DOI:
10.1039/D1AN01826D
(Paper)
Analyst, 2022,
147, 66-71
Halogen-modified carbazole derivatives for lipid droplet-specific bioimaging and two-photon photodynamic therapy†
Received
8th October 2021
, Accepted 5th November 2021
First published on 8th November 2021
Abstract
Lipid droplets (LDs) are dynamic multifunctional organelles that participate in the regulation of many metabolic processes, visualization of which is necessary for biological research. In this work, a series of two-photon responsive fluorescent probes (C–H, C–Br, and C–I) based on carbazole units were designed and synthesized. Thereinto, an iodine-modified carbazole derivative C–I exhibited an exciting lipid droplet targeting ability due to its excellent lipophilicity. Meanwhile, benefiting from its larger Stokes shift and two-photon absorption cross-section, C–I was employed for two-photon confocal laser scanning microscopy (CLSM) and stimulated emission depletion (STED) microscopy imaging to observe LDs more accurately. In addition, given the heavy atom effect, C–I can effectively generate reactive oxygen species (ROS) leading to cancer cell apoptosis under near-infrared light irradiation. Notably, we explained the process of cell apoptosis through in vitro simulation experiments. This study provides a promising platform for visualization of lipid droplets.
1. Introduction
Lipid droplets (LDs) are multifunctional suborganelles containing different neutral lipids (such as trioctanoic acid and cholesterol esters) surrounded by a single layer of phospholipids, which play important roles in dynamically regulating the balance of cellular energy.1–6 In addition, numerous studies show that abnormal lipid storage often leads to a variety of metabolic diseases,7 such as obesity, fatty liver, cardiovascular disease, diabetes,8,9etc. Therefore, it is important to provide a valid method to monitor LDs for biomedical research.
In recent years, the technology used for LD imaging has become more and more mature, such as 1H NMR spectroscopy10 and Raman scattering imaging.11 However, the difficult operation and heavy cost have seriously hindered their further research in the field of biology. Since fluorescence imaging technology has the advantages of low cost, high sensitivity, easy operation, and an extremely wide application range, it has been widely used in biological imaging analysis and detection.12–15 In this regard, several conventional fluorescent probes have been used for LD imaging, such as Sudan Red III, BODIPY493/503 and Nile red. However, the excitation wavelength of these is relatively short (usually at 488 nm) causing damage to cells and making it difficult to achieve long-term monitoring of living cells.16–19 In this sense, two-photon fluorescent probes excited by the near-infrared light can overcome the photobleaching and phototoxicity of the conventional fluorescent probes, which are more suitable for biological detection and imaging.20–27 In particular, the search for two-photon fluorescent probes, which provide deeper penetration and non-invasive lipid droplet targeting and bioimaging, is a highly desired target.28–37
Considering the above, a series of two-photon responsive fluorescent probes (C–H, C–Br and C–I), consisting of an electron-donating unit (D, carbazole), electron-accepting unit (A, 2-(1-(pyridin-4-yl)ethylidene)malononitrile) and a π-bridge, have been synthesized for recognizing LDs at the cellular level. Herein, the introduction of long alkyl chains and halogen atoms (Br and I) increases their lipophilic nature and improves their penetration of the lipid membrane.38 Among them, C–I showed the best lipophilicity and the largest Stokes shift. Moreover, C–I exhibited a considerable two-photon absorption cross-section (363 GM) at 880 nm excitation in the near-infrared region. Therefore, we have conducted in-depth research on its lipid droplet targeting ability by stimulated emission depletion (STED) microscopy and two-photon fluorescence imaging. In addition, the application of C–I in two-photon photodynamic therapy was further discussed given its excellent singlet oxygen (1O2) generation ability (Scheme 1). We also explained the process of cell apoptosis through in vitro simulation experiments.
