DOI:
10.1039/D2LC00552B
(Perspective)
Lab Chip, 2023,
23, 1410-1419
Recent advances in gold electrode fabrication for low-resource setting biosensing
Received
19th June 2022
, Accepted 28th August 2022
First published on 5th January 2023
Abstract
Gold electrodes are some of the most prevalent electrochemical biosensor substrate materials because they are readily functionalized with thiolated biomolecules. Yet, conventional methods to fabricate gold electrodes are costly and require onerous equipment, precluding them from implementation in low-resource settings (LRS). Recently, a number of alternative gold electrode fabrication methods have been developed to simplify and lower the cost of manufacturing. These methods include screen and inkjet printing as well as physical fabrication with common materials such as wire or gold leaf. All electrodes generated with these methods have successfully been functionalized with thiolated molecules, demonstrating their suitability for use in biosensors. Here, we detail recent advances in the fabrication, characterization and functionalization of these next-generation gold electrodes, with an emphasis on comparisons between cost and complexity with traditional cleanroom fabrication. We highlight gold leaf electrodes for their potential in LRS. This class of electrodes is anticipated to be broadly applicable beyond LRS due to their numerous inherent advantages.
Gold electrodes for biosensing purposes
Biosensors, which are devices that utilize a biorecognition element to detect an analyte of interest, are frequently used in point-of-care testing, as they enable rapid and accurate diagnoses with minimal equipment and personnel (Fig. 1).2 These attributes are crucial in low-resource settings (LRS) that lack the infrastructure required to perform laboratory-based tests (i.e. polymerase chain reaction, Western blotting, enzyme-linked immunosorbent assays).3 Electrochemical transduction is often used in combination with biosensors for point-of-care deployment because electrochemistry enables precise, sensitive, and quantitative readout with inexpensive, portable instrumentation.4 Gold is often employed as a substrate for such technologies because it is readily modified with thiolated molecules through the spontaneous formation of gold–thiol bonds.5 As thiols are readily incorporated into biomolecules ranging from proteins to nucleic acids, a wide range of biosensors can be made. For example, monolayers of thiolated DNA can be immobilized onto gold electrodes for detection of DNA,6 small molecules7 or enzymatic activity. Similarly, thiolated antibodies or antigens can be immobilized on gold in order to build immunosensors that detect specific biomarkers.8 Despite these advantages, the processing required for conventional gold electrode fabrication is costly and requires cumbersome, highly-specialized equipment, limiting the fabrication of such sensors to very few highly-specialized facilities.9
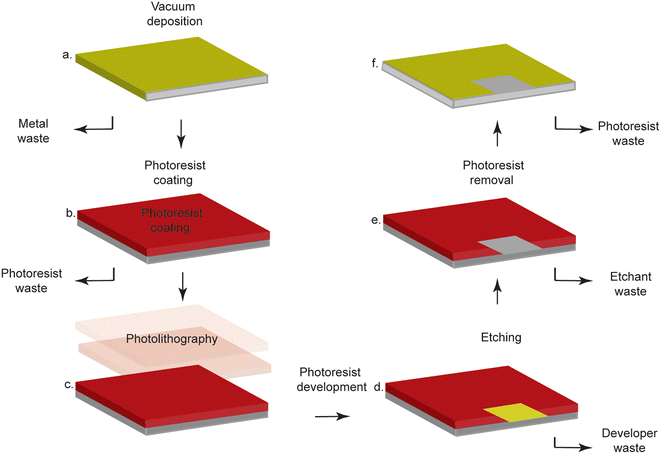 |
| Fig. 1 Typical subtractive photolithography methods involve laborious steps, specialized equipment, and expensive, dangerous chemicals. a. Gold is deposited on a substrate via vacuum deposition. b. The gold-coated surface is covered in photoresist. c. Photolithography is performed through a mask to selectively cure the photoresist. d. The photoresist is developed to selectively remove cured or uncured photoresist. e. The exposed gold is etched away. f. The photoresist is removed, and the substrate now contains a patterned gold coating. Figure reproduced and adapted from ref. 9 (Sui et al., 2020) with permission. Copyright Journal of the Electrochemical Society 2020. | |
Conventional gold electrode fabrication
Conventional gold electrode fabrication involves deposition to add gold to a substrate and lithography to pattern it. Most of these techniques require cleanroom facilities, limiting fabrication to a few specialized labs. Chemical vapor deposition (CVD)10 and physical vapor deposition (PVD)11 are used to deposit gold, resulting in thin films of pure metal on a substrate.11,12 During CVD, a chemical reaction between a metal-containing precursor and a gas results in the deposition of the metal on a substrate,12,13 while in PVD, the source metal is vaporized prior to deposition.14 To pattern the gold, lithographic techniques are incorporated, including shadowmask15 and photolithography.16 In shadowmask lithography, a physical mask is placed in contact with the substrate, and PVD or CVD is performed.15 Only the exposed regions of the substrate are coated with gold. These methods are costly and not conducive to high-throughput prototyping.15 Photolithography, in contrast, generates micron or submicron features using photoresist, a set of chemicals whose properties change after light exposure.16 Photolithography enables high resolution patterning of fine features, but it is costly and laborious (Fig. 1). Lastly, dry and wet etching processes can be used.17 The most common form of dry etching is reactive ion etching (REI), during which reactive plasma etches away the exposed gold, but this method is difficult because the products of the reaction between gold and the etchant are largely non-volatile.17 Wet etching exposes the gold to an etching solution that contains a ligand that forms soluble Au(I) complexes and an oxidant that generates Au(II).17 While wet etching is more cost effective than dry etching, it is only capable of precision for features larger than 5 μm.
