn, P. Thiamngoen and K. Pinpatthanapong, Anaerobic co-digestion of elephant dung and biological pretreated Napier grass: Synergistic effect and kinetics of methane production, Biomass Bioenergy, 2023, 175, 106849, DOI:10.1016/j.biombioe.2023.106849.
- J. Krümpel, T. George, B. Gasston, G. Francis and A. Lemmer, Suitability of Opuntia ficus-indica (L) Mill. and Euphorbia tirucalli L. as energy crops for anaerobic digestion, J. Arid Environ., 2020, 174, 104047, DOI:10.1016/j.jaridenv.2019.104047.
- K. Sahoo and S. Mani, Economic and environmental impacts of an integrated-state anaerobic digestion system to produce compressed natural gas from organic wastes and energy crops, Renewable Sustainable Energy Rev., 2019, 115, 109354, DOI:10.1016/j.rser.2019.109354.
- S. Nanda and F. Berruti, Municipal solid waste management and landfilling technologies: a review, Environ. Chem. Lett., 2020, 19, 1433–1456, DOI:10.1007/s10311-020-01100-y.
- M. Ferdeş, B. S. Zăbavă, G. Paraschiv, M. Ionescu, M. N. Dincă and G. Moiceanu, Food waste management for Biogas Production in the context of sustainable development, Energies, 2022, 15(17), 6268, DOI:10.3390/en15176268.
- K. A. Kolekar, T. Hazra and S. N. Chakrabarty, A review on prediction of municipal solid waste generation models, Procedia Environ. Sci., 2016, 35, 238–244, DOI:10.1016/j.proenv.2016.07.087.
- K. D. Sharma and S. Jain, Municipal solid waste generation, composition, and management: the global scenario, Soc. Responsib. J., 2020, 6, 917–948, DOI:10.1108/srj-06-2019-0210.
- The World Bank, Trends in Solid Waste Management, 2020, https://datatopics.worldbank.org/what-a-waste/trends_in_solid_waste_management.html Search PubMed.
- A. R. Kalinska, K. L. Serafin and P. Manczarski, The Circular Economy and Organic Fraction of Municipal Solid Waste Recycling Strategies, Energies, 2020, 13, 4366, DOI:10.3390/en13174366.
- B. Singh, Z. Szamosi, Z. Siménfalvi and M. Rosas-Casals, Decentralized biomass for biogas production. Evaluation and potential assessment in Punjab (India), Energy Rep., 2020, 6, 1702–1714, DOI:10.1016/j.egyr.2020.06.009.
- S. Mittal, E. O. Ahlgren and P. R. Shukla, Future biogas resource potential in India: A bottom-up analysis, Renewable Energy, 2019, 141, 379–389, DOI:10.1016/j.renene.2019.03.133.
- B. Batidzirai, E. M. W. Smeets and A. P. C. Faaij, Harmonising bioenergy resource potentials - Methodological lessons from review of state-of-the-art bioenergy potential assessments Industrial Wastes, Renewable Sustainable Energy Rev., 2012, 16(9), 6598–6630, DOI:10.1016/j.rser.2012.09.002.
- B. Gunes, J. Stokes, P. Davis, C. Connolly and J. Lawler, Pre-treatments to enhance biogas yield and quality from anaerobic digestion of whiskey distillery and brewery wastes: A review, Renewable Sustainable Energy Rev., 2019, 113, 109281, DOI:10.1016/j.rser.2019.109281.
- T. R. des Santos Mathias, P. P. M. de Mello and E. F. C. Servulo, Solid wastes in brewing process: A review, J. Brew. Distill., 2014, 5(1), 1–9, DOI:10.5897/JBD2014.0043.
- S. Alexander, P. Harris and B. K. McCabe, Biogas in the suburbs: An untapped source of clean energy, J. Cleaner Prod., 2019, 215, 1025–1035, DOI:10.1016/j.jclepro.2019.01.118.
- A. C. Codony, B. Ruiz, R. R. Gil, L. A. Popartan, E. S. Clotas, M. Martín and E. Fuente, From biocollagenic waste to efficient biogas purification: Applying circular economy in the leather industry, Environ. Technol. Innovation, 2020, 21(307), 101229, DOI:10.1016/j.eti.2020.101229.
- X. Yuan, B. Wen, X. Ma, W. Zhu, X. Wang, S. Chen and Z. Cui, Enhancing the anaerobic digestion of lignocellulose of municipal solid waste using a microbial pretreatment method, Bioresour. Technol., 2014, 154, 1–9, DOI:10.1016/j.biortech.2013.11.090.
