DOI:
10.1039/D2NH00214K
(Review Article)
Nanoscale Horiz., 2022,
7, 1136-1160
Polysaccharide-based nanocomposites for biomedical applications: a critical review
Received
1st May 2022
, Accepted 27th June 2022
First published on 28th June 2022
Abstract
Polysaccharides (PSA) have taken specific position among biomaterials for advanced applications in medicine. Nevertheless, poor mechanical properties are known as the main drawback of PSA, which highlights the need for PSA modification. Nanocomposites PSA (NPSA) are a class of biomaterials widely used as biomedical platforms, but despite their importance and worldwide use, they have not been reviewed. Herein, we critically reviewed the application of NPSA by categorizing them into generic and advanced application realms. First, the application of NPSA as drug and gene delivery systems, along with their role in the field as an antibacterial platform and hemostasis agent is discussed. Then, applications of NPSA for skin, bone, nerve, and cartilage tissue engineering are highlighted, followed by cell encapsulation and more critically cancer diagnosis and treatment potentials. In particular, three features of investigations are devoted to cancer therapy, i.e., radiotherapy, immunotherapy, and photothermal therapy, are comprehensively reviewed and discussed. Since this field is at an early stage of maturity, some other aspects such as bioimaging and biosensing are reviewed in order to give an idea of potential applications of NPSA for future developments, providing support for clinical applications. It is well-documented that using nanoparticles/nanomaterials above a critical concentration brings about concerns of toxicity; thus, their effect on cellular interactions would become critical. We compared nanoparticles used in the fabrication of NPSA in terms of toxicity mechanism to shed more light on future challenging aspects of NPSA development. Indeed, the neutralization mechanisms underlying the cytotoxicity of nanomaterials, which are expected to be induced by PSA introduction, should be taken into account for future investigations.
1. Introduction
Polysaccharides (PSAs) are ubiquitous biomacromolecules, which can be found in every living organism. They contain sugar units (i.e., saccharoses) in their structure and there exist a wide spectrum of PSAs possessing monosaccharides of different type, molecular weight, and glycosidic bonds. Chitin, chitosan, cellulose, agarose, starch, hyaluronic acid, guar gum, heparin, alginate, pectin, pullulan, dextran, and cyclodextrin can be mentioned as well-known PSAs with broad biomedical applications.1 They are green alternatives to synthetic polymers and in combination with nanofiller, nanoparticles, and nanosheets, they can be utilized instead of different synthetic polymer composites. There exist a wide range of PSAs with low or high molecular weight, linear or branched as well as mono- or multifunctional groups, which mainly benefit from hydrophilicity, no/low level of toxicity, and non-immunogenicity, which amplify their applications.1 Noteworthily, in addition to metabolic conformity and enzyme-triggered degradation, these biomolecules are more stable in comparison to nucleic acids or proteins. Different types of PSAs are utilized for biomedical applications, food additives/packaging, agricultural purposes, and water treatment.2 For instance, sulfated PSAs have a unique ability to mimic the extracellular matrix (ECM) environment (i.e., heparan sulfate molecules in the ECM), leading to an enhancement in the alkaline phosphatase activity for bone regeneration3 or nerve tissue engineering, where the biodegradation of PSA plays an important role after replacement surgery.4
The development of PSA green nanocomposites has been the subject of many papers. PSA nanocomposites containing bionanomaterials (e.g., silver, gold or titanium oxide, especially in the form of nanofibers/nanowire or nanocrystal), are promising scaffolds possessing a Young's modulus of 100–200 GPa and a higher specific surface area (hundreds of m2 g−1).5,6 Moreover, PSA-based fibrous scaffolds more closely mimic the heterogeneity of the native ECM. PSAs with micro and nanoscale fibrous structures can also be fabricated through dry, wet, melt, gel, reaction, or electrospinning processes.7 The resulting nanocomposites fibers can be used as constructing components of tissue scaffolds (e.g., fiber-reinforced hydrogel) or they may be the only constituent of fibrous scaffolds. PSA-based nanocomposites can be applied as biomedical platforms through a two-stage approach. In the first step, nanomaterials are blended with PSAs (as matrix) with appropriate distribution and dispersion, e.g., via solution mixing. In the second stage, the prepared nanocomposite undergoes a process, such as electrospinning, to obtain a proper shape (e.g., electrospun fibers). Fig. 1 illustrates the most well-known methods used for shaping PSA nanocomposites.8,9 Among the above approaches, electrospinning is the most practical and cost-effective because of its ability to mimic the fibrous structure of natural ECM, which affects the cells’ biological behaviors.10–12
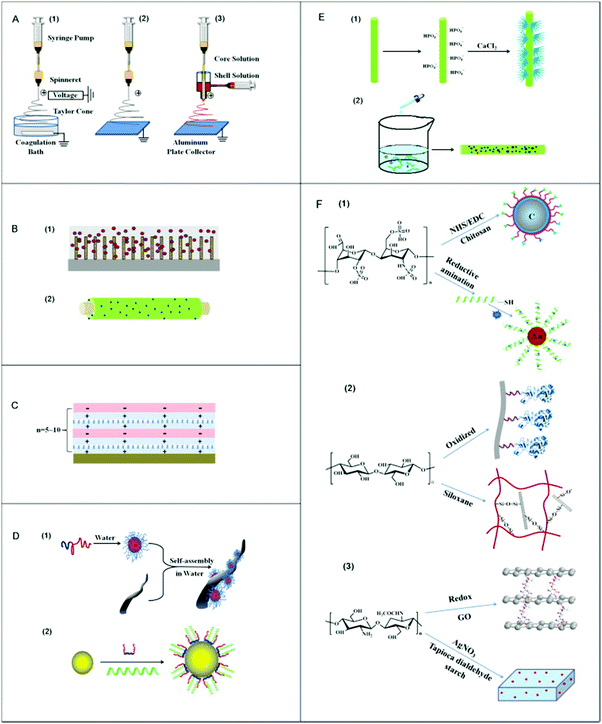 |
| Fig. 1 Schematic illustration of the methods used for nanocomposite fabrication. (A) Electrospinning process, which has utilized (1) wet-wet, (2) wet-dry, and (3) co-axial method, respectively, (B) film coating procedure, (1) to convert cellulose from the sphere form to a cylinder on silicon wafer surface, and (2) lactose modification with chitosan and enclosed Ag nanoparticles, (C) layer-by-layer deposition method, (D) colloidal assembly, (1) self-assembly of the block polymer on a fiber from the anionic PSA core/shell structure, and (2) assembly of poly(lactide-co-glycolide) within the water (E) in situ nanoparticles preparation procedure, (1) phosphorylation of cellulose fibers and growth of hydroxyapatite nanocrystals, and (2) cellulose nanofibers within sodium borohydride (NaBH4) solution with the addition of silver nitrate (AgNO3) as well as (F) covalent coupling, (1) coating of poly(methyl methacrylate) with carbon and heparin linked with chitosan, which is fluorescently labeled, and (2) coupling of cellulose oxidized lysostaphin or siloxane 3-grafting of chitosan with graphene oxide (GO) nanosheets.8 | |
Although PSA-based nanocomposites used for biomedical purposes are becoming increasingly widespread, there is no review paper that summarizes and interprets the available reports on their application in biomedical engineering. This paper aims to summarize and discuss numerous studies that have utilized bio-nanocomposites with PSA as a matrix or PSA-based nanostructure additives. A number of review papers and books have assorted PSA sub-families,13 their origins,14 their general applications (no focus on biomedical ones),15 applications of PSAs (not their nanocomposites) in tissue engineering (no other biomedical fields16,17), methods of preparation and functionalization,8,18 details about their pharmacogenomics,19 different possible bio-additives,20 the electrically conductive PSAs, and existing challenges,4 and eventually their use in very few branches of biomedical engineering.21 However, none of them have discussed the existing opportunities and challenges in all biomedical fields, ranging from delivery systems (both gene and drug), tissue engineering (skin, bone, nerve, and cartilage), and cancer treatment (radiotherapy, immunotherapy, and phototherapy) to bioimaging as well as biosensors. It is attempted to comprehensively review and discuss PSA nanocomposites in the quest for developing scaffolds for biomedical application. Moreover, a concise glimpse at the fabrication methodologies and discussion about existing challenges (such as nonmaterial toxicity) and the future ahead of this field of research is taken into account. It is believed that such a well-organized review would help researchers working in the field in taking next steps toward a profound understanding of the limitations and possible remedies, all of which would support the future horizon.
2. Polysaccharide family
PSAs can be found in various types and with different properties. There are a variety of PSAs with different molecular weights, functionalities (e.g., hydroxyl, amine, and carboxyl), and properties (e.g., hydrophilic or hydrophobic). The main motivation for the development of PSA-based nanocomposites roots in their relatively poor mechanical properties, which has limited their potential applications. The utilization of PSAs in combinations with nanoscale materials enhances their properties, mechanically (such as the addition of graphene nanosheets), and in some cases, biologically (such as adding silver nanoparticles for improving the antibacterial performance).4,22 On the other hand, it may induce other functions, e.g., electrical conductivity (such as the addition of carbon nanotubes), to the base PSAs.
Generally speaking, alginate with biocompatible properties and ionic crosslinking has numerous biomedical benefits, such as cell delivery, 3D and 4D bioprinting, as well as 3D cell culturing.12,23,24 In addition, reports have indicated that alginate proposes a strong intrinsic anticoagulant characteristic.25 Pectin can be obtained from plant cell walls and pullulan with perfect water solubility and nontoxicity, which is typically isolated from Aureobasidium.22,25 Dextran and hyaluronic acid with high water solubility can be mentioned as excellent candidates for food and medical applications.25 Gums are reported as cheap and easy operable materials, which are well known as water treatment agents.26 Cellulose and chitosan with biodegradability, biocompatibility, good mechanical properties, and facile chemical modifications are captivated by developers in medicine, food and paper industries, and water treatment.
Some PSAs such as starch have weak mechanical characteristics. For instance, starch suffers from poor mechanical properties when it is moist and fragile when it is dry.27 Physical and chemical crosslinking are well-known strategies to enhance the mechanical properties of PSA. Physical interactions are generally weaker than chemical crosslinking but can induce dynamic features such as self-healing capability. It is worth mentioning that the pretreatment, such as chemical modifications, may be necessary prior to crosslinking. Chemical crosslinking also affects the cytotoxicity and rheological behavior (e.g., gelation time, gel strength, swelling ratio, and absolute viscosity). For instance, the gelation time and gel strength of dextran hydrogels can be controlled by altering the chain length of the chemical crosslinker agent.28,29 On the other hand, the incorporation of nanomaterials with high mechanical properties is another general strategy to enhance the mechanical properties of starch. From a molecular view, interfacial interactions between nanomaterials and PSA are key factors for property enhancement. Table 1 shows different PSAs sub-families and their properties, and Table 2 summarizes PSAs extraction methods associated with its advantages and disadvantages, the purification methods, as well as PSAs bioactivities.
Table 1 A summary of the PSAs and their properties together with a discussion about their benefits/consequences
PSA family |
Properties and applications |
Ref. |
Alginate |
Biocompatible/ionic crosslinking/cell delivery and 3D culture applications/strong anticoagulant properties |
25, 30 and 31
|
Pectin |
Achieved from plant cell walls/nontoxicity/biocompatibility |
22, 25 and 30
|
Pullulan |
Water solubility/nontoxicity/achieved from Aureobasidium |
22, 25 and 30
|
Dextran and hyaluronic acid |
Great biocompatibility/great water solubility |
32
|
Gums |
A cheap biomaterial/easily operable/usable for water treatment agent/biocompatibility |
26 and 32
|
Cellulose |
Biodegradability/biocompatibility/good mechanical properties/facile chemical modifications |
33
|
Starch |
Poor mechanical properties/fragile/biodegradability/biocompatibility |
34
|
Chitin/chitosan |
Positive charge/solubility in acidic aqueous/pH responsive/electric sensitivity/magnetic sensitivity/biocompatibility |
35
|
Table 2 A summary of extraction and purification methods for PSAs as well as their bioactivities
Extraction methods |
Advantage |
Disadvantage |
Ref. |
Hot water extraction |
Facile operation, widely used, and cost-effective |
Low quality (in terms of purity), very time consuming, and too repetitive |
36
|
Acid–base extraction |
Short processing and fast rate |
Too selective and hard controlling over the acid concentration |
37
|
Enzyme extraction |
High quality and purity, applicable in mild condition, and fast speed processing |
Enzymatic degradation byproducts, very high costs, high concentration of the required enzymes |
38
|
Ultrasonic extraction |
Widely used, facile, cost-effective, and low level of energy consumption |
Possible alteration of PSA structural properties |
39
|
Ultrahigh pressure extraction |
Time saving and high yield |
Not appropriate for the PSAs with a high level of starch (carbohydrate) |
40
|
Microwave extraction |
Can be used for the thermally unstable PSAs and the most time-saving technique |
No possibility of large-scale production and possible degradation of PSAs under extraction process |
41
|
Supercritical fluid extraction |
No residue and a possibility to reuse the solvent |
Complexity and high expenses |
42
|
Purification |
Purification |
Ref. |
Gel permeation chromatography |
Anion Exchange chromatography |
43
|
For PSAs with different molecular weights |
For neutral and acidic PSAs |
44
|
Bioactivities |
Bioactivities |
Bioactivities |
Antitumor activity |
Anti-inflammatory activity |
Immunomodulatory activity |
45
|
46
|
47
|
Hypolipidemic activity |
Hypoglycemic activity |
Vascular protective activity |
48
|
49
|
50
|
Antithrombotic activity |
Antiobesity activity |
Ant arteriosclerosis activity |
51
|
52
|
53
|
Cryoprotective activity |
Renoprotective activity |
Regulating gut microbiota |
54
|
55
|
56
|
Antiviral activity |
Neuroprotective activity |
Antibacterial activity |
57
|
58
|
59
|
Prebiotic activity |
Antioxidant activity |
Whitening activity |
60
|
61
|
62
|
2.1. Polysaccharide nanocomposites
A variety of synthetic polymers and biopolymers are ecofriendly and non-toxic. However, ‘nanotechnology’ leads them to another dimension for performance.63 When it comes to nanocomposites, the addressed properties are of extraordinarily higher performance for appropriate tissue engineering, increasing drug release stability, gene delivery performance, as well as antibacterial wound dressings.64–66 The incorporation of nanoscale materials with high specific surface area and favorable inherent properties enhances the functionalities of PSA-based matrixes and enlarges their application window. For example, PSA nanocomposites containing carbon nanotubes can not only can be used as conductive hydrogels for nerve or cardiac tissue engineering but also reveal improved thermal stability. The combination of physicochemical properties of each component results in a nanocomposite with tunable properties, which are desirable for a specific application.67 It is noteworthy to mention that the utilization of these systems as drug delivery platforms is as sophisticated as it can be used for contact lenses to release desired drug within the eyes due to the high stability of the drug release profile.68–70
The properties of the base PSA matrix can be enhanced by blending with synthetic polymers, which generally possesses higher mechanical properties.71 For example, chitosan was blended with poly(ε-caprolactone) prior to embedding with zinc-doped hydroxyapatite nanoparticles (Zn-HA). The obtained PSA-based nanocomposite benefits from biodegradation, appropriate cytocompatibility, bioactivity, as well as mechanical properties (elastic modulus (3 times more than the controlled group) and tensile strength (1.5 times more than the controlled group)).72,73 Some of the proposed biodegradable nanocomposite scaffolds serve as excellent supports for cell attachment, spreading, growth, and ECM secretion. These scaffolds have such high biological compatibility that they can form cell sheets only within 14 days and the cells continue their metabolic activity up to 21 days.74 On the other hand, blends of PSAs with proteins (as matrix) before the addition of nanomaterials can be used to adjust the degradation profile, thermal stability, biointerface characteristics, and release behavior of PSAs. Some suggested a nanocomposite scaffold from chitosan nanoparticles and gelatin (the added protein) using the gelation method. They loaded the basic fibroblast growth factors (bFGFs) within the scaffold and their results indicated that the sustained release of bFGFs is possible. Such a system is invaluable for use as a tissue regeneration platform that requires vascularization such as wound healing platforms.75 Similarly, Shokrani et al. reported that the combination of gelatin and chitosan is a strong natural platform for improving the angiogenesis density and tissue regeneration.76 In some studies, PSAs have been utilized as the particles to enhance the cytocompatibility of graphene oxide nanostructures (GO) and to decrease their toxicity. For instance, a nanocomposite made of GO nanostructures/hyaluronan/chitosan with great cell adhesion and protein absorption properties has been reported by Andreeva et al.77 Their results revealed that in addition to the unique thermal and mechanical properties, a range of platelet adhesion quality and thrombosis (74%) can be obtained using the described platform.77 In addition, the incorporation of some PSAs with silver (Ag) nanoparticles provides antibacterial properties to the nanocomposite scaffolds. For example, Chitlac (as a kind of PSA film) combined with Ag nanoparticles destroys P. aeruginosa and S. aureus bacteria for tooth tissue engineering applications.78 Remarkably, halloysite nanotubes (HNTs) and silica nanoparticles are promising nanostructures for wound healing applications owing to their exceptional mechanical stability as well as hemostatic features.79,80 A combination of HNTs and chitosan not only hinders bleeding but also supports faster reepithelization.81
2.2. Stimuli responsive polysaccharides
Different stimuli have been introduced to obtain the desired response from smart PSA nanocomposites. Almost all PSAs are responsive to pH and ions. However, there are some differences in responsiveness of PSAs in terms of their chemical structure and functional groups. For instance, chitosan is specifically responsive to electrical fields and glucose, which is a necessity for developing biosensors for diabetic patients. The mechanism of insulin delivery using glucose responsive chitosan is shown in Fig. 2.35,82 On the other hand, alginate is responsive to light and surfactants. Table 3 summarizes the most frequent stimuli responsive PSAs. Many smart PSA nanocomposites may respond to physical stimuli such as temperature, light, electricity, magnetic field, or even pressure, while some others may respond to chemical species such as reactive oxygen species (ROS), redox species (e.g., glutathione), glucose, enzymes, and some ions (e.g., calcium). Among the chemical stimulus, glucose is the most prevalent one (Fig. 2). Notably, the incorporation of nanoparticles into PSAs may considerably change or worsen their response ability/mechanism.
