DOI:
10.1039/D4SC03561E
(Edge Article)
Chem. Sci., 2024, Advance Article
Electronic energy transfer ionization in naphthalene–CO2 clusters reveals excited states of dry ice†
Received
30th May 2024
, Accepted 17th July 2024
First published on 31st July 2024
Abstract
Electronic energy relaxation and transfer shapes the photochemistry in molecules and materials that are exposed to UV radiation in areas ranging from astrochemistry to biology. The interaction between CO2 and polycyclic aromatic hydrocarbons (PAHs) specifically, is of paramount interest in astrochemically relevant ices, the transition to renewable energy and the development of green chemistry. We investigate the vacuum UV excitation of the naphthalene–CO2 complex and observe excited states of CO2 through a newly identified molecular electronic energy transfer ionization mechanism. We evaluate the spectral development upon cluster growth with time-dependent density functional theory and show that the photoionization spectrum of naphthalene–CO2 closely resembles the photon-stimulated desorption spectrum of CO2 ice. The molecular electronic energy transfer ionization mechanism may affect the energy redistribution and charge balance in the interstellar medium significantly and therefore we discuss its implications for astrochemical models.
Introduction
Electronic energy relaxation plays a crucial role in determining the dynamics and outcome of the photophysics and photochemistry in molecules and materials. It is a key process to understand in systems ranging from astrochemical grains to biomolecules that are exposed to (solar) UV radiation.1 Electronic energy transfer is central to Förster resonance energy transfer (FRET), Penning ionization (ionization following collision of an excited molecule) and excitation transfer ionization (ETI).2–4 Here, we report on the observation of ETI in a bimolecular cluster which would aptly be called molecular electronic energy transfer ionization (MEETI). The two molecules involved are carbon dioxide (CO2) and naphthalene. CO2 interacts with organic molecules such as naphthalene in a wide range of naturally occurring astrochemical objects as well as in terrestrial applications.5–8 Insights into the interactions between CO2 and organic molecules will not only benefit our understanding of the condensation of matter in space, but also aid in further developing the use of (supercritical) CO2 in green chemistry and various renewable energy solutions.9,10 Furthermore, graphene based photocatalysts are receiving increasing attention for the activation and chemical transformation of CO2.11
In astrophysics, it is well established that ices of small volatiles such as CO2, in which larger molecules are embedded, serve to bring reactants together and thereby allow for more complex molecules to be formed. Vacuum ultraviolet (VUV) photons, between ∼6.0 and 13.5 eV, are the drivers behind these processes, especially in photodissociation regions, but also in more shielded objects where secondary photons originate from penetrating cosmic rays.1,12 These VUV photons initiate chemical reactions by creating reactants in the form of radicals or ions, that can subsequently react without a barrier towards increasing complexity.13 If ices are subject to sufficient VUV radiation, CO2 even becomes the primary carbon carrier.12,14 The two main events following VUV irradiation of interstellar nebula are the photoelectric effect on PAHs and small grains and the pumping and collisional de-excitation of molecular hydrogen.15 It has been recognized that, especially when polycyclic aromatic hydrocarbons (PAHs) are entrained within ice, energy transfer is of fundamental importance in determining which chemistry occurs within the grain and that PAH concentration in ices influences the response to radiation.12,16
The interaction of VUV light with CO2 has been studied using various experimental techniques focusing on the monomer, through clusters and finally the (supercritical) condensed phase.6,14,17–19 A recent study using VUV absorption spectroscopy of gas phase CO2 at high (supercritical) densities, revealed that the close proximity between CO2 entities affects the vibronic spectrum.6 Upon condensation, the VUV spectrum of CO2 loses the vibrational structure observed in the gas-phase, but maintains its general appearance with the first two bands blue shifted by 0.3 and 0.6 eV to 8.8 and 9.9 eV. Finally, wavelength resolved (V)UV photodesorption of CO2 ices reveals a similar spectrum and thereby points to an efficient indirect desorption mechanism (DIET – Desorption induced by electronic transition) that involves energy transfer from CO2.20
At the basis of an indirect desorption mechanism such as DIET, or more in general, for (V)UV electronic energy dissipation in ices, is the propensity either to transfer energy, to molecular fragmentation or to down-convert radiation which greatly affects the physical conditions in interstellar clouds. For example, studies have shown that a PAH can be ionized in pure CO2 ice,2,21–23 creating free electrons that can induce chemical reactions21 or CO2 can be efficiently dissociated, creating radical species.24 Both mechanisms are contrary to the energy dissipation mechanism by solely releasing heat. We demonstrate that indeed the presence of a PAH can change the fate of electronic excitation of CO2 in a bimolecular cluster through MEETI and discuss the implications for astrochemistry. The study also reveals how electronic spectral properties of CO2 change upon condensation, thereby connecting the gas and condensed phase.25
Results & discussion