- M. O. Daramola and A. O. Ayeni, Valorization of Biomass to Value-Added Commodities Current Trends, Challenges, and Future Prospects, Green Energy and Technol., 2020, DOI:10.1007/978-3-030-38032-8, 978-3-030-38031-1 ISBN 978-3-030-38032-8 (eBook).
- A. O. Wagner, N. Lackner, M. Mutschlechner, E. M. Prem, R. Markt and P. Illmer, Biological pretreatment strategies for second-generation lignocellulosic resources to enhance biogas production, Energies, 2018, 11, 1797, DOI:10.3390/en11071797.
- B. Beig, M. Riaz, S. R. Naqvi, M. Hassan, Z. Zheng, K. Karimi, A. Pugazhendhi, A. E. Atabani and N. T. L. Chi, Current challenges and innovative developments in pretreatment of lignocellulosic residues for biofuel production: A review, Fuel, 2021, 119670, DOI:10.1016/j.fuel.2020.119670.
- D. B. Meneses, G. M. D. Oca-Vásquez and J. R. Vega-Baudrit, et al., Pretreatment methods of lignocellulosic wastes into value-added products: recent advances and possibilities, Biomass Convers. Biorefin., 2020, 12, 547–564, DOI:10.1007/s13399-020-00722-0.
- S. Rezania, B. Oryani, J. Cho, A. Talaiekhozani, F. Sabbagh, B. Hashemi, P. F. Rupani and A. A. Mohammadi, Different pretreatment technologies of lignocellulosic biomass for bioethanol production: An overview, Energy, 2020, 199, 117457, DOI:10.1016/j.energy.2020.117457.
- E. Rouches, I. Herpoël-Gimbert, J. P. Steyer and H. Carrere, Improvement of anaerobic degradation by white-rot fungi pretreatment of lignocellulosic biomass: a review, Renewable Sustainable Energy Rev., 2016, 59, 179–198, DOI:10.1016/j.rser.2015.12.317.
- M. Tišma, M. Planinić, A. Bucić-Kojić, M. Panjičko, G. D. Zupančič and B. Zelić, Corn silage fungal-based solid-state pretreatment for enhanced biogas production in anaerobic co-digestion with cow manure, Bioresour. Technol., 2018, 253, 220–226, DOI:10.1016/j.biortech.2018.01.037.
- E. Rouches, S. Zhou, M. Sergent, S. Raouche and H. Carrere, Influence of white-rot fungus Polyporus brumalis BRFM 985 culture conditions on the pretreatment efficiency for anaerobic digestion of wheat straw, Biomass Bioenergy, 2018, 110, 75–79, DOI:10.1016/j.biombioe.2018.01.018.
- V. Wyman, J. Henríquez, C. Palma and A. Carvajal, Lignocellulosic waste valorisation strategy through enzyme and biogas production, Bioresour. Technol., 2018, 247, 402–411, DOI:10.1016/j.biortech.2017.09.055.
- J. Lalak, A. Kasprzycka, D. Martyniak and J. Tys, Effect of biological pretreatment of Agropyron elongatum “BAMAR” on biogas production by anaerobic digestion, Bioresour. Technol., 2016, 200, 194–200, DOI:10.1016/j.biortech.2015.10.022.
- X. Ge, T. Matsumoto, L. M. Keith and Y. Li, Fungal pretreatment of Albizia chips for enhanced Biogas production by solid-state anaerobic digestion, Energy Fuels, 2015, 29, 200–204, DOI:10.1021/ef501922t.
- A. M. Mustafa, T. G. Poulsen and K. Sheng, Fungal pretreatment of rice straw with Pleurotus ostreatus and Trichoderma reesei to enhance methane production under solid-state anaerobic digestion, Appl. Energy, 2016, 180, 661–671, DOI:10.1016/j.apenergy.2016.07.135.
- N. Darabzadeh, Z. Hamidi-Esfahani and P. Hejazi, Optimization of cellulase production under solid-state fermentation by a new mutant strain of Trichoderma reesei, Food Sci. Nutr., 2019, 7, 572–578, DOI:10.1002/fsn3.852.
- B. Wen, X. Yuan, Q. X. Li, J. Liu, J. Ren, X. Wang and Z. Cui, Comparison and evaluation of concurrent saccharification and anaerobic digestion of Napier grass after pretreatment by three microbial consortia, Bioresour. Technol., 2014, 175, 102–111, DOI:10.1016/j.biortech.2014.10.043.
- Z. Zhang, G. Zhang, W. Li, C. Li and G. Xu, Enhanced biogas production from sorghum stem by codigestion with cow manure, Int. J. Hydrogen Energy, 2016, 41, 9153–9158, DOI:10.1016/j.ijhydene.2016.02.042.