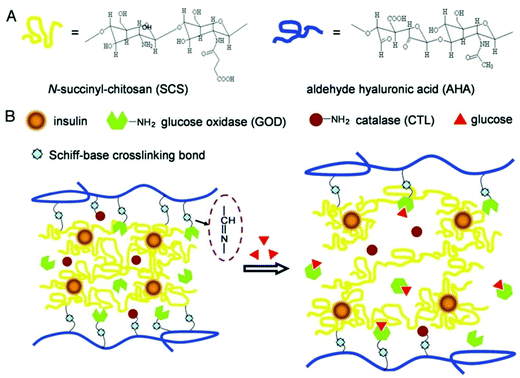 |
| Fig. 2 (A) The chemical structure of chitosan and hyaluronic acid-based composite. (B) Mechanism of glucose-responsive PSA hydrogel for the delivery of insulin.83 | |
Table 3 A summary of the more frequently used stimuli-responsive PSAs, stimuli, their resources, and molecular weights
Polysaccharide |
Molecular weight ranges (Da) |
Abundances |
Responsiveness |
Ref. |
Chitosan |
3.8 × 103 to 9 × 106 |
Exoskeleton of crabs and shrimps, and cell walls of fungi |
Ions, pH, electrical field, glucose |
84
|
Alginate |
104 to 6 × 106 |
Cell walls of algae |
Ions, pH, electrical field, surfactants, light |
85
|
Heparin |
3 × 103 to 3 × 104 |
Mucosal tissues |
Ions, pH, redox |
86
|
Hyaluronic acid |
5 × 103 to 2 × 107 |
Extracellular matrix, epithelial and neural tissues |
Ions, pH, electrical field, light, temperature, and redox |
87
|
Cellulose |
3 × 103 to 4 × 106 |
Microbes |
Ions, pH, and temperature |
88
|
Some PSAs such as chitosan have basic amine groups, which results in pH response behavior. The pH responsiveness of chitosan-based nanocomposites is due to the protonation of amine groups under acidic conditions.89,90 Interestingly, the protonation of amine groups is the responsible driving force for its solubility in acidic aqueous media. In some cases, two or more different stimuli are introduced to take advantages of their synergistic effects. For instance, chitosan indicates both pH and electric sensitivity within an electric field, which enables one to make a dual responsive biomaterial.35 To add magnetic sensitivity to the scaffold, the addition of magnetic nanoparticles to PSAs (such as cellulose) seems to be a promising solution. It can be utilized for the delivery of therapeutic drugs into a specific tissue or organ by directing carriers with the aid of a magnetic field.91 Moreover, according to Liu et al., the addition of phenylboronic acid is one way to sensitize the scaffold to glucose.92 Akbari et al. reported that the combination of carboxymethyl cellulose and zinc oxide nanoparticles yields a pH-sensitive nanocomposite, which is the result of synergistic effects of cellulose responsiveness as well as zinc oxide sensitivity.93,94 Moreover, the incorporation of acrylic acid and N-isopropyl acrylamide (NIPAM) with chitosan results in dual responsive scaffolds that respond to both pH and temperature fluctuations. This smart system has been utilized for doxorubicin (DOX) release in cancer therapy.95 The collaboration of chitosan and (3-aminopropyl)triethoxysilane is another smart pH/temperature-responsive platform for curcumin delivery in cancer treatment applications. From the molecular view, when the pH or temperature increases, a higher swelling ratio leads to water penetration into the structure, which can finally affect the drug release pattern.96 A dual responsive nanocomposite platform based on aminated nanodextran and carboxylate nanocellulose containing GO nanoplatelets was used for pH and near-infrared (NIR) sensitive delivery of curcumin. To the best of our knowledge, such a system can be an inspiring option for bioimaging applications (because of being sensitive to NIR).97 Generally speaking, dual responsive nanocomposites (especially hydrogel nanocomposites) can be chiefly assorted to pH/temperature sensitive systems, pH/redox platforms, pH/electric field scaffolds, temperature/light systems, as well as pH/glucose nanocomposites.35
3. Generic applications of polysaccharides in biomedical engineering
3.1. Drug delivery
Drug delivery systems (DDSs) that target specific tissue or cells and release therapeutics in a sustained and controlled manner have attracted much interest in the biomedical fields.16,98,99 Targeted DDSs for cancers enable to carry drug molecules to tumor cells, while ignoring healthy cells/tissue such that adverse side effects are minimized. Various PSAs have been utilized as constituents of delivery platforms formulation since they benefit from advantages such as biocompatibility and low immunogenicity.100–102 On the other hand, the nanotechnology strategies (such as making different nanostructures including nanoparticles, nanosheets, as well as nanofillers) have always played crucial roles in the development of DDSs.103 The development of biocompatible and stimuli-responsive PSA-based nanocomposites enable both passive and active targeting drug delivery benefiting from small dimensions and responsiveness.104,105
On the other hand, PSA-based nanocomposites (e.g., nanogels) can be decorated with targeting ligands to target specific receptors on the cell membrane (e.g., CD44, which is a cell surface glycoprotein106,107). Remarkably, chemical modification strategies provide robust tools for the conjugation of targeting moieties such as nanobodies.108 In the PSA-based nanocomposites, the presence of accessible reactive functional groups, e.g., amines, enables facile and effective chemical functionalization. Brain targeting drug delivery is a good example of this case.109 Although there are plenty of barriers in terms of biological or physical obstructions, nano-based DDSs can circumvent many of these barriers. These systems exhibit high capacity to carry various drugs (e.g., anticancer drugs and Alzheimer's medications) to the brain. Interestingly, they not only support sustained drug release, which brings about a stable release profile, but also decreases the level of toxicity of administrated drug, especially when the drug has low water solubility.79,110–112
PSAs are great options among the existing biomaterials and their combinations with graphene-derivatives (as nanocomposites) can be a perfect recommendation to reduce the toxicity, increase the targetability, and accelerate their release efficacy. Moreover, this class of carriers can be responsive toward external stimuli such as NIR light or internal stimuli such as pH and some ions.113 The combination of HNTs with PSA is a novel platform to prepare nanocomposites for drug delivery aims, which is due to their perfect supermolecular interactions and mesoscopic features. Moreover, miscellaneous active molecules can be loaded within the HNTs and their ultimate characteristics can also be controlled by fabrication methods and modification strategies.79 In addition, PSAs can be a nanoscale component of another matrix. Noteworthily, the final release rate is closely related to the biomaterial's molecular weight, constituents' ratios, crosslinker type, crosslinker concentration, as well as drug curing time.114 The applications of PSA-based delivery systems can be further expanded to theragnostic platforms that enable both delivery of therapeutics and imaging of internal body parts.115
3.2. Gene delivery
Gene therapy is a novel treatment methodology for various diseases such as osteoarthritis or even cancer. This is why devising safe, practical, and targeted gene carriers is crucial in biomedical engineering. PSA-based nanocomposites are among the existing options with exceptional ability in marker expressions. For instance, they present a great media to support the expression of early and late bone markers with bone marrow stem cells even in an environment that lacks osteo-inducive factors.116–119 As another instance, Patnaik et al. proposed an interesting delivery system for siRNAs delivery, which consisted of polyethylenimine (PEI) nanoparticles, alginate, alginic acid, and polyethylene glycol (PEG). The PEG shell enhances the blood circulation and enables the DDS to evade the immune cells, while the cationic PEI nanoparticles enable gene transduction across the plasma membrane and alginate increases the compatibility of the whole system. Their results revealed that the flexible structure with a positive charge can easily interact with the negative charge of the cells' membrane.120 However, the possibility of enzymatic degradation in this creative system can be alarming. After several years, further studies have demonstrated that the surface coating of dopamine will postpone enzymatic degradation, which is very important in delivery systems, especially the sustained ones (that need to follow prolonged release pattern).121 In addition, chitosan-g-polyethylene glycol nanocomposite is suggested for the sustained delivery of genes with a highly optimized circulation time in a rat model.122 The utilization of hyaluronic acid and chitosan nanocomposite is a great platform for plasmid DNA delivery to the cornea with great cytocompatibility toward corneal epithelial cells.123–127 As another example of gene delivery using PSAs, Kashkouli et al. took advantage of chitosan nanocarriers, organosilane-modified 5-amino-1H-tetrazol, and Fe3O4 combination for plasmid delivery. Their results indicated an increase in the gene expression for the HEK-293T cell line (human embryonic kidney cells) for cancer therapy. Their assessments revealed that the percentage of transfected cells within the magnetic field is three times more that the transfection without inducing any magnetic field (a 45% transection in magnetic field compared to 15% without field).128 Likewise, different types of chitosan-based nanostructures as a non-viral gene delivery vector have been investigated. In a typical study, pCRISPR (a family of DNA sequences) was selected as the gene, and different combinations of calcium nanoparticles along with chitosan were selected as the promising non-viral gene delivery vectors (Fig. 3). The results showed that after the addition of different weight ratios of chitosan to the calcium and calcium phosphate nanoparticles, the zeta potential increased considerably and led to considerable interactions with the pCRISPR.129 Elsewhere, they demonstrated that by the addition of these PSAs to the nanostructures (nanocarriers), even highly toxic nanocarriers such as (ZnO)x(GaN)1−x, the relative cell viability as well as the biocompatibility and biodegradability could be increased considerably on different cell lines, leading to considerable and successful drug/gene delivery to the targeted tissues/cells.130Table 4 has gathered various nanocomposite scaffolds that endorse drug/gene delivery.
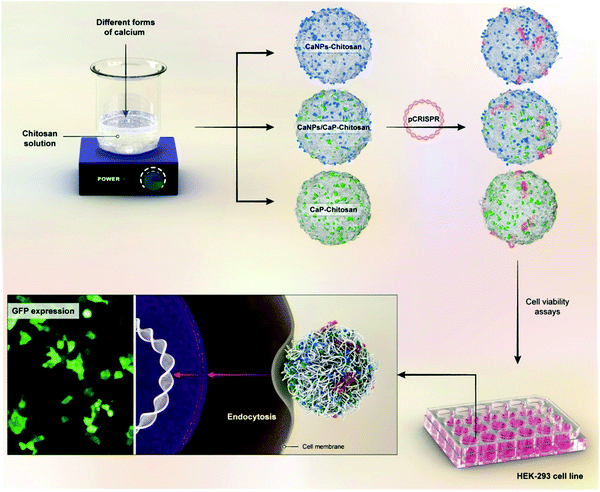 |
| Fig. 3 A schematic illustrations on the preparation of nanoblends of chitosan and different calcium forms for pCRISPR delivery on the HEK-293 cell lines.129 | |
Table 4 A summary of nanocomposite scaffolds based on their based PSAs, other constituents, and their outstanding features for drug/gene delivery
Base polysaccharide |
Nano additive(s) |
Payload |
Comment |
Ref. |
Chitosan |
Aminopropylsilane nanoparticles |
Metformin |
An abrupt release within 22 h and the rest of that in 15 days |
131
|
Zinc oxide nanoparticles |
Naproxen |
Great cytocompatibility for human dermal fibroblast cells with antibacterial activities |
132
|
Solid lipid nanoparticles |
Atorvastatin |
98% membrane degradation percentage after 5 days/75–79% percentage of drug release |
133
|
GO sheets |
5-FU |
A great option for pH sensitive drug release |
134
|
Cellulose |
HNTs |
Vanillin |
A great option for antimicrobial or flavor release even in the food industry or biomedical application |
135
|
Copper(II) oxide nanoparticles |
NPX |
Excellent antibacterial properties and great swelling behavior for colon drug delivery |
136
|
Starch |
Sericin nanofibers |
Doxorubicin |
High capacity of drug encapsulation/great stability and biodegradability |
137
|
Hyaluronic acid |
Polyethylene glycol nanoparticles |
Plasmid |
Slight increase of liver toxicity/24–96 h release time without polyethylene glycol |
122
|
Chitosan |
Fe3O4 nanoparticles |
Plasmid |
An increase in gene expression for HEK-293T cell line for cancer therapy |
128
|
3.3. Polysaccharide nanocomposites as antibacterial platforms
The preparation of antibacterial and antimicrobial platforms has numerous merits not only in biomedical applications but also in the food industry. Antibacterial scaffolds can be utilized for different applications ranging from hemostasis agents and wound dressings138 to bone tissue engineering139 and food packaging.140 PSA-based nanocomposites with excellent antibacterial activities toward Escherichia coli141,142 and Staphylococcus aureus138,143 play an essential role in this field. However, their nanocomposites are preferred because of the fact that some nanomaterials have strong antibacterial properties by themselves (such as silver and zinc oxide nanoparticles) and form a great platform with PSAs.144–147 For example, Anugrah et al. reported that the combination of zinc oxide nanoparticles with PSAs can accelerate the food shelf time by hindering the microbial activities. According to them, zinc nanoparticles increase the oxidative stress, which harms the bacteria cell wall. Synergistically, Zn2+ release can penetrate the bacterial cell membrane and endanger their life.148Fig. 4 shows the mechanism of action of antibacterial activities of zinc oxide and a PSA-based stimuli-responsive nanocomposite fabrication process.136,148
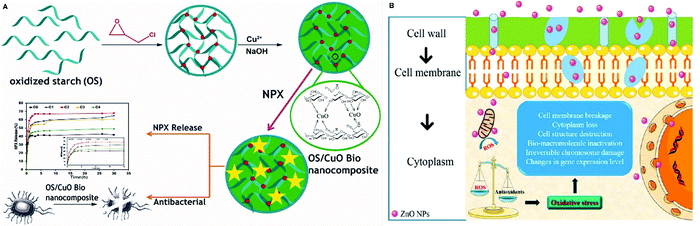 |
| Fig. 4 Illustration of antibacterial mechanisms of two different scaffolds. (A) A scaffold used for drug delivery with great antibacterial properties consisting of oxidized starch and copper(II) oxide. (B) The mechanism of action of antibacterial properties for zinc oxide.136,148 | |
Noteworthily, nanohydroxyapatite, chitosan, and Trigonella foenum-graecum can form a nanocomposite (using co-precipitation method) with a very low hemolysis (below 4%), great compressive strength (6.7 MPa), excellent compressive modulus (100 MPa), as well as high antibacterial activities.149 Interestingly, Lu et al. demonstrated that hydroxypropyl chitosan has strong biocompatibility and antibacterial performance. However, the assigned biomaterial was not able to easily show the desired mechanical properties. Hence, the combination of nanohydroxyapatite with hydroxypropyl chitosan and genipin (as a crosslinking agent) brought about an improvement in the compressive strength (from 19.1 kPa to 52.8 kPa with increasing amount of nanohydroxyapatite) and capacity of fluorescence emission.150 Moreover, to induce antibacterial activity toward Staphylococcus aureus and Escherichia coli using the chitosan-based nanocomposite, polyethylene oxide nanofibers were loaded with an optimized amount of zeolitic imidazolate framework-8 nanoparticles.138,143 As mentioned above, when Ag nanoparticles are constituents of a nanocomposite, a perfect level of antibacterial activities is observed. For instance, Shariatinia et al. demonstrated that a promising nanocomposite from chitosan, phosphor amide, as well as Ag nanoparticles will leave a crucial antibacterial trace on two kinds of Gram positive bacteria and two kinds of Gram negative bacteria.151Table 5 summarizes different kinds of scaffolds with PSA and their constituents, fabrication method, and their antibacterial features.