Photoionization efficiency of naphthalene CO2 clusters
The photoionization efficiency curves (PIE) of mass-selected naphthalene and the naphthalene–CO2 complex produced in a continuous molecular beam are shown in Fig. 1. Detailed methods are described in the ESI.† The PIE of naphthalene compares well with previously recorded ones of naphthalene25 and, as expected, the ionization efficiency increases in a relatively featureless manner with higher photon energy. The slight dip in both spectra at around 11.8 eV is due to the strong absorption by argon used in the gas filter to remove residual higher harmonic radiation emanating from the synchrotron based undulator.26 In contrast, the PIE of the naphthalene–CO2 complex has significantly more structure compared to naphthalene. Three large peaks at 11.1, 12.0 and 13.35 eV are clearly discernible and smaller ones emerge at 8.5, 9.2 and 10.0 eV. The difference between the PIE of the naphthalene–CO2 complex and the naphthalene monomer, visible as the red shaded area, is likely the result of absorption of CO2 and hence compares well to the black dotted line that corresponds to the calculated spectrum of CO2. These new features and the increase in intensity with respect to the naphthalene PIE, visible in Fig. 1, are below the ionization energy (IE) of the CO2 monomer (13.8 eV).27 This suggests that the photo-excited CO2 monomer (and possibly clusters) transfers electronic energy to the naphthalene moiety, with a lower IE of 8.1 eV (ref. 25) which subsequently ionizes and hence leads to an ion that can be detected in our mass spectrometer. The absorption spectrum of CO2 is thus imprinted on the naphthalene–CO2 PIE as a result of the electronic energy transfer after absorption of CO2. This likely arises since the vibronic states of naphthalene, nicely overlap with the excitation spectrum of CO2.28 In Fig. 1, we show a calculated VUV photoabsorption spectra (vide infra) of the CO2 monomer and allow for comparison to the derivative of the naphthalene–CO2 PIE to illustrate this point. From our results, it is not possible to estimate the efficiency of energy transfer-ionization, as only the naphthalene–CO2 ions that have undergone this process are actually detected.
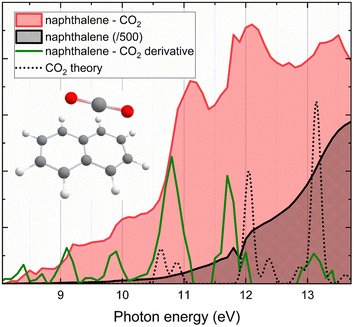 |
| Fig. 1 PIE curves of naphthalene and naphthalene–CO2 cluster showing the enhancement in ionization efficiency below the IE of CO2. Inset shows the structure of naphthalene–CO2 optimized using DFT in this work. The black dotted line corresponds to the calculated absorption spectrum of pure CO2 using TDDFT at the LC-BLYP/daug-cc-pVTZ level. The green solid line shows the derivative of the naphthalene–CO2 PIE, revealing peaks that correspond to the CO2 excitations. | |
Comparison of experimental and calculated spectra for excited state CO2 clusters
Since the co-expansion of naphthalene with CO2 produces multiple sized naphthalene–(CO2)p (p = 1–6) complexes which are mass selected, the VUV absorption spectra of a series of (CO2)n (n = 1–6) is indirectly obtained. Fig. 2 displays a zoom-in of the naphthalene–(CO2)p (p = 1–6) PIEs in the region where significant CO2 absorption is expected. The spectra are normalized to the second peak at 12.1 eV to facilitate comparison. It can be noted that the first peak around 11.1 eV increases in relative intensity, whereas the third peak around 13.4 eV decreases in intensity and is no longer distinguishable for clusters larger than the trimer. The absorption energy of the first and second peak is relatively constant, whereas for the third peak, the central photon energy is difficult to determine. In order to assign the observed features and trends in these indirectly obtained (CO2)n (n = 1–4) cluster absorption features we turn to time dependent density functional theory (TDDFT) calculations. Typically, TDDFT is only suitable below the ionization energy, or more conservatively, below the first Rydberg state. However, previous studies on CO2 excited states in this region have shown that the TDDFT LC-BLYP method performs well compared to equation-of-motion coupled-cluster with single and double excitations (EOM-CCSD) for the excited states of CO2 in the region between 10.0–13.7 eV.9 Moreover, TDDFT allows us to relatively effectively calculate the excited state spectra of different geometries of higher order clusters. The spectra of the dimer, trimer and tetramer are an average of spectra for different geometries likely to be present in our experiment and are displayed in Fig. 2 as shaded areas.29–36 Individual spectra and their geometries are presented in ESI Fig. 1 and 2,† respectively. For comparison, in this case, the theoretically calculated spectra are redshifted by 0.6 eV (a shift that is consistent with previous benchmarking studies37) and normalized by the peak around 12.1 eV. The three features observed in the experiment are reproduced by theory. Whereas experimentally, the first peak around 11.1 eV does not show a significant shift for larger clusters, theory predicts a small blueshift and reveals this first peak could consist of a doublet. In the experiment, the relative intensity increases with larger clusters, which is reproduced theoretically with an average 25% increase in integrated intensity per CO2 molecule added. The second peak does not show a shift in frequency, in line with experiment. Most notable is the change in the third peak with increasing cluster size, which spectrally diffuses and blue-shifts. This spectral diffusion is consistent with our experimental observation, namely the disappearance of the third peak for higher order clusters.