- C. Zhong, C. Wang, F. Wang, H. Jia, P. Wei and Y. Zhao, Enhanced biogas production from wheat straw with the application of synergistic microbial consortium pretreatment, RSC Adv., 2016, 6, 60187, 10.1039/c5ra27393e.
- S. S. Ali, A. M. Mustafa, M. Kornaros, A. Manni, J. Sun and M. A. Khalil, Construction of novel microbial consortia CS-5 and BC-4 valued for the degradation of catalpa sawdust and chlorophenols simultaneously with enhancing methane production, Bioresour. Technol., 2020, 301, 122720, DOI:10.1016/j.biortech.2019.122720.
- X. P. Kong, J. Du, X. Ye, Y. Xi, H. Jin, M. Zhang and D. Guo, Enhanced methane production from wheat straw with the assistance of lignocellulolytic microbial consortium TC-5, Bioresour. Technol., 2018, 263, 33–39, DOI:10.1016/j.biortech.2018.04.079.
- S. O. Thong, A. Hniman, P. Prasertsan and T. Imai, Biohydrogen production from cassava starch processing wastewater by thermophilic mixed cultures, Int. J. Hydrogen Energy, 2011, 36(5), 3409–3416, DOI:10.1016/j.ijhydene.2010.12.053.
- B. M. Cappelletti, V. Reginatto, E. R. Amante and R. Antonio, Fermentative production of hydrogen from cassava processing wastewater by Clostridium acetobutylicum, Renewable Energy, 2011, 36(12), 3367–3372, DOI:10.1016/j.renene.2011.05.015.
- E. G. Ozbayram, Ç. Akyol, B. Ince, C. Karakoç and O. Ince, Rumen bacteria at work: Bioaugmentation strategies to enhance biogas production from cow manure, J. Appl. Microbiol., 2018, 124, 491–502, DOI:10.1111/jam.13668.
- K. Sophanodorn, Y. Unpaprom, K. Whangchai, N. Homdoung, N. Dussadee and R. Ramaraj, Environmental management and valorization of cultivated tobacco stalks by combined pretreatment for potential bioethanol production, Biomass Convers. Biorefin., 2020, 12 DOI:10.1007/s13399-020-00992-8.
- H. K. Sharma, C. Xu and W. Qin, Biological pretreatment of lignocellulosic biomass for biofuels and bioproducts: an overview, Waste Biomass Valorization, 2017, 1–17, DOI:10.1016/j.biortech.2015.08.030.
- L. A. Fdez-Guelfo, C. Alvarez-Gallego, D. Sales Marquez and L. I. Romero Garcia, The effect of different pretreatments on biomethanation kinetics of industrial organic fraction of municipal solid wastes (OFMSW), Chem. Eng. J., 2011, 171, 411–417, DOI:10.1016/j.cej.2011.03.095.
- E. Ten Brummeler and I. W. Koster, Enhancement of dry anaerobic batch digestion of the organic fraction of municipal solid waste by anaerobic pretreatment step, Biol. Wastes, 1990, 31, 199–210, DOI:10.1016/0269-7483(90)90159-P.
- Y. Ueno, M. Tatara, H. Fukui, T. Makiuchi, M. Goto and K. Sode, Production of hydrogen and methane from organic solid wastes by phase-separation of anaerobic process, Bioresour. Technol., 2007, 98, 1861–1865, DOI:10.1016/j.biortech.2006.06.017.
- C. Escamilla-Alvarado, E. Rios-Leal, M. T. Ponce-Noyola and H. M. Poggi-Varaldo, Gas biofuels from solid substrate hydrogenogenic-methanogenic fermentation of the organic fraction of municipal solid waste, Process Biochem., 2012, 47, 1572–1587, DOI:10.1016/j.procbio.2011.12.006.
- W. Xu, S. Fu, Z. Yang, J. Lu and R. Guo, Improved methane production from corn straw by microaerobic pretreatment with a pure bacteria system, Bioresour. Technol., 2018, 259, 18–23, DOI:10.1016/j.biortech.2018.02.046.
- V. B. Barua, V. V. Goud and A. S. Kalamdhad, Microbial pretreatment of water hyacinth for enhanced hydrolysis followed by biogas production, Renewable Energy, 2018, 126, 21–29, DOI:10.1016/j.renene.2018.03.028.
- T. A. Shah, C. C. Lee, W. J. Orts and R. Tabassum, Biological pretreatment of rice straw by ligninolytic Bacillus sp. strains for enhancing biogas production, Environ. Prog. Sustainable Energy, 2019, 38, 3, DOI:10.1002/ep.13036.