Table 5 A summary of different kinds of antibacterial scaffolds with PSA based and their constituents, fabrication methods, and their features
Base polysaccharide |
Nanoadditive(s) |
Fabrication method |
Main findings |
Ref. |
Chitosan |
Nanohydroxyapatite |
Coprecipitation method |
Great capacity of water and protein absorption via the porous structure/excellent antibacterial properties/good choice for bone engineering |
149
|
Chitosan |
Nanohydroxyapatite |
Coprecipitation |
High antibacterial activity due to presence of hydroxypropyl chitosan/good swelling ratio as well as fluorescence emission (as a unique feature) |
150
|
Chitosan |
Polyethylene oxide nanofiber mats/zeolitic imidazolate framework-8 nanoparticles |
Electrospinning technique |
Antimicrobial activity/antibacterial activity against Staphylococcus aureus and Escherichia coli |
143
|
Chitosan |
Polyethylene oxide nanofibrous mats/bioactive silver nanoparticles |
Electrospinning technique |
Antimicrobial activity/antibacterial activity against Staphylococcus aureus and Escherichia coli/great option for wound dressing applications |
138
|
Chitosan |
Silver nanoparticles |
Citrate reduction method |
Excellent antibacterial activities against two kinds of Gram+ and two kinds of Gram− bacteria and the Ag nanoparticles brings about higher antibacterial property |
151
|
Gum |
Nanohydroxyapatite |
Coprecipitation approach |
Excellent mechanical properties (such as compressive strength and compressive modulus)/great capacity of protein adsorption with nice ability to swell/powerful antibacterial properties/good choice for bone tissue engineering |
152
|
3.4. Polysaccharide nanocomposites as hemostasis agents
PSA-based nanocomposites are repetitively used as hemostatic platforms.153 Great biocompatibility, intrinsic antibacterial features (chitosan), and natural hemostasis performance (chitosan, cellulose, oxidized cellulose, carboxymethyl cellulose) are the reasons behind the use of PSAs as blood-clotting biomaterials.154 Importantly, they must be able to be excreted from the body by natural mechanisms (such as enzymatic degradation), especially if injected into the internal parts of body (such as liver155). Thus, the high biodegradability of PSA-based nanocomposites is of a great importance. The addition of nanomaterials not only gives antibacterial and drug delivery properties to such systems but also intensifies the coagulation pathways and enhances the interactions of the antibleeding agent with the wounded tissue.156,157 In this regard, HNTs, bioactive glasses, and silica nanoparticles are the most frequently used nanoparticles that all have hemostatic performance. The combination of PSA with the abovementioned nanomaterials will strongly increase their cytocompatibility as well as their hemostasis efficacy. Also, it helps in regulating wound moisture and faster healing.158
4. Advanced applications of polysaccharides in biomedical engineering
4.1. Tissue engineering
4.1.1 Skin tissue engineering.
Skin plays an essential role in preserving the body from the attack of the stimuli that come from outside. As the largest organ, it has a great control over heat and water loss.159–161 There are two main approaches to rescue a damaged skin. Autografts and allografts are practical approaches for saving highly injured skin (for instance, a high percentage burnt skin), but they may cause immune response such as morbidity.162,163 Hence, tissue engineering seems to be a perfect approach to heal tissue damages as an alternative for natural skin.164 In addition to having good mechanical properties (skin stretching ratio is 70%, which is drastic), appropriate biomaterials for skin scaffold fabrication have to support cell attachment, growth, proliferation, differentiation, and migration, besides enabling cell–cell signaling (fibroblasts, keratinocytes, as well as melanocytes as the foremost three types of skin cells) and the minimum possible immunogenic response.165–168 A wide variety of PSA-based biomaterials and nanoadditives with good mechanical and biological properties have been reported.169 Among various biomaterials that can be utilized for skin tissue engineering, PSAs are great potent options. Although hydrophilicity is crucial in all biomaterials used in tissue engineering, it is underscored for skin scaffolds.170–172 PSA-based nanocomposite can be referred to as a great biodegradable 3D structure to entrap a large amount of water.173 Also, there are several reports on PSA-based nanocomposites simulating biological and mechanical properties of human skin for tissue regeneration purposes. For instance, the composition of copper nanoparticles with chitosan and gelatin (using the freeze-drying method) is reported as a great scaffold for skin tissue engineering. Their result showed that in spite of enhanced physiochemical properties (about 91% porosity), no negative effect was observed on cell behaviors (adhesion to scaffold or cell proliferation) after the addition of cooper nanostructures and, also, no negative influence on ROS production was observed.174 The combination of chitosan with bacterial cellulose and medical grade nanodiamonds (using electrospinning method) is another good example of a scaffold for skin regeneration. The results indicated that the scaffold strength increased from 13 to 25 MPa after the addition of only 1 wt% medical grade nanodiamonds without any damage to the biological properties (up to 90% cell viability). They demonstrated that the easier electrospinning process is another benefit of this combination with highly diminished size of the fibers (80 nm).175 Ultimately, it is worth mentioning that chitosan-based nanocomposites,174 cellulose-based nanocomposites,176,177 and gum-based nanocomposites178–180 are the most widely used PSAs in the skin engineering field. In addition to skin tissue engineering, wound healing can be referred to as another challenging field. PSAs are also reported as practical structures in the wound healing process.181,182 Typically, a natural PSA, such as calcium alginate, comprises D-mannuronic, L-guluronic acid, and abundant calcium ions, which upregulate the glycosaminoglycan (GAG) activities. GAG is a kind of ECM molecule not only responsible for fibroblast production, but also for cell–cell and cell–matrix interactions. Therefore, the upregulation of GAG can bring about improved interactions and increased fibroblasts production, which helps the wound healing process as well as skin regeneration.183
4.1.2 Bone tissue engineering.
There are plenty of studies that have suggested PSA-based nanocomposites with chiefly acceptable performance and functionality. Therefore, tissue engineering has considerably contributed to the progression in this field.119,184–188 However, the proposed scaffold has some features such as the preparation of a good media for cell differentiation, great cell attachment, as well as desired mechanical properties (mechanical properties are underscored in bone engineering and, sometimes, even antibacterial activities are needed139). Anionic PSAs are outstanding considering the fact that GAGs (glycosaminoglycans as the actual components of ECM) support the cell signaling capability. In this regard, an inspiring platform was firstly suggested by Fricain et al. Natural hydrophilic pullulan combined with nanocrystalline hydroxyapatite particles is proposed as a great structure to induce bone marker expression. Moreover, the supportive behavior of this scaffold brought about morphogenetic protein 2 expression. Hence, the mentioned scaffold could mimic the required media for mesenchymal stem cells differentiation, which is practical in surgical procedures.116 To enhance the mechanical properties similar to a real bone, the combination of chitosan, chondroitin sulfate, as well as hydroxyapatite was examined utilizing the freeze-drying method. The obtained nanocomposite supports osteoblast cell growth and osteoblast adhesion along with good mechanical properties.189 However, without the presence of different nanostructures (organic, inorganic, and polymeric) near the PSA matrix, improving the mechanical features190–192 was completely impossible.
4.1.3 Nerve tissue engineering.
Nerve regeneration is reported as a sophisticated biological process that needs nerve gap bridging utilizing autologous grafts, which have a few number of donors.193 Other issues such as non-functionality after the surgery as well as immune rejection of the graft can be pointed out.194 As an alternative, tissue engineers propose some options. For instance, the fabrication of appropriate scaffolds to host stem cells with differentiation capability is in demand. In this regard, polymeric nanocomposites seem to be highly appealing due to their controllable features and electroconductive behaviors195 (electroconductivity in nerve grafts is important195). Suitable scaffolds should also support cell attachment, differentiation, and growth because they simulate the real extracellular environment, which is required by nerve cells.196 Among various biomaterials that can be utilized, PSAs are highly potent options. There are studies that have used PSA-based nanocomposites to emulate autologous nerve. Among all, chitosan-based nanocomposites197–205 are the most frequent ones. To name a few, Karami et al. utilized the electrospinning method to fabricate a scaffold consisting of poly(hydroxybutyrate) nanostructures and chitosan, which is reported as a suitable nerve graft. Their results indicated that this biocompatible scaffold is highly hydrophilic and has great mechanical/electrical and morphological properties similar to the real nerve.199 Manzari et al. constructed a scaffold consisting of polypyrrole (PPy), alginate, and chitosan-based nanoparticles, in which they utilized the oxidative polymerization method to synthesize PPy. Their findings revealed that the fabricated scaffold is a great option for nerve engineering due to electrical conductivity (2
:
10 ratio of pyrrole and alginate) and the good condition of the scaffold for neural or fibroblast cells’ activation and proliferation 72 h after cell culturing.201
4.1.4 Cartilage tissue engineering.
Cartilage self-repair is an important issue (because of very high mechanical properties (8.3 MPa tensile strength) and very low repair rate (due to lacking vascular network)), and the engineered alternative scaffolds must have specific and unique features.206 Several tissue engineers have felt the arduous task of fabricating a qualified scaffold for this target. PSA-based nanocomposites are proper options because of the biocompatibility and good mechanical properties inherited from the embedded nanomaterials and/or acquired chemical crosslinking. Moreover, their similarity to the real tissue media (i.e., the ECM) make them more outstanding.207,208 Scientists did their best to fabricate a scaffold from multiwalled carbon nanotubes and poly(3-hydroxybutyrate) (PHB)–chitosan to exploit the desired mechanical properties similar to a real cartilage. The resulting scaffold not only supports chondrocyte adhesion and growth but also has excellent hydrophilicity and tensile strength due to the addition of carbon nanotubes to chitosan (8 MPa yield strength and 20% elongation at break).209 Among other suitable nanostructures to be combined with PSAs, nanohydroxyapatite plays an important role. Using freeze-gelation method, a nanocomposite hydrogel from chitosan, collagen, and nanohydroxyapatite was fabricated with low cost and a Young's modulus of 80–800 kPa, which is closely similar to the modulus of cartilage.210Table 6 summarizes different kinds of PSA-based scaffolds with their constituents, fabrication methods, main findings, as well as the targeted tissue.
Table 6 A summary of different kinds of scaffolds with PSA based and their constituents, fabrication methods, main findings, as well as the targeted tissue
Base polysaccharide |
Nano additive(s) |
Target tissue |
Fabrication method |
Main findings |
Ref. |
Chitosan |
Gold nanoparticles |
Bone |
Tilting method/WAXS and UV-VIS techniques |
Highly cytocompatible toward normal kidney epithelial cells/epithelial colorectal adenocarcinoma/positive human cervical tumor/and murine macrophage cells |
211
|
Chitosan |
Nanohydroxyapatite |
Bone |
Freeze-drying technique |
Great condition for osteoblast cell adhesion/proliferation and growth |
189
|
Chitosan |
Nanohydroxyapatite |
Bone |
Combination of freeze-drying with a foaming agent method |
Great hydrophilic media that endorses protein adsorption/great support for fibronectin binding/good osteoblast adhesion/great osteoblast proliferation |
212
|
Chitosan |
Hydroxyapatite nano crystallites |
Bone |
Coprecipitation procedure |
Excellent compressive strength/great condition for osteoblast proliferation |
213
|
Chitosan |
Methacrylated silk fibroin micro |
Cartilage |
Photocrosslinking method |
Excellent compressive modulus/a great cytocompatibility for mouse articular chondrocytes |
214
|
Chitosan |
Alumina nanowires |
Cartilage |
Electrospinning method |
High porosity/great hydrophilicity/Chondrocyte cytocompatibility |
215
|
Chitosan |
Nanohydroxyapatite |
Cartilage |
Freeze-gelation method |
Low-cost preparation/Young's modulus of 80–800 kPa |
210
|
Chitosan |
β-tricalcium phosphate nanoparticles |
Cartilage |
Sol–gel method |
Good degradation rate/hydrophilicity/porosity |
216
|
Chitosan |
Multiwalled carbon nanotubes |
Cartilage |
Electrospinning method |
Weak mechanical properties without carbon nanotubes and enhanced mechanical features after the addition of carbon nanotubes |
217
|
Chitosan |
Multiwalled carbon nanotubes |
Cartilage |
Electrospinning method |
High tensile strength/chondrocyte cytocompatibility |
209
|
Chitosan |
Graphene nano-sheets |
Nerve |
Solution casting method |
Excellent electrical conductivity/great cell proliferation after 72 hours |
197
|
Chitosan |
Polycaprolactone and chitosan nanofiber |
Nerve |
Electrospinning method |
Excellent condition for mesenchymal stem cells differentiation to neuron-like cells |
205
|
Chitosan |
Chitosan-based nanoparticles |
Nerve |
Oxidative polymerization method |
Great electrical conductivity/nice condition for neural or fibroblast cells activation and proliferation |
201
|
Chitosan |
Poly(hydroxybutyrate) nanostructures |
Nerve |
Electrospinning method |
Great hydrophilicity and mechanical properties similar to nerve tissue |
199
|
Chitosan |
Copper nanoparticles |
Skin |
Freeze-drying method |
In spite of enhanced physicochemical properties, no effect was observed on cell behaviors (adhesion to scaffold or proliferation) after addition of cooper, no influence on ROS production |
174
|
Chitosan |
Medical grade nanodiamonds |
Skin |
Electrospinning method |
Scaffold strength increase from 13 MPa to 25 MPa after addition of only 1 wt% MND/easier electrospinning process/diminished size of fibers |
175
|
Chitosan |
HNTs |
Skin |
Simple solid liquid interaction technique |
Reepithelization and reorganization of fibroblast cells/great capacity for wound healing |
218
|
Chitosan |
Polystyrene sulfonate nanofibers |
Cardiac |
Electrospinning method |
Great mechanical properties/electrical conductivity/increasing the tensile strength only with addition of 1 wt% of synthetic polymers |
219
|
Chitosan |
SrAl2O4:Eu2+/Dy3+ nanophosphor |
Eye |
Sol–gel method |
Promising growth and differentiation of the recognized retinal progenitors within the scaffold |
220
|
Cellulose |
HNTs |
Bone |
Freeze-drying technique |
Injectability in various conditions |
221
|
Cellulose |
Nanohydroxyapatite |
Bone |
Free radical polymerization |
Great compressive strength and elastic modulus consistent with bone tissue |
222
|
Cellulose |
Nanohydroxyapatite |
Bone/cartilage |
Freeze-drying technique |
Excellent cytocompatibility toward wide range of cells (osteosarcoma cells, human articular chondrocytes, as well as human adipose-derived mesenchymal stem cells) |
223
|
Cellulose |
Cellulose nanofibers |
Cartilage |
Freeze-drying technique |
High porosity (95%)/high compression moduli = 1Mpa/great similarity with natural cartilage due to viscoelasticity behavior |
224
|
Cellulose |
Cellulose nanocrystals |
Skin |
Electrospinning technique |
Great cytocompatibility toward 3T3 fibroblast cells/great hydrophilicity |
176
|
Cellulose |
Bacterial cellulose nanofibers |
Skin |
Colorimetric method/shindai extraction method |
Support for adhesion of keratinocyte cells/skin fibroblast cells |
177
|
Guar gum |
Poly(ε-caprolactone) nanofibers |
Skin |