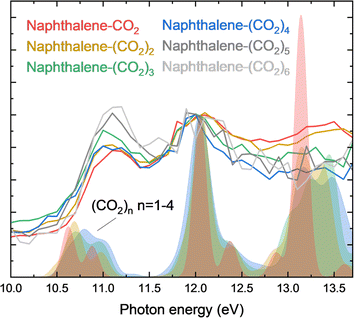 |
| Fig. 2 Lines represent the PIE of naphthalene–(CO2)p (p = 1–6) clusters showing the three main peaks associated with the excited states of CO2. The shaded curves are calculated absorption spectra of pure CO2 clusters (n = 1–4) using TDDFT on the LC-BLYP/daug-cc-pVTZ level. Corresponding structures are displayed in ESI Fig. 2† and a full scale plot in ESI Fig. 3.† Theory is redshifted by 0.6 eV, matching the experimental band at 12 eV. Theory and experiment are normalized at 12 eV. | |
Fragmentation of CO2 clusters decoded via mass spectrometry
The indirectly obtained CO2 experimental spectra through MEETI could be affected by fragmentation, distorting the size selectivity in our experiment, since the binding energy of CO2 is small (on the order of 0.6 eV (ref. 8)) relative to the photon energy. Clusters can lose one or more CO2 moieties after photon absorption, leading to a smaller than the neutral ‘absorbing’ cluster in the mass spectrometer. There are conflicting reports19,38 in the literature on the extent of fragmentation in this spectral region for CO2 (clusters). Hence, we performed an analysis based upon dissociative photoionization, to tease out the extent of this fragmentation and, thus, the best estimate of the size of the original neutral cluster.
For that purpose, we determine the abundance of the pure CO2 clusters detected in our mass spectrometer, relative to the abundance of the CO2 dimer. The dimer is chosen as a reference because from the dimer onwards, the PIEs only change marginally.19 Fig. 3 shows the ion count of pure CO2 clusters relative to that of the dimer as function of photon energy (see ESI Fig. 4† for a full-scale plot). At higher photon energies (>∼13.5 eV), fewer larger clusters are detected compared to lower photon energies (<13 eV). The size distribution at the ionization energy should best reflect the distribution of neutral clusters in our beam. As can be seen from the figure, this holds true in our experiment, where a peak in clusters larger than (CO2)2 is visible at 13.2 eV, the previously determined IE of (CO2)2. The peak reflects the lowest probability of fragmentation and hence we use the distribution here to estimate the neutral CO2 cluster sizes. The CO2 trimer abundance is distorted by the naphthalene monomer ion signal, which is close in mass and affects the experimental baseline and should therefore be ignored in this analysis. The absorption of photons by the naphthalene moiety at low photon energy can result in fragmentation in these heterogeneous clusters, hence, we have to focus on the pure CO2 clusters. However, the pure CO2 cluster size distribution should reflect the size of clusters that condenses on naphthalene in the cluster beam.