- C. Wang, J. Zhang, F. Hu, S. Zhang, J. Lu and S. Liu, Bio-pretreatment promote hydrolysis and acidification of oilseed rape straw: roles of fermentation broth and micro-oxygen, Bioresour. Technol., 2020, 308, 123272 CrossRef CAS PubMed.
- J. Feng, G. Liu, J. Ma, L. Zhao, Z. Yao, J. Yu, M. Li and H. Wang, Study on integrated technology of large-scale straw biogas plant and its operation effect, Trans. Chin. Soc. Agric. Eng., 2018, 34, 81–85, DOI:10.11975/j.issn.1002-6819.2018.z.013.
- Y. Cai, Z. Zheng, F. Schafer, W. Stinner, X. Yuan, H. Wang, Z. Cui and X. Wang, A review about pretreatment of lignocellulosic biomass in anaerobic digestion: Achievement and challenge in Germany and China, J. Cleaner Prod., 2021, 299, 126885, DOI:10.1016/j.jclepro.2021.126885.
- X. Yuan, B. Wen, X. Ma, W. Zhu, X. Wang, S. Chen and Z. Cui, Enhancing the anaerobic digestion of lignocellulose of municipal solid waste using a microbial pretreatment method, Bioresour. Technol., 2014, 154, 1–9, DOI:10.1016/j.biortech.2013.11.090.
- M. J. Lopez, F. Suarez-Estrella, M. C. Vargas-Garcia, J. A. Lopez-Gonzales, S. Verstichel, L. Debeer, I. Wierinck and J. Moreno, Biodelignification of agricultural and forest wastes: Effect on anaerobic digestion, Biomass Bioenergy, 2013, 58, 343–349, DOI:10.1016/j.biombioe.2013.10.021.
- J. Zhao, X. Ge, J. Vasco-Correa and Y. Li, Fungal pretreatment of unsterilized yard trimmings for enhanced methane production by solid-state anaerobic digestion, Bioresour. Technol., 2014, 158, 248–252, DOI:10.1016/j.biortech.2014.02.029.
- H. W. Kim, S. K. Han and H. S. Shin, Anaerobic co-digestion of sewage sludge and food waste using temperature-phased anaerobic digestion process, Water Sci. Technol., 2004, 50, 107–114 CrossRef CAS.
- D. Verrier, F. Roy and G. Albagnac, Two-phase methanization of solid vegetable wastes, Biol. Wastes, 1987, 22, 163–177, DOI:10.1016/0269-7483(87)90022-X.
- M. A. Voelklein, D. Rusmanis and J. D. Murphy, Increased loading rates and specific methane yields facilitated by digesting grass silage at thermophilic rather than mesophilic temperatures, Bioresour. Technol., 2016, 216, 486–493, DOI:10.1016/j.biortech.2016.05.109.
- V. N. Nkemka, B. Gilroyed, J. Yanke, R. Gruninger, D. Vedres, T. McAllister and X. Hao, Bioaugmentation with an anaerobic fungus in a two-stage process for biohydrogen and biogas production using corn silage and cattail, Bioresour. Technol., 2015, 185, 79–88, DOI:10.1016/j.biortech.2015.02.100.
- D. Yadav, L. Barbora, D. Bora, S. Mitra, L. Rangan and P. Mahanta, An assessment of duckweed as a potential lignocellulosic feedstock for biogas production, Int. Biodeterior. Biodegrad., 2017, 119, 253–259, DOI:10.1016/j.ibiod.2016.09.007.
- R. Guan, X. Li, A. C. Wachemo, H. Yuan, Y. Liu, D. Zou, X. Zuo and J. Gu, Enhancing anaerobic digestion performance and degradation of lignocellulosic components of rice straw by combined biological and chemical pretreatment, Sci. Total Environ., 2018, 637, 9–17, DOI:10.1016/j.scitotenv.2018.04.366.
- M. Koyama, S. Yamamoto, K. Ishikawa, S. Ban and T. Toda, Inhibition of anaerobic digestion by dissolved lignin derived from alkaline pre-treatment of an aquatic macrophyte, Chem. Eng. J., 2017, 311, 55–62, DOI:10.1016/j.cej.2016.11.076.
- S. S. Ali, A. E. F. Abomohrab and J. Sun, Effective bio-pretreatment of sawdust waste with a novel microbial consortium for enhanced biomethanation, Bioresour. Technol., 2017, 238, 425–432, DOI:10.1016/j.biortech.2017.03.187.