Electrospinning method |
Great elongation and tensile strength similar to skin tissue |
180
|
Guar gum |
Gum arabic nanofibers |
Skin |
Electrospinning method |
Elongation and tensile strength similar to skin tissue/antibacterial activity/support for L929 cells proliferation |
179
|
Guar gum |
Gum arabic nanofibers |
Skin |
Suspension, two-nozzle and multilayer electrospinning |
Appropriate fibroblast cells adhesion and proliferation |
178
|
Alginate |
GO nanosheets/nanohydroxyapatite |
Bone |
Freeze-drying process |
Great compressive strength similar to bone/low rate of biodegradation/cytocompatibility for MG-63 bone cells |
225
|
4.2. Polysaccharide nanocomposites for cell encapsulation
Nanocomposite hydrogels are highly examined as platforms, in which cells can be encapsulated and protected from high shear forces and successfully delivered to the aimed region. One approach is to add 2D or 3D nanomaterials to the PSAs in order to make them strongly interacted and accelerate their shear thinning behavior.226,227 As an instance, 2D nanosilicate reinforced kappa-carrageenan (κCA) hydrogel was prepared in order to give it shear thinning characteristics, higher mechanical stiffness, elastomeric properties, and physiological stability. Scientists utilized this new platform for the delivery of human mesenchymal stem cells and their analysis demonstrated the high cell viability (85% cell viability) (Fig. 5). This study seems to be promising for cell delivery aims (such as cartilage tissue regeneration systems) as well as 3D bioprinting.228 In this regard, the utilization of stimuli-responsive PSAs (such as chitosan) will also support the possibility of 4D-printed scaffold fabrication for cell encapsulation (4D printed scaffolds have the ability to deform and change the release pattern as a function of time, which is of a great importance, if needed229). Another study reports the successful encapsulation of ATDC5 cells (mouse teratocarcinoma cells) within a xanthan gum-based microscale system (xanthan gum is a high weight type of PSA) and their results suggested that their PSA-based platform can preserve the cellular metabolic activities up to 21 days after incubation with a great level of viability, shear-thinning, and gelling behaviors.21 In addition, a sort of pH-responsive (responsive to the pH less than 4.2) PSA nanocomposite consisting of chitosan (CS) and oxidized hydroxypropyl cellulose (HPC) nanofibers with great mechanical property at 49 s gelation time shows a perfect and stable network for the high percentage delivery of the stem cells. Their analysis could demonstrate a promising stability, cytocompatibility (more than 93%), as well as biodegradability (after 15 days at 37 °C).230
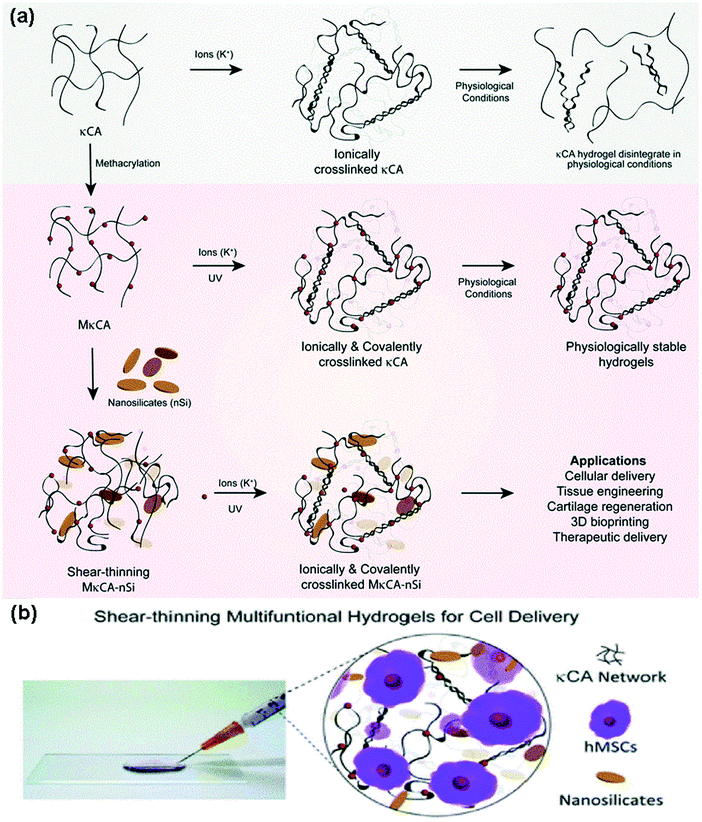 |
| Fig. 5 (A) The illustration of the synthesis process of a hydrogel with PSA base (kappa-carrageenan (κCA)) using potassium ions coated with nanosilicates, which gives it shear thinning properties and bioactive features. (B) Encapsulation of human mesenchymal stem cells within the synthesized network for cell delivery applications.228 | |
4.3. Polysaccharide nanocomposites for cancer diagnosis and treatment
Some PSAs or chemically-modified PSAs possess inherent bioactivities for early cancer diagnosis and therapy. The effectiveness of PSA-based nanocomposites for cancer arises from embedded nanomaterials. For example, gold nanoparticles with NIR-absorbing properties can be incorporated with PSA nanogels for the photothermal therapy (PTT) of various cancers. As another instance, nanometals incorporated with PSAs create great platforms for selectively targeting tumor cells (Fig. 6).231,232 Another study demonstrated that the combination of gold nanoparticles and immune-active PSAs can successfully enhance the dendritic cell and T cell activation parallelly with the inhibition of tumor growth and metastasis when loaded with doxorubicin.233 In addition to the abovementioned examples, the surface biofunctionalization of graphene with PSAs can be employed for cancer diagnosis and treatment due to increased water solubility, drug capacity, large surface area, and photothermal properties. These systems are promising owing to the fact that they underline the cancer cell responses to internal and external stimulus, which help us trap cancerous cells.234
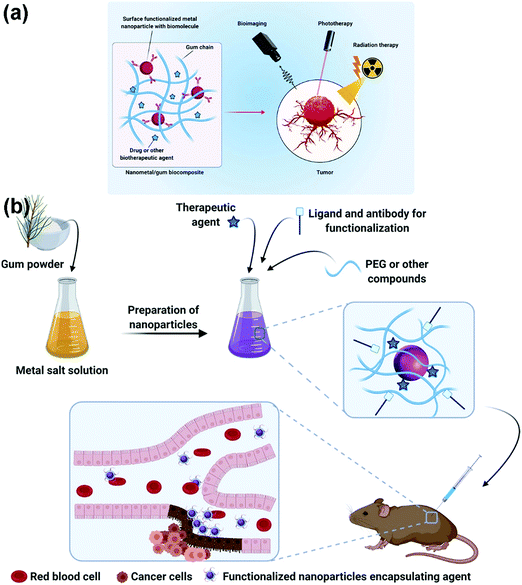 |
| Fig. 6 (A) A schematic illustration of PSA-based platform incorporated with nanometal usable in cancer treatment and diagnosis, detectable via phototherapy, bioimaging, as well as radiation therapy. (B) The schematics of synthesis and functionalization process of the encapsulated agent as well as the tumor cell detection strategy after reaching the targeted site.232 | |
Recent studies have achieved unparalleled progress in the fabrication of graphene nanocomposites for cancer therapy. In spite of this progress, toxicity is sometimes an unavoidable problem that walks hand-in-hand with the use of inorganic-nanomaterials.235 Nonetheless, the tunable properties of graphene nanocomposites help scientists to utilize it in the field of oncology. For instance, the combination of GO with chitosan supports the prepared scaffold to have sustained doxorubicin release and excellent stability as well as biocompatibility.236 Thus, the combination of graphene or GO with biocompatible materials such as PSAs (specifically chitosan) provides the user with a great opportunity to use these materials as smart nanoprobes for cancer treatment or diagnosis. For example, the combination of chitosan, hyaluronic acid, and GO is proposed for SNX-2112 (it is a heat shock protein with anticancer properties) delivery. The results indicated that this scaffold enhances the ability of recognition of the cancer cells with higher efficacy in sustained SNX-2112 release within an acidic condition, which causes A549 cell death (human lung carcinoma cell line).237 The combination of hydroxyapatite and chitosan for celecoxib delivery ended in excellent sustained release, which brought about apoptosis of the colon cancer cells.238 Rabiee et al. showed that the addition of PSAs such as chitosan to the highly toxic nanomaterials containing CoNi2S4 (Fig. 7)239 could lead to an increase in the relative cell viability, considerably. This approach is promising due to a wide range of nanocarriers based on inorganic-components possessing poor biocompatibility/bioavailability. Moreover, they have shown that the addition of chitosan leads to a reduction in the interactions between the toxic components and cell walls, in both the HeLa (cervical cell line) and HEK-293 cell lines (human embryonic kidney 293 cells), but increases the constructive interactions for chemotherapy drug delivery.239 Also, the combination of chitosan with alginate nanoparticles for antisense oligonucleotide delivery has the ability to downregulate the EGFR (epidermal growth factor receptor) expression for the ones that suffer from breast cancer cell.240,241 In addition, ganoderma lucidum PSA and gold are a promising amalgamate for doxorubicin delivery and increase the T cells growth due to the acceleration of dendritic cells activities, which ultimately hinders 4T1 tumor cells proliferation for pulmonary cancer.233Table 7 compares different scaffolds, their base PSA, constituents, the loaded anticancer drug, and the main findings. However, the main findings based on the use of PSAs on the surface of the nanostructures (nanocarriers) for cancer therapy was a challenge between the routine synthetic co- and/or triblock polymers including poly(HEMA) and poly(NIPAM). It should be noted that by the addition of these PSAs, some of the advantageous of the synthetic polymers including well-defined interactions to the cells as well as the considerable biocompatibility decreased. The synthetic polymers could lead to well-defined nanostructures (nanocarriers), but they are not simple to prepare, cost-effective, and highly green. Thus, the need for using PSA-based polymers instead of synthetic polymers has been felt seriously. Moreover, the need for a combination of these PSAs with inorganic- and organic-based nanomaterials have been felt.
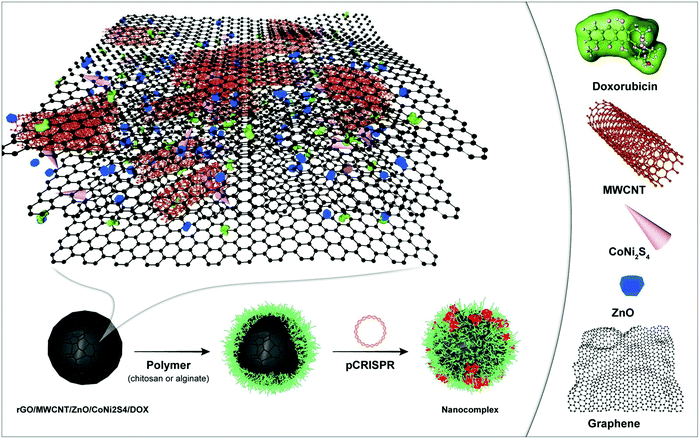 |
| Fig. 7 A schematic illustration of the highly toxic nanomaterial based on CoNi2S4 coated with chitosan for targeted DOX delivery orientated toward cancer therapy.239 | |
Table 7 A summary of different scaffolds, their base PSA, constituents, loaded anticancer, and main findings
Base PSA |
Nanoadditive(s) |
Payload |
Main findings |
Ref. |
Pullulan |
Gold nanorods |
— |
Due to acidic environment in addition to concentrated amount of glutathione (that endosomes have)/self-destruction behavior/great influence on cancer cells inactivation |
242
|
Hyaluronic acid |
GO nanoparticles |
SNX-2112 |
Enhanced ability of recognition of the cancer cells/high efficacy in sustained SNX-2112 release within an acidic environment caused A549 cells death |
237
|
Alginate |
Poly-deoxyadenylic acid nanostructure |
Phosphorothioated antisense oligodeoxyribonucleotide of TNF-α |
A great option for intestine inflammation/influence on macrophage cells’ performance/great reduction of TNF-α secretion |
243
|
Cellulose |
Nanocellulose |
5-Fluorouracil |
pH sensitive/ability to destroy the colon cancer cells |
244
|
Ganoderma lucidum PSA |
Gold nanoparticles |
Doxorubicin |
Accelerated T cells growth due to the acceleration of dendritic cells activities/hindering 4T1 tumor cells proliferation for pulmonary cancer |
233
|
4.3.1 Polysaccharide nanocomposites for cancer radiotherapy.
The advent of X-rays was a significant revolution in cancer treatment. Radiation therapy (derived from X-rays technology) is a huge improvement in controlling cancer progression. However, safer and more efficient radiation delivery is the reason behind the use of biomaterials. Nowadays, biomaterials have turned the field of theragnostic and combination therapy upside down.245 In this regard, PSA-based nanocomposites are utilized to deliver immunostimulatory radioisotopes and they can also be modified with radiosensitizer moieties (see Fig. 8245). Remarkably, the utilization of PSAs for siRNA delivery to a desired area is of great importance in order to overcome a possible radiation resistance.246 Among different PSAs, the chitosan-based nanocomposite is an outstanding one because this biopolymer can easily maintain its structural integrity before being exposed to the acidic media of cancerous cells. For instance, according to Yang et al., the encapsulated substance can be stored inside the chitosan-loaded polylactic-co-glycolic acid (PLGA) nanoparticles without any leakage.247,248
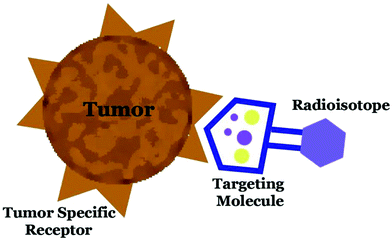 |
| Fig. 8 A schematic illustration of radioisotope delivery to cancer cells using biomolecules.245 | |
4.3.2 Polysaccharide nanocomposites for cancer immunotherapy.
Immunosuppression (known as immunotherapy) is a very powerful tool for cancer cell eradication.249 Immunotherapy has made significant progress and also has a very long way to go. However, the inappropriate specificity of this method is a serious limitation. Natural and synthetic biomaterials have been utilized to realize efficient immunotherapeutic targeting and address the existing challenges.250 In this regard, immunotherapy agents such as immunostimulatory small molecules,251 some nucleic acid adjuvants,252 different proteins253,254 or antibodies,255,256 as well as exogenous immune cells257 can be encapsulated inside the nanocomposite in order to induce a gradient of agent's concentration. Among different biomaterials and platforms, PSA-based nanocomposites, especially alginate nanocomposites, are able to play the role of a reservoir for the abovementioned factors (see Fig. 9258). The main reasons behind using alginate as a matrix are it being FDA-approved, cost effective, has potential for large scale manufacturing, low immunogenicity, as well as inherent ionic behavior and hydrophilicity.259 As an instance, one group utilized an alginate nanocomposite scaffold for the codelivery of programmed CAR T cells in conjunction with cyclic-dinucleotide (CDN), which was an effective platform for treating mild tumors.250 Despite the advances in immunotherapy-conjugated materials and nanotechnology, a great of deal of time and energy must be expended to translate the ideas to clinic. The major challenges that may hinder the clinical translation of immunotherapy are designing pre-clinical models translating to human immunity, determining the main drivers of cancer immunity process, discerning the organ-specific tumor immune contexture, and the molecular and cellular diversity of immune escape. Moreover, developing advanced personalized approaches for cancer immunotherapy as well as understanding the effect of immune suppression on autoimmune toxicities must be taken into consideration in the clinical implementation of immunotherapy.260
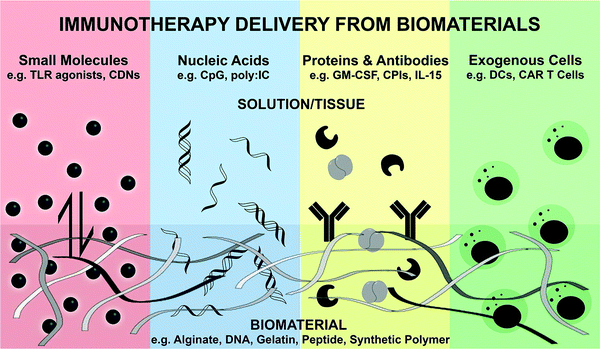 |
| Fig. 9 A schematic illustration of the biomaterial for the controlled release of bioactive immunotherapies.258 | |
4.3.3 Polysaccharide nanocomposites for cancer phototherapy.
Light-induced cancer treatment, which is also called phototherapy, is a state-of-the-art progressing field of cancer treatment. Tumor-associated antigens (TAAs) release (from the cancerous cells that are dying after being exposed to phototherapy) as well as damage-associated molecular patterns (DAMPs) have been considered as phototherapeutic immunological responses that must be amplified. The amplification of the abovementioned responses is a perfect cue for immune cells to recognize and kill the cancerous cells.261 If the responses are amplified, auxiliary methods such as immune checkpoint blockade (ICB) may also be available.262 In this regard, different types of biomaterials have been served as photoabsorbers or sensitizing agents to improve the performance of the therapeutics as well as minimize the possible side effects of this new technique. For instance, the encapsulation of oligodeoxynucleotides inside chitosan-coated hollow copper sulfide (CuS) nanoparticles was an effective template as a photoabsorbent agent (Fig. 10263). Under laser irradiation (near-infrared laser (900 nm, 2.0 W cm−2, 40 s)), the structural breaking of chitosan–CuS is the result of cancer cells’ rise in temperature until they burn. Then, oligodeoxynucleotides will be released and the tumor will be affected.263,264 Another study indicated that polydopamine nanoparticles are also a great option as a photothermal additive agent265,266 to be combined with PSAs.267–269 Remarkably, gold nanoworms (5 ± 1.5 nm) can strongly enhance the light-heat conversion efficiency.270,271
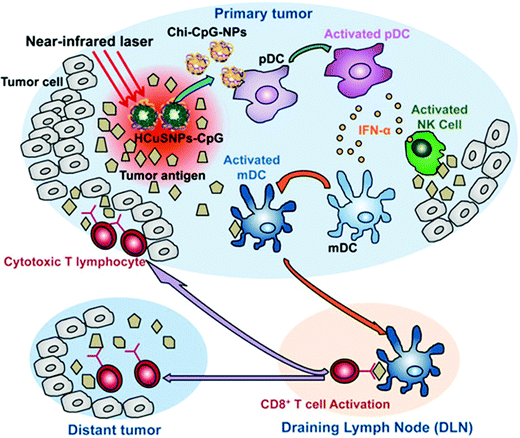 |
| Fig. 10 Mechanism of chitosan–CuS oligodeoxynucleotides-loaded photothermal therapy for both primary and distant tumors.263 | |
4.4 Polysaccharide nanocomposites for Bioimaging
There exist many imaging techniques (such as optical and magnetic resonance imaging) that play a significant role in disease recognition and treatment. However, state-of-the-art bioimaging probes are urgently required to enhance the sensitivity and specificity. Recently, chemists and bioimaging scientists have opened a new window of bioimaging, which is known as polymer-based bioimaging. In this regard, biomaterials and imaging modalities have been combined to produce effective bioimaging probes.272 The designed structures need to have specific features in order to be qualified, for instance, prolonged half-life, stability, low toxicity, as well as target specificity. Among various biomaterials, PSAs are of great interest in the bioimaging field owing to the fact that their nanocomposites have excellent stability, very high biocompatibility, and they are great stimuli responsive platforms.273 The chelation of gadolinium-based contrast agents (GBCAs) (as a very frequently used magnetic agent in bioimaging) with PSAs will strongly enhance the compatibility of gadolinium (Gd) due to the fact that the diffusion of Gd to the surrounding media (it is a low molecular weight component that can diffuse in the vein) will decrease the imaging quality. Dextran- and starch-conjugated Gd nanocomposites have been repetitively used as MRI bioimaging probes.68 Noteworthily, PSAs’ modifiable backbone (presence of different chemical structures on their backbonem which facilitates the modification) as well as good biodegradability are the other factors that make PSAs desirable options in this regard.