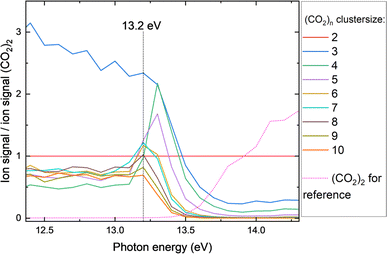 |
| Fig. 3 PIE of pure CO2 clusters divided by (CO2)2 as function of photon energy showing the relative abundance of higher order clusters. As a reference, the (CO2)2 cluster PIE curve is plotted (pink, dotted line), showing that the cluster size peaks at around the appearance energy. (Note that the AE of (CO2)n n > 1 are very similar). | |
Fig. 4 shows the mass spectra at 13.2 eV, determined in the previous section as the photon energy where the ions best reflect the neutral size distribution of CO2 clusters in our experiment. We find that a Lorentzian with a mean at 5.6 CO2 units provides a good description of the CO2 clusters distribution, not including the monomer. The mass bias of our spectrometer should be of minimal influence in the mass range relevant to this experiment. The heterogeneous clusters with naphthalene show a different distribution at 13.2 eV, with nap–(CO2)1 being the most prominent. Assuming that the original nap–(CO2)p clusters have a similar CO2 size distribution as the pure CO2 clusters, some CO2 units would have desorbed at 13.2 eV, most likely because the heterogeneous complexes have a lower IE (more analogues to the IE of naphthalene) than the pure CO2 clusters and excess energy leads to desorption. The desorption energy of CO2 on graphene was determined to be 0.26–0.31 eV, which is on the order of the amount of excess energy supplied.11 Examining the CO2 cluster size distribution at slightly higher photon energy (13.4 eV, see ESI Fig. 5†), reveals that the mean has shifted to smaller sizes of 3.3 CO2 units on average. It appears that the excess energy readily leads to dissociation of the cluster (the CO2 dimer having a binding energy of about 0.06 eV (ref. 8)).
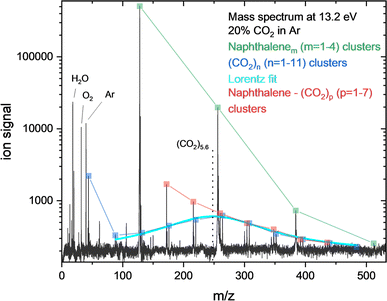 |
| Fig. 4 Mass spectra at 13.2 eV photon energy (black) where least fragmentation of pure CO2 clusters is expected (see Fig. 3). Indicated are the pure CO2 clusters (dark blue), the naphthalene clusters (green) and the naphthalene–CO2 clusters (red). A Lorentz fit of the pure CO2 cluster size distribution (light blue), not including the monomer, indicates a mean cluster size of 5.6 CO2 units. | |
Comparison of excited state CO2 clusters with solid state (ice) CO2 spectra
These results allow us to discuss another remarkable observation in our work, that an isolated naphthalene–(CO2) spectrum in the gas phase is very similar to spectra observed in condensed CO2 ices. The gas-phase VUV photo absorption spectrum of CO2, changes dramatically upon complexation with naphthalene becoming diffuse and all the rich vibronic structure disappears completely. Instead, between about 10.5 and 13.6 eV, three peaks are distinguishable that are similar to features observed at the same photon energies in the photon-stimulated desorption (PSD) spectrum of 13CO2 ice maintained at 10 K. A smaller feature in the PSD spectrum at 9.9 eV may be associated with the step at 10 eV in the naphthalene–CO2 spectrum. The resemblance of the gas-phase and PSD spectra may originate from a similarity in energy transfer process that in the first case leads to dissociative ionization and in the latter to desorption. The electronic energy transfer in astrophysical ices, DIET – desorption induced by electronic transition, is thought to be dominant in desorption of light volatiles in interstellar ices between 7 and 12 eV.20 Our study suggests that the presence of a PAH and MEETI energy transfer to it, can also be important for the energy dissipation in ices, in this case by emitting an electron. A lower resemblance to direct absorption spectra of solid phase CO2 (Fig. 5) supports the hypothesis that energy transfer has a large effect on the appearance of the naphthalene–CO2 spectrum. The largest peak in the solid phase direct absorption spectrum at 10.7 eV is not observed in the naphthalene–CO2 spectrum; and the intensity significantly drops at 11.8 eV in the solid phase whereas the naphthalene–CO2 contains a peak at 11.9 eV. The molecular electronic energy transfer ionization (MEETI) appears to affect both the PSD as well as the naphthalene–CO2 spectrum in a similar manner. This is also consistent with our hypothesis, because both photodesorbed and photoionized species detected in the two experiments are (at least partially) the result of electronic energy transfer.