- N. Pérez-Rodríguez, D. García-Bernet and J. M. Domínguez, Extrusion and Enzymatic Hydrolysis as Pretreatments on Corn Cob for Biogas Production, Renewable Energy, 2017, 107, 597–603, DOI:10.1016/j.renene.2017.02.030.
- S. Wang, F. Li, D. Wu, P. Zhang, H. Wang, X. Tao, J. Ye and M. Nabi, Enzyme pretreatment enhancing biogas yield from corn stover: feasibility, optimization, and mechanism analysis, J. Agric. Food Chem., 2018, 66, 10026–10032, DOI:10.1021/acs.jafc.8b03086.
- K. Ziemiński, I. Romanowska and M. Kowalska, Enzymatic pretreatment of lignocellulosic wastes to improve biogas production, Waste Manage., 2012, 32, 1131–1137, DOI:10.1016/j.wasman.2012.01.016.
- H. M. E. Mashad, Biomethane and ethanol production potential of Spirulina platensis algae and enzymatically saccharified switchgrass, Biochem. Eng. J., 2015, 93, 119–127, DOI:10.1016/j.bej.2014.09.009.
- K. Ziemiński and M. Kowalska, Effect of enzymatic pretreatment on anaerobic co-digestion of sugar beet pulp silage and vinasse, Bioresour. Technol., 2015, 180, 274–280, DOI:10.1016/j.biortech.2014.12.035.
- M. Schroyen, H. Vervaeren, H. Vandepitte, S. W. H. Van Hulle and K. Raes, Effect of enzymatic pretreatment of various lignocellulosic substrates on production of phenolic compounds and biomethane potential, Bioresour. Technol., 2015, 192, 696–702, DOI:10.1016/j.biortech.2015.06.051.
- Y. Lin, J. Liang, C. Zeng, D. Wang and H. Lin, Anaerobic digestion of pulp and paper mill sludge pretreated by microbial consortium OEM1 with simultaneous degradation of lignocellulose and chlorophenols, Renewable Energy, 2017, 108, 108–115, DOI:10.1016/j.renene.2017.02.049.
- M. Rollini, C. Sambusiti, A. Musatti, E. Ficara, I. Retino and F. Malpei, Comparative performance of enzymatic and combined alkaline enzymatic pretreatments on methane production from ensiled sorghum forage, Bioprocess Biosyst. Eng., 2014, 37, 2587–2595, DOI:10.1007/s00449-014-1235-0.
- R. F. Domingues, T. Sanches, G. S. Silva, B. E. Bueno, R. Ribeiro, E. S. Kamimura, R. F. Neto and G. Tommaso, Effect of enzymatic pretreatment on the anaerobic digestion of milk fat for biogas production, Food Res. Int., 2015, 73, 26–30, DOI:10.1016/j.foodres.2015.03.027.
- M. Schroyen, H. Vervaeren, S. W. H. V. Hulle and K. Raes, Impact of enzymatic pre-treatment on corn stover degradation and biogas production, Bioresour. Technol., 2014, 173, 59–66, DOI:10.1016/j.biortech.2014.09.030.
- E. H. Koupaie, S. Dahadha, A. A. BazyarLakeh, A. Azizi and E. Elbeshbishy, Enzymatic pretreatment of lignocellulosic biomass for enhanced biomethane production – a review, J. Environ. Manage., 2019, 233, 774–784, DOI:10.1016/j.jenvman.2018.09.106.
- S. Wei, The application of biotechnology on the enhancing of biogas production from lignocellulosic waste, Appl. Microbiol. Biotechnol., 2016, 100, 9821–9836, DOI:10.1007/s00253-016-7926-5.
- E. U. Kiran, A. P. Trzcinski and Y. Liu, Enhancing the hydrolysis and methane production potential of mixed food waste by an effective enzymatic pretreatment, Bioresour. Technol., 2015, 183, 47–52, DOI:10.1016/j.biortech.2015.02.033.
- L. Thomas, B. Parameswaran and A. Pandey, Hydrolysis of pretreated rice straw by an enzyme cocktail comprising acidic xylanase from Aspergillus sp. for bioethanol production, Renewable Energy, 2016, 98, 9–15, DOI:10.1016/j.renene.2016.05.011.
- Y. Zheng, J. Zhao, F. Xu and Y. Li, Pretreatment of lignocellulosic biomass for enhanced biogas production, Prog. Energy Combust. Sci., 2014, 42, 35–53, DOI:10.1016/j.pecs.2014.01.001.