4.5. Polysaccharide nanocomposites as biosensors
The ever-expanding utilization of biosensors has been observed in recent years. It is expected that the value of biosensors sale will reaching from 0.58 U.S. dollars in 2017 to 1.4 billion U.S. dollars globally by 2027 (almost 2.5 times increase in the income over one decade).274 The applications of biosensors are extremely diverse and some of them are as follows: clinical and diagnostic applications, environmental applications, as well as industrial ones. The most effective factor for producing a sensitive and efficient biosensor is the accurate immobilization of biological elements on the polymeric matrix surface.275,276 PSA-based biomaterials and their nanocomposites can be mentioned as a great matrix for surface immobilization.277 The reason behind the high usage of PSA-based nanocomposites (as a matrix) is that they have several chemical functionalities (useful for the immobilization of biological elements), high permeability, great biocompatibility, no toxicity, cost-effectiveness, and very high availability.278 Being low cost and highly available as a biosensor matrix is invaluable because biosensors are usually mass-produced and they should be available to all segments of the population. Among different members of the PSA family, the chitosan nanocomposite is of great importance for biosensor production and biodetection applications.278–281 The presence of amino and hydroxyl groups on the chitosan backbone (for the facile modification or the chelation of nanoparticles), very good sensitivity to different stimuli (such as glucose, pH (under acidic conditions due to the presence of basic amine groups), or even an electric field), and high stability for the detection of various kinds of targets84 (such as different proteins, DNA structures, various biomolecules, and even bacteria) are the reasons that highlight the use of chitosan nanocomposites in biosensors.278 In addition, the very porous structure of chitosan effectively increases not only the loading capacity but also the sensitivity to outside signals.282 Remarkably, biodegradability, renewability, safe for humans, as well as very low immunogenicity are the other important behaviors of chitosan as a matrix for bioagents.283 According to Zhang et al., the combination of chitosan with carbon nanotubes will be a great platform not only for aflatoxin B1 (a kind of contaminant in a variety of foods such as grains) detection but also for antibody binding.284 Noteworthily, Guner et al. suggested the combination of chitosan and gold nanoparticles for antibody immobilization as well as Escherichia coli (E. coli) detection.285 The chemical modification of chitosan (such as chitosan-poly(acrylic acid)-metal ions nanospheres) is also suggested for the preparation of reliable binding sites to immobilize antibodies. The conjugation of chitosan and graphene nanosheets is also an interesting option to provide adequate sites for DNA hybridization reactions.286,287
5. Limitations and challenges
Nanocomposites are a new class of platforms that have several applications in biomedical engineering. The most important reason for the development of these systems is that despite their excellent biological properties, biomaterials are not able to provide excellent physical and mechanical properties. In addition to increasing the mechanical stability, the addition of nanomaterials enhanced some biological properties such as cellular adhesion and antibacterial features. Also, the possibility of loading the drug into these nanoparticles is one of the attractive points of these systems. However, we have to keep it in mind that nanomaterials can be very toxic (dependent on the used weight percentage288). In this regard, a holistic understanding of their toxicity mechanisms will be a huge step toward neutralizing toxic behaviors.289 In this section, we briefly discuss the cellular view of toxicity mechanisms.
Silver nanoparticles are among the most repetitively used nanostructures in biomedical engineering (silver is highly antibacterial). However, it strongly affects the mitochondrial respiratory chains and will bring about the production of reactive oxygen species (ROS) (reactive molecules containing oxygen). The more the mitochondria are poisoned, the more the cells ‘energy chains will be distracted, which finally harms the’ DNA of the cells and will lead to cell apoptosis.290 The mechanism underlying titanium oxide toxicity is ROS production, inflammation responses, as well as genotoxicity, which finally eradicates the cells. Interestingly, cell poisoning is not only a function if the titanium oxide weight is in percentage, but also its physical properties such as size, crystal structure, as well as photoactivation.291,292 It is worth addressing that the possible mechanism of zinc toxicity is the penetration of nanostructures into the pits or protrusion of the cell walls, which kills cells through the extrusion of cytoplasmic contents.293 Finally, and importantly, the potent toxicity of gold and copper nanoparticles is almost somewhat similar. The poisoning of cells' redox system, mitochondrial damage, as well as proteins and DNA injuries will kill the cells.294,295
Besides the possibilities with nanocomposite PSAs in biomedical applications, there are some serious limitations that are mainly caused by the natural resources from which PSAs are originated, which degrades their pharmaceutical and biological applications. To name a few, batch-to-batch variations, the possibility of microbial contamination, viscosity drop during the storage stages, viscosity thickening, as well as uncontrolled rate of hydration can be addressed. Moreover, the extraction and purification of PSAs in view of the amalgamated nature of the presence of PSAs in nature with proteins and lipids.296 Thus, a precise isolation of PSAs requires smarter methods other than co-extraction, which may contaminate them.297 Eventually, a comprehensive understanding of the structural reactivity of PSAs has been recognized as another challenging aspect, which narrowed down the windows of pharmaceutical and biological applications.298
6. Concluding remarks and future perspective
Recent advances in the applications of PSAs in biomedical engineering witness the importance of dealing with the improvement of their properties, mainly poor mechanical strength, by the use of reinforcing agents, mainly nanomaterials. Taking advantage of nanoparticles such as silver, copper, gold, titanium oxide, and zinc in giving mechanical strength along with antioxidant, antibacterial, and/or conductivity, the use of PSA nanocomposites is becoming widespread as reliable biomedical platforms, leading to a paradigm shift from fantasy to practical use for clinical purposes. The resulting stimuli-responsive systems not only have great media supporting drug, gene, and cell delivery templates but also induce nanocharacteristics with multidimensional physicochemical features for skin, bone, nerve, and cartilage tissue engineering. Moreover, they can play an essential role in cancer diagnosis and treatment (radio, immune, and photothermal therapy), bioimaging, as well as biosensing. From the biosensing application, enzyme engineering offers precise and efficient enzyme immobilization techniques, which localizes the enzymes (e.g., laccase) onto the PSA backbone.299 From the immobilization perspective, PSAs are promising biomaterials because they are chemically modifiable. PSA composites are also able to improve the lifetime as well as the stability of the immobilized enzyme in catalytic reactions.300 Notably, the selectivity of the immobilized enzyme is effectively preserved for any particular application after immobilization on the surface of PSA.301
In this review, we have looked at the superiority of PSA-based nanocomposites over neat PSA, which are classified into two main categories as generic and advanced applications of PSA nanocomposites. After the precise analysis of the literature, we understood that there are some existing challenges such as nanomaterial toxicity and also some opportunities for future studies ahead of PSA-based nanocomposites in biomedicine. Some unsolved problems and unanswered questions can be counted accordingly:
(1) Difficulties in controlling PSA-based nanocomposite fabrication techniques, in order to achieve desired properties, specifically electrical and mechanical features, are of importance. For instance, one can address the thermal sensitivity of several PSA-based systems, which leads to degradation even before the melting point. This is the case when it especially requires thermomechanical stability or mechanical toleration and durability in surgical procedures (if needed).
(2) An increasing demand still exists for devising new PSA-based nanostructures for personalized diagnostic outputs as well as therapeutic such as personalized anticancer delivery in order to minimize the side effects.
(3) Weak immunogenic response, toxicity, or immunogenicity of their modification or collaboration with other materials, or discovering new toxicity neutralizing mechanisms to diminish nanomaterials toxicity.
To overcome the abovementioned obstacles, we suggest four main approaches. First of all, we believe that devising more complicated systems by the combination of different PSAs with different abilities can lead to overcoming the existing weaknesses. Secondly, we anticipate that deeper investigations on the effects of each component in a composite and the exact mechanism for each effect can be a huge step toward the future progression in this field. In fact, we need to be aware of the details and mechanisms more explicitly. Thirdly, it would be great to utilize the most state-of-the-art systems (such as electrospinning, 3D and 4D printing, and artificial intelligence) in order to improve our understanding of details or to enhance the manufacturing and biomodification processes. For instance, devising methods to optimize the composites’ features utilizing artificial intelligence can be a great topic for future studies. In addition to technological advances, the ability to use and apply them in various fields purposefully is a key factor. Ultimately, considering the limited number of practical PSAs, there is a huge demand for the modification of their properties, which can be obtained via the addition of nanoparticles or bulk modification that boosts the matrix and additive surface biointeractions. Based on the abovementioned discussion, in spite of the excellent progress in this field, a long way is still needed to be paved. Moreover, we are still at an early stage of using PSA nanocomposites, making the clinical implementation of the strategies the ultimate objective.
Abbreviations
PSAs | Polysaccharides |
ECM | Extracellular matrix |
nZnHA | Zinc-doped hydroxyapatite nanoparticles |
hAD-MSCs | Human adipose-derived stem cells |
bFGFs | Basic fibroblast growth factors |
GO | Graphene oxide |
HNTs | Halloysite nanotubes |
Ag | Silver |
ROS | Reactive oxygen species |
NIPAM |
N-Isopropyl acrylamide |
NIR | Near-infrared |
DDSs | Drug delivery systems |
PPy | Polypyrrole |
PHB | Poly(3-hydroxybutyrate) |
PTT | Photothermal therapy |
DOX | Doxorubicin |
AgNO3 | Silver nitrate |
PEI | Polyethylenimine |
PEG | Polyethylene glycol |
NaBH4 | Sodium borohydride |
PSS | Polystyrene sulfonate |
GAG | Glycosaminoglycan |
Conflicts of interest
There are no conflicts to declare.
References
- F. Seidi, M. K. Yazdi, M. Jouyandeh, S. Habibzadeh, M. T. Munir, H. Vahabi, B. Bagheri, N. Rabiee, P. Zarrintaj and M. R. Saeb, Carbohydr. Polym., 2021, 118624 Search PubMed.
-
M. S. Hasnain, S. A. Ahmad, N. Chaudhary, M. N. Hoda and A. K. Nayak, Applications of nanocomposite materials in orthopedics, Elsevier, 2019, pp. 1–37 Search PubMed.
- J. Venkatesan, S. Anil, S. Rao, I. Bhatnagar and S.-K. Kim, Curr. Pharm. Des., 2019, 25, 1200–1209 CrossRef CAS PubMed.
- S. Vandghanooni and M. Eskandani, Int. J. Biol. Macromol., 2019, 141, 636–662 CrossRef CAS PubMed.
-
Z. Shariatinia, Advanced Biopolymeric Systems for Drug Delivery, Springer, 2020, pp. 233–290 Search PubMed.
- M. K. Yazdi, V. Vatanpour, A. Taghizadeh, M. Taghizadeh, M. R. Ganjali, M. T. Munir, S. Habibzadeh, M. R. Saeb and M. Ghaedi, Mater. Sci. Eng., C, 2020, 114, 111023 CrossRef CAS PubMed.
-
L. Lucia and A. Ayoub, Chemical and Engineering Fundamentals and Industrial Applications. Springer Book, 2018 Search PubMed.
- Y. Zheng, J. Monty and R. J. Linhardt, Carbohydr. Res., 2015, 405, 23–32 CrossRef CAS PubMed.
- S. Mohebbi, M. N. Nezhad, P. Zarrintaj, S. H. Jafari, S. S. Gholizadeh, M. R. Saeb and M. Mozafari, Curr. Stem Cell Res. Therapy, 2019, 14, 93–116 CrossRef CAS PubMed.
- M. Heidari, S. H. Bahrami, M. Ranjbar-Mohammadi and P. Milan, Mater. Sci. Eng., C, 2019, 103, 109768 CrossRef CAS PubMed.
- M. Rahmati, D. K. Mills, A. M. Urbanska, M. R. Saeb, J. R. Venugopal, S. Ramakrishna and M. Mozafari, Prog. Mater. Sci., 2021, 117, 100721 CrossRef CAS.
- S. Manouchehri, B. Bagheri, S. H. Rad, M. N. Nezhad, Y. C. Kim, O. O. Park, M. Farokhi, M. Jouyandeh, M. R. Ganjali and M. K. Yazdi, Prog. Org. Coat., 2019, 131, 389–396 CrossRef CAS.
- L. Cheng, Y. Wang, X. He and X. Wei, Int. J. Biol. Macromol., 2018, 120, 82–92 CrossRef CAS PubMed.
-
P. Gupta, H. Gupta and K. M. Poluri, Microbial and Natural Macromolecules, Elsevier, 2021, pp. 233–272 Search PubMed.
- Y. Yu, M. Shen, Q. Song and J. Xie, Carbohydr. Polym., 2018, 183, 91–101 CrossRef CAS PubMed.
- A. Sood, A. Gupta and G. Agrawal, Carbohydr. Polym. Technol. Appl., 2021, 2, 100067 CAS.
- M. Jin, J. Shi, W. Zhu, H. Yao and D.-A. Wang, Tissue Eng., Part B, 2021, 27, 604–626 CrossRef CAS PubMed.
- K. Ganesan, T. Budtova, L. Ratke, P. Gurikov, V. Baudron, I. Preibisch, P. Niemeyer, I. Smirnova and B. Milow, Materials, 2018, 11, 2144 CrossRef PubMed.
- P. Makvandi, M. Ghomi, M. Ashrafizadeh, A. Tafazoli, T. Agarwal, M. Delfi, J. Akhtari, E. N. Zare, V. V. Padil and A. Zarrabi, Carbohydr. Polym., 2020, 116952 CrossRef CAS PubMed.
- M. Liu, R. He, J. Yang, Z. Long, B. Huang, Y. Liu and C. Zhou, Clay Miner., 2016, 51, 457–467 CrossRef CAS.
- A. Kumar, K. M. Rao and S. S. Han, Carbohydr. Polym., 2018, 180, 128–144 CrossRef CAS PubMed.
-
S. Gowthami and S. Angayarkanny, Green Biopolymers and their Nanocomposites, Springer, 2019, pp. 379–402 Search PubMed.
- D. Nguyen, D. A. Hägg, A. Forsman, J. Ekholm, P. Nimkingratana, C. Brantsing, T. Kalogeropoulos, S. Zaunz, S. Concaro and M. Brittberg, Sci. Rep., 2017, 7, 1–10 CrossRef PubMed.
- P. Zarrintaj, M. R. Saeb, S. Ramakrishna and M. Mozafari, Curr. Opin. Biomed. Eng., 2018, 6, 99–109 CrossRef.
- S. Salatin and M. Jelvehgari, Pharm. Sci., 2017, 23, 84–94 CrossRef.
-
S. Mallakpour and F. Tabesh, Handbook of Polymer Nanocomposites for Industrial Applications, Elsevier, 2021, pp. 503–528 Search PubMed.
- M. Ji, F. Li, J. Li, J. Li, C. Zhang, K. Sun and Z. Guo, Mater. Des., 2021, 198, 109373 CrossRef CAS.
- H. Salimi-Kenari, F. Mollaie, E. Dashtimoghadam, M. Imani and B. Nyström, Carbohydr. Polym., 2018, 181, 141–149 CrossRef CAS PubMed.
- A. Tchobanian, H. Van Oosterwyck and P. Fardim, Carbohydr. Polym., 2019, 205, 601–625 CrossRef CAS PubMed.
- M. A. Nilforoushzadeh, M. Khodadadi Yazdi, S. Baradaran Ghavami, S. Farokhimanesh, L. Mohammadi Amirabad, P. Zarrintaj, M. R. Saeb, M. R. Hamblin, M. Zare and M. Mozafari, ACS Biomater. Sci. Eng., 2020, 6, 5096–5109 CrossRef CAS PubMed.
- J.-S. Kim, M. Kim, D.-A. Won and G. Tae, Eur. Polym. J., 2015, 72, 632–641 CrossRef CAS.
- P. Manivasagan and J. Oh, Int. J. Biol. Macromol., 2016, 82, 315–327 CrossRef CAS PubMed.
- M. K. Yazdi, F. Seidi, Y. Jin, P. Zarrintaj, H. Xiao, A. Esmaeili, S. Habibzadeh and M. R. Saeb, Polysaccharides, 2021, 283–300 Search PubMed.
- R. A. Ilyas, S. M. Sapuan, R. Ibrahim, H. Abral, M. R. Ishak, E. S. Zainudin, M. S. N. Atikah, N. M. Nurazzi, A. Atiqah, M. N. M. Ansari and E. Syafri, J. Mater. Res. Technol., 2019, 8(5), 4819–4830 CrossRef CAS.
- P. C. McCarthy, Y. Zhang and F. Abebe, Molecules, 2021, 26, 4735 CrossRef CAS PubMed.
- Y. Liu and G. Huang, J. Enzyme Inhib. Med. Chem., 2019, 34, 1690–1696 CrossRef CAS PubMed.
- G. Huang, F. Chen, W. Yang and H. Huang, Trends Food Sci. Technol., 2021, 109, 564–568 CrossRef CAS.
- J. Fang, Z. Wang, P. Wang and M. Wang, Int. J. Biol. Macromol., 2020, 162, 1897–1905 CrossRef CAS PubMed.
- Y. K. Leong, F.-C. Yang and J.-S. Chang, Carbohydr. Polym., 2021, 251, 117006 CrossRef CAS PubMed.
- H. Huang and G. Huang, Chem. Biol. Drug Des., 2020, 96, 1209–1222 CrossRef CAS PubMed.
- M. Mirzadeh, M. R. Arianejad and L. Khedmat, Carbohydr. Polym., 2020, 229, 115421 CrossRef CAS PubMed.
- S. Nigam, R. Singh, S. K. Bhardwaj, R. Sami, M. P. Nikolova, M. Chavali and S. Sinha, J. Polym. Environ., 2021, 1–25 Search PubMed.
- X. Li, F. Xiong, Y. Liu, F. Liu, Z. Hao and H. Chen, Int. J. Biol. Macromol., 2018, 111, 319–325 CrossRef CAS PubMed.
- C. Hou, M. Yin, P. Lan, H. Wang, H. Nie and X. Ji, Chem. Biol. Technol. Agric., 2021, 8, 1–14 CrossRef PubMed.
- L. Xie, M. Shen, Y. Hong, H. Ye, L. Huang and J. Xie, Carbohydr. Polym., 2020, 229, 115436 CrossRef CAS PubMed.
- C. Hou, L. Chen, L. Yang and X. Ji, Int. J. Biol. Macromol., 2020, 153, 248–255 CrossRef CAS PubMed.
- L. Ren, J. Zhang and T. Zhang, Food Chem., 2021, 340, 127933 CrossRef CAS PubMed.
- P. Kalita, A. B. Ahmed, S. Sen and R. Chakraborty, Int. J. Biol. Macromol., 2022, S0141-8130(0122), 00445–00447 Search PubMed.
- W.-X. Duan, X.-H. Yang, H.-F. Zhang, J. Feng and M.-Y. Zhang, Biol. Trace Elem. Res., 2021, 1–15 Search PubMed.
- M. Xie, W. Tao, F. Wu, K. Wu, X. Huang, G. Ling, C. Zhao, Q. Lv, Q. Wang and X. Zhou, Int. J. Biol. Macromol., 2021, 185, 917–934 CrossRef CAS PubMed.
- F. Carvalhal, R. R. Cristelo, D. I. Resende, M. M. Pinto, E. Sousa and M. Correia-da-Silva, Mar. Drugs, 2019, 17, 170 CrossRef CAS PubMed.
- Y. Zhang, Q. Xie, L. You, P. C.-K. Cheung and Z. Zhao, eFood, 2021, 2, 59–72 CrossRef.
- J. Zhang, C. Wen, H. Zhang and Y. Duan, Int. J. Biol. Macromol., 2019, 139, 409–420 CrossRef CAS PubMed.
- X. Sun, Y. Wu, Z. Song and X. Chen, Bioact. Carbohydr. Diet. Fibre, 2022, 27, 100291 CrossRef CAS.
- J. Li, B. Shen, S. Nie, Z. Duan and K. Chen, Carbohydr. Polym., 2019, 206, 163–173 CrossRef CAS PubMed.
- Q. Song, Y. Wang, L. Huang, M. Shen, Y. Yu, Q. Yu, Y. Chen and J. Xie, Food Res. Int., 2021, 140, 109858 CrossRef CAS PubMed.
- L. Chen and G. Huang, Int. J. Biol. Macromol., 2018, 115, 77–82 CrossRef CAS PubMed.
- Q.-H. Gao, X. Fu, R. Zhang, Z. Wang and M. Guo, Int. J. Biol. Macromol., 2018, 106, 749–754 CrossRef CAS PubMed.
- B.-D. Zheng, J. Ye, Y.-C. Yang, Y.-Y. Huang and M.-T. Xiao, Carbohydr. Polym., 2022, 275, 118770 CrossRef CAS PubMed.
- L.-X. Zheng, X.-Q. Chen and K.-L. Cheong, Int. J. Biol. Macromol., 2020, 151, 344–354 CrossRef CAS PubMed.
- Q. Zhong, B. Wei, S. Wang, S. Ke, J. Chen, H. Zhang and H. Wang, Mar. Drugs, 2019, 17, 674 CrossRef CAS PubMed.
- I. Priyan Shanura Fernando, K.-N. Kim, D. Kim and Y.-J. Jeon, Crit. Rev. Biotechnol., 2019, 39, 99–113 CrossRef CAS PubMed.
- L. Zhang, T. Liu, Y. Xie, Z. Zeng and J. Chen, Nanotechnol. Rev., 2020, 9, 820–832 CrossRef CAS.
- J. Ouyang, S. Rao, R. Liu, L. Wang, W. Chen, W. Tao and N. Kong, Adv. Drug Delivery Rev., 2022, 114268 CrossRef CAS PubMed.
- M. A. Salati, J. Khazai, A. M. Tahmuri, A. Samadi, A. Taghizadeh, M. Taghizadeh, P. Zarrintaj, J. D. Ramsey, S. Habibzadeh and F. Seidi, Polymers, 2020, 12, 1150 CrossRef CAS PubMed.
- M. K. Yazdi, A. Taghizadeh, M. Taghizadeh, F. J. Stadler, M. Farokhi, F. Mottaghitalab, P. Zarrintaj, J. D. Ramsey, F. Seidi and M. R. Saeb, J. Controlled Release, 2020, 326, 523–543 CrossRef PubMed.
-
M. Ahmad, K. Manzoor and S. Ikram, Applications of Nanocomposite Materials in Drug Delivery, Elsevier, 2018, pp. 27–38 Search PubMed.
-
Z. Shariatinia, Natural polysaccharides in drug delivery and biomedical applications, Elsevier, 2019, pp. 15–57 Search PubMed.
- B. Duan, P. Sun, X. Wang and C. Yang, Starch-Stärke, 2011, 63, 528–535 CrossRef CAS.
- R. Zafar, K. M. Zia, S. Tabasum, F. Jabeen, A. Noreen and M. Zuber, Int. J. Biol. Macromol., 2016, 92, 1012–1024 CrossRef CAS PubMed.
- A. Khosravi, A. Fereidoon, M. M. Khorasani, G. Naderi, M. R. Ganjali, P. Zarrintaj, M. R. Saeb and T. J. Gutiérrez, Food Packag. Shelf Life, 2020, 23, 100429 CrossRef.
- M. U. A. Khan, S. Haider, S. A. Shah, S. I. Abd Razak, S. A. Hassan, M. R. A. Kadir and A. Haider, Int. J. Biol. Macromol., 2020, 151, 584–594 CrossRef CAS PubMed.
- F. M. Ghorbani, B. Kaffashi, P. Shokrollahi, E. Seyedjafari and A. Ardeshirylajimi, Carbohydr. Polym., 2015, 118, 133–142 CrossRef CAS PubMed.
- K. B. Narayanan, S. M. Zo and S. S. Han, Int. J. Biol. Macromol., 2020, 149, 724–731 CrossRef CAS PubMed.
- S. Azizian, A. Hadjizadeh and H. Niknejad, Carbohydr. Polym., 2018, 202, 315–322 CrossRef CAS PubMed.
- H. Shokrani, A. Shokrani, S. M. Sajadi, F. Seidi, A. H. Mashhadzadeh, N. Rabiee, M. R. Saeb, T. Aminabhavi and T. J. Webster, Int. J. Nanomed., 2022, 17, 1035 CrossRef CAS PubMed.
- T. D. Andreeva, S. Stoichev, S. G. Taneva and R. Krastev, Carbohydr. Polym., 2018, 181, 78–85 CrossRef CAS PubMed.
- Z. Qin, Y. Zheng, Y. Wang, T. Du, C. Li, X. Wang and H. Jiang, Coord. Chem. Rev., 2021, 449, 214218 CrossRef CAS.
- V. Bertolino, G. Cavallaro, S. Milioto and G. Lazzara, Carbohydr. Polym., 2020, 245, 116502 CrossRef CAS PubMed.
- S. Ferraris, I. Corazzari, F. Turci, A. Cochis, L. Rimondini and E. Vernè, ACS Biomater. Sci. Eng., 2021, 7, 2309–2316 CrossRef CAS PubMed.
- M. Delyanee, A. Solouk, S. Akbari and M. Daliri Joupari, Polym. Adv. Technol., 2021, 32, 3934–3947 CrossRef CAS.
- I. Gholamali, Regener. Eng. Transl. Med., 2021, 7, 91–114 CrossRef CAS.
- T. Thambi, V. G. Phan and D. S. Lee, Macromol. Rapid Commun., 2016, 37, 1881–1896 CrossRef CAS PubMed.
- P. Sabourian, M. Tavakolian, H. Yazdani, M. Frounchi, T. G. van de Ven, D. Maysinger and A. Kakkar, J. Controlled Release, 2020, 317, 216–231 CrossRef CAS PubMed.
- C. Maity and N. Das, Top. Curr. Chem., 2022, 380, 1–67 CrossRef PubMed.
- C. He, H. Ji, Y. Qian, Q. Wang, X. Liu, W. Zhao and C. Zhao, J. Mater. Chem. B, 2019, 7, 1186–1208 RSC.
- X. Zhao, J. Bai and W. Yang, Cancer Biol. Med., 2021, 18, 319 Search PubMed.
- R. Nasseri, C. Deutschman, L. Han, M. Pope and K. Tam, Mater. Today Adv., 2020, 5, 100055 CrossRef.
- Y. Zhang, Y. Thomas, E. Kim and G. F. Payne, J. Phys. Chem. B, 2012, 116, 1579–1585 CrossRef CAS PubMed.
- D.-q Li, S.-y Wang, Y.-j Meng, Z.-w Guo, M.-m Cheng and J. Li, Carbohydr. Polym., 2021, 268, 118244 CrossRef CAS PubMed.
- Y. Fu, Z. Wan, G. Zhao, W. Jia and H. Zhao, Smart Mater. Struct., 2021, 30, 075027 CrossRef CAS.
- C. Liu, R. Wang, Y. Sun, C. Yin, Z. Gu, W. Wu and X. Jiang, ACS Cent. Sci., 2022, 8, 258–267 CrossRef CAS PubMed.
- Z. Zare-Akbari, H. Farhadnejad, B. Furughi-Nia, S. Abedin, M. Yadollahi and M. Khorsand-Ghayeni, Int. J. Biol. Macromol., 2016, 93, 1317–1327 CrossRef CAS PubMed.
-
N. Vignesh, K. Chandraraj, S. Suriyaraj and R. Selvakumar, Advanced Materials for Biomechanical Applications, CRC Press, 2022, pp. 59–83 Search PubMed.
- S. Hosseinzadeh, H. Hosseinzadeh, S. Pashaei and Z. Khodaparast, Int. J. Biol. Macromol., 2019, 121, 677–685 CrossRef CAS PubMed.
- K. Soleimani, H. Derakhshankhah, M. Jaymand and H. Samadian, Carbohydr. Polym., 2020, 117422 Search PubMed.
- T. Anirudhan, F. Shainy and J. P. Thomas, Int. J. Biol. Macromol., 2019, 135, 776–789 CrossRef PubMed.
-
M. K. Yazdi, P. Zarrintaj, M. Ghavami, R. Alizadeh and M. R. Saeb, Nanoengineered Biomaterials for Advanced Drug Delivery, Elsevier, 2020, pp. 145–161 Search PubMed.
-
P. Zarrintaj, M. K. Yazdi, B. Bagheri, Y. C. Kim, J. D. Ramsey and M. R. Saeb, Nanoengineered Biomaterials for Advanced Drug Delivery, Elsevier, 2020, pp. 181–200 Search PubMed.
-
M. K. Yazdi, M. R. Ganjali, M. Rezapour, P. Zarrintaj, S. Habibzadeh and M. R. Saeb, Ionically Gelled Biopolysaccharide Based Systems in Drug Delivery, Springer, 2021, pp. 121–133 Search PubMed.
-
M. K. Yazdi, M. R. Ganjali, P. Zarrintaj, B. Bagheri, Y. C. Kim and M. R. Saeb, Ionically Gelled
Biopolysaccharide Based Systems in Drug Delivery, Springer, 2021, pp. 93–103 Search PubMed.
- S. Voci, M. Fresta and D. Cosco, J. Controlled Release, 2021, 329, 385–400 CrossRef CAS PubMed.
- S. Das, B. Ghosh and K. Sarkar, Sens. Int., 2022, 3, 100135 CrossRef.
- Q. Yu, Y. Wang, X. Cao, W. Deng, M. Adu Frimpong, J. Yu and X. Xu, Adv. Polym. Technol., 2019, 2019, 801–809 CrossRef.
-
I. D. Ana, Functional Biomaterials, Springer, 2022, pp. 409–434 Search PubMed.
- S. Kunjiappan, P. Pavadai, S. Vellaichamy, S. Ram Kumar Pandian, V. Ravishankar, P. Palanisamy, S. Govindaraj, G. Srinivasan, A. Premanand and M. Sankaranarayanan, Drug Dev. Res., 2021, 82, 309–340 CrossRef CAS PubMed.
- M. J. Mitchell, M. M. Billingsley, R. M. Haley, M. E. Wechsler, N. A. Peppas and R. Langer, Nat. Rev. Drug Discovery, 2021, 20, 101–124 CrossRef CAS PubMed.
- Y. Liang, L. Duan, J. Lu and J. Xia, Theranostics, 2021, 11, 3183 CrossRef CAS PubMed.
- S. Jaiswal, P. Dutta, S. Kumar and R. Chawla, J. Drug Delivery Sci. Technol., 2021, 62, 102407 CrossRef CAS.
- A. O. Elzoghby, M. M. Abd-Elwakil, K. Abd-Elsalam, M. T. Elsayed, Y. Hashem and O. Mohamed, Curr. Pharm. Des., 2016, 22, 3305–3323 CrossRef CAS PubMed.
- S. Karnik, K. Hines and D. K. Mills, J. Biomed. Mater. Res., Part A, 2015, 103, 2416–2426 CrossRef CAS PubMed.
- N. Rabiee, M. Bagherzadeh, M. Kiani, A. M. Ghadiri, F. Etessamifar, A. H. Jaberizadeh and A. Shakeri, Int. J. Nanomed., 2020, 15, 3983 CrossRef CAS PubMed.
- S. P. Bandi, S. Bhatnagar and V. V. K. Venuganti, Acta Biomater., 2021, 119, 13–29 CrossRef CAS PubMed.
- M. Đuranović, S. Obeid, M. Madžarević, S. Cvijić and S. Ibrić, Int. J. Pharm., 2021, 592, 120053 CrossRef PubMed.
- M. K. Yazdi, P. Zarrintaj, H. Hosseiniamoli, A. H. Mashhadzadeh, M. R. Saeb, J. D. Ramsey, M. R. Ganjali and M. Mozafari, J. Mater. Chem. B, 2020, 8, 5992–6012 RSC.
- J. C. Fricain, S. Schlaubitz, C. Le Visage, I. Arnault, S. M. Derkaoui, R. Siadous, S. Catros, C. Lalande, R. Bareille and M. Renard, Biomaterials, 2013, 34, 2947–2959 CrossRef CAS PubMed.
- M. Wang, W. Deng, M. Fu, X. Cao, Y. Yang, W. Su, J. Yu and X. Xu, Carbohydr. Polym., 2011, 86, 1509–1518 CrossRef CAS.
- Z. He, X. Zhou, Y. Wang, J. Lin, S. Huang, R. Hu, Y. Zhou, Q. Qian and H. Deng, Carbohydr. Polym., 2021, 273, 118525 CrossRef CAS PubMed.
- S. P. Malliappan, A. A. Yetisgin, S. B. Sahin, E. Demir and S. Cetinel, Carbohydr. Polym., 2022, 119142 CAS.
- S. Patnaik, A. Aggarwal, S. Nimesh, A. Goel, M. Ganguli, N. Saini, Y. Singh and K. Gupta, J. Controlled Release, 2006, 114, 398–409 CrossRef CAS PubMed.