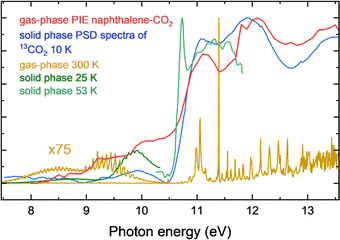 |
| Fig. 5 Comparison of the PIE curve of naphthalene–CO2 with solid phase photon-stimulated desorption (PSD) spectrum of 13CO2,20 CO2 gas-phase spectrum39 and CO2 solid phase absorption spectra.40–42 | |
The spectral region investigated here overlaps well with the radiation that is thought to drive chemical conversion (between 6 and 13.5 eV) in photodissociation regions in the interstellar medium and radiative transfer is largely regulated by smaller dust grains, making our study of particular interest to astrochemistry.15 The process we identified, namely the photoionization of a PAH following electronic energy transfer from CO2 may be a yet unconsidered route for energy relaxation in ices. Although the efficiency of this process is yet to be determined and in general, photoionization is a minor pathway following (V)UV absorption, the free electrons and ions generated may facilitate barrier-less reactions towards increasing complexity, much more than heat generated from collisional de-excitation.15,43–45 Electronic energy transfer has been considered to some extent, however, mostly from a condensed phase point of view as the photoelectric effect rather than the molecular point of view that we provide here.15 In a generic way, photoionization of small grains and PAHs molecules has been included in astrochemical models for heating and charging of interstellar gas. There, it was recognized that some photon energies may not directly lead to ionization, and that PAHs strongly affect photoionization yields.15 It is important to extend such models and include chemical information of specific ices. As we show, resonances and ionization energies, which are highly specific chemical properties, can play a role in MEETI and relaxation. This has been recognized before in the literature albeit for different processes, by the observation that photodesorption mechanisms in the condensed phase are highly molecule specific, and also in heterogeneous systems in terms of co-desorption.14
Conclusion
In this work we observe molecular electronic energy transfer ionization (MEETI) in weakly bound naphthalene–CO2 complexes by molecular beam vacuum-UV photoionization mass spectrometry and follow the development of excited states upon CO2 cluster growth. We find that the spectrum of a small number of CO2 molecules condensed on naphthalene already closely resembles the photon-stimulated desorption spectrum of CO2 ice and speculate on the origin of the observed spectral features. Finally, we discuss the astrochemical implications, such as the creation of free electrons, that sets in motion reactions towards more complex chemistry and influence the charge distribution in the interstellar medium.
Data availability
The data that support the findings of this study are available within the article and its ESI.† The raw mass spectral data is available from the corresponding author upon reasonable request.
Author contributions
Alexander Lemmens: conceptualization (equal); data curation (equal); formal analysis (lead); visualization (lead); writing – original draft (lead); writing – review & editing (lead). Nureshan Dias: data curation (equal); writing – review & editing (equal). Anna Wannenmacher: data curation (equal); writing – review & editing (equal). Musahid Ahmed: conceptualization (equal); funding acquisition (lead); supervision (lead); writing – review & editing (equal).
Conflicts of interest
There are no conflicts to declare.
Acknowledgements
This work is supported by the Gas Phase Chemical Physics Program, in the Chemical Sciences Geosciences and Biosciences Division of the Office of Basic Energy Sciences of the U.S. Department of Energy under Contract No. DE-AC02-05CH11231 and the U.S. Department of Energy, Office of Science, Office of Workforce Development for Teachers and Scientists, Office of Science Graduate Student Research (SCGSR) program (Contract No. DE-SC0014664). This research used resources from the Advanced Light Source, which is a DOE Office of Science User Facility under Contract No. DE-AC02-05CH11231.
References
- O. Berné, E. Habart, E. Peeters, I. Schroetter, A. Canin, A. Sidhu, D. van de Putte, F. Alarcón, M. Zannese, A. Abergel and E. A. Bergin, A Far-Ultraviolet–Driven Photoevaporation Flow Observed in a Protoplanetary Disk, Science, 2024, 383, 988–992, DOI:10.1126/science.adh2861.
- A. Golan and M. Ahmed, Ionization of Water Clusters Mediated by Exciton Energy Transfer from Argon Clusters, J. Phys. Chem. Lett., 2012, 3, 458–462 CrossRef CAS PubMed.
- W. Lu, R. B. Metz, T. P. Troy, O. Kostko and M. Ahmed, Exciton Energy Transfer Reveals Spectral Signatures of Excited States in Clusters, Phys. Chem. Chem. Phys., 2020, 22(25), 14284–14292, 10.1039/d0cp02042g.
- K. Gokhberg, A. B. Trofimov, T. Sommerfeld and L. S. Cederbaum, Ionization of Metal Atoms Following Valence-Excitation of Neighbouring Molecules, Europhys. Lett., 2005, 72(2), 228–234, DOI:10.1209/epl/i2005-10227-7.
- J. H. Bredehöft, CO2 : A Small Ubiquitous Molecule With a Lot of Astrochemical Debate Attached, Front. Astron. Space Sci., 2020, 7(33), 1–5, DOI:10.3389/fspas.2020.00033.
- T. W. Marin and I. Janik, Ultraviolet Spectroscopy of Pressurized and Supercritical Carbon Dioxide, Commun. Chem., 2021, 4(77), 1–9, DOI:10.1038/s42004-021-00516-z.
- J. Wang, W. Ding, B. Zhang and H. Jin, Polycyclic Aromatic Hydrocarbons Dissolution in Supercritical Carbon Dioxide by Molecular Dynamics Simulation, J. Mol. Liq., 2023, 391, 123358, DOI:10.1016/j.molliq.2023.123358.
- Y. N. Kalugina, I. A. Buryak, Y. Ajili, A. A. Vigasin, N. E. Jaidane and M. Hochlaf, Explicit Correlation Treatment of the Potential Energy Surface of CO2 Dimer, J. Chem. Phys., 2014, 140, 234310, DOI:10.1063/1.4882900.
- B. J. Costa Cabral, R. Rivelino, K. Coutinho and S. Canuto, A First Principles Approach to the Electronic Properties of Liquid and Supercritical CO2, J. Chem. Phys., 2015, 142(024504), 1–9, DOI:10.1063/1.4905256.
- P. Tafur-Escanta, R. Valencia-Chapi, M. López-Guillem, O. Fierros-Peraza and J. Muñoz-Antón, Electrical Energy Storage Using a Supercritical CO2 Heat Pump, Energy Rep., 2022, 8, 502–507, DOI:10.1016/j.egyr.2022.01.073.
- K. Takeuchi, S. Yamamoto, Y. Hamamoto, Y. Shiozawa, K. Tashima, H. Fukidome, T. Koitaya, K. Mukai, S. Yoshimoto, M. Suemitsu, Y. Morikawa, J. Yoshinobu and I. Matsuda, Adsorption of CO2 on Graphene: A Combined TPD, XPS, and VdW-DF Study, J. Phys. Chem. C, 2017, 121(5), 2807–2814, DOI:10.1021/acs.jpcc.6b11373.
- K. I. Öberg, Photochemistry and Astrochemistry: Photochemical Pathways to Interstellar Complex Organic Molecules, Chem. Rev., 2016, 116(17), 9631–9663, DOI:10.1021/acs.chemrev.5b00694.
- R. I. Kaiser and N. Hansen, An Aromatic Universe − A Physical Chemistry Perspective, J. Phys. Chem. A, 2021, 125(18), 3826–3840, DOI:10.1021/acs.jpca.1c00606.
- K. I. Öberg, E. F. Van Dishoeck and H. Linnartz, Photodesorption of Ices I: CO, N2, and CO2, Astron. Astrophys., 2009, 496(1), 281–293, DOI:10.1051/0004-6361/200810207.
- D. J. Hollenbach and A. G. G. M. Tielens, Photodissociation Regions in the Interstellar Medium of Galaxies, Rev. Mod. Phys., 1999, 71(1), 173–230 CrossRef CAS.
- S. H. Cuylle, E. D. Tenenbaum, J. Bouwman, H. Linnartz and L. J. Allamandola, Ly-Alfa-Induced Charge Effects of Polycyclic Aromatic Hydrocarbons Embedded in Ammonia and Ammonia: Water Ice, Mon. Not. R. Astron. Soc., 2012, 423(2), 1825–1830, DOI:10.1111/j.1365-2966.2012.21006.x.
- R. J. Buenker, M. Honigmann, H. P. Liebermann and M. Kimura, Theoretical Study of the Electronic Structure of Carbon Dioxide: Bending Potential Curves and Generalized Oscillator Strengths, J. Chem. Phys., 2000, 113(3), 1046–1054, DOI:10.1063/1.481884.
- K. S. Kalogerakis, C. Romanescu, M. Ahmed, K. R. Wilson and T. G. Slanger, CO Prompt Emission as a CO2 Marker in Comets and Planetary Atmospheres, Icarus, 2012, 220(1), 205–210, DOI:10.1016/j.icarus.2012.04.028.
- S. H. Linn and C. Y. Ng, Photoionization Study of CO2, N2O Dimers and Clusters, J. Chem. Phys., 1981, 75, 4921–4926 CrossRef CAS.
- J. H. Fillion, E. C. Fayolle, X. Michaut, M. Doronin, L. Philippe, J. Rakovsky, C. Romanzin, N. Champion, K. I. Öberg, H. Linnartz and M. Bertin, Wavelength Resolved UV Photodesorption and Photochemistry of CO2 Ice, Faraday Discuss., 2014, 168, 533–552, 10.1039/c3fd00129f.
- S. Radhakrishnan, M. S. Gudipati, W. Sander and A. Lignell, Photochemical Processes in CO2/H2O Ice Mixtures with Trapped Pyrene, a Model Polycyclic Aromatic Hydrocarbon, Astrophys. J., 2018, 864(151), 9pp, DOI:10.3847/1538-4357/aad4a6.
- H. Kimura, On the Photoelectric Quantum Yield of Small Dust Particles, Mon. Not. R. Astron. Soc., 2016, 459(3), 2751–2761, DOI:10.1093/mnras/stw820.
- S. Misra, S. K. Mishra and M. S. Sodha, Charging of Ice Grains in Saturn's E Ring: Theory and Observations, Mon. Not. R. Astron. Soc., 2012, 423(1), 176–184, DOI:10.1111/j.1365-2966.2012.20774.x.
- Z. Lu, Y. C. Chang, Y. Benitez, Z. Luo, A. B. Houria, T. Ayari, M. M. Al Mogren, M. Hochlaf, W. M. Jackson and C. Y. Ng, State-to-State Vacuum Ultraviolet Photodissociation Study of CO2 on the Formation of State-Correlated CO(X1Σ+; v) with O(1D) and O(1S) Photoproducts at 11.95–12.22 eV, Phys. Chem. Chem. Phys., 2015, 17(17), 11752–11762, 10.1039/c5cp01321f.
- B. Xu, T. Stein, U. Ablikim, L. Jiang, J. Hendrix, M. Head-Gordon and M. Ahmed, Probing Solvation and Reactivity in Ionized Polycyclic Aromatic Hydrocarbon-Water Clusters with Photoionization Mass Spectrometry and Electronic Structure Calculations, Faraday Discuss., 2019, 217, 414–433, 10.1039/c8fd00229k.
- A. G. Suits, P. Heimann, X. Yang, M. Evans, C. W. Hsu, K. T. Lu, Y. T. Lee and A. H. Kung, A Differentially Pumped Harmonic Filter on the Chemical Dynamics Beamline at the Advanced Light Source, Rev. Sci. Instrum., 1995, 66(10), 4841–4844, DOI:10.1063/1.1146161.
- L. S. Wang, J. E. Reutt, Y. T. Lee and D. A. Shirley, High Resolution UV Photoelectron Spectroscopy of CO2+, COS+ and CS2+ Using Supersonic Molecular Beams, J. Electron Spectrosc. Relat. Phenom., 1988, 47, 167–186, DOI:10.1016/0368-2048(88)85010-2.
- E. Rühl, S. D. Price and S. Leach, Single and Double Photoionization Processes in Naphthalene between 8 and 35 EV, J. Phys. Chem., 1989, 93(17), 6312–6321, DOI:10.1021/j100354a011.
- T. E. Gough, R. E. Miller and G. Scoles, Infrared Spectra and Vibrational Predissociation of (CO2)n Clusters Using Laser-Molecular Beam Techniques, J. Phys. Chem., 1981, 85(26), 4041–4046, DOI:10.1021/j150626a018.
- M. Dehghany, A. R. W. McKellar, M. Afshari and N. Moazzen-Ahmadi, High-Resolution Infrared Spectroscopy of Carbon Dioxide Dimers, Trimers, and Larger Clusters, Mol. Phys., 2010, 108(17), 2195–2205, DOI:10.1080/00268976.2010.496742.
- M. Lippe, U. Szczepaniak, G. L. Hou, S. Chakrabarty, J. J. Ferreiro, E. Chasovskikh and R. Signorell, Infrared Spectroscopy and Mass Spectrometry of CO2 Clusters during Nucleation and Growth, J. Phys. Chem. A, 2019, 123(12), 2426–2437, DOI:10.1021/acs.jpca.9b01030.
- Y. Inokuchi, A. Muraoka, T. Nagata and T. Ebata, An IR Study of CO2+n (N = 3–8) Cluster Ions in the 1000–3800 cm−1 Region, J. Chem. Phys., 2008, 129, 044308, DOI:10.1063/1.2953710.
- H. Takeuchi, Geometry Optimization of Carbon Dioxide Clusters (CO2)n for 4 ≤ n ≤ 40, J. Phys. Chem. A, 2008, 112(33), 7492–7497, DOI:10.1021/jp802872p.
- J. Andreas, M. L. Mckee and H. B. Schlegel, Ab Initio Study of the CO2 Dimer and the CO2 Ion Complexes (CO2)2+ and (CO2)3+, J. Phys. Chem., 1987, 91, 3489–3494 CrossRef.
- G. T. Fraser, A. S. Pine, W. J. Lafferty and R. E. Miller, Sub-Doppler Infrared Spectrum of the Carbon Dioxide Trimer, J. Chem. Phys., 1987, 87(3), 1502–1506, DOI:10.1063/1.453260.
- M. J. Weida and D. J. Nesbitt, Geometric Isomerism in Clusters: High Resolution Infrared Spectroscopy of a Noncyclic CO2 Trimer, J. Chem. Phys., 1996, 105(23), 10210–10223, DOI:10.1063/1.472930.
- A. K. Narsaria, J. D. Ruijter, T. A. Hamlin, A. W. Ehlers, C. F. Guerra, K. Lammertsma and F. M. Bickelhaupt, Performance of TDDFT Vertical Excitation Energies of Core-Substituted Naphthalene Diimides, J. Comput. Chem., 2020, 41(15), 1448–1455, DOI:10.1002/jcc.26188.
- G. G. Jones and J. W. Taylor, A Photoionization Study of Carbon Dioxide Dimers in a Supersonic Molecular Beam, J. Chem. Phys., 1978, 68(4), 1768–1775, DOI:10.1063/1.435947.
- D. L. Huestis and J. Berkowitz, Critical Evaluation of the Photoabsorption Cross Section of CO2 From 0.125 to 201.6 nm at Room Temperature, Adv. Geosci., 2010, 25, 229–242 Search PubMed.
- K. M. Monahan and W. C. Walker, Vacuum Ultraviolet Absorption Spectra of Solid N2O and CO2 at 53 K, J. Chem. Phys., 1975, 63, 1676–1681, DOI:10.1063/1.432493.
- K. M. Monahan and W. C. Walker, Photoabsorption of Solid Carbon Dioxide from 7 to 12 eV, J. Chem. Phys., 1974, 61(10), 3886–3889, DOI:10.1063/1.1681680.
- N. J. Mason, A. Dawes, P. D. Holtom, R. J. Mukerji, M. P. Davis, B. Sivaraman, R. I. Kaiser, S. V. Hoffmann and D. A. Shaw, VUV Spectroscopy and Photo-Processing of Astrochemical Ices: An Experimental Study, Faraday Discuss., 2006, 133, 311–329, 10.1039/b518088k.
- W. D. Watson, Photoelectron Emission from Interstellar Grains, Interstellar Dust and Related Topics. IAU Symposium no. 52, 1973, pp. 335–339 Search PubMed.
- G. B. Esplugues, S. Cazaux, R. Meijerink, M. Spaans and P. Caselli, Surface Chemistry in Photodissociation Regions, Astron. Astrophys., 2016, 591, A52, DOI:10.1051/0004-6361/201528001.
- N. J. Mason, B. Nair, S. Jheeta and E. Szymańska, Electron Induced Chemistry: A New Frontier in Astrochemistry, Faraday Discuss., 2014, 168, 235–247, 10.1039/c4fd00004h.
Footnote |
† Electronic supplementary information (ESI) available: The supplemental material contains details of the experimental and theoretical methods, separate calculated excitation spectra and corresponding conformers, a full-scale plot with the photoionization efficiencies (PIE) of naphthalene–(CO2)p (p = 1–6) clusters, a full scale plot the PIEs of pure CO2 clusters divided by that of the (CO2)2 and a mass spectra at 13.4 eV photon energy. See DOI: https://doi.org/10.1039/d4sc03561e |
|
This journal is © The Royal Society of Chemistry 2024 |