- A. Abraham, A. K. Mathew, H. Park, O. Choi, R. Sindhu, P. Binod, A. Pandey, J. H. Park and B. I. Sang, Pretreatment strategies for enhanced biogas production from lignocellulosic biomass, Bioresour. Technol., 2020, 301, 122725, DOI:10.1016/j.biortech.2019.122725.
- K. O. Olatunji, N. A. Ahmed and O. Ogunkunle, Optimization of biogas yield from lignocellulosic materials with different pretreatment methods: a review, Biotechnol. Biofuels, 2021, 14, 159, DOI:10.1186/s13068-021-02012-x.
- B. Zhu, P. Gikas, R. Zhang, J. Lord, B. Jenkins and X. Li, Characteristics and biogas production potential of municipal solid wastes pretreated with rotary drum reactor, Bioresour. Technol., 2009, 100, 1122–1129, DOI:10.1016/j.biortech.2008.08.024.
- T. Subramani and S. Ponkumar, Anaerobic digestion of aerobic pretreated organic waste, Int. J. Mod. Eng. Res., 2012, 2, 607–611 Search PubMed.
- T. L. Hansen, J. L. C. Jansen, A. Davidsson and T. H. Christensen, Effects of pre-treatment technologies on quantity and quality of source-sorted municipal organic waste for biogas recovery, Waste Manage., 2007, 27, 389–405, DOI:10.1016/j.wasman.2006.02.014.
- C. Veluchamy and A. S. Kalamdhad, Influence of Pretreatment Techniques on Anaerobic Digestion of Pulp and Paper Mill Sludge: A Review, Bioresour. Technol., 2017, 245, 1206–1219, DOI:10.1016/j.biortech.2017.08.179.
- S. K. Sharma, I. Mishra, M. P. Sharma and J. S. Saini, Effect of Particle Size on Biogas Generation from Biomass Residues, Biomass, 1988, 17, 251–263, DOI:10.1016/0144-4565(88)90107-2.
- M. A. De la Rubia, V. Fernández-Cegrí, F. Raposo and R. Borja, Influence of particle size and chemical composition on the performance and kinetics of anaerobic digestion process of sunflower oil cake in batch mode, Biochem. Eng., 2011, 58–59, 162–167, DOI:10.1016/j.bej.2011.09.010.
- P. Tsapekos, P. G. Kougias, H. Egelund, U. Larsen, J. Pedersen, P. Trénel and I. Angelidaki, Mechanical Pretreatment at Harvesting Increases the Bioenergy Output from Marginal Land Grasses, Renewable Energy, 2017, 111, 914–921, DOI:10.1016/j.renene.2017.04.061.
- C. Herrmann, M. Heiermann, C. Idler and A. Prochnow, Particle Size Reduction during Harvesting of Crop Feedstock for Biogas Production I: Effects on Ensiling Process and Methane Yields, BioEnergy Res., 2012, 5, 926–936, DOI:10.1007/s12155-012-9207-1.
- S. Sumardiono, A. B. Riyanta, H. H. Abdul Matin, T. D. Kusworo and B. Jos, Budiyono, Increasing biogas production from sugar
cane baggase by anaerobic co-digestion with animal manure, MATEC Web Conf., 2017, 101, 02014, DOI:10.1051/matecconf/201710102014.
- S. Menardo, G. Airoldi and P. Balsari, The effect of particle size and thermal pre-treatment on the methane yield of four agricultural by-products, Bioresour. Technol., 2012, 104, 708–714, DOI:10.1016/j.biortech.2011.10.061.
- R. Chandra, H. Takeuchi, T. Hasegawa and V. K. Vijay, Experimental Evaluation of Substrate’s Particle Size of Wheat and Rice Straw Biomass on Methane Production Yield, Agric. Eng. Int.: CIGR J., 2015, 17, 93–104 Search PubMed.
- P. Dell'Omo and S. La Froscia, Enhancing Anaerobic Digestion of Wheat Straw through Multistage Milling, Modell., Meas. Control, C, 2018, 79, 127–132, DOI:10.18280/mmc_c.790310.
- H. L. Thomas, S. Arnoult, H. Brancourt-Hulmel and H. Carrère, Methane Production Variability According to Miscanthus Genotype and Alkaline Pretreatments at High Solid Content, BioEnergy Res., 2019, 12, 325–337, DOI:10.1007/s12155-018-9957-5.
- P. Tsapekos, P. Kougias and I. Angelidaki, Biogas production from ensiled meadow grass; effect of mechanical pretreatments and rapid determination of substrate biodegradability via physicochemical methods, Bioresour. Technol., 2015, 182, 329–335, DOI:10.1016/j.biortech.2015.02.025.