- F. Ding, X. Gao, X. Huang, H. Ge, M. Xie, J. Qian, J. Song, Y. Li, X. Zhu and C. Zhang, Biomaterials, 2020, 245, 119976 CrossRef CAS PubMed.
-
M. A. Ansari, M. K. Yadav, D. Rathore, A. Svedberg and Z. Karim, Nanostructured Polymer Composites for Biomedical Applications, Elsevier, 2019, pp. 211–226 Search PubMed.
- X. Zhang, D. Wei, Y. Xu and Q. Zhu, Carbohydr. Polym., 2021, 264, 118006 CrossRef CAS PubMed.
- S. Vasvani, P. Kulkarni and D. Rawtani, Int. J. Biol. Macromol., 2020, 151, 1012–1029 CrossRef CAS PubMed.
- P. Apaolaza, M. Busch, E. Asin-Prieto, K. Peynshaert, R. Rathod, K. Remaut, N. Dünker and A. Göpferich, Exp. Eye Res., 2020, 198, 108151 CrossRef CAS PubMed.
- A. M. Burhan, B. Klahan, W. Cummins, V. Andrés-Guerrero, M. E. Byrne, N. J. O’Reilly, A. Chauhan, L. Fitzhenry and H. Hughes, Pharmaceutics, 2021, 13, 1685 CrossRef CAS PubMed.
- B. Kumara, R. Shambhu and K. S. Prasad, Int. J. Biol. Macromol., 2021, 176, 47–65 CrossRef CAS PubMed.
- K. I. Kashkouli, M. Torkzadeh-Mahani and E. Mosaddegh, Mater. Sci. Eng., C, 2018, 89, 166–174 CrossRef PubMed.
- N. Rabiee, M. Bagherzadeh, A. M. Ghadiri, M. Kiani, S. Ahmadi, V. Jajarmi, Y. Fatahi, A. Aldhaher, M. Tahriri and T. J. Webster, J. Nanostruct. Chem., 2021, 1–14 Search PubMed.
- M. Bagherzadeh, N. Rabiee, Y. Fatahi and R. Dinarvand, New J. Chem., 2021, 45, 4077–4089 RSC.
- Z. Shariatinia and Z. Zahraee, J. Colloid Interface Sci., 2017, 501, 60–76 CrossRef CAS PubMed.
- R. Rakhshaei, H. Namazi, H. Hamishehkar, H. S. Kafil and R. Salehi, J. Appl. Polym. Sci., 2019, 136, 47590 CrossRef.
- M. Ahmadi, M. Mehdikhani, J. Varshosaz, S. Farsaei and H. Torabi, J. Biomater. Appl., 2021, 35, 958–977 CrossRef CAS PubMed.
- R. Wang, D. Shou, O. Lv, Y. Kong, L. Deng and J. Shen, Int. J. Biol. Macromol., 2017, 103, 248–253 CrossRef CAS PubMed.
- J. Ren, L. Han, H. Cai, K. Wu, L. Avérous and W. Guo, Starch-Stärke, 2018, 70, 1700358 CrossRef.
- H. Namazi, M. Pooresmaeil and M. Hasani, Int. J. Polym. Mater. Polym. Biomater., 2020, 1–10 Search PubMed.
- M. Arjama, S. Mehnath, M. Rajan and M. Jeyaraj, Int. J. Biol. Macromol., 2018, 120, 1561–1571 CrossRef CAS PubMed.
- I. Kohsari, Z. Shariatinia and S. M. Pourmortazavi, Carbohydr. Polym., 2016, 140, 287–298 CrossRef CAS PubMed.
- M. U. Aslam Khan, H. Mehboob, S. I. Abd Razak, M. Y. Yahya, A. H. Mohd Yusof, M. H. Ramlee, T. J. Sahaya Anand, R. Hassan, A. Aziz and R. Amin, Polymers, 2020, 12, 1238 CrossRef PubMed.
- P. Nechita, Coatings, 2020, 10, 566 CrossRef CAS.
- F. Liu, X. Liu, F. Chen and Q. Fu, Prog. Polym. Sci., 2021, 123, 101472 CrossRef CAS.
- S. Shen, X. Chen, Z. Shen and H. Chen, Pharmaceutics, 2021, 13, 1666 CrossRef CAS PubMed.
- I. Kohsari, Z. Shariatinia and S. M. Pourmortazavi, Int. J. Biol. Macromol., 2016, 91, 778–788 CrossRef CAS PubMed.
- H. Babapour, H. Jalali and A. Mohammadi Nafchi, Food Sci. Nutr., 2021, 9, 3893–3905 CrossRef CAS PubMed.
- N. Tamimi, A. Mohammadi Nafchi, H. Hashemi-Moghaddam and H. Baghaie, Food Sci. Nutr., 2021, 9, 4497–4508 CrossRef CAS PubMed.
- A. A. Ahmad and N. M. Sarbon, Food Biosci., 2021, 43, 101250 CrossRef CAS.
- Y. He, H. Li, X. Fei and L. Peng, Carbohydr. Polym., 2021, 252, 117156 CrossRef CAS PubMed.
- D. S. B. Anugrah, H. Alexander, R. Pramitasari, D. Hudiyanti and C. P. Sagita, Coatings, 2020, 10, 988 CrossRef CAS.
- I. Zia, S. Mirza, R. Jolly, A. Rehman, R. Ullah and M. Shakir, Int. J. Biol. Macromol., 2019, 124, 88–101 CrossRef CAS PubMed.
- H.-T. Lu, T.-W. Lu, C.-H. Chen and F.-L. Mi, Int. J. Biol. Macromol., 2019, 128, 973–984 CrossRef CAS PubMed.
- Z. Shariatinia, Z. Nikfar, K. Gholivand and S. Abolghasemi Tarei, Polym. Compos., 2015, 36, 454–466 CrossRef CAS.
- S. Mirza, I. Zia, R. Jolly, S. Kazmi, M. Owais and M. Shakir, Int. J. Biol. Macromol., 2018, 119, 215–224 CrossRef CAS PubMed.
- Y. Chen, L. Wu, P. Li, X. Hao, X. Yang, G. Xi, W. Liu, Y. Feng, H. He and C. Shi, Macromol. Biosci., 2020, 20, 1900370 CrossRef CAS PubMed.
- L. Zhu, S. Zhang, H. Zhang, L. Dong, Y. Cong, S. Sun and X. Sun, J. Drug Delivery Sci. Technol., 2021, 66, 102890 CrossRef CAS.
- Y. Zhong, H. Hu, N. Min, Y. Wei, X. Li and X. Li, Ann. Transl. Med., 2021, 9, 577 CrossRef CAS PubMed.
- L. Wang, X. You, C. Dai, T. Tong and J. Wu, Biomater. Sci., 2020, 8, 4396–4412 RSC.
- X. X. Wang, Q. Liu, J. X. Sui, S. Ramakrishna, M. Yu, Y. Zhou, X. Y. Jiang and Y. Z. Long, Adv. Healthcare Mater., 2019, 8, 1900823 CrossRef CAS PubMed.
- W. Jirong, G. Hai, L. Guangzhao and D. Li, J. Pharm. Pract., 2021, 39, 211–214 Search PubMed.
- M. Fang, G.-J. Huang and W.-C. Sung, LWT, 2021, 137, 110494 CrossRef CAS.
- H. M. Srivastava, M. Irfan and F. A. Shah, Energies, 2021, 14, 2254 CrossRef.
- M. Hondzo, J. You, J. Taylor, G. Bartlet and V. R. Voller, Geophys. Res. Lett., 2022, e2021GL093226 Search PubMed.
- T. Yamamoto, H. Iwase, T. W. King, H. Hara and D. K. Cooper, Burns, 2018, 44, 1738–1749 CrossRef PubMed.
- K. C. S. Roballo and J. Bushman, Trans. Immunol., 2019, 53, 61–71 CrossRef CAS PubMed.
- D. M. L. Ribeiro, A. R. Carvalho Júnior, G. H. R. Vale de Macedo, V. L. Chagas, L. D. S. Silva, B. D. S. Cutrim, D. M. Santos, B. L. L. Soares, A. Zagmignan and R. D. C. M. de Miranda, Biomolecules, 2020, 10, 63 CrossRef CAS PubMed.
- M. Shakir, I. Zia, A. Rehman and R. Ullah, Int. J. Biol. Macromol., 2018, 111, 903–916 CrossRef CAS PubMed.
- J. A. S. Quaresma, Clin. Microbiol. Rev., 2019, 32, e00034–00018 CrossRef CAS PubMed.
- A. Kaur, S. Midha, S. Giri and S. Mohanty, Stem Cells Int., 2019, 2019, 1286054 Search PubMed.
- R. Tarrahi, A. Khataee, A. Karimi and Y. Yoon, Chemosphere, 2022, 288, 132529 CrossRef CAS PubMed.
- H. Shokrani, A. Shokrani, M. Jouyandeh, F. Seidi, F. Gholami, S. Kar, M. T. Munir, D. Kowalkowska-Zedler, P. Zarrintaj and N. Rabiee, ACS Appl. Bio Mater., 2022, 5, 2107–2121 CrossRef CAS PubMed.
- M. Liu, X. Zeng, C. Ma, H. Yi, Z. Ali, X. Mou, S. Li, Y. Deng and N. He, Bone Res., 2017, 5, 1–20 CrossRef PubMed.
- H. Nosrati, M. Khodaei, Z. Alizadeh and M. Banitalebi-Dehkordi, Int. J. Biol. Macromol., 2021, 192, 298–322 CrossRef CAS PubMed.
- Y. He, Y. Li, Y. Sun, S. Zhao, M. Feng, G. Xu, H. Zhu, P. Ji, H. Mao and Y. He, Carbohydr. Polym., 2021, 261, 117870 CrossRef CAS PubMed.
- V. Dadwal, R. Joshi and M. Gupta, LWT, 2021, 152, 112290 CrossRef CAS.
- S. Kumari, B. N. Singh and P. Srivastava, 3 Biotechnol., 2019, 9, 1–14 Search PubMed.
- M. Mahdavi, N. Mahmoudi, F. Rezaie Anaran and A. Simchi, Mar. Drugs, 2016, 14, 128 CrossRef PubMed.
- A. Huang, X. Peng, L. Geng, L. Zhang, K. Huang, B. Chen, Z. Gu and T. Kuang, Polym. Test., 2018, 71, 101–109 CrossRef CAS.
- Z. Keskin, A. S. Urkmez and E. E. Hames, Mater. Sci. Eng., C, 2017, 75, 1144–1153 CrossRef CAS PubMed.
- Z. P. Rad, J. Mokhtari and M. Abbasi, Int. J. Biol. Macromol., 2019, 135, 530–543 CrossRef PubMed.
- Z. P. Rad, J. Mokhtari and M. Abbasi, Mater. Sci. Eng., C, 2018, 93, 356–366 CrossRef PubMed.
- Z. P. Rad, J. Mokhtari and M. Abbasi, Iran. Polym. J., 2019, 28, 51–63 CrossRef.
- A.-T. Iacob, M. Drăgan, O.-M. Ionescu, L. Profire, A. Ficai, E. Andronescu, L. G. Confederat and D. Lupaşcu, Pharmaceutics, 2020, 12, 983 CrossRef CAS PubMed.
- T. Zhu, J. Mao, Y. Cheng, H. Liu, L. Lv, M. Ge, S. Li, J. Huang, Z. Chen and H. Li, Adv. Mater. Interfaces, 2019, 6, 1900761 CrossRef.
- J.-H. Cherng, Wound Healing-Current Perspectives, 2018, 65–80 Search PubMed.
- M. Sattary, A. Kefayat, A. Bigham and M. Rafienia, Mater. Technol., 2020, 1–14 Search PubMed.
- P. Zadehnajar, S. Karbasi, B. Akbari and L. Ghasemi, Mater. Technol., 2020, 35, 39–49 CrossRef CAS.
- S. Gautam, C. Sharma, S. D. Purohit, H. Singh, A. K. Dinda, P. D. Potdar, C.-F. Chou and N. C. Mishra, Mater. Sci. Eng., C, 2021, 119, 111588 CrossRef CAS PubMed.
-
S. S. Murugan, S. Anil and J. Venkatesan, Polysaccharide Nanoparticles, Elsevier, 2022, pp. 603–614 Search PubMed.
- G. P. Chaves Filho, M. E. G. B. Lima, H. A. de Oliveira Rocha and S. M. G. Moreira, Carbohydr. Polym., 2022, 119204 CrossRef CAS PubMed.
- T. Fan, J. Chen, P. Pan, Y. Zhang, Y. Hu, X. Liu, X. Shi and Q. Zhang, Colloids Surf., B, 2016, 147, 217–223 CrossRef CAS PubMed.
- K. Qiao, L. Xu, J. Tang, Q. Wang, K. S. Lim, G. Hooper, T. B. Woodfield, G. Liu, K. Tian and W. Zhang, J. Nanobiotechnol., 2022, 20, 1–42 CrossRef PubMed.
-
A. Singh, K. Kumari and P. Kundu, Engineered Nanomaterials for Innovative Therapies and Biomedicine, Springer, 2022, pp. 373–403 Search PubMed.
-
A. K. Maurya and N. Mishra, Tissue Engineering, Apple Academic Press, 2022, pp. 59–88 Search PubMed.
- L. Song, Q. Guo, J. Guo, X. Xu, K. Xu, Y. Li, T. Yang, X. Gu, R. Cao and S. Cui, J. Neural Eng., 2022, 19, 026010 CrossRef PubMed.
- S. Zhang, Y. Zhou, H. Xian, Y. Shi, Y. Liu, Z. Li and Y. Huang, Eur. J. Neurosci., 2022, 55, 1895–1916 CrossRef PubMed.
- F. Zhang, M. Zhang, S. Liu, C. Li, Z. Ding, T. Wan and P. Zhang, Gels, 2022, 8, 41 CrossRef CAS PubMed.
- W. S. Horne and T. N. Grossmann, Nat. Chem., 2020, 12, 331–337 CrossRef CAS PubMed.
- S. Soltani, M. Ebrahimian-Hosseinabadi and A. Z. Kharazi, Tissue Eng. Regener. Med., 2016, 13, 684–690 CrossRef CAS PubMed.
- M. A. Shokrgozar, F. Mottaghitalab, V. Mottaghitalab and M. Farokhi, J. Biomed. Nanotechnol., 2011, 7, 276–284 CrossRef CAS PubMed.
- A. Karimi, S. Karbasi, S. Razavi and E. N. Zargar, Adv. Biomed. Res., 2018, 7, 44 CrossRef PubMed.
- Y.-L. Lin, J.-C. Jen, S.-H. Hsu and M. Chiu, Surgical Neurology, 2008, 70, S9–S18 CrossRef PubMed.
- A. Manzari-Tavakoli, R. Tarasi, R. Sedghi, A. Moghimi and H. Niknejad, Sci. Rep., 2020, 10, 1–10 CrossRef PubMed.
- H. Baniasadi, A. R. Sa and S. Mashayekhan, Int. J. Biol. Macromol., 2015, 74, 360–366 CrossRef CAS PubMed.
- B. Bagheri, P. Zarrintaj, S. S. Surwase, N. Baheiraei, M. R. Saeb, M. Mozafari, Y. C. Kim and O. O. Park, Colloids Surf., B, 2019, 184, 110549 CrossRef CAS PubMed.
- P. Zarrintaj, A. M. Urbanska, S. S. Gholizadeh, V. Goodarzi, M. R. Saeb and M. Mozafari, J. Colloid Interface Sci., 2018, 516, 57–66 CrossRef CAS PubMed.
- M. Habibizadeh, S. Nadri, A. Fattahi, K. Rostamizadeh, P. Mohammadi, S. Andalib, M. Hamidi and N. Forouzideh, J. Biomed. Mater. Res., Part A, 2021, 109, 2237–2254 CrossRef CAS PubMed.
- W. Wei, Y. Ma, X. Yao, W. Zhou, X. Wang, C. Li, J. Lin, Q. He, S. Leptihn and H. Ouyang, Bioactive Mater., 2021, 6, 998–1011 CrossRef CAS PubMed.
- P. Baei, H. Daemi, F. Mostafaei, F. A. Sayahpour, H. Baharvand and M. B. Eslaminejad, Chem. Eng. J., 2021, 418, 129277 CrossRef CAS.
-
R. Cassano, F. Curcio, M. L. Di Gioia, D. Procopio and S. Trombino, Tissue Engineering, Apple Academic Press, 2022, pp. 275–308 Search PubMed.
- Z. Mohammadalizadeh, S. Karbasi and S. Arasteh, Polym.-Plast. Technol. Mater., 2020, 59, 417–429 CAS.
- A. Kaviani, S. M. Zebarjad, S. Javadpour, M. Ayatollahi and R. Bazargan-Lari, Int. J. Polym. Anal. Charact., 2019, 24, 191–203 CrossRef CAS.