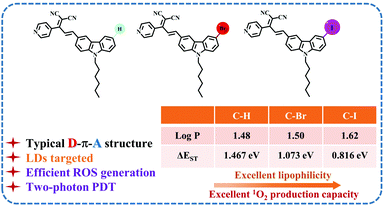 |
| Scheme 1 Schematic illustration of the structures of the halogen-modified carbazole derivatives in this work (C–H, C–Br and C–I) and the related performance. | |
2. Results and discussion
2.1 Structural characteristics
Three D–π-A carbazole derivatives, C–H, C–Br and C–I (the synthetic route for C–H, C–Br, and C–I complexes is shown in Scheme S1†), were obtained through the Claisen–Schmidt condensation reaction with moderate yields. Fortunately, we obtained a single crystal structure of C–Br (CCDC 2095605) (Fig. 1a), and the dihedral angle of the P1 (a donor moiety) and P2 (an acceptor moiety) planes was 12.54° (Fig. 1b), suggesting its considerable planarity. In addition, the bond length of C(19)–C(20) for C–Br was 1.335 Å, which is between the normal single (1.53 Å) and double (1.32 Å) bond lengths, indicating its strong electron delocalization feature within the molecule (Table S3†).It is noteworthy that according to the theoretical calculations (Fig. S12†), the introduction of H, Br and I atoms in the molecular tail plays a negligible role in the molecular configuration. This implied that C–H and C–I possessed considerable planarity and an electron delocalization character, revealing their promising two-photon activity as well.
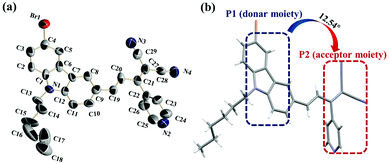 |
| Fig. 1 (a) The ORTEP structure of C–Br with an atomic labeling scheme (with 50% thermal ellipsoid probability); all hydrogen atoms are omitted for clarity. (b) Schematic diagram of the dihedral angle and special bond length of C–Br. | |
2.2 Photophysical properties
The optical properties of C–H, C–Br and C–I were studied by UV-vis and photoluminescence (PL) spectroscopy. As shown in Fig. 2a, the maximum absorption peak and maximum emission peak of C–H, C–Br and C–I were located around 470 nm and 590 nm in DMSO solution, respectively, with a large Stokes shift (∼120 nm). The large Stokes shift of the three compounds implied their potential biological application value for STED microscopy imaging.
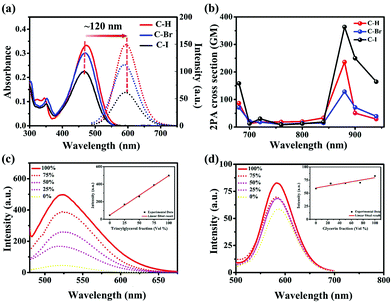 |
| Fig. 2 (a) UV-vis absorption and fluorescence spectra of C–H, C–Br and C–I (10 μM) in DMSO solution. (b) Two-photon absorption cross-sections of C–H, C–Br and C–I (excitation wavelength: 680–940 nm, identical energy: 500 mW, c = 1 mM). (c) Emission spectra of C–I in PBS with different trioctanoin ratios (10 μM). Inset: a linear relationship between the fluorescence intensity and ratio of trioctanoin, R2 = 0.997. (d) Emission spectra of C–I (10 μM) in PBS with different glycerol fractions. Inset: a linear relationship between the fluorescence intensity and ratio of glycerol, R2 = 0.780. | |
In addition, the theoretical calculation results showed that the electron cloud of the HOMO was distributed in the electron-donating carbazole group, while the electron cloud of the LUMO was transferred and distributed in the electron-accepting dicyanopyridine group. In this process, the HOMO and LUMO of the three compounds showed obvious intramolecular charge transfer (ICT) characteristics, which may be beneficial for the nonlinear optical properties of the compounds (Fig. S12†). Moreover, the emission wavelengths of C–H, C–Br and C–I obviously showed positive solvation effects at an excitation wavelength of 470 nm with the increase of solvent polarity. This positive solvation effect was consistent with the characteristics of ICT (Fig. S10, and Table S1†).