A plethora of electrodes made with conventional fabrication techniques have been used to generate biosensors that detect DNA, proteins and molecules. Cheng-Chi Chou et al. sputtered a thin film of gold on a silicon substrate.18 This film was then treated with 6-hexane dithiol to support a monolayer of AuNPs, which were functionalized with thiolated single-stranded DNA (ssDNA). These electrodes were used to detect genetically modified soybeans from real samples with a limit of detection (LOD) of 1.792 ng mL−1 and a linear range of 17.9–19.2 ng mL−1. The same technique was used by Wang et al. to create a monolayer of AuNPs on a sputtered thin film of gold. These NPs were immobilized with antibodies used to detect neutrophil gelatinase-associated lipocalin (NGAL) in urine samples. The sensor had an LOD of 0.47 ng mL−1 and a linear range of 1–100 ng mL−1, which were clinically relevant levels that could be used to detect kidney injury.1 These examples shed light on the versatility of gold substrates for biosensing purposes, as they can be functionalized with a wide range of biomolecules.19
Despite their use in biosensor fabrication, electrodes fabricated using conventional cleanroom methods suffer from high capital costs and are inaccessible in most LRS; the specialized laboratory space required for cleanroom fabrication can cost up to USD $1 million.20,21 This number does not include instrumentation or the cost of the gold itself (which can require an initial investment of up to $2000).22 Thus, significant costs are incurred during the time-consuming processes involved in cleanroom fabrication.
Screen printed gold electrodes
Gold screen-printed electrodes (SPEs) are a more affordable alternative to cleanroom-fabricated platforms. Screen printing involves the application of the appropriate metal ink, through a mask, on a substrate (Fig. 2). The shape of the mask determines the shape of the electrode. Screen printing is favorable because it can be performed on a diverse array of substrate materials, including plastic, polymers,23 ceramic and paper, while allowing for versatility in electrode design.24–28 Screen printed electrodes are highly reproducible and readily mass-produced.24
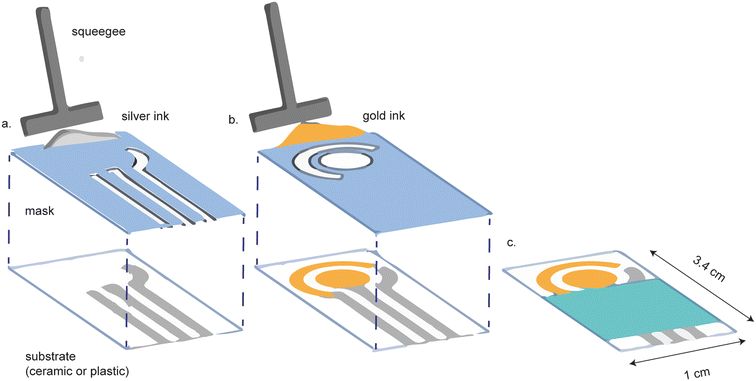 |
| Fig. 2 Screen printed electrodes are made by screen printing conductive ink onto a substrate. (a) A mask is first used to screen print the reference and contact electrodes. (b) A separate mask is used to screen print the gold working and counter electrodes. (c) The final device is assembled with a hydrophobic barrier covering the contact electrodes. Figure reproduced and modified from ref. 28 (Ozkan et al., 2015) with permission. Copyright Springer Berlin Heidelberg 2015. | |
SPE surfaces can be functionalized to make immuno, genetic, and enzymatic sensors, demonstrating their versatility and suitability for biosensor construction.29 Furthermore, the surface morphology and roughness of the electrode can be altered by varying the gold ink used. This ability to generate multiple surface topologies broadens the applicability of the SPEs.30,31 For example, the most commonly used commercial SPEs are made by Dropsens and use either high temperature curing inks (AT) or low temperature curing inks (BT). The AT electrodes are suitable for assays where steric hindrance is not concern. For example, Dizaji et al., used the DropSens AT electrodes to detect whole-cell antibiotic-susceptible bacteria.32 They immobilized thiolated vancomycin molecules on the SPEs and monitored the binding of the vancomycin-susceptible bacteria S. aureus. The LOD of the sensor was 34 CFU mL−1 and the linear range was 10–108 CFU mL−1. The low temperature curing inks have a rougher surface morphology, which allows for more sensitive biosensors due to decreased steric hindrance effects.33 Bialobrzeska et al., used the Dropsens BT electrodes to detect the cancer antigen AGR2 in lysed cell samples with a LOD of 0.093 fg mL−1 and a linear range of 0.001 fg mL−1 to 0.900 fg mL−1.34 These examples shed light on the potential of SPEs to be used in biosensor fabrication.