- F. R. Tentor, J. H. de Oliveira, D. B. Scariot, D. Lazarin-Bidoia, E. G. Bonafe, C. V. Nakamura, S. A. Venter, J. P. Monteiro, E. C. Muniz and A. F. Martins, Int. J. Biol. Macromol., 2017, 102, 1186–1194 CrossRef CAS PubMed.
- P. Kazimierczak, A. Benko, M. Nocun and A. Przekora, Int. J. Nanomed., 2019, 14, 6615 CrossRef CAS PubMed.
- H.-T. Lu, T.-W. Lu, C.-H. Chen, K.-Y. Lu and F.-L. Mi, Int. J. Biol. Macromol., 2018, 120, 2335–2345 CrossRef CAS PubMed.
- Y. Zhou, K. Liang, S. Zhao, C. Zhang, J. Li, H. Yang, X. Liu, X. Yin, D. Chen and W. Xu, Int. J. Biol. Macromol., 2018, 108, 383–390 CrossRef CAS PubMed.
- E. B. Toloue, S. Karbasi, H. Salehi and M. Rafienia, J. Med. Signals Sens., 2019, 9, 111 CrossRef PubMed.
- S. Keikhaei, Z. Mohammadalizadeh, S. Karbasi and A. Salimi, Mater. Technol., 2019, 34, 615–625 CrossRef CAS.
- S. Karbasi and Z. M. Alizadeh, Bull. Mater. Sci., 2017, 40, 1247–1253 CrossRef CAS.
- G. Sandri, C. Aguzzi, S. Rossi, M. C. Bonferoni, G. Bruni, C. Boselli, A. I. Cornaglia, F. Riva, C. Viseras and C. Caramella, Acta Biomater., 2017, 57, 216–224 CrossRef CAS PubMed.
- A. Abedi, M. Hasanzadeh and L. Tayebi, Mater. Chem. Phys., 2019, 237, 121882 CrossRef CAS.
- A. Sepahvandi, M. Eskandari and F. Moztarzadeh, Mater. Sci. Eng., C, 2016, 66, 306–314 CrossRef CAS PubMed.
- S. Del Buffa, E. Rinaldi, E. Carretti, F. Ridi, M. Bonini and P. Baglioni, Colloids Surf., B, 2016, 145, 562–566 CrossRef CAS PubMed.
- S. Saber-Samandari, S. Saber-Samandari, S. Kiyazar, J. Aghazadeh and A. Sadeghi, Int. J. Biol. Macromol., 2016, 86, 434–442 CrossRef CAS PubMed.
- J. V. Kumbhar, S. H. Jadhav, D. S. Bodas, A. Barhanpurkar-Naik, M. R. Wani, K. M. Paknikar and J. M. Rajwade, Int. J. Nanomed., 2017, 12, 6437 CrossRef CAS PubMed.
- N. Naseri, J.-M. Poirier, L. Girandon, M. Fröhlich, K. Oksman and A. P. Mathew, RSC Adv., 2016, 6, 5999–6007 RSC.
- S. D. Purohit, H. Singh, R. Bhaskar, I. Yadav, S. Bhushan, M. K. Gupta, A. Kumar and N. C. Mishra, Front. Mater., 2020, 7, 1–10 CrossRef.
- P. Wu, X. Xi, R. Li and G. Sun, Macromol. Biosci., 2021, 21, 2100141 CrossRef CAS PubMed.
- N. C. Ngwuluka, N. Y. Abu-Thabit, O. J. Uwaezuoke, J. O. Erebor, M. O. Ilomuanya, R. R. Mohamed, S. Soliman, M. Elella and N. Ebrahim, Nano Microencapsul Tech. Appl., 2020 DOI:10.5772/intechopen.94856.
- A. Thakur, M. K. Jaiswal, C. W. Peak, J. K. Carrow, J. Gentry, A. Dolatshahi-Pirouz and A. K. Gaharwar, Nanoscale, 2016, 8, 12362–12372 RSC.
-
S. Liu, X. Chen and Y. Zhang, 3D and 4D Printing of Polymer Nanocomposite Materials, Elsevier, 2020, pp. 427–465 Search PubMed.
- X. Zhang, Y. Meng, W. Shen, J. Dou, R. Liu, Q. Jin and S. Fang, React. Funct. Polym., 2021, 158, 104773 CrossRef CAS.
- W. Xu, J. Wang, J. Qian, G. Hou, Y. Wang, L. Ji and A. Suo, Mater. Sci. Eng., C, 2019, 103, 109854 CrossRef CAS PubMed.
- P. Makvandi, Z. Baghbantaraghdari, W. Zhou, Y. Zhang, R. Manchanda, T. Agarwal, A. Wu, T. K. Maiti, R. S. Varma and B. R. Smith, Biotechnol. Adv., 2021, 48, 107711 CrossRef CAS PubMed.
- S. Zhang, G. Pang, C. Chen, J. Qin, H. Yu, Y. Liu, X. Zhang, Z. Song, J. Zhao and F. Wang, Carbohydr. Polym., 2019, 205, 192–202 CrossRef CAS PubMed.
- D. Feldman, Appl. Sci., 2019, 9, 3899 CrossRef CAS.
- S. Z. N. Ahmad, W. N. W. Salleh, A. F. Ismail, N. Yusof, M. Z. M. Yusop and F. Aziz, Chemosphere, 2020, 248, 126008 CrossRef CAS PubMed.
- T. Anirudhan, V. C. Sekhar and V. Athira, Int. J. Biol. Macromol., 2020, 150, 468–479 CrossRef CAS PubMed.
- X. Liu, X. Cheng, F. Wang, L. Feng, Y. Wang, Y. Zheng and R. Guo, Carbohydr. Polym., 2018, 185, 85–95 CrossRef CAS PubMed.
-
M. T. Ansari, M. S. Hasnain, A. K. Nayak and E.-R. Kenawy, Chitosan in Drug Delivery, Elsevier, 2022, pp. 411–432 Search PubMed.
- N. Rabiee, M. Bagherzadeh, A. M. Ghadiri, Y. Fatahi, A. Aldhaher, P. Makvandi, R. Dinarvand, M. Jouyandeh, M. R. Saeb and M. Mozafari, ACS Appl. Bio Mater., 2021, 4, 5336–5351 CrossRef CAS PubMed.
- M. Saadat, F. Mostafaei, S. Mahdinloo, M. Abdi, F. Zahednezhad, P. Zakeri-Milani and H. Valizadeh, J. Drug Delivery Sci. Technol., 2021, 63, 102557 CrossRef CAS.
- W. Song, X. Su, D. A. Gregory, W. Li, Z. Cai and X. Zhao, Nanomaterials, 2018, 8, 907 CrossRef PubMed.
- L. Song, X. Zhou, X. Dai, R. Wang, G. Cheng, N. Zhao and F.-J. Xu, NPG Asia Mater., 2018, 10, 509–521 CrossRef CAS.
- B. Duan, M. Li, Y. Sun, S. Zou and X. Xu, Adv. Healthcare Mater., 2019, 8, 1801389 CrossRef PubMed.
- T. S. Anirudhan and J. Christa, New J. Chem., 2017, 41, 11979–11990 RSC.
- S. Shi, R. Vissapragada, J. Abi Jaoude, C. Huang, A. Mittal, E. Liu, J. Zhong and V. Kumar, Bioactive Mater., 2020, 5, 233–240 CrossRef PubMed.
- G. Yilmaz, E. Guler, F. B. Barlas, S. Timur and Y. Yagci, Macromol. Rapid Commun., 2016, 37, 1046–1051 CrossRef CAS PubMed.
- W. Ngwa, F. Boateng, R. Kumar, D. J. Irvine, S. Formenti, T. Ngoma, C. Herskind, M. R. Veldwijk, G. L. Hildenbrand and M. Hausmann, Int. J. Radiat. Oncol., Biol., Phys., 2017, 97, 624–637 CrossRef CAS PubMed.
- C. Du, M. Zhou, F. Jia, L. Ruan, H. Lu, J. Zhang, B. Zhu, X. Liu, J. Chen and Z. Chai, Biomaterials, 2021, 269, 120642 CrossRef CAS PubMed.
- M. L. Bookstaver, S. J. Tsai, J. S. Bromberg and C. M. Jewell, Trends Immunol., 2018, 39, 135–150 CrossRef CAS PubMed.
- J. M. Gammon, N. M. Dold and C. M. Jewell, Oncotarget, 2016, 7, 15421 CrossRef PubMed.
- G. A. Paredes-Juarez, B. J. De Haan, M. M. Faas and P. De Vos, Materials, 2014, 7, 2087–2103 CrossRef PubMed.
- A. Bibi, S.-U. Rehman and A. Yaseen, Mater. Res. Express, 2019, 6, 092001 CrossRef CAS.
- X. Yu, T. Wen, P. Cao, L. Shan and L. Li, J. Colloid Interface Sci., 2019, 556, 258–265 CrossRef CAS PubMed.
- L. Ji, F. Zhang, L. Zhu and J. Jiang, Int. J. Biol. Macromol., 2021, 170, 459–468 CrossRef CAS PubMed.
- D. Massana Roquero, O. Smutok, A. Othman, A. Melman and E. Katz, ACS Appl. Bio Mater., 2021, 4, 8487–8497 CrossRef CAS PubMed.
- E. Rostami, Polym. Bull., 2021, 1–20 Search PubMed.
- H. Yan, X. Chen, C. Bao, J. Yi, M. Lei, C. Ke, W. Zhang and Q. Lin, Colloids Surf., B, 2020, 191, 110983 CrossRef CAS PubMed.
- D. G. Leach, S. Young and J. D. Hartgerink, Acta Biomater., 2019, 88, 15–31 CrossRef CAS PubMed.
- S. Yan, Z. Luo, Z. Li, Y. Wang, J. Tao, C. Gong and X. Liu, Angew. Chem., 2020, 132, 17484–17495 CrossRef.
- P. S. Hegde and D. S. Chen, Immunity, 2020, 52, 17–35 CrossRef CAS PubMed.
- L.-Y. Li, Y.-M. Zhou, R.-Y. Gao, X.-C. Liu, H.-H. Du, J.-L. Zhang, X.-C. Ai, J.-P. Zhang, L.-M. Fu and L. H. Skibsted, Biomaterials, 2019, 190, 86–96 CrossRef PubMed.
- M. Chen and Q. Chen, Biomater. Sci., 2020, 8, 5846–5858 RSC.
- L. Guo, D. D. Yan, D. Yang, Y. Li, X. Wang, O. Zalewski, B. Yan and W. Lu, ACS Nano, 2014, 8, 5670–5681 CrossRef CAS PubMed.
- Q. Chen, M. Chen and Z. Liu, Chem. Soc. Rev., 2019, 48, 5506–5526 RSC.
- T. Ding, Y. Xing, Z. Wang, H. Guan, L. Wang, J. Zhang and K. Cai, Nanoscale Horiz., 2019, 4, 652–657 RSC.
- Y. Wang, Y. Wu, K. Li, S. Shen, Z. Liu and D. Wu, Adv. Funct. Mater., 2019, 29, 1805582 CrossRef.
- H. Chen, H. Chen, Y. Wang, Y. Bai, P. Yuan, Z. Che and L. Zhang, Colloids Surf., B, 2021, 200, 111596 CrossRef CAS PubMed.
- T. Wang, K. Niu, S. Ni, W. Zhang, Z. Liu and X. Zhang, ACS Sustainable Chem. Eng., 2022, 1585–1594 CrossRef CAS.
- P. Samyn, Int. J. Biol. Macromol., 2021, 178, 71–93 CrossRef CAS PubMed.
- Z. Liao, W. Zhang, Z. Qiao, J. Luo, A. Erpuding, A. Niwaer, X. Meng, H. Wang, X. Li and F. Zuo, J. Colloid Interface Sci., 2020, 562, 81–90 CrossRef CAS PubMed.
- K. Shen, Y. Huang, Q. Li, M. Chen and L. Wu, ACS Omega, 2019, 4, 18118–18125 CrossRef CAS PubMed.
- K. F. Akhter, M. A. Mumin, E. M. Lui and P. A. Charpentier, Int. J. Biol. Macromol., 2018, 109, 254–262 CrossRef CAS PubMed.
- C. P. Santana, A. A. Mansur, S. M. Carvalho, A. da Silva-Cunha Jr and H. S. Mansur, Mater. Lett., 2019, 252, 333–337 CrossRef CAS.
- Estimated sales of biosensors worldwide in 2017 and 2027 (in million U.S. dollars), https://www.statista.com/statistics/935683/estimated-global-sales-biosensors/.
- J. Kim, A. S. Campbell, B. E.-F. de Ávila and J. Wang, Nat. Biotechnol., 2019, 37, 389–406 CrossRef CAS PubMed.
- Y. Lin, M. Bariya and A. Javey, Adv. Funct. Mater., 2021, 31, 2008087 CrossRef CAS.
-
N. Shah, W. A. Khan, T. Rehan, D. Lin, H. Tetik and S. Haider, Renewable Polymers and Polymer-Metal Oxide Composites, Elsevier, 2022, pp. 371–394 Search PubMed.
- Y. Jiang and J. Wu, Electrophoresis, 2019, 40, 2084–2097 CrossRef CAS PubMed.
- S. Hroncekova, T. Bertok, M. Hires, E. Jane, L. Lorencova, A. Vikartovska, A. Tanvir, P. Kasak and J. Tkac, Processes, 2020, 8, 580 CrossRef CAS PubMed.
- E. Muthusankar and D. Ragupathy, Sens. Lett., 2018, 16, 81–91 CrossRef.
- A. Karrat and A. Amine, Arab. J. Chem. Environ. Res, 2020, 7, 66–93 CAS.
- S. Takeshita, S. Zhao, W. J. Malfait and M. M. Koebel, Angew. Chem., Int. Ed., 2021, 60, 9828–9851 CrossRef CAS PubMed.
-
P. Venkatachalam and S. Karuppiah, Chitosan in Biomedical Applications, Elsevier, 2022, pp. 229–244 Search PubMed.
- X. Zhang, C.-R. Li, W.-C. Wang, J. Xue, Y.-L. Huang, X.-X. Yang, B. Tan, X.-P. Zhou, C. Shao and S.-J. Ding, Food Chem., 2016, 192, 197–202 CrossRef CAS PubMed.
- A. Güner, E. Çevik, M. Şenel and L. Alpsoy, Food Chem., 2017, 229, 358–365 CrossRef PubMed.
- Q. Rong, F. Feng and Z. Ma, Biosens. Bioelectron., 2016, 75, 148–154 CrossRef CAS PubMed.
- S. Xu, J. Zhan, B. Man, S. Jiang, W. Yue, S. Gao, C. Guo, H. Liu, Z. Li and J. Wang, Nat. Commun., 2017, 8, 1–10 CrossRef PubMed.
- Y. Yao, T. Zhang and M. Tang, Environ. Pollut., 2022, 119270 CrossRef CAS PubMed.
- Y. Liu, S. Zhu, Z. Gu, C. Chen and Y. Zhao, Particuology, 2022, 69, 31–48 CrossRef CAS.
- Z. Zhao, L. Xu, Y. Wang, B. Li, W. Zhang and X. Li, Environ. Sci. Pollut. Res., 2021, 28, 15032–15042 CrossRef CAS PubMed.
- Z. Wang and M. Tang, J. Appl. Toxicol., 2021, 41, 683–700 CrossRef CAS PubMed.
- S. Bekeschus, Nanomaterials, 2021, 11, 806 CrossRef CAS PubMed.
- S. Singh, Toxicol. Mech. Methods, 2019, 29, 300–311 CrossRef CAS PubMed.
- M. C. Crisan, M. Teodora and M. Lucian, Appl. Sci., 2021, 12, 141 CrossRef.
- C. Tao, Lett. Appl. Microbiol., 2018, 67, 537–543 CrossRef CAS PubMed.
- A. S. A. Mohammed, M. Naveed and N. Jost, J. Polym. Environ., 2021, 29, 2359–2371 CrossRef CAS PubMed.
- N. M. Saidin, N. K. Anuar and M. M. M. Affandi, J. Appl. Pharm. Sci., 2018, 8, 141–157 CrossRef CAS.
- J. E. van Dam, L. A. van den Broek and C. G. Boeriu, Nat. Prod. Commun., 2017, 12, 1934578X1701200604 CrossRef.
- Z. Shokri, F. Seidi, S. Karami, C. Li, M. R. Saeb and H. Xiao, Carbohydr. Polym., 2021, 262, 117963 CrossRef CAS PubMed.
- J. Zdarta, A. S. Meyer, T. Jesionowski and M. Pinelo, Biotechnol. Adv., 2019, 37, 107401 CrossRef CAS PubMed.
- M. Deska and B. Kończak, Process Biochem., 2019, 84, 112–123 CrossRef CAS.
|
This journal is © The Royal Society of Chemistry 2022 |