The above results motivated us to investigate the nonlinear optical properties of C–H, C–Br, and C–I by a two-photon excited fluorescence (TPEF) method. The slope of the logarithmic fitting curve of two-photon fluorescence and laser power was ≈2, verifying their two-photon characteristics (Fig. S13 and S14†). The two-photon absorption cross-section (σ2PA) in the wavelength range of 680–940 nm was calculated. Among them, the two-photon absorption cross-section of C–I was the largest (363 GM) (Fig. 2b), indicating that it can be an excellent candidate for two-photon fluorescence imaging.
2.3 Lipophilicity
The introduction of long alkyl chains (-hexyl) and halogens (Br and I) endows them (C–H, C–Br and C–I) with considerable lipophilicity. The logarithm of the octanol–water partition coefficient (log
P) estimated by the oil–water separation experiment was 1.48 (C–H), 1.50 (C–Br) and 1.62 (C–I), respectively (Fig. S15†), suggesting their considerable lipophilicity and implying the potential ability to target LDs.39
2.4 The sensitivity to LD simulators in vitro
As is known, LDs are mainly composed of triglycerides.40 Before bioimaging experiments, the sensitivity of C–H, C–Br and C–I to trioctanoin (a triglyceride analogue, LD simulator) was studied in detail. As shown in Fig. S16† and Fig. 2c, the fluorescence intensity of C–H, C–Br and C–I in pure PBS was relatively weak. However, the fluorescence intensity gradually enhanced with the increase of the ratio of trioctanoin, indicating the high sensitivity of C–H, C–Br and C–I to the LD simulator. In order to eliminate the effect of the viscosity of trioctanoin, the emission spectra were collected with different ratios of glycerol/PBS mixtures. As the proportion of glycerin in the solution increased, the fluorescence intensity of the solution remained almost unchanged (Fig. S17† and Fig. 2d), suggesting their negligible sensitivity to viscosity. The above results revealed the high sensitivity of C–H, C–Br and C–I to trioctanoin (LD in vitro simulator), implying their potential lipid droplet-specific targeting ability.41,42
2.5 LD-specific bioimaging in HeLa cells
The sensitivity of C–H, C–Br and C–I to trioctanoin motivated us to investigate their preliminary biological applications. As shown in Fig. S18 and S19,†C–H, C–Br and C–I showed excellent light stability and low dark toxicity, which were in line with the biological experimental conditions. Subsequently, as shown in Fig. 3a and Fig. S20,† the high overlap of C–H, C–Br and C–I with the commercial LD dye HCS LipidTOX™ Deep Red exhibited their LD targeting capacity. Thereinto, the Pearson coefficient of C–I and HCS LipidTOX™ Deep Red reached 0.89, showing its higher targeting ability for lipid droplets. Furthermore, the two-photon imaging experiments and 3D images clearly showed the LD-specific targeting ability of C–I (Fig. 3b and Fig. S21†).
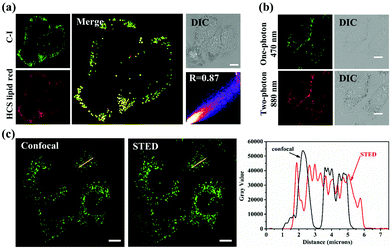 |
| Fig. 3 (a) Determination of the intercellular localization of C–I by confocal microscopy in HeLa cells: colocalization images of HeLa cells stained with HCS Deep Red (0.5 μM, red channel, λex = 633 nm, λem = 650 nm); C–I (10 μM, green channel, λex = 470 nm, λem = 580 ± 20 nm); a merged image (yellow channel); and a bright-field image of C–I and the Pearson coefficient. Scale bar: 10 μm. (b) One-photon (λex = 470 nm, λem = 594 nm) and two-photon (λex = 880 nm, λem = 594 nm) fluorescence images of live HeLa cells incubated with 4 μM C–I and the bright-field image of C–I. Scale bar: 10 μm; (c) confocal fluorescence images of living HeLa cells incubated with 4 μM C–I in DMSO/PBS (λex = 470 nm, λem = 594 nm, scale bar: 10 μm). The STED micrographs of C–I staining lipid droplets with a higher resolution using a 470 nm STED depletion laser (λex = 470 nm, λem = 594 nm, scale bar: 10 μm). | |
Benefiting from the large Stokes shift (∼120 nm) (Fig. 2a) and high selectivity to LDs of C–I, its LD targeting and imaging abilities were further evaluated via stimulated emission depletion (STED) microscopy in living HeLa cells. As shown in Fig. 3c, compared with the traditional confocal lipid droplet imaging, super-resolution imaging showed more details on the lipid droplets with much higher resolution. The above results indicated that C–I can be used as a tool for monitoring lipid droplets by two-photon fluorescence and STED imaging with high resolution.
2.6 Detection of ROS in vitro
During the bioimaging assay upon excitation at 880 nm, it was found that the HeLa cells stained with C–Br and C–I showed different degrees of apoptosis over time. Considering the introduction of heavy atoms (Br and I) with the molecular framework, the increased intersystem crossing (ISC) ability enabled their reactive oxygen species (ROS) generation ability to cause cell apoptosis.42–44 Then, the singlet-triplet energy gaps (ΔEST) of C–H, C–Br and C–I were calculated using time-dependent density functional theory (TD-DFT) and were found to be 1.467 eV, 1.073 eV and 0.816 eV, respectively. The smallest ΔEST of C–I was conducive to ROS generation and provided a theoretical basis for photodynamic therapy (PDT).45–47 Subsequently, we chose 2,7-dichlorofluorescein (DCFH-DA) as an indicator to test the ROS generation ability of C–H, C–Br, and C–I in PBS solution upon light irradiation (400–800 nm, 60 mW cm−2). As shown in Fig. 4a and Fig. S22,† compared with C–H and C–Br, C–I exhibited a stronger ROS generation ability. Furthermore, to confirm the type of ROS generation, 9,10-anthracenedipropanoic acid (ABDA) was selected as the 1O2 indicator (Fig. 4b and Fig. S23†). As we thought, C–I exhibited good 1O2 generation ability under white light (400–800 nm, 60 mW cm−2) irradiation compared with C–H and C–Br. The relative 1O2 quantum yield (ΦPS) of C–H, C–Br and C–I was measured using rose bengal (RB) as the standard photosensitizer and was found to be 5.2%, 21.3% and 59%, respectively (the detailed calculation process and data are shown in Fig. S24 and Table S2†).48 Moreover, the type of ROS species was further determined using electron spin resonance (ESR) trapping measurements. 2,2,6,6-tetramethylpiperidine (TEMP) was added into the targeted systems (C–H, C–Br and C–I) to trap as the 1O2 trapping agents, respectively. As shown in Fig. 4c, C–I showed the strongest characteristic signal of 4-oxo-TEMPO (g = 2.0055, 1
:
1
:
1 triplet) under light irradiation which indicating the production of 1O2.