Despite these advantages, mass production of SPEs require an initial investment of at least $30
000,35 which remains inaccessible to labs with limited funding. Gold ink itself is also expensive,36 which can cost over $1000 per oz (Table 1). Furthermore, electrodes must be cured at elevated temperatures (130–800 °C)23 prior to use, necessitating additional equipment that is not available outside of select labs. An additional disadvantage of screen printing is that this technology does not reproducibly produce micrometer-scale electrodes, which may be necessary to achieve improved signal/noise ratios and faster mass-transport rates.24 Furthermore, the inks can contain impurities that interfere with sensor performance.37
Table 1 Capital costs for various types of gold electrode fabrication
Electrode type |
Capital equipment |
Capital cost |
References |
Cleanroom fabricated |
Bare cleanroom rental |
$200–400 per sq ft |
98
|
Cleanroom ratesheet |
$30–350 per hour |
99
|
E-beam lithographer |
$100 000–1 000 000 |
20
|
Gold target |
$600–2000 |
22
|
Sputterer |
$50 000–1 000 000 |
21
|
Inkjet printed |
Dimatix materials printer |
$40 000 |
54
|
Gold ink |
$1000 per oz |
58
|
Screen printed |
Screen printer |
$30000–80 000 |
35
|
Gold ink |
$1100 per oz |
58
|
Micro wire |
Gold 25 μM microwire electrode |
$270 per 100 ft |
100
|
White gold leaf |
White gold leaf |
$32.31 per 25 (14 cm × 14 cm) sheets |
86
|
Gold leaf |
Gold leaf |
$51.87 per 25 (14 cm × 14 cm) sheets |
86
|
Inkjet-printed gold electrodes
Inkjet printing of gold electrodes is a contact-less, mask-less procedure (Fig. 3).38–42 Compared to screen-printing, inkjet printing offers more control over gold ink deposition43 and micron-resolution features.44 A wide variety of materials have been used as substrates for inkjet-printed gold electrodes, including paper,45,46 polyethylene teraphalate (PET),47 kapton film,48 cyclein olefin copolymer,40 and cellulose,49 making them favorable for flexible electronics. Depending on the fabrication method, some inkjet-printed gold electrodes exhibit as high as tenfold improved sensitivity as compared to sputtered gold, as measured by differential pulse voltammetry (DPV).47
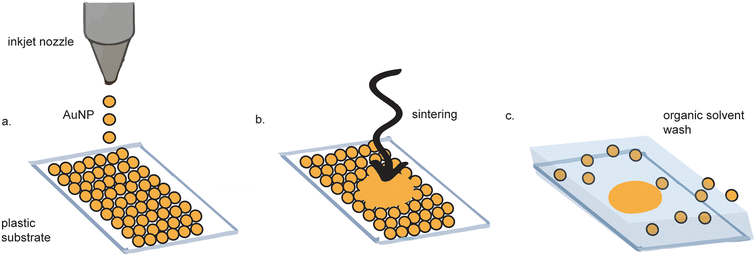 |
| Fig. 3 Inkjet printed electrode fabrication. (a) Gold nanoparticles (AuNPs) are inkjet printed onto a substrate. (b) The AuNPs are sintered to form a continuous surface. (c) Excess AuNPs are washed away. Figure adapted from ref. 62 (Ko et al., 2007). | |
Inkjet-printed gold electrodes have been used to detect proteins,39,46,47 protein–nucleic acid interactions,50 DNA hybridization,40 antioxidants,51 glucose45 and bacteria,52 demonstrating their broad utility in biosensor fabrication. Kant et al., 2021 made inkjet-printed gold electrodes on paper that detected glucose from serum without any enzymes.53 They achieved a LOD of 10 μM and a linear range of 0.05–35 mM. This sensor is critical because it demonstrates a proof-of-concept for low-cost glucose sensors that, once fabricated, can be easily deployed in LRS, especially because enzyme stability is not a concern. In another study, Sui et al., 2019 developed a novel class of nanoparticle inks that are sintered with plasma rather than high temperatures.47 This allowed for the development of a biosensor for amyloid beta, a critical marker for Alzheimer's disease, on a PET substrate. While limits of detection were not reported for this sensor, the sensor demonstrated that gold electrodes can be inkjet printed on a flexible PET substrate, which is not possible when using traditional AuNP inks that require sintering at high temperatures above the glass transition temperature of PET, thereby extending the number of substrates that can be used for inkjet printing gold.
Despite the recent advances in inkjet printing, similarly to SPEs, the costly fabrication of inkjet-printed electrodes limits their production to well-funded labs. While commercial inkjet printers can be repurposed to print metallic inks, the FUJIFILM Dimatix Materials Printer ($40
000)54 offers greater control over the printing process43 and is the most common printer for inkjet printing gold.40,43,46,48,52,55–57 Gold ink is similarly costly, costing around $1100 per oz, which fluctuates with commodities markets.58 Another disadvantage of inkjet-printed electrodes is that they require sintering prior to use to transform the ink into uniform, conductive electrodes. Gold nanoparticle (AuNP) inks are the are most commonly used. Such inks are stabilized with capping agents that prevent excessive clustering of the NPs and improve their wettability, jettability and adhesion.47,59 Yet, the capping agents act as insulators and must be removed to generate a conductive surface. This removal is most commonly performed via thermal sintering (∼130–440 °C) but can also be done with photonic curing,40 chemical,60 plasma,47,50 laser61,62 or IR45,46 sintering for substrates with lower glass transition temperatures.59 These processes all necessitate complex equipment that is not available in LRS. Recently, a group developed an alternative ink comprised of inorganic salts that could be activated via plasma at low temperatures (<130 °C).47,50 Despite the improvements offered by the ink, plasma activation requires a specialized etcher and an oven, which remain difficult to procure in many cases.
Gold wire electrodes
For certain applications, the small size of micro- and nano-wire electrodes offers benefits that are not achieved using their milli-scale counterparts, including decreased capacitances, greater mass transfer rates, reduced overall current, and lower ohmic drop.63,64 The retail cost of 25 μM 99.9% gold wires is $270 per 100 ft, which is significantly more affordable than cleanroom fabrication, screen-printing, or inkjet printing. Gold microwires63,65–68 and nanowires.64,69 have been incorporated into paper analytical devices (PADs) for use at the point-of-care. In static paperfluidic devices, microwires can attain quasi-steady state current responses, which is beneficial for detecting freely-diffusing analytes.63 Channon et al., 2018 incorporated gold microwires into a paperfluidic device to detect West Nile viral particles.67 The Au microwires were functionalized with antibodies that captured the virus particles; the LOD was 10.2 particles per 50 μL media. A similar strategy was used by (Wang et al., 2019) to functionalize a gold microwire with antigens to monitor pathogen-specific antibody responses in serum, with a low LOD of 10 molecules per 30 μL of solution.68 These devices cost ~$1 per test, demonstrating that the wires can be used to make affordable diagnostics for infectious diseases in LRS. However, microwires often require functionalization prior to device incorporation,63,70 rendering them incompatible with high-throughput manufacturing. Furthermore, the microwires are very fragile and difficult to assemble into devices, limiting their use.
Nanoporous gold electrodes
Nanoporous gold electrodes are more sensitive than planar gold electrodes due to their high surface area.71,72 One study found that the linear range of detection for DNA was 500 pM–200 μM using nanoporous gold electrodes and 1–10 μM using planar gold electrodes.73,74 Nanoporous gold electrodes also exhibit anti-biofouling properties because they act as a mesh barrier against electrode fouling agents while allowing smaller analytes of interest reach the sensor.74–79 Nanoporous gold electrodes have been used to make immuno, enzymatic, small molecule, and DNA sensors, demonstrating their broad applicability in biosensor development.80
There are many methods to fabricate nanoporous gold electrodes, including electrochemical etching,71,81 electrodeposition,71,81 dealloying an alloy of gold and a non-noble metal,71,81 dynamic hydrogen bubble templating,81 and metal-induced crystallization.82 The least expensive and most straightforward method is to de-alloy white gold leaf, which can be purchased for $32.31 per 25 (14 cm × 14 cm) sheets. The starting gold material is doped with silver that is etched away to produce nanopores in the gold. Recently, Hondred et al. made an integrated, three-electrode device using white gold leaf (Fig. 4).83 White gold leaf was applied to a polyamide film and patterned using maskless lithography. The electrodes were then functionalized with the enzyme acetylcholinesterase and used to detect organophosphates in soil samples with a LOD of 0.53 pM and a linear range of 1 nM–10 μM. The high surface area of the nanoporous gold electrodes lends itself to high sensitivity for biosensors, which is a favorable characteristic. While the lithography method described in this paper was much simpler and more cost-effective than traditional techniques, their process still necessitated equipment and etchant chemicals that are not suitable for use or storage in LRS. Typically, dealloying to make nanoporous gold involves dissolution of the gold alloy in concentrated nitric acid. Hondred et al. were able to de-alloy in dilute nitric acid (35%), which enabled the dealloying process to occur on substrates that would otherwise be corroded by concentrated nitric acid. Nevertheless, their process still necessitates nitric acid, which requires controlled storage and disposal, incompatible with facilities in LRS.
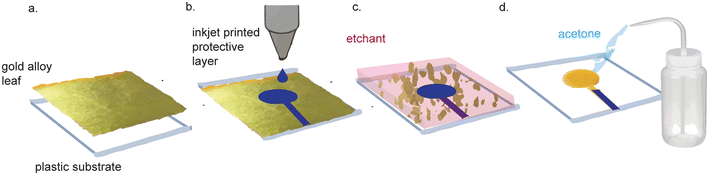 |
| Fig. 4 Nanoporous gold electrode fabrication with gold alloy leaf. (a) Gold alloy leaf and silver leaf is adhered to a substrate. (b) A protective layer is inkjet printed on the gold leaf. (c) Unprotected leaf is etched away. (d) Protective layer is washed with acetone to reveal the leaf electrodes that will be dealloyed in nitric acid. Figure adapted from ref. 83 (Hondred et al., 2020) with permission. Copyright Royal Society of Chemistry 2020. | |
Electrodeposition of gold electrodes
While electrodeposition of gold electrodes is an important technique that is extensively reviewed.84 It is beyond the scope of this review because it needs to be paired with other techniques such as cleanroom fabrication techniques (photolithography, sputtering, evaporation, etching), or screen printing to develop integrated-three electrode systems suitable for POC use. Furthermore, the solutions used during the electrodeposition process are toxic unstable, rendering them incompatible with LRS.85
Pure gold leaf electrodes
Pure gold leaf sheets are extremely thin, affordable sheets of gold that cost $51.87 per 25 (14 cm × 14 cm) sheets.86 Pure gold leaf has been used to make electrodes,87–95 using equipment-free fabrication (Fig. 5). These electrodes were made by sandwiching the gold leaf between adhesive, laser-patterned polyamide film.92,96 The exposed gold leaf served as a working electrode that required pairing with an external counter and reference electrode for use. These fabrication methods did not require specialized equipment or harsh chemicals, making them suitable for use in standard labs and in LRS. However, these devices were not functionalized for biosensing purposes. Prasertying et al. fabricated an integrated three-electrode device comprised of a PVC backing, a gold leaf working electrode, a screen-printed carbon counter electrode and a screen-printed silver reference electrode. While their fabrication method could be carried out in LRS, they did not demonstrate the suitability of their electrodes for biosensing purposes and their electrodes were inflexible, making them unsuitable for wearable electronics.
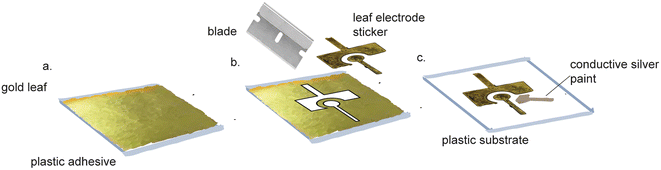 |
| Fig. 5 Pure gold leaf electrode fabrication does not require specialized equipment. (a) Gold leaf adhesive is applied to a side of Fellowes. (b) Gold leaf is applied to the adhesive and cut. (c) The gold leaf stickers are peeled off and placed on a transparency film. Conductive silver paint is applied to the leads of all three electrodes. Figure reproduced and adapted from ref. 95 (Zamani et al., 2020) with permission. Copyright American Chemical Society 2021. | |
We recently reported an integrated, flexible, three-electrode system containing a gold leaf working electrode, a gold leaf counter electrode, and a silver paint pseudoreference electrode on a transparency film backing. Our devices only required adhesives, stencils and gold leaf to assemble. Briefly, gold leaf adhesive is applied to the plastic side of Fellowes adhesive sheets. Gold leaf is then applied to the gold leaf adhesive and cut. The gold leaf stickers are removed and added to a transparency film. A silver pseudoreference electrode is then painted on using conductive silver paint. The final device is assembled after adding well stickers and aluminum foil contacts to the electrode leads. The fabrication does not require any specialized equipment or harsh chemicals. Not only does each device cost only $0.50 to make, nearly an order of magnitude less costly than commercially-available gold SPEs, they outperform these SPEs in an assay developed to detect DNase activity.33 Electrodes were further functionalized with methylene – blue tagged oligonucleotides that acted as reporter molecules for target-activated CRISPR Cas12a enzymatic activity. This system was used to detect human papillomavirus (HPV) from clinical samples with an LOD of 104 copies, demonstrating the potential for inexpensive, gold leaf-based diagnostic sensors. To reiterate, we fabricated gold leaf electrodes that cost $0.50 each without specialized equipment and used them to accurately detect pathogenic DNA from clinical samples.
Concluding remarks and future perspectives
Generally speaking, gold electrodes with higher surface areas exhibit higher sensitivity due to decreased steric hindrance affects and greater electron transfer. For a given electrode fabrication process, parameters can be tuned to yield varying surface morphologies. Therefore, the sensitivity of a given biosensor is determined by the specific parameters used during the electrode fabrication process; as a result, one method of gold electrode fabrication is not going to be more sensitive than another method, for a range of sensitivities are created by a given fabrication method depending on the specific parameters used. For example, during conventional fabrication, electrochemical treatment of thin layers of gold with HAuCl4 and HCl results in nanostructured electrodes that increase the sensitivity of conventionally-sputtered gold by nearly an order of magnitude. The type of inks used in screen printing affect surface morphology and sensor performance. It has been shown that the low temperature curing inks significantly improve the biosensing performance compared to high temperature curing inks. Similarly, the morphology of inkjet-printed electrodes depends on the type of ink used, printing speed, and annealing temperature. Inkjet printing of Au electrodes using particle-free inks made from inorganic metal salts with plasma annealing at low-temperatures results in electrode surfaces with extremely high surface areas; one study found that these electrodes were used to detect amyloid-B42 with 10× higher sensitivity than conventionally sputtered gold electrodes. The pores in nanoporous electrodes and the surface roughness of gold leaf electrodes lend themselves to highly sensitive biosensing capabilities due to the increased surface area of the electrodes. The leaf electrodes have a rough surface morphology that results in a higher surface area and reduced packing of functionalized biomolecules, thereby reducing steric hindrance and increasing biosensor sensitivity.
Gold leaf as a promising material for low-cost biosensor development
In addition to their sensitivity, gold leaf electrodes have a capital cost that is orders of magnitude less than that of electrodes fabricated using other methods. Although white gold leaf is less expensive than pure gold leaf, this material requires dealloying with harsh chemicals such as nitric acid to yield pure gold electrodes.83,97 Pure gold leaf does not necessitate the use of such harsh chemicals, making their fabrication better-suited for LRS and in non-laboratory space. Preliminary demonstrations of biomolecule modification have been shown with nucleic acids to detect endonuclease activity, and we propose that gold leaf should be further explored as a superior substrate for biosensors. The gold leaf is low-cost and fabrication does not require specialized laboratory equipment, making it well-suited for use in LRS as well as for rapid prototyping in standard labs. The accessibility of these gold leaf electrodes represents a major step towards achieving healthcare equity across the globe.
Author contributions
The manuscript was written through contributions of all authors.
Conflicts of interest
There are no conflicts to declare.
Acknowledgements
We would like to acknowledge the MIT Research Support Committee for supporting this work. M. Z. was supported through a training grant in environmental toxicology from the National Institute of Environmental Health Sciences (NIEHS) from the National Institutes of Health (NIH), Grant # T32-ES007020.
References
- W. J. Wang, M. C. Chou, Y. J. Lee, W. L. Hsu and G. J. Wang, A simple electrochemical immunosensor based on a gold nanoparticle monolayer electrode for neutrophil gelatinase-associated lipocalin detection, Talanta, 2022, 246, 123530, DOI:10.1016/J.TALANTA.2022.123530
.
- A. Kaushik and M. A. Mujawar, Point of care sensing devices: Better care for everyone, Sensors, 2018, 18(12), 4303, DOI:10.3390/s18124303
.
- S. Sharma, J. Zapatero-Rodríguez, P. Estrela and R. O'Kennedy, Point-of-Care diagnostics in low resource settings: Present status and future role of microfluidics, Biosensors, 2015, 5(3), 577–601, DOI:10.3390/bios5030577
.
- W. Zhang, R. Wang, F. Luo, P. Wang and Z. Lin, Miniaturized electrochemical sensors and their point-of-care applications, Chin. Chem. Lett., 2020, 31(3), 589–600, DOI:10.1016/j.cclet.2019.09.022
.
- T. Wink, S. J. Van Zuilen, A. Bult and W. P. Van Bennekom, Tutorial Review Self-Assembled Monolayers for Biosensors, Analyst, 1997, 122, 43R–50R, 10.1039/A606964I
.
- M. Trotter, D. Juric and Z. Bagherian,
et al., Inkjet-Printing of Nanoparticle Gold and Silver Ink on Cyclic Olefin Copolymer for DNA-Sensing Applications, Sensors, 2020, 20(5), 1333, DOI:10.3390/s20051333
.
- F. V. Oberhaus, D. Frense and D. Beckmann, Immobilization techniques for aptamers on gold electrodes for the electrochemical detection of proteins: A review, Biosensors, 2020, 10(5), 45, DOI:10.3390/BIOS10050045
.
- P. Ihalainen, H. Majumdar and T. Viitala,
et al., Application of Paper-Supported Printed Gold Electrodes for Impedimetric Immunosensor Development, Biosensors, 2012, 3(1), 1–17, DOI:10.3390/bios3010001
.
- Y. Sui and C. A. Zorman, Review — Inkjet Printing of Metal Structures for Electrochemical Sensor Applications: A Review, J. Electrochem. Soc., 2020, 167, 037571, DOI:10.1149/1945-7111/ab721f
.
- C. E. Larson, T. H. Baum and R. L. Jackson, Chemical Vapor Deposition of Gold, MRS Online Proc. Libr., 1986, 75, 721–724, DOI:10.1557/proc-75-721
.
-
D. L. Chambers and C. T. Wan, Physical Vapor Deposition of Gold and its Alloys, in Precious Metals 1981, Elsevier, 1982, pp. 219–238, DOI:10.1016/b978-0-08-025392-3.50030-2
.
-
The chemistry of metal CVD, ed. T. Kodas and M. Hampden-Smith, VCH, Weinheim; New York; Basel; Cambridge; Tokyo; 1994, ISBN: 3-527-29071-0 Search PubMed
.
-
A. Behera, P. Mallick and S. S. Mohapatra, Nanocoatings for anticorrosion, in Corrosion Protection at the Nanoscale, Elsevier, 2020, pp. 227–243, DOI:10.1016/b978-0-12-819359-4.00013-1
.
-
D. L. Chambers and C. T. Wan, Physical Vapor Deposition of Gold and its Alloys, in Precious Metals 1981, Elsevier, 1982, pp. 219–238, DOI:10.1016/b978-0-08-025392-3.50030-2
.
- F. E. Nik, I. Matthiesen, A. Herland and T. E. Winkler, Low-Cost PVD shadow masks with submillimeter resolution from laser-cut paper, Micromachines, 2020, 11(7), E676, DOI:10.3390/mi11070676
.
-
C. K. Dixit and A. Kaushik, Microfluidics for Biologists, Fundamentals and Applications, 2016, DOI:10.1007/978-3-319-40036-5
.
- T. A. Green, Gold etching for microfabrication, Gold Bull., 2014, 47(3), 205–216, DOI:10.1007/s13404-014-0143-z
.
- C. C. Chou, Y. T. Lin, I. Kuznetsova and G. J. Wang, Genetically Modified Soybean Detection Using a Biosensor Electrode with a Self-Assembled Monolayer of Gold Nanoparticles, Biosensors, 2022, 12(4), 207, DOI:10.3390/BIOS12040207
.
- J. Yoon, S. N. Lee and M. K. Shin,
et al., Flexible electrochemical glucose biosensor based on GOx/gold/MoS2/gold nanofilm on the polymer electrode, Biosens. Bioelectron., 2019, 140, 111343, DOI:10.1016/J.BIOS.2019.111343
.
-
J. G. McConville, 2019 Pharmaceutical/biological facility cleanroom cost data benchmarks Compass International, 2022, https://compassinternational.net/2019-pharmaceutical-biological-facility-cleanroom-cost-data-benchmarks/ Search PubMed.
- Magnetron Sputtering Systems R&D|Small Batch, Accessed February 27, 2021, https://www.ajaint.com/sputtering-systems.html.
- Disk or Annular Sputter Targets, Accessed February 27, 2021, https://www.tedpella.com/cressington_html/Cressington-Targets.htm.
- R. García-González, M. T. Fernández-Abedul, A. Pernía and A. Costa-García, Electrochemical characterization of different screen-printed gold electrodes, Electrochim. Acta, 2008, 53(8), 3242–3249, DOI:10.1016/j.electacta.2007.07.059
.
- R. A. S. Couto, J. L. F. C. Lima and M. B. Quinaz, Recent developments, characteristics and potential applications of screen-printed electrodes in pharmaceutical and biological analysis, Talanta, 2016, 146(February), 801–814, DOI:10.1016/j.talanta.2015.06.011
.
- N. B. Mincu, V. Lazar, D. Stan, C. M. Mihailescu, R. Iosub and A. L. Mateescu, Screen-Printed Electrodes (SPE) for in vitro diagnostic purpose, Diagnostics, 2020, 10(8), 1–21, DOI:10.3390/diagnostics10080517
.
- E. Costa-Rama and M. T. Fernandez-Abedul, Paper-based screen printed electrodes: a new generation of low-cost electroanalytical platforms, Biosensors, 2021, 11(2), 51, DOI:10.3390/bios11020051
.
- A. García-Miranda Ferrari, S. J. Rowley-Neale and C. E. Banks, Screen-printed electrodes: Transitioning the laboratory in-to-the field, Talanta Open, 2021, 3, 100032, DOI:10.1016/j.talo.2021.100032
.
-
S. A. Ozkan, J. M. Kauffmann and P. Zuman, Screen-Printed Electrodes (SPE) for Drug Compounds Determination, Springer, Berlin, Heidelberg, 2015, pp. 119–140, DOI:10.1007/978-3-662-47138-8_5
.
- K. Yamanaka, M. C. Vestergaard and E. Tamiya, Printable electrochemical biosensors: A focus on screen-printed electrodes and their application, Sensors, 2016, 16(10), 1761, DOI:10.3390/s16101761
.
- Metrohm Dropsens Disposable Electrodes, Accessed April 11, 2020, https://www.dropsens.com/pdfs_productos/gold_spes.pdf.
- E. Bernalte, C. Marín-Sánchez, E. Pinilla-Gil and C. M. A. Brett, Characterisation of screen-printed gold and gold nanoparticle-modified carbon sensors by electrochemical impedance spectroscopy, J. Electroanal. Chem., 2013, 709, 70–76, DOI:10.1016/j.jelechem.2013.09.007
.
- A. Norouz Dizaji, Z. Ali, H. Ghorbanpoor, Y. Akcakoca, H. Avci and F. D. Guzel, Electrochemical-based ‘‘antibiotsensor’’ for the whole-cell detection of the vancomycin-susceptible bacteria, Talanta, 2021, 234, 122695, DOI:10.1016/j.talanta.2021.122695
.
- M. Zamani, V. Yang, L. Maziashvili, G. Fan, C. M. Klapperich and A. L. Furst, Surface Requirements for Optimal Biosensing with Disposable Gold Electrodes, ACS Meas. Sci. Au, 2021, 2(2), 91–95, DOI:10.1021/acsmeasuresciau.1c00042
.
- W. Białobrzeska, K. Dziąbowska, M. Lisowska, M. A. Mohtar, P. Muller, B. Vojtesek, R. Krejcir, R. O'Neill, T. R. Hupp, N. Malinowska, E. Bięga, D. Bigus, Z. Cebula, K. Pala, E. Czaczyk, S. Żołędowska and D. Nidzworski, An Ultrasensitive Biosensor for Detection of Femtogram Levels of the Cancer Antigen AGR2 Using Monoclonal Antibody Modified Screen-Printed Gold Electrodes, Biosensors, 2021, 11(6), 184, DOI:10.3390/BIOS11060184
.
- Buying an Automatic Screen Printing Press? What to Know, What You Really Need, and How Much It Cost - Printavo, Accessed February 27, 2021, https://www.printavo.com/blog/from-manual-to-automatic-screen-printing.
- Z. Taleat, A. Khoshroo and M. Mazloum-Ardakani, Screen-printed electrodes for biosensing: A review (2008-2013), Microchim. Acta, 2014, 181(9–10), 865–891, DOI:10.1007/s00604-014-1181-1
.
- E. Bernalte, C. Marín-Sánchez, E. Pinilla-Gil and C. M. A. Brett, Characterisation of screen-printed gold and gold nanoparticle-modified carbon sensors by electrochemical impedance spectroscopy, J. Electroanal. Chem., 2013, 709, 70–76, DOI:10.1016/j.jelechem.2013.09.007
.
- Y. Sui and C. A. Zorman, Review—Inkjet Printing of Metal Structures for Electrochemical Sensor Applications, J. Electrochem. Soc., 2020, 167(3), 037571, DOI:10.1149/1945-7111/ab721f
.
- G. C. Jensen, C. E. Krause, G. A. Sotzing and J. F. Rusling, Inkjet-printed gold nanoparticle electrochemical arrays on plastic. Application to immunodetection of a cancer biomarker protein, Phys. Chem. Chem. Phys., 2011, 13(11), 4888–4894, 10.1039/c0cp01755h
.
- M. Trotter, D. Juric and Z. Bagherian,
et al., Inkjet-printing of nanoparticle gold and silver ink on cyclic olefin copolymer for DNA-sensing applications, Sensors, 2020, 20(5), 1–15, DOI:10.3390/s20051333
.
- Y. Khan, F. J. Pavinatto and M. C. Lin,
et al., Inkjet-Printed Flexible Gold Electrode Arrays for Bioelectronic Interfaces, Adv. Funct. Mater., 2016, 26(7), 1004–1013, DOI:10.1002/adfm.201503316
.
- F. J. Pavinatto, Biosensors with printed active layer, Display and Imaging, 2015, 2, 69–91 Search PubMed
.
- R. Tortorich, H. Shamkhalichenar and J. W. Choi, Inkjet-Printed and Paper-Based Electrochemical Sensors, Appl. Sci., 2018, 8(2), 288, DOI:10.3390/app8020288
.
- P. M. Grubb, H. Subbaraman, S. Park, D. Akinwande and R. T. Chen, Inkjet printing of high performance transistors with micron order chemically set gaps, Sci. Rep., 2017, 7(1), 1–8, DOI:10.1038/s41598-017-01391-2
.
- A. Määttänen, U. Vanamo and P. Ihalainen,
et al., A low-cost paper-based inkjet-printed platform for electrochemical analyses, Sens. Actuators, B, 2013, 177, 153–162, DOI:10.1016/j.snb.2012.10.113
.
- P. Ihalainen, H. Majumdar and T. Viitala,
et al., Application of paper-supported printed gold electrodes for impedimetric immunosensor development, Biosensors, 2013, 3(1), 1–17, DOI:10.3390/bios3010001
.
- Y. Sui, Y. Dai, C. C. Liu, R. M. Sankaran and C. A. Zorman, A New Class of Low-Temperature Plasma-Activated, Inorganic Salt-Based Particle-Free Inks for Inkjet Printing Metals, Adv. Mater. Technol., 2019, 4(8), 1900119, DOI:10.1002/admt.201900119
.
- G. C. Jensen, C. E. Krause, G. A. Sotzing and J. F. Rusling, Inkjet-printed gold nanoparticle electrochemical arrays on plastic. Application to immunodetection of a cancer biomarker protein, Phys. Chem. Chem. Phys., 2011, 13(11), 4888–4894, 10.1039/c0cp01755h
.
- C. Hu, X. Bai, Y. Wang, W. Jin, X. Zhang and S. Hu, Inkjet Printing of Nanoporous Gold Electrode Arrays on Cellulose Membranes for High-Sensitive Paper-Like Electrochemical Oxygen Sensors Using Ionic Liquid Electrolytes, Anal. Chem., 2012, 84, 36, DOI:10.1021/ac3003243
.
- Y. Dai, L. Y. Chiu and Y. Sui,
et al., Nanoparticle based simple electrochemical biosensor platform for profiling of protein-nucleic acid interactions, Talanta, 2019, 195, 46–54, DOI:10.1016/j.talanta.2018.11.021
.
- F. J. Pavinatto, C. W. A. Paschoal and A. C. Arias, Printed and flexible biosensor for antioxidants using interdigitated ink-jetted electrodes and gravure-deposited active layer, Biosens. Bioelectron., 2015, 67, 553–559, DOI:10.1016/j.bios.2014.09.039
.
-
S. Demuru, A. Marette, W. Kooli, P. Junier and D. Briand, Flexible Organic Electrochemical Transistor with Functionalized Inkjet-Printed Gold Gate for Bacteria Sensing, 2019 20th International Conference on Solid-State Sensors, Actuators and Microsystems and Eurosensors XXXIII, TRANSDUCERS 2019 and EUROSENSORS XXXIII, 2019, pp. 2519–2522, DOI:10.1109/TRANSDUCERS.2019.8808309
.
- T. Kant, K. Shrivas, K. Tapadia, R. Devi, V. Ganesan and M. K. Deb, Inkjet-printed paper-based electrochemical sensor with gold nano-ink for detection of glucose in blood serum, New J. Chem., 2021, 45(18), 8297–8305, 10.1039/D1NJ00771H
.
-
Fujifilm, Quotation for Dimatix Materials Printer, 2016, Published online.
- M. Shariq, S. Chattopadhyaya, R. Rudolf and A. Rai Dixit, Characterization
of AuNPs based ink for inkjet printing of low cost paper based sensors, Mater. Lett., 2020, 264, 127332, DOI:10.1016/j.matlet.2020.127332
.
- V. Beedasy and P. J. Smith, Printed Electronics as Prepared by Inkjet Printing, Materials, 2020, 13(3), 704, DOI:10.3390/ma13030704
.
- A. Määttänen, P. Ihalainen, P. Pulkkinen, S. Wang, H. Tenhu and J. Peltonen, Inkjet-printed gold electrodes on paper: Characterization and functionalization, ACS Appl. Mater. Interfaces, 2012, 4(2), 955–964, DOI:10.1021/am201609w
.
- V. Beedasy and P. J. Smith, Printed Electronics as Prepared by Inkjet Printing, Materials, 2020, 13(3), 704, DOI:10.3390/ma13030704
.
- Y. Sui and C. A. Zorman, Review—Inkjet Printing of Metal Structures for Electrochemical Sensor Applications, J. Electrochem. Soc., 2020, 167(3), 037571, DOI:10.1149/1945-7111/ab721f
.
- M. J. Coutts, M. B. Cortie, M. J. Ford and A. M. McDonagh, Rapid and controllable sintering of gold nanoparticle inks at room temperature using a chemical agent, J. Phys. Chem. C, 2009, 113(4), 1325–1328, DOI:10.1021/jp808927t
.
- M. Deng, X. Zhang, Z. Zhang, Z. Xin and Y. Song, A gold nanoparticle ink suitable for the fabrication of electrochemical electrode by inkjet printing, J. Nanosci. Nanotechnol., 2014, 14(7), 5114–5119, DOI:10.1166/jnn.2014.7208
.
- S. H. Ko, H. Pan, C. P. Grigoropoulos, C. K. Luscombe, J. M. J. Fréchet and D. Poulikakos, All-inkjet-printed flexible electronics fabrication on a polymer substrate by low-temperature high-resolution selective laser sintering of metal nanoparticles, Nanotechnology, 2007, 18(34), 345202, DOI:10.1088/0957-4484/18/34/345202
.
- P. R. DeGregory, M. J. Anderson, J. A. Loussaert, R. M. Crooks, C. Renault and S. E. Fosdick, Wire, Mesh, and Fiber Electrodes for Paper-Based Electroanalytical Devices, Anal. Chem., 2014, 86(7), 3659–3666, DOI:10.1021/ac5004294
.
- H. Tang, J. Zhu, D. Wang and Y. Li, Dual-signal amplification strategy for miRNA sensing with high sensitivity and selectivity by use of single Au nanowire electrodes, Biosens. Bioelectron., 2019, 131(February), 88–94, DOI:10.1016/j.bios.2019.02.023
.
- J. A. Adkins and C. S. Henry, Electrochemical detection in paper-based analytical devices using microwire electrodes, Anal. Chim. Acta, 2015, 891, 247–254, DOI:10.1016/j.aca.2015.07.019
.
- J. A. Adkins, E. Noviana and C. S. Henry, Development of a Quasi-Steady Flow Electrochemical Paper-Based Analytical Device, Anal. Chem., 2016, 88(21), 10639–10647, DOI:10.1021/acs.analchem.6b03010
.
- R. B. Channon, Y. Yang, K. M. Feibelman, B. J. Geiss, D. S. Dandy and C. S. Henry, Development of an Electrochemical Paper-Based Analytical Device for Trace Detection of Virus Particles, Anal. Chem., 2018, 90, 7777–7783, DOI:10.1021/acs.analchem.8b02042
.
- L. Wang, J. E. Filer, M. M. Lorenz, C. S. Henry, D. S. Dandy and B. J. Geiss, An ultra-sensitive capacitive microwire sensor for pathogen-specific serum antibody responses, Biosens. Bioelectron., 2020, 131, 46–52, DOI:10.1016/j.bios.2019.01.040
.
- H. Hua, Y. Liu, X. Guan and Y. Li, DNA nanosensors based on the use of single gold nanowire electrodes and Methylene Blue as an intercalator, Microchim. Acta, 2018, 185(2), 152, DOI:10.1007/s00604-018-2703-z
.
- R. B. Channon, Y. Yang, K. M. Feibelman, B. J. Geiss, D. S. Dandy and C. S. Henry, Development of an Electrochemical Paper-Based Analytical Device for Trace Detection of Virus Particles, Anal. Chem., 2018, 90(12), 7777–7783, DOI:10.1021/acs.analchem.8b02042
.
- J. K. Bhattarai, D. Neupane, B. Nepal, V. Mikhaylov, A. V. Demchenko and K. J. Stine, Preparation,
modification, characterization, and biosensing application of nanoporous gold using electrochemical techniques, Nanomaterials, 2018, 8(3), 171, DOI:10.3390/nano8030171
.
- M. M. Collinson, N. Chaniotakis, D. Kara, V. A. Lemos, E. Lodyga-Chruscinska and B. Rittich, Nanoporous Gold Electrodes and Their Applications in Analytical Chemistry, ISRN Anal. Chem., 2013, 1–20, DOI:10.1155/2013/692484
.
- P. Daggumati, Z. Matharu and E. Seker, Effect of Nanoporous Gold Thin Film Morphology on Electrochemical DNA Sensing, Anal. Chem., 2015, 87(16), 8149–8156, DOI:10.1021/acs.analchem.5b00846
.
- P. Daggumati, Z. Matharu, L. Wang and E. Seker, Biofouling-Resilient Nanoporous Gold Electrodes for DNA Sensing, Anal. Chