DOI:
10.1039/C9SM01985E
(Paper)
Soft Matter, 2020,
16, 162-169
Fabrication of novel MXene (Ti3C2)/polyacrylamide nanocomposite hydrogels with enhanced mechanical and drug release properties†
Received
3rd October 2019
, Accepted 10th November 2019
First published on 13th November 2019
Abstract
A highly stretchable nanocomposite (NC) hydrogel was fabricated via in situ free radical polymerization of acrylamide. In particular, an exfoliated two-dimensional MXene (Ti3C2) nanosheet was utilized as a crosslinker instead of traditional organic crosslinkers. The exfoliated Ti3C2 nanosheets were confirmed by atomic force microscopy (AFM) and dynamic light scattering (DLS) measurements. Compared with traditional organic crosslinked N,N-methylene bisacrylamide (BIS)/polyacrylamide (PAM) hydrogels (fracture strength of 32.0 kPa and elongation of 109.6%), the synthesized Ti3C2/PAM NC hydrogels exhibited greatly improved mechanical properties with fracture strengths of 66.5 to 102.7 kPa, compressive strengths of 400.6 to 819.4 kPa and elongations at break of 2158.6% to 3047.5% as the Ti3C2 content increases from 0.0145% to 0.0436%. The enhanced mechanical performances can be attributed to the honeycomb-like fine structure with uniform pores as well as more flexible polymer chains in NC hydrogel networks. When loaded with drugs, Ti3C2/PAM NC hydrogels exhibited good sustained-release performance, higher drug loading amounts (97.5–127.7 mg g−1) and higher percentage releases (62.1–81.4%), greatly superior to those of the BIS/PAM hydrogel (46.4 mg g−1, 45.0%). Our work reveals the application of MXene materials in the fabrication of NC hydrogels with enhanced mechanical and drug release behaviors.
1. Introduction
Polymer hydrogels are a class of three-dimensional crosslinked hydrophilic polymer networks with high water content. The applications of hydrogels have been developed in the fields of tissue engineering, drug carriers, and chemical sensors.1–3 However, the scope of their applications is often severely limited due to the modest mechanical performances.4–6 In order to improve the mechanical properties, a series of new hydrogels7 have been developed such as topological (TP) hydrogels,8 nanocomposite (NC) hydrogels9–12 and double network (DN) hydrogels.13–15 Among these, NC hydrogels, prepared through a relatively simple technology, can not only greatly improve the mechanical properties, but also significantly improve the swelling ratio. The simultaneously enhanced mechanical and swelling properties could provide a stable environment for drug immigration and protect them from denaturation.16 By introducing rigid nanoparticles into polymer networks, a series of high-strength and tough NC hydrogels have been prepared by physical interactions (hydrogen bonding and electrostatic interaction) or chemical crosslinking between nanoparticles and polymer networks. Haraguchi et al. reported the first example of poly(N-isopropylacrylamide) NC hydrogels employing nanoclay as the crosslinker which showed improved mechanical properties (tensile strength of 41 kPa and elongation at break of 1424%) and swelling/de-swelling abilities.9 Owing to the layered structure and unique properties, two-dimensional (2D) nanomaterials are employed for the fabrication of NC hydrogels and widely studied by researchers over the past decades.17,18 However, NC hydrogels such as biocompatible layered double hydroxide (LDH) crosslinked hydrogels are vulnerable to acid and suffered dissolving, limiting their application as drug carriers.19 Besides, these investigations mainly focused on clay,9,20,21 graphene oxide (GO)22,23 and LDHs,24–26 and there are rare reports on other 2D nanomaterials.
MXenes are a new family of 2D transition metal carbides/nitrides produced by selective chemical etching of “A” from the MAX phase, where M is a transition metal, A is a IIIA or IVA element and X is C or N.27–30 Gogotsi and Barsoum28 first reported the preparation of Ti3C2 layers by selective etching of Al in Ti3AlC2 by HF, indicating their acid resistance. After the removal of Al, dangling bonds are formed on the outer layer of the Mn+1Xn structure, which can easily combine with –OH and/or –F groups in solution. The large number of –OH and –F groups on the surface of Ti3C2 facilitate further functional modification.31 In addition, monolayer MXene nanosheets with graphene-like structure can be obtained by means of organic solvent intercalation or sonication, which is of great significance for expanding the application of MXene materials.29,30 Benefitting from the unique 2D structure, excellent conductivity, biocompatibility, ion intercalation ability and hydrophilicity, MXenes are widely used in chemical sensing,32 energy storage,33–35 catalysis,36–39 and environmental restoration.39,40 Recently, Zhang and Liu41 reported the application of Ti3C2 as a light absorbent in cellulose hydrogels for biomedicine, which is crosslinked by epichlorohydrin. Nevertheless, Ti3C2 has never been reported as a crosslinker.
Herein, we report a NC hydrogel prepared from Ti3C2 and acrylamide via in situ free radical polymerization. To the best of our knowledge, this is the first report on the utilization of Ti3C2 as a crosslinker for the preparation of NC hydrogels. Compared with traditional organic crosslinked hydrogels, the as-prepared Ti3C2/polyacrylamide (PAM) NC hydrogels with ultralow Ti3C2 contents exhibit excellent mechanical properties, swelling behaviors and much better sustained drug release performance. More importantly, the Ti3C2/PAM NC hydrogels show good acid resistance in HCl solution (pH = 1.2), endowing them with practical applications under acidic conditions. The microstructures of the as-prepared hydrogels are discussed as well.