- J. C. Frigon, P. Mehta and S. R. Guiot, Impact of Mechanical, Chemical and Enzymatic Pre-Treatments on the Methane Yield from the Anaerobic Digestion of Switchgrass, Biomass Bioenergy, 2012, 36, 1–11, DOI:10.1016/j.biombioe.2011.02.013.
- Y. Nalinga and I. Legonda, The Effect of Particles Size on Biogas, Int. J. Innov. Res. Technol. Sci., 2016, 4, 9–13 Search PubMed.
- C. Dumas, G. S. G. Damasceno, B. Abdellatif, H. Carrère, J. P. Steyer and X. Rouau, Effects of Grinding Processes on Anaerobic Digestion of Wheat Straw, Ind. Crops Prod., 2015, 74, 450–456, DOI:10.1016/j.indcrop.2015.03.043.
- S. Bolado-Rodríguez, C. Toquero, J. Martín-Juárez, R. Travaini and P. A. García-Encina, Effect of thermal, acid, alkaline and alkaline peroxide pretreatments on the biochemical methane potential and kinetics of the anaerobic digestion of wheat straw and sugarcane bagasse, Bioresour. Technol., 2016, 201, 182–190, DOI:10.1016/j.biortech.2015.11.047.
- A. Gupta and J. P. Verma, Sustainable Bio-Ethanol Production from Agro-Residues: A Review, Renewable Sustainable Energy Rev., 2015, 41, 550–567, DOI:10.1016/j.rser.2014.08.032.
- M. Germec, N. B. Bader and I. Turhan, Dilute Acid and Alkaline Pretreatment of Spent Tea Leaves to Determine the Potential of Carbon Sources, Biomass Convers. Biorefin., 2018, 8, 529–544, DOI:10.1007/s13399-018-0301-2.
- S. Kumar, K. Paritosh, N. Pareek, A. Chawade and V. Vivekanand, De-Construction of Major Indian Cereal Crop Residues through Chemical Pretreatment for Improved Biogas Production: An Overview, Renewable Sustainable Energy Rev., 2018, 90, 160–170, DOI:10.1016/j.rser.2018.03.049.
- B. C. Saha and M. A. Cotta, Comparison of Pretreatment Strategies for Enzymatic Saccharification and Fermentation of Barley Straw to Ethanol, New Biotechnol., 2010, 27, 10–16, DOI:10.1016/j.nbt.2009.10.005.
- I. Syaichurrozi, P. K. Villta, N. Nabilah and R. Rusdi, Effect of Sulfuric Acid Pretreatment on Biogas Production from Salvinia molesta, J. Environ. Chem. Eng., 2019, 7, 102857, DOI:10.1016/j.jece.2018.102857.
- D. Jiang, X. Ge, Q. Zhang and Y. Li, Comparison of Liquid Hot Water and Alkaline Pretreatments of Giant Reed for Improved Enzymatic Digestibility and Biogas Energy Production, Bioresour. Technol., 2016, 216, 60–68, DOI:10.1016/j.biortech.2016.05.052.
- K. Xihui, S. Yongming, L. Lianhua, K. Xiaoying and Y. Zhenhong, Improving Methane Production from Anaerobic Digestion of Pennisetum Hybrid by Alkaline Pretreatment, Bioresour. Technol., 2018, 255, 205–212, DOI:10.1016/j.biortech.2017.12.001.
- M. S. Romero-Güiza, R. Wahid, V. Hernández, H. Møller and B. Fernández, Improvement of Wheat Straw Anaerobic
Digestion through Alkali Pre-Treatment: Carbohydrates Bioavailability Evaluation and Economic Feasibility, Sci. Total Environ., 2017, 595, 651–659, DOI:10.1016/j.scitotenv.2017.04.006.
- Y. Gu, Y. Zhang and X. Zhou, Effect of Ca(OH)2 Pretreatment on Extruded Rice Straw Anaerobic Digestion, Bioresour. Technol., 2015, 196, 116–122, DOI:10.1016/j.biortech.2015.07.004.
- M. Lopez Torres and M. D. C. Espinosa Llorens, Effect of alkaline pretreatment on anaerobic digestion of solid wastes, Waste Manage., 2008, 28, 2229–2234, DOI:10.1016/j.wasman.2007.10.006.
- J. Gao, L. Chen, K. Yuan, H. Huang and Z. Yan, Ionic Liquid Pretreatment to Enhance the Anaerobic Digestion of Lignocellulosic Biomass, Bioresour. Technol., 2013, 150, 352–358, DOI:10.1016/j.biortech.2013.10.026.