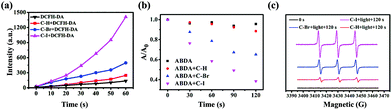 |
| Fig. 4 (a) Fluorescence intensity of DCFH-DA in the presence of C–H, C–Br and C–I at 525 nm after irradiation for 60 s (10 μM). (b) The relative absorption of ABDA in the presence of C–H, C–Br and C–I (10 μM) under light irradiation in PBS. (c) ESR signals of C–H, C–Br and C–I (10 mM) trapped by TEMP. | |
2.7 Two-photon photodynamic therapy
Encouraged by the high 1O2 production performance of C–Iin vitro, we subsequently evaluated the PDT efficacy of C–I in HeLa cells. As shown in Fig. 5a and Fig. S25,† the green fluorescence of SOSG (1O2 probe) was observed by CLSM in HeLa cells after exposure to the 880 nm laser. With the extension of the incubation time, the green fluorescence signal of SOSG became stronger and stronger, showing the considerable 1O2 generation ability in the cells under laser irradiation. In addition, Vitamin C (Vc), used as the specific scavenger for 1O2, was added into the above system. Obviously, Vc dramatically decreased SOSG oxidation in the C–I system, further demonstrating its light-induced 1O2 generation ability. Considering the two-photon activity of C–I, the NIR-induced cytotoxicity of C–I was studied by MTT and CLSM assays in HeLa cells. On treating cancer cells with C–I at a concentration of 20 μM, more than 80% of the cancer cells survived, indicating its low dark toxicity. In contrast, upon 880 nm laser irradiation, only about 20% of the cells survived (Fig. 5b). In addition, the live/dead cell co-staining of HeLa cells with calcein AM (green, live cell indicator) and PI (red, dead cell indicator) was performed to visualize the cell viability.49 As shown in Fig. 5c, a strong red fluorescence signal was observed after 880 nm light irradiation suggesting that C–I can induce HeLa cell apoptosis under 880 nm light irradiation.
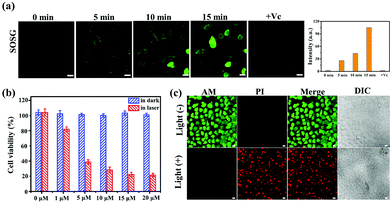 |
| Fig. 5 (a) SOSG was used as an indicator to detect 1O2 production in HeLa cells by the confocal imaging of C–I. The fluorescence intensity of SOSG at different illumination times (880 nm, 100 mW cm−2, 15 min). Scale bar: 20 μm. (b) Cell viability of HeLa cells after treatment with C–I at different concentrations for 2 h and irradiation with/without light (880 nm, 100 mW cm−2, 15 min). Cell viability was tested by an MTT assay. (c) Confocal fluorescence images of HeLa cells after treatment with C–I (10 μM) for 1 h and two-photon light irradiation (880 nm, 100 mW cm−2, 15 min), loading with calcein AM (3 μM, live cell marker, λex = 473 nm, λem = 490–590 nm) and propidium iodide (PI, 2 μM, dead cell marker, λex = 559 nm, λem = 575–675 nm). Scale bar: 20 μm. | |
The above experimental results prompted us to explore the apoptosis mechanism induced by C–I. To achieve the purpose, the tricaprylin solvent containing C–I (lipid droplet environment, oil phase) was mixed with the PBS solution containing a ROS indicator DCFH-DA (cytoplasmic environment, water phase). As shown in Fig. 6a, the oil–water phase interface was clear before light irradiation. As the illumination time was extended to 4 minutes, the fluorescence intensity of the water phase at 525 nm reached the maximum (Fig. 6b), indicating that ROS had transferred from the oil phase to the water phase. This phenomenon showed that the ROS in LDs could enter the cytoplasmic matrix.40,41 Therefore, the transfer of the reactive oxygen species from LDs to the cytoplasmic matrix may be related to cell apoptosis.
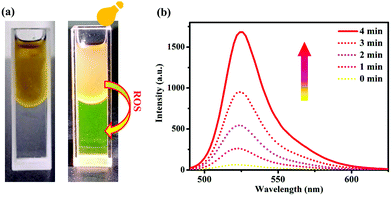 |
| Fig. 6 (a) Simulation experiment of the transfer of ROS from the lipid droplet environment to the cytoplasmic matrix environment. (b) PBS phase fluorescence intensity changes with the prolonged light time. | |
3. Conclusions
In summary, a series of two-photon active fluorescent probes (C–H, C–Br and C–I) based on carbazole units were designed and synthesized. The results showed that the iodine-modified carbazole derivative C–I exhibits an exciting LD targeting ability due to its excellent lipophilicity (log
P = 1.62). Notably, C–I was employed in STED and two-photon fluorescence imaging with high resolution for visualization of lipid droplets due to its large Stokes shift (∼120 nm) and two-photon absorption cross-section (362 GM). Moreover, benefiting from the heavy atom effect, C–I can effectively produce 1O2 (Φ = 0.59) and then lead to cancer cell apoptosis under NIR laser irradiation at a wavelength of 880 nm. In addition, the simulation of the transfer of reactive oxygen species from LDs to the cytoplasmic matrix in vitro provides a theoretical basis for the application of LD targeting probes in PDT research. Overall, this work provides a promising route for two-photon imaging of lipid droplets and switching on two-photon excited ROS generation.
Author contributions
Wenli Du: investigation, data curation, and writing – original draft. Xin Lu: investigation, software, formal analysis, and writing – original draft. Tong Yuan: investigation and methodology. Zhimin Sun: investigation and methodology. Xiaocheng Li: investigation and methodology. Xiaohe Tian: resources and formal analysis. Shengli Li: formal analysis, resources, and data curation. Qiong Zhang: resources and formal analysis. Dandan Li: conceptualization, methodology, and supervision. Yupeng Tian: conceptualization, methodology, writing – review & editing, and supervision.
Conflicts of interest
There are no conflicts to declare.
Acknowledgements
This work was supported by grants from the National Natural Science Foundation of China (21871003 and 22171001), the Natural Science Foundation of Anhui Province of China (2108085MB49 and 1908085MB30), and the Doctor Start-up Fund (S020118002/026 and S020118002/073).
Notes and references
- L. F. Guo, M. G. Tian, Z. Y. Zhang, Q. Lu, Z. Q. Liu, G. L. Niu and X. Q. Yu, J. Am. Chem. Soc., 2021, 143, 3169–3179 CrossRef CAS PubMed.
- J. R. Wang, N. Fang, J. Xiong, Y. J. Du, Y. Cao and W. K. Ji, Nat. Commun., 2021, 12, 1252 CrossRef CAS PubMed.
- D. I. Danylchuk, P. H. Jouard and A. S. Klymchenko, J. Am. Chem. Soc., 2021, 143, 912–924 CrossRef CAS PubMed.
- J. K. Zehmer, Y. Huang, G. Peng, J. Pu, R. G. Anderson and P. Liu, Proteomics, 2009, 9, 914–921 CrossRef CAS PubMed.
- J. M. Goodman, J. Biol. Chem., 2008, 283, 28005–28009 CrossRef CAS PubMed.
- R. Bartz, J. K. Zehmer and P. S. Liu, Prog. Biochem. Biophys., 2005, 32, 387–392 CAS.
- M. Mori, H. Itabe, Y. Higashi, Y. Fujimoto, M. Shiomi, M. Yoshizuimi, Y. Ouchi and T. Takano, J. Lipid Res., 2001, 42, 1771–1781 CrossRef CAS.
- C. Londos, D. L. Brasaemle, C. J. Schultz, D. C. Adler-Wailes, D. M. Levin, A. R. Kimmel and C. M. Rondinone, Ann. N. Y. Acad. Sci., 1999, 892, 155–168 CrossRef CAS PubMed.
- R. A. Igal and R. A. Coleman, J. Lipid Res., 1998, 39, 31–43 CrossRef CAS.
- X. Y. Pan, M. Wilson, C. McConville, T. N. Arvanitis, R. A. Kauppinen and A. C. Peet, Magn. Reson. Mater. Phys., Biol. Med., 2012, 25, 479–485 CrossRef CAS PubMed.
- C. Zhang, J. J. Li, L. Lan and J. X. Cheng, Anal. Chem., 2017, 89, 4502–4507 CrossRef CAS.
- Y. X. Ma, Q. H. Chen, X. Y. Pan and J. Zhang, Top. Curr. Chem., 2021, 379, 10 CrossRef CAS PubMed.
- J. Geng, K. Li, D. Ding, X. Zhang, W. Qin, J. Liu, B. Z. Tang and B. Liu, Small, 2012, 8, 3655–3663 CrossRef CAS.
- J. O. Escobedo, O. Rusin, S. Lim and R. M. Strongin, Curr. Opin. Chem. Biol., 2010, 14, 64–70 CrossRef CAS PubMed.
- Y. M. Yang, Q. Zhao, W. Feng and F. Y. Li, Chem. Rev., 2013, 113, 192–270 CrossRef CAS PubMed.
- Y. Shinohara, M. Suzuki and T. Fujimoto, Histochem. Cell Biol., 2010, 133, 477–480 CrossRef PubMed.
- P. Greenspan, E. P. Mayer and S. D. Fowler, J. Cell Biol., 1985, 100, 965–973 CrossRef CAS PubMed.
- J. Spandl, D. J. White, J. Peychl and C. Thiele, Traffic, 2009, 10, 1579–1584 CrossRef CAS PubMed.
- B. Qiu and M. C. Simon, Bio-Protoc., 2016, 6, 1–6 CAS.
- H. M. Zhong, S. G. Yu, B. Q. Li, K. G. He, D. Li, X. Wang and Y. X. Wu, Chem. Commun., 2021, 57, 6288 RSC.
- L. L. Wu, J. H. Liu, P. Li, B. Tang and T. D. James, Chem. Soc. Rev., 2021, 50, 702 RSC.
- N. N. Zhang, C. Y. Lu, M. J. Chen, X. L. Xu, G. F. Shu, Y. Z. Du and J. S. Ji, J. Nanobiotechnol., 2021, 19, 132 CrossRef.
- H. M. Zhong, Y. X. Wu, S. R. Yu, X. Wang, K. D. He, D. Li, Y. T. Cao and N. Gan, Anal. Chem., 2021, 93, 5691–5699 CrossRef CAS.
- D. Cheng, Y. Pan, L. Wang, Z. Zeng, L. Yuan and X. Zhang, J. Am. Chem. Soc., 2016, 139, 285–292 CrossRef.
- T. Ebina, Y. Masamizu, Y. R. Tanaka, A. Watakabe, R. Hirakawa and Y. Hirayama, Nat. Commun., 2018, 9, 1879 CrossRef PubMed.
- Y. N. Tu, W. K. Xia, X. Wu and L. Wang, Org. Biomol. Chem., 2021, 19, 6098 RSC.
- Y. L. Pak, S. J. Park, Q. Xu, H. M. Kim and J. Yoon, Anal. Chem., 2018, 90, 9510–9514 CrossRef CAS.
- L. Fan, X. D. Wang, Q. Zan, L. F. Fan, F. Li, Y. M. Yang, C. H. Zhang, S. M. Shuang and C. Dong, Anal. Chem., 2021, 93, 8019–8026 CrossRef CAS PubMed.
- X. J. Ma, Z. L. Zhi, S. Y. Zhang, C. Zhou, A. Mechler and P. S. Liu, IScience, 2021, 24, 102834 CrossRef PubMed.
- K. Colas, S. Doloczki, A. Kesidou, L. Sainero-Alcolado, A. Rodriguez-Garcia, M. Arsenian-Henriksson and C. Dyrager, ChemPhotoChem, 2021, 5, 632–643 CrossRef CAS.
- K. N. Wang, S. Y. Ma, Y. Y. Ma, Y. P. Zhao, M. M. Xing, L. Y. Zhou, D. X. Cao and W. Y. Lin, Anal. Chem., 2020, 92, 6631–6636 CrossRef CAS.
- Y. Y. Zhao, Y. Zhao, C. J. Yu, S. W. Liu, Y. Liu, E. Hao, L. J. Jiao, X. M. Xu, Z. Zhang and J. Z. Li, Dyes Pigm., 2021, 196, 109748 CrossRef CAS.