2. Experimental
2.1 Materials
Ti3AlC2 was obtained from Forsman Scientific (Beijing) Co. Ltd. Hydrofluoric acid, hydrochloric acid and acrylamide were purchased from Sinopharm Chemical Reagent Co. Ltd, China. Potassium persulfate (KPS), N,N-methylene bisacrylamide (BIS) and chloramphenicol were purchased from Aladdin Reagent Co., China. All agents were used as obtained without further purification. Deionized water was used throughout the experiment.
2.2 Instruments
Scanning electron microscopy (SEM) was performed using a HITACHI S-4800 instrument to obtain morphological images of the products. X-ray diffraction (XRD) was carried out using a D8 advance diffractometer to evaluate the crystal structure of the prepared products. Atomic force microscopy (AFM) was carried out using an ICON2-SYS instrument to determine the thickness and morphology of the Ti3C2 nanosheets. The size of the Ti3C2 nanosheets was determined by dynamic light scattering (DLS) using a BT-90. X-ray photoelectron spectroscopy (XPS) was performed to determine the binding energy of elements on an ESCALAB 250 XI. The as-prepared hydrogels were freeze-dried before SEM and XRD analyses. The mechanical properties of the hydrogels were investigated using an HY-0580 (Shanghai Heng Yi Testing Instruments Co. Ltd, China). UV-Vis absorption spectroscopy was performed using a UV-2700 (Shimadzu, Japan).
2.3 Synthesis of Ti3C2/PAM NC hydrogels
Ti3C2/PAM NC hydrogels were prepared by in situ free radical polymerization. In a typical synthesis process, Ti3C2 was obtained by etching 600 mg Ti3AlC2 with 10 mL HF (30%) under vigorous stirring for 24 h. Then the product was centrifuged, rinsed repeatedly with water and ethanol, and dried for 12 h at 60 °C in a vacuum oven. A certain amount of Ti3C2 was dispersed in 50 mL water by ultrasonication for 72 h to form Ti3C2 aqueous suspensions (0.2 g L−1, 0.4 g L−1 and 0.6 g L−1). 8 mL of the Ti3C2 suspension and 2 g acrylamide were mixed in a glass bottle placed in an ice water bath in a N2 atmosphere for 30 min to exclude oxygen gas. Subsequently, 1 mL of the redox initiator (KPS, 15 mg mL−1) was added into the mixture to initiate the in situ free radical polymerization without utilizing any organic crosslinkers. Then the mixture was allowed to react in the ice water bath under the protection of N2 for 15 min. Finally, the whole mixture was transferred into a 6 mm diameter tube for 24 h to form hydrogels. The obtained hydrogels were denoted as TnPAM hydrogels, where n represents the concentration (×10) of the Ti3C2 aqueous suspension. Freeze-dried hydrogels (xerogels) were prepared for swelling and drug release tests.
2.4 Synthesis of organic crosslinked BIS/PAM NC hydrogels
For comparison, a traditional organic crosslinked BIS/PAM hydrogel was prepared by an analogous procedure in the absence of Ti3C2. 8 mL of the Ti3C2 aqueous suspension was replaced with 8 mL BIS (15 mg) solution. The detailed reactant amounts are listed in Table S1 (ESI†).
2.5 Mechanical measurements
For the tensile tests, clubbed samples with 6 mm diameter and 60 mm length were prepared. The distance between two clamps was kept at ∼25 mm and the tensile speed was 40 mm min−1. Columned hydrogels with 15 mm height and 15 mm diameter were prepared for compressive measurements. The compressive performances were estimated at a strain up to 85%.
2.6 Swelling measurements
The swelling properties of hydrogels were evaluated by immersing ∼0.1 g xerogels in 100 g water at room temperature. At given time intervals, the swollen samples were taken out from water, wiped immediately and then weighed. The swelling ratio at time t, Qt (g g−1), was calculated using eqn (1), and the equilibrium swelling ratio Qe (g g−1) was calculated using eqn (2).where Wt is the mass of the swollen samples at time t; W0 is the mass of xerogels; We is the mass of the swollen samples at swelling equilibrium.
2.7 Estimation of drug release in hydrogels
Chloramphenicol is a broad-spectrum antimicrobial agent. However, its application is severely restricted owing to its toxicity. The development of effective drug carriers and retaining the activity of the drug at the site of action are urgently needed.42 Herein, chloramphenicol was selected as a model hydrophobic drug to investigate the drug sustained release effect of as-prepared hydrogels. A certain amount of xerogels (∼0.1 g) was immersed in 50 mL chloramphenicol solution (2 g L−1) for 60 h to allow drug molecules to migrate into the hydrogel networks. The concentration of chloramphenicol was determined by UV-Vis spectroscopy at an absorbance of λ = 278 nm and calculated by regression analysis based on the standard curve. The drug loading amount of hydrogels L (mg g−1) was calculated using eqn (3).where C0 (g L−1) and Ct (g L−1) are the concentration of chloramphenicol at initial time and time t, respectively; V0 (mL) and Vt (mL) represent the initial volume and volume at t of chloramphenicol solution, respectively. And W0 is the mass of xerogels.
The swollen hydrogels were taken out from the chloramphenicol solutions and washed with distilled water to remove the surficial chloramphenicol molecules. The in vitro release of chloramphenicol from the hydrogels was performed in hydrochloric acid solution (200 mL, pH = 1.2) at 37 °C. 3 mL of the solution was withdrawn at given time intervals and measured to calculate the percentage of chloramphenicol release from the hydrogels.