- N. R. Katukuri, S. F. Fu, S. He, X. Xu, X. Yuan, Z. Yang and R. B. Guo, Enhanced Methane Production of Miscanthus Floridulus by Hydrogen Peroxide Pretreatment, Fuel, 2017, 199, 562–566, DOI:10.1016/j.fuel.2017.03.014.
- B. R. A. Alencar, A. L. S. Reis, R. D. F. R. De Souza, M. A. Morais, R. S. C. Menezes and E. D. Dutra, Recycling the Liquid Fraction of Alkaline Hydrogen Peroxide in the Pretreatment of Corn Stover, Bioresour. Technol., 2017, 241, 928–935, DOI:10.1016/j.biortech.2017.06.022.
- Z. Song, G. Yang, X. Liu, Z. Yan, Y. Yuan and Y. Liao, Comparison of Seven Chemical Pretreatments of Corn Straw for Improving Methane Yield by Anaerobic Digestion, PLoS One, 2014, 9, e93801, DOI:10.1371/journal.pone.0101617.
- K. Xihui, S. Yongming, L. Lianhua, K. Xiaoying and Y. Zhenhong, Improving methane production from anaerobic digestion of Pennisetum Hybrid by alkaline pretreatment, Bioresour. Technol., 2018, 255, 205–212, DOI:10.1016/j.biortech.2017.12.001.
- J. Ma, T. H. Duong, M. Smits, W. Vestraete and M. Carballa, Enhanced biomethanation of kitchen waste by different pretreatments, Bioresour. Technol., 2011, 102, 592–599, DOI:10.1016/j.biortech.2010.07.122.
- N. Chamchoi, H. Garcia and I. Angelidaki, Methane potential of household waste; batch assays determination, J. Environ. Resour., 2011, 33, 13–26 Search PubMed.
- C. Dong, J. Chen, R. Guan, X. Li and Y. Xin, Dual-Frequency Ultrasound Combined with Alkali Pretreatment of Corn Stalk for Enhanced Biogas Production, Renewable Energy, 2018, 127, 444–451, DOI:10.1016/j.renene.2018.03.088.
- F. M. Pellera and E. Gidarakos, Chemical Pretreatment of Lignocellulosic Agroindustrial Waste for Methane Production, Waste Manage., 2018, 71, 689–703, DOI:10.1016/j.wasman.2017.04.038.
- S. Sarto, R. Hildayati and I. Syaichurrozi, Effect of chemical pre-treatment using sulfuric acid on biogas production from water hyacinth and kinetics, Renewable Energy, 2019, 132, 335–350, DOI:10.1016/j.renene.2018.07.121.
- G. Mancini, S. Papirio, P. N. L. Lens and G. Esposito, Increased biogas production from wheat straw by chemical pretreatments, Renewable Energy, 2018, 119, 608–614, DOI:10.1016/j.renene.2017.12.045.
- K. Rana, N. Rana and B. Singh, Physiological and Biotechnological Aspects of Extremophiles, Chapter 10 – Applications of Sulfur Oxidizing Bacteria, 2020, pp. 131–136, DOI:10.1016/B978-0-12-818322-9.00010-1.
- P. Gao and K. Fan, Sulfur-oxidizing bacteria (SOB) and sulfate-reducing bacteria (SRB) in oil reservoir and biological control of SRB: a review, Arch. Microbiol., 2023, 205, 162, DOI:10.1007/s00203-023-03520-0.
- I. M. Mühlemeier, R. Speight and P. J. Strong, Biogas, Bioreactors and Bacterial Methane Oxidation, Methane Biocatalysis: Paving the Way to Sustainability, Springer, Chap, 2018, pp. 213–235, DOI:10.1007/978-3-319-74866-5_14.
- R. Kapoor, P. Ghosh, B. Tyagi, V. K. Vijay, V. Vijay, I. S. Thakur, H. Kamyab, D. D. Nguyen and A. Kumar, Advances in biogas valorization and utilization systems: A comprehensive review, J. Cleaner Prod., 2020, 273, 123052, DOI:10.1016/j.jclepro.2020.123052.
- S. Achinas, V. Achinas and G. J. W. Euverink, A Technological Overview of Biogas Production from Biowaste, Engineering, 2017, 3(3), 299–307, DOI:10.1016/j.eng.2017.03.002.
- M. J. B. Kabeyi and O. A. Olanrewaju, Biogas Production and Applications in the Sustainable Energy Transition, J. Energy, 2022, 8750221, DOI:10.1155/2022/8750221.
|
This journal is © The Royal Society of Chemistry 2024 |