- H. Wang, K. Xue, P. Li, Y. Yang, Z. He and W. Zhang, Anal. Chem., 2019, 90, 6020–6027 CrossRef PubMed.
- Y. W. Jun, T. Wang, S. Hwang, D. Kim, D. Ma and K. H. Kim, Angew. Chem., Int. Ed., 2018, 57, 10142–10147 CrossRef CAS PubMed.
- Z. H. Feng, D. D. Li, M. Z. Zhang, Q. Zhang, S. L. Li, J. Y. Wu and Y. P. Tian, Chem. Sci., 2019, 10, 7228 RSC.
- Y. L. Bai, J. J. Zhao, S. L. Wang, T. R. Lin, F. G. Ye and S. L. Zhao, ACS Appl. Mater. Interfaces, 2021, 13, 35365–35375 CrossRef CAS PubMed.
- X. F. Wu, R. Wang, S. J. Qi, N. Kwon, J. J. Han, H. Kim, H. D. Li, F. B. Yu and J. Yoon, Angew. Chem., Int. Ed., 2021, 60, 15418–15425 CrossRef CAS.
- C. T. Prabhakara, S. A. Patil, S. S. Toragalmath, S. M. Kinnal and P. S. Badami, J. Photochem. Photobiol., B, 2016, 157, 1–14 CrossRef CAS PubMed.
- S. J. Zhang, Z. H. Yang, M. H. Li, Q. Zhang, X. H. Tian, D.
D. Li, S. L. Li, J. Y. Wu and Y. P. Tian, Analyst, 2020, 145, 7941 RSC.
- Y. Cai, P. P. Liang, Q. Y. Tang, X. Y. Yang, W. L. Si, W. Huang, Q. Zhang and X. C. Dong, ACS Nano, 2017, 11, 1054–1063 CrossRef CAS.
- Z. G. Sun, T. M. Liu, P. L. Guan, B. S. Yang and B. Liu, Dyes Pigm., 2021, 185, 108884 CrossRef CAS.
- F. Zhang, Y. M. Liu, B. S. Yang, G. M. Wen and B. Liu, Sens. Actuators, B, 2020, 322, 12858 CrossRef.
- T. Wang, Y. Hou, Y. Chen, K. Li, X. Cheng, Q. Zhou and X. Wang, Dalton Trans., 2015, 44, 12726–12734 RSC.
- L. Huang, Z. Li, Y. Zhao, Y. Zhang, S. Wu, J. Zhao and G. Han, J. Am. Chem. Soc., 2016, 138, 14586–14591 CrossRef CAS PubMed.
- C. Zhang, Y. Zhao, D. Li, J. Liu, H. Han, D. He, X. Tian, S. Li, J. Wu and Y. Tian, Chem. Commun., 2019, 55, 1450–1453 RSC.
- Z. An, C. Zheng, Y. Tao, R. Chen, H. Shi, T. Chen, Z. Wang, H. Li, R. Deng, X. Liu and W. Huang, Nat. Mater., 2015, 14, 685–690 CrossRef CAS PubMed.
- J. A. Li, J. Zhou, Z. Mao, Z. Xie, Z. Yang, B. Xu, C. Liu, X. Chen, D. Ren, H. Pan, G. Shi, Y. Zhang and Z. Chi, Angew. Chem., Int. Ed., 2018, 57, 6449–6453 CrossRef CAS PubMed.
- B. Ni, H. Z. Cao, C. K. Zhang, S. L. Li, D. D. Li, J. Y. Wu and Y. P. Tian, Inorg. Chem., 2020, 59, 13671–13678 CrossRef CAS.
- S. J. Qi, N. Kwon, Y. Yim, V. N. Nguyen and J. Yoon, Chem. Sci., 2020, 11, 6479 RSC.
Footnotes |
† Electronic supplementary information (ESI) available. See DOI: 10.1039/d1an01826d |
‡ These authors contributed equally to this work. |
|
This journal is © The Royal Society of Chemistry 2022 |