3. Results and discussion
3.1 Formation of NC hydrogels
Ti3C2 was prepared and exfoliated through a typical method according to the literature.28 Ti3C2/PAM NC hydrogels were fabricated by in situ free radical polymerization of acrylamide in an aqueous suspension of Ti3C2 nanosheets. The schematic illustration of the preparation of Ti3C2/PAM hydrogels is depicted in Scheme 1. Here, it is worth noting that Ti3C2/PAM hydrogels were synthesized at ultralow Ti3C2 contents (0.0145–0.0436%) without adding any traditional organic crosslinkers.
 |
| Scheme 1 Preparation procedures of Ti3C2/PAM hydrogels by in situ free radical polymerization with Ti3C2 nanosheets as a crosslinker. | |
The exfoliation and dispersion of 2D Ti3C2 nanosheets in solution play a key role in the fabrication of Ti3C2/PAM NC hydrogels. Ti3C2 was prepared by etching the Al layer in bulk Ti3AlC2 with 30% HF solution for 24 h at room temperature. The SEM images before (Fig. 1A) and after etching (Fig. 1B) show the exfoliated multilayer Ti3C2 after removing the sandwiched Al layer. The bulk Ti3C2 exhibits an accordion-like structure with a thickness of ∼4 μm (Fig. 1B). The highly dispersed Ti3C2 nanosheet suspension was obtained by long-term sonication of the acquired Ti3C2 multilayer solution. The AFM image shows that the multilayer Ti3C2 sheets are fully exfoliated (Fig. 1C) and the thickness of the layered Ti3C2 nanosheets is 11.4 nm (the inset image in Fig. 1C), which is much thinner than bulk Ti3C2 (∼4 μm). The size of the Ti3C2 nanosheets is about 300–400 nm, which is in accordance with the DLS result (Fig. 1D). The XPS results show the binding energy of Ti 2p, Al 2p, O 1s and C 1s before and after HF etching treatment (Fig. 1E–H). The absence of Al 2p after etching indicates the complete removal of the Al layer in Ti3AlC2 as shown in Fig. 1F. The binding energy of C 1s for Ti3C2 (Fig. 1G) at 282.0, 284.1, 284.9 and 286.3 eV corresponds to Ti–C, C–C, C–OH and C
O, respectively.30,43 The O 1s peaks of Ti3C2 (Fig. 1H) located at 529.9, 531.6 and 532.9 eV can be ascribed to Ti–O (TiOX), Ti–O (TiO2) and O–H (Ti3C2(OH)X).36 The XPS results imply the formation of –OH groups after etching treatment.
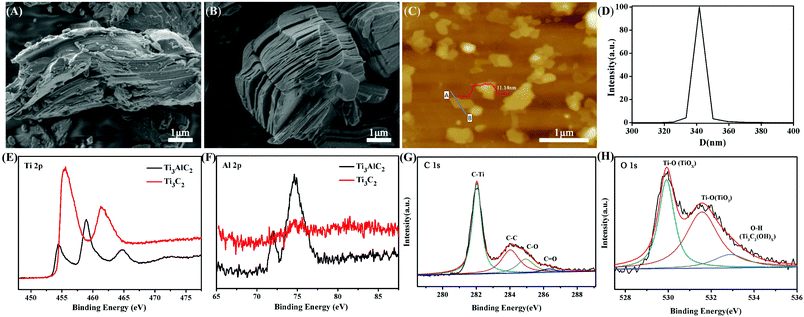 |
| Fig. 1 SEM images of (A) Ti3AlC2 and (B) bulk Ti3C2. (C) AFM images of Ti3C2 nanosheets, and the inset image in AFM shows the thickness of Ti3C2 nanosheets. (D) DLS of the Ti3C2 nanosheet suspension. XPS spectra of Ti3C2 before and after etching, (E) Ti 2p, (F) Al 2p and XPS spectra of (G) C 1s and (H) O 1s for Ti3C2. | |
Freeze-dried BIS/PAM, Ti3C2/PAM hydrogels (xerogels) were prepared for structural characterization. The XRD patterns of Ti3AlC2, Ti3C2 and Ti3C2/PAM xerogels are shown in Fig. 2. As for the raw Ti3AlC2 materials, a series of sharp peaks located at 9.5°, 19.2°, 34.0°, 36.7°, 39.0°, 41.8°, 48.5°, 52.4°, 56.5° and 60.2° can be ascribed to the (002), (004), (101), (103), (104), (105), (107), (108), (109), and (112) crystal planes of Ti3AlC2 (JCPDS card 00-052-0875), respectively. After etching by HF, an obvious loss in the crystallinity and structural order of Ti3C2 can be clearly observed in Ti3C2 diffraction patterns. A similar phenomenon was also reported in the literature.30,44 And the disappearance of the (104) peak corresponding to the characteristic peak of Ti3AlC2 demonstrates the complete removal of the Al layer in Ti3AlC2,33 which is consistent with the XPS results (Fig. 1F). It can also be observed that the (002) plane in the patterns of Ti3C2 shifted to smaller angles, indicating a higher c lattice parameter, resulting from the replacement of the Al layer with –OH and/or –F.45 In the case of Ti3C2/PAM NC hydrogels, an obvious isolated and broad peak appeared at 20.6°. No characteristic diffraction peaks of Ti3C2 can be observed in Ti3C2/PAM xerogels (Fig. 2 and Fig. S1, ESI†), elucidating the fact that no bulk Ti3C2 exists in the matrix of Ti3C2/PAM NC hydrogels and Ti3C2 nanosheets are well distributed in the hydrogel networks without aggregation. Additionally, the ultralow content of Ti3C2 is also responsible for the absence of the Ti3C2 diffraction peaks.
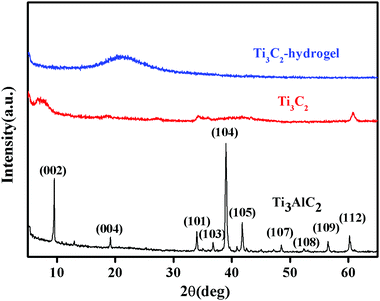 |
| Fig. 2 XRD patterns of Ti3AlC2, Ti3C2 and Ti3C2/PAM hydrogels (T6PAM). | |
The SEM images of BIS/PAM and Ti3C2/PAM NC xerogels are shown in Fig. 3. Evidently, the BIS/PAM xerogel exhibits quite different structures from the Ti3C2/PAM NC xerogel. The microstructure of the BIS/PAM xerogel appears with irregular and collapsed pores of 1–3 μm after the removal of water from the matrix (Fig. 3B). Moreover, the pores are sandwiched by solid bulk structures (Fig. 3A). This structural inhomogeneity always occurs in organic crosslinked hydrogels,46 especially when the concentration of the crosslinker is high.47 Unlike the BIS/PAM xerogel, the Ti3C2/PAM NC xerogel shows a honeycomb-like fine structure with a uniform pore distribution in the range of 2–3 μm (Fig. 3C and D). The pores and structure are much more uniform and regular than those of the BIS/PAM xerogel. The uniform and fine structures of Ti3C2/PAM NC hydrogels are beneficial for stress release and migration of drug molecules, promising enhanced mechanical properties and drug release performances.25,48 Additionally, no obvious aggregation of bulk Ti3C2 can be observed in Ti3C2/PAM NC hydrogel networks, demonstrating that Ti3C2 nanosheets are well dispersed during Ti3C2/PAM NC hydrogel formation, which is in good accordance with XRD characterization (Fig. 2). Furthermore, the energy dispersive spectroscopy (EDS) mapping images of elements C and Ti (Fig. 3E and F) for T2PAM confirm that Ti3C2 nanosheets are uniformly distributed in the network of Ti3C2/PAM NC hydrogels, acting as crosslinkers.
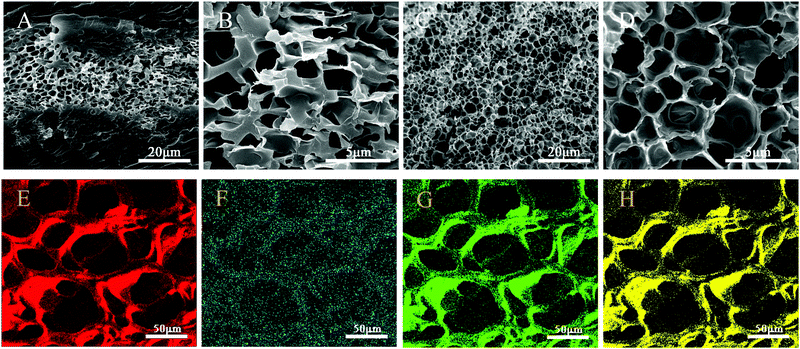 |
| Fig. 3 SEM images of (A and B) BIS/PAM (T0PAM) xerogels, (C and D) Ti3C2/PAM (T6PAM) xerogels. EDS mapping images of C (E), Ti (F), N (G) and O (H) for T2PAM xerogels. | |
3.2 Mechanical properties
Tensile and compressive tests were performed to evaluate the mechanical properties of as-synthesized hydrogels. The as-prepared Ti3C2/PAM NC hydrogels can withstand high degrees of deformations, such as elongation, bending, knotting and compression (Fig. 4). The Ti3C2/PAM NC hydrogels can be easily elongated over ten times and have a high level of 180 degrees bending (Fig. 4A and B). No fractures were observed on the surface of the Ti3C2/PAM NC hydrogels, even when they were made into a knot followed by elongation (Fig. 4C and D). Under high pressure, Ti3C2/PAM NC hydrogels showed an obvious strain without any breakage or damage. In addition, the hydrogels can automatically and rapidly recover after removing the compression force (Fig. 4E–G). Tensile and compressive tests were performed to quantitatively evaluate the mechanical properties of the as-prepared hydrogels (Fig. 4H and I). The obtained values including elongation at break (λts), fracture strength (σf) and compressive strength (σc) are listed in Table 1. In the case of tensile properties, the Ti3C2/PAM NC hydrogels exhibit much higher break elongation and fracture strength (2158.6–3047.5%, 66.5–102.7 kPa) than organic crosslinked BIS/PAM hydrogels (109.6%, 32.0 kPa). The participation of Ti3C2 dramatically improves the tensile properties of the hydrogels. Besides, Ti3C2/PAM NC hydrogels also exhibit dramatically high stress (400.6–819.4 kPa) in compressive tests. The compression properties of BIS/PAM hydrogels cannot be measured due to their brittle nature.49 The enhanced mechanical performances can be attributed to the uniformly dispersed micropores and more flexible linear polymer chains (confirmed by the later estimation of hydrogel microstructures), which facilitate the energy dissipation during the deformations.23,24 The tensile stresses of the as-prepared Ti3C2/PAM NC hydrogels are higher than those of clay, LDH and C-dot crosslinked NC hydrogels, slightly inferior to those of GO/PAM NC hydrogels (Table S2, ESI†). Furthermore, the mechanical performance of Ti3C2/PAM NC hydrogels improved with the content of Ti3C2. These results reveal that the mechanical properties of Ti3C2/PAM NC hydrogels can be tuned by adjusting the content of Ti3C2, which shows great potential in improving the mechanical properties of hydrogels. The improved mechanical properties are of vital importance for fabricating stable networks and practically utilizing NC hydrogels in various applications, such as catalysis, chemical sensors and drug carrier systems.
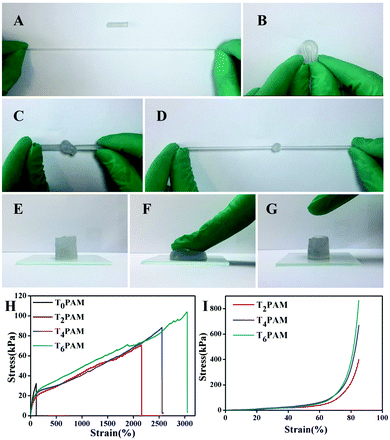 |
| Fig. 4 Deformations of as-prepared Ti3C2/PAM (T6PAM) hydrogels. (A) elongation, (B) bending, (C) knotting, (D) knotting and elongation, and (E–G) compression. Stress–strain curves of (H) tensile and (I) compressive tests for BIS/PAM (T0PAM) and Ti3C2/PAM (T2PAM, T4PAM, T6PAM) hydrogels. | |
Table 1 Mechanical properties of BIS/PAM (T0PAM) and Ti3C2/PAM (T2PAM, T4PAM, and T6PAM) hydrogels
Sample |
Ti3C2 content (%) |
λ
ts (%) |
σ
f (kPa) |
σ
c (kPa) |
λ
ts: elongation at break; σf: fracture strength; σc: compressive strength at a strain up to 85%. |
T0PAM |
0 |
109.6 |
32.0 |
|
T2PAM |
0.0145 |
2158.6 |
66.5 |
400.6 |
T4PAM |
0.0291 |
2555.0 |
88.4 |
670.0 |
T6PAM |
0.0436 |
3047.5 |
102.7 |
819.4 |
3.3 Swelling behavior and in vitro drug release
The swelling properties of BIS/PAM and Ti3C2/PAM NC xerogels were investigated by immersing ∼0.1 g xerogels in 100 g water at room temperature. A good swelling performance is beneficial for the uptake of drug solutions.50 All the samples display similar swelling behaviors (Fig. 5A). The water uptake can be attributed to hydrogen bonding interactions between the hydrophilic groups (–CONH2) of polymer chains, the functional groups (–OH, –F) on the Ti3C2 surface and the H2O molecules in aqueous solution. The equilibrium swelling ratios of the Ti3C2/PAM NC hydrogels (∼16–33 g g−1) are higher than that of BIS/PAM hydrogels (9.6 g g−1), indicating enhanced swelling performances of Ti3C2/PAM NC hydrogels. The water contents after reaching the equilibrium swelling ratios were calculated to be 89.6%, 97.0%, 95.0% and 93.8% for T0PAM, T2PAM, T4PAM, and T6PAM, respectively, which were higher than those of the original samples (81.8%, Table S1, ESI†). The Ti3C2 nanosheet crosslinked NC hydrogels significantly improved the swelling performance of the PAM hydrogels, which might be ascribed to the fact that flexible polymer chains have few limitations between nanosheets (confirmed by the later estimation of hydrogel microstructures).9,22,25 Besides, the uniform distribution of the pores and hydrophilic groups on the Ti3C2 nanosheets might also facilitate the uptake of water. Furthermore, the swelling ratio of the Ti3C2/PAM NC hydrogels decreased with the increase of the Ti3C2 content, indicating that an adjustable swelling ratio was achieved by controlling the Ti3C2 content. Ti3C2/PAM NC hydrogels need a longer time (∼70 h) to reach the swelling equilibrium state than BIS/PAM hydrogels (∼35 h) due to their high swelling equilibrium ratios. Moreover, no dissolving phenomenon was observed, demonstrating the formation of a stable and tough network between the Ti3C2 nanosheets and the PAM chains in the Ti3C2/PAM NC hydrogels.
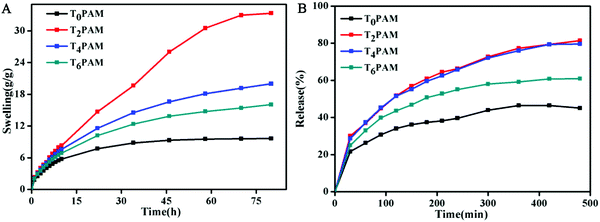 |
| Fig. 5 (A) Swelling kinetics and (B) chloramphenicol release in HCl (pH = 1.2) of freeze-dried BIS/PAM (T0PAM) and Ti3C2/PAM (T2PAM, T4PAM, T6PAM) hydrogels. | |
Chloramphenicol was selected as a model hydrophobic drug to investigate the drug sustained release effect of the as-prepared hydrogels.51 The cumulative release effect of chloramphenicol from freeze-dried hydrogels was determined in HCl solution (pH = 1.2) at 37 °C (Fig. 5B). The drug loads were calculated to be 46.4, 127.7, 126.2 and 97.5 mg g−1 for T0PAM, T2PAM, T4PAM and T6PAM, respectively (Fig. S2, ESI†). Ti3C2/PAM NC hydrogels exhibited higher drug loads than BIS/PAM hydrogels. This drug load difference can be attributed to the uniform porous structure and excellent swelling behavior of Ti3C2/PAM NC hydrogels. The drug release reached an equilibrium at ∼360 min for T0PAM, slightly faster than those of Ti3C2/PAM NC hydrogels (∼420 min). The slightly slower equilibrium for NC hydrogels might result from the fact that NC hydrogels exhibited higher drug loads. Furthermore, the percentage release values (Fig. S3, ESI†) of chloramphenicol from Ti3C2/PAM NC hydrogels are 81.4%, 79.6%, and 62.1% (T2PAM, T4PAM and T6PAM, respectively), which are much higher than that of BIS/PAM hydrogels (45.0%). That is to say, higher drug loads and higher percentage release values were simultaneously achieved in Ti3C2/PAM NC hydrogels. The uniform pore distribution facilitates the migration of drug molecules and leads to higher drug release percentages. Unlike LDH-based nanocomposites,19 no dissolving phenomenon was observed throughout the in vitro drug release tests, indicating that Ti3C2/PAM NC hydrogels are acid resistant. Thus, a stable and mechanical strong network was constructed for improving the drug release performance of NC hydrogels.
3.4 Estimation of hydrogel microstructures
The excellent mechanical properties and swelling behaviors can be attributed to the fine microstructures of the Ti3C2/PAM NC hydrogels. It has been revealed that NC hydrogels have a much lower crosslinking density (the number of network chains per unit volume, N*)52 and a higher molecular weight of polymer chains between crosslinking points (the molecular weight of chains between crosslinks, Mc) than those of traditional organic crosslinked hydrogels.9,24,53,54 The higher Mc values endow the polymer chains in hydrogels with flexibility and extensibility to endure deformations. The classic theory of rubber elasticity was applied to estimate the N* and Mc values.52
The classical equation of networks for tensile stress is24,52–54
where
F is the force per unit original cross sectional area of the hydrogel (
F = force/
A0).
Φ,
k and
T are the front factor, Boltzmann's constant and absolute temperature, respectively.
α is the extension ratio (
α =
L/
L0). Here, let
Φ = 1 and
F at
α = 2 (
λts = 100%),
N* can be calculated using
eqn (4).
24,53,54 The
Mc value is determined using the following equation
53,54where
ρ* is the density of the polymer in hydrogels and
NA is Avogadro's constant.
The calculated N* and Mc of BIS/PAM and Ti3C2/PAM NC hydrogels are listed in Table 2. Compared with the BIS/PAM hydrogel (4.39 × 1018 chains per cm3 and 2.6 × 104 g mol−1), all Ti3C2/PAM NC hydrogels exhibit lower N* values (2.63 × 1018 ∼ 3.15 × 1018 chains per cm3) and higher Mc values (3.7 × 104 g mol−1 ∼ 4.3 × 104 g mol−1). The longer polymer chains in the hydrogels enable hydrogels bear higher level of deformations without severing the constituent polymer chains and give rise to enhanced mechanical properties. When it comes to BIS/PAM hydrogels, the higher density of chains (N*) and shorter length of chains between crosslinking points (Mc) indicate severe restriction of polymer chains and lead to their brittle and weak mechanical performance.
Table 2 Parameters of crosslinking networks for the BIS/PAM (T0PAM) hydrogel and Ti3C2/PAM (T2PAM, T4PAM, and T6PAM) NC hydrogels
Sample |
F
α=2 (kPa) |
N* (1018 chains per cm3) |
M
c (104 g mol−1) |
n (1014 sheets per cm3) |
N*/n (chains per sheet) |
T0PAM |
30.6 |
4.39 |
2.6 |
— |
— |
T2PAM |
18.3 |
2.63 |
4.3 |
1.04 |
25 247 |
T4PAM |
21.1 |
3.03 |
3.8 |
2.09 |
14 471 |
T6PAM |
22.0 |
3.15 |
3.7 |
3.18 |
9898 |
By assuming Ti3C2 nanosheets as disk-like sheets with a diameter of 350 nm and a thickness of 11 nm (according to the AFM and DLS results, Fig. 1C and D), the number of sheets per cm3 (n), and the number of chains per sheet (N*/n) can be obtained.9,25,53 The results are also listed in Table 2. The so-obtained values of N*/n indicated that thousands of (0.99 × 104–2.5 × 104) polymer chains were grafted on the surface of the Ti3C2 nanosheets.
The crosslinking points of BIS/PAM hydrogels are estimated to be 5.6 × 1018 sites per cm3 depending on the BIS content.25,53 The inter-crosslinking distance of the polymer chains in NC hydrogels is deemed to be equivalent to the distance between adjacent inorganic nanosheets9,24,53,54 owing to the fact that the number of sheets per unit volume in Ti3C2/PAM NC hydrogels (1.04 × 1014–3.18 × 1014) is much smaller than that of BIS/PAM hydrogels (5.6 × 1018 sites per cm3). Thus, the inter-crosslinking distance of polymer chains in NC hydrogels is much longer than that in BIS/PAM hydrogels. Hence, the crosslinked polymer chains in Ti3C2/PAM NC hydrogels are more flexible and behave more like free, linear polymers.24,54,55 That is to say, the polymers in NC hydrogels have less restriction with regard to swelling and mechanical characterization in comparison with those in BIS/PAM hydrogels.
4. Conclusions
In summary, we have successfully fabricated Ti3C2/PAM NC hydrogels by in situ free radical polymerization. The Ti3C2 nanosheets are proved to be evenly distributed in the matrix of Ti3C2/PAM NC hydrogels and act as inorganic crosslinkers. Compared with BIS/PAM hydrogels, Ti3C2/PAM NC hydrogels exhibit excellent mechanical properties, high degrees of deformations, extraordinary stretching ability and enhanced swelling properties owing to their uniform fine structures. When the content of Ti3C2 is only 0.0436%, the fracture strength and break elongation of Ti3C2/PAM NC hydrogels are 102.7 kPa and 3047.5%, respectively. Furthermore, the tensile and compressive mechanical performances of the Ti3C2/PAM NC hydrogels increase with joining Ti3C2 while the swelling ratio decreases, making it possible to tune the performances by controlling the contents of Ti3C2. In the case of drug release performance, Ti3C2/PAM NC hydrogels exhibit higher drug loads and drug release percentages simultaneously (97.5–127.7 mg g−1 and 62.1–81.4%), showing their practical applications in biomedicine. The uniform pore structures and flexible linear polymer chains are suggested to account for the high mechanical strength and enhanced drug release performance. It is supposed that the incorporation of Ti3C2 might endow hydrogels with electrical conductivity, catalytic performance and mechanical performances. The new Ti3C2 crosslinked NC hydrogel may pave the way for its applications in gel electrolytes, chemical sensors and hydrogel-based supercapacitors.
Conflicts of interest
There are no conflicts to declare.
Acknowledgements
We acknowledge the funding from the National Natural Science Foundation of China (No. 21502227), the Fundamental Research Funds for the Central Universities (No. 19CX02040A, 15CX02015A, and 16CX05009A), the State Key Laboratory of Heavy Oil Processing (No. SLKZZ-2017009), the Shandong Province Major Science and Technology Innovation Project (No. 2018CXGC1002), and the Key Laboratory of Photochemical Conversion and Optoelectronic Materials, TIPC, CAS.
Notes and references
- K. Y. Lee and D. J. Mooney, Chem. Rev., 2001, 101, 1869 CrossRef CAS.
- O. P. Varghese, W. Sun, J. Hilborn and D. Ossipov, J. Am. Chem. Soc., 2009, 131, 8781 CrossRef CAS.
- J. H. Holtz and S. A. Asher, Nature, 1997, 389, 829 CrossRef CAS.
- P. Calvert, Adv. Mater., 2009, 21, 743 CrossRef CAS.
- J.-Y. Sun, X. Zhao, W. R. K. Illeperuma, O. Chaudhuri, K. H. Oh, D. J. Mooney, J. J. Vlassak and Z. Suo, Nature, 2012, 489, 133 CrossRef CAS.
- Y. Sun, G. Gao, G. Du, Y. Cheng and J. Fu, ACS Macro Lett., 2014, 3, 496 CrossRef CAS.
- A. Wu, X. Gao, P. Sun, F. Lu and L. Zheng, Angew. Chem., Int. Ed., 2018, 57, 4025 CrossRef CAS.
- Y. Okumura and K. Ito, Adv. Mater., 2001, 13, 485 CrossRef CAS.
- K. Haraguchi and T. Takehisa, Adv. Mater., 2002, 14, 1120 CrossRef CAS.
- H.-J. Li, H. Jiang and K. Haraguchi, Macromolecules, 2018, 51, 529 CrossRef CAS.
- J. Xu, Y. Zhang, W. Zhu and Y. Cui, Macromolecules, 2018, 51, 2672 CrossRef CAS.
- J. Du, S. Xu, S. Feng, L. Yu, J. Wang and Y. Liu, Soft Matter, 2016, 12, 1649 RSC.
- S. Gu, L. Duan, X. Ren and G. H. Gao, J. Colloid Interface Sci., 2017, 492, 119 CrossRef CAS.
- J. Gong, Y. Katsuyama, T. Kurokawa and Y. Osada, Adv. Mater., 2003, 15, 1155 CrossRef CAS.
- J. Gong, Soft Matter, 2010, 6, 2583 RSC.
- M. Kurisawa, L. Fan, L. Wang and J. Chung, J. Mater. Chem., 2010, 20, 5371 RSC.
- X. Cai, Y. Luo, B. Liu and H.-M. Cheng, Chem. Soc. Rev., 2018, 47, 6224 RSC.
- Y. Chen, Z. Fan, Z. Zhang, W. Xin, C. Li, N. Yang, B. Chen and H. Zhang, Chem. Rev., 2018, 118, 6409 CrossRef CAS.
- Y. Zhang, H. Li, N. Du, S. Song and W. Hou, Appl. Clay Sci., 2017, 143, 336 CrossRef CAS.
- T. Su, L. Wu, X. Pan, C. Zhang, M. Shi, R. Gao, X. Qi and W. Dong, J. Colloid Interface Sci., 2019, 542, 253 CrossRef CAS.
- L. Z. Zhao, C. H. Zhou, J. Wang, D. S. Tong, W. H. Yu and H. Wang, Soft Matter, 2015, 11, 9229 RSC.
- R. Liu, S. Liang, X.-Z. Tang, D. Yan, X. Li and Z. Z. Yu, J. Mater. Chem., 2012, 22, 14160 RSC.
- S. Liu, A. K. Bastola and L. Li, ACS Appl. Mater. Interfaces, 2017, 9, 41473 CrossRef CAS.
- Z. Hu and G. Chen, Adv. Mater., 2014, 26, 5950 CrossRef CAS.
- Y. Zhang, J. Ji, H. Li, N. Du, S. Song and W. Hou, Soft Matter, 2018, 14, 1789 RSC.
- X.-J. Yang, P. Zhang, P. Li, Z. Li, W. Xia, H. Zhang, Z. Di, M. Wang, H. Zhang and Q. J. Niu, J. Mol. Liq., 2019, 280, 128 CrossRef CAS.
- M. Naguib, O. Mashtalir, J. Carle, V. Presser, J. Lu, L. Hultman, Y. Gogotsi and M. W. Barsoum, ACS Nano, 2012, 6, 1322 CrossRef CAS.
- M. Naguib, M. Kurtoglu, V. Presser, J. Lu, J. Niu, M. Heon, L. Hultman, Y. Gogotsi and M. W. Barsoum, Adv. Mater., 2011, 23, 4248 CrossRef CAS.
- H. Lin, Y. Wang, S. Gao, Y. Chen and J. Shi, Adv. Mater., 2018, 30, 1703284 CrossRef.
- O. Mashtalir, M. Naguib, V. N. Mochalin, Y. Dall’Agnese, M. Heon, M. W. Barsoum and Y. Gogotsi, Nat. Commun., 2013, 4, 1716 CrossRef.
- C. Dai, Y. Chen, X. Jing, L. Xiang, D. Yang, H. Lin, Z. Liu, X. Han and R. Wu, ACS Nano, 2017, 11, 12696 CrossRef CAS.
- J. Chen, K. Chen, D. Tong, Y. Huang, J. Zhang, J. Xue, Q. Huang and T. Chen, Chem. Commun., 2015, 51, 314 RSC.
- M. Boota, B. Anasori, C. Voigt, M. Q. Zhao, M. W. Barsoum and Y. Gogotsi, Adv. Mater., 2016, 28, 1517 CrossRef CAS.
- X. Wang, S. Kajiyama, H. Iinuma, E. Hosono, S. Oro, I. Moriguchi, M. Okubo and M. Yamada, Nat. Commun., 2015, 6, 6544 CrossRef CAS.
- M.-Q. Zhao, C. E. Ren, Z. Ling, M. R. Lukatskaya, C. Zhang, K. L. V. Aken, M. W. Barsoum and Y. Gogotsi, Adv. Mater., 2015, 27, 339 CrossRef CAS.
- J. Guo, Y. Zhao, A. Liu and T. Ma, Electrochim. Acta, 2019, 305, 164 CrossRef CAS.
- J. Ran, G. Gao, F.-T. Li, T.-Y. Ma, A. Du and S.-Z. Qiao, Nat. Commun., 2016, 8, 13907 CrossRef.
- W. Zhou, J. Zhu, F. Wang, M. Cao and T. Zhao, Mater. Lett., 2017, 206, 237 CrossRef CAS.
- O. Mashtalir, K. M. Cook, V. N. Mochalin, M. Crowe, M. W. Barsoum and Y. Gogotsi, J. Mater. Chem. A, 2014, 2, 14334 RSC.
- Q. Zhang, J. Teng, G. Zou, Q. Peng, Q. Du, T. Jiao and J. Xiang, Nanoscale, 2016, 8, 7085 RSC.
- C. Xing, S. Chen, X. Liang, Q. Liu, M. Qu, Q. Zhou, J. Li, H. Tan, L. Liu, D. Fan and H. Zhang, ACS Appl. Mater. Interfaces, 2018, 10, 27631 CrossRef CAS.
- S. G. Ingebrigtsen, A. Didriksen, M. Johannessen, N. Škalko-Basnet and A. M. Holsæter, Int. J. Pharm., 2017, 526, 538 CrossRef CAS.
- M. R. Lukatskaya, J. Halim, B. Dyatkin, M. I. Naguib, Y. S. Buranova, M. W. Barsoum and Y. Gogotsi, Angew. Chem., Int. Ed., 2014, 53, 4877 CrossRef CAS.
- Y. Sun, D. Jin, Y. Sun, X. Meng, Y. Gao, Y. Dall’Agnese, G. Chen and X.-F. Wang, J. Mater. Chem. A, 2018, 6, 9124 RSC.
- M. Naguib, V. N. Mochalin, M. W. Barsoum and Y. Gogotsi, Adv. Mater., 2014, 26, 992 CrossRef CAS.
- K. Shi, Z. Liu, C. Yang, X.-Y. Li, Y.-M. Sun, Y. Deng, W. Wang, X.-J. Ju, R. Xie and L.-Y. Chu, ACS Appl. Mater. Interfaces, 2017, 9, 21979 CrossRef CAS.
- M. Shibayama, S.-I. Takata and T. Norisuye, Physica A, 1998, 249, 245 CrossRef CAS.
- C. Teng, J. Qiao, J. Wang, L. Jiang and Y. Zhu, ACS Nano, 2016, 10, 413 CrossRef CAS.
- J. Shen, B. Yan, T. Li, Y. Long, N. Li and M. Ye, Soft Matter, 2012, 8, 1831 RSC.
- N. Cheng, Q. Hu, Y. Guo, Y. Wang and L. Yu, ACS Appl. Mater. Interfaces, 2015, 7, 10258 CrossRef CAS.
- G. Giammanco, C. Sosnofsky and A. Ostrowski, ACS Appl. Mater. Interfaces, 2014, 7, 3068 CrossRef.
- A. Tobolsky, D. Carlson and N. Indictor, J. Polym. Sci., Polym. Chem., 1961, 54, 175 CAS.
- K. Haraguchi, T. Toru and S. Fan, Macromolecules, 2002, 35, 10162 CrossRef CAS.
- L. Wu, Z. Hu, G. Chen and Z. Li, Soft Matter, 2015, 11, 9038 RSC.
- M. Hu, Y. Yang, X. Gu, Y. Hu, Z. Du and C. Wang, Macromol. Mater. Eng., 2015, 300, 1043 CrossRef CAS.
Footnote |
† Electronic supplementary information (ESI) available: Compositions of reactants for as-prepared Ti3C2/PAM and BIS/PAM hydrogels, XRD patterns of hydrogels, mechanical properties of NC hydrogels reported in the literature, and UV-Vis spectra of chloramphenicol in vitro released from hydrogels. See DOI: 10.1039/c9sm01985e |
|
This journal is © The Royal Society of Chemistry 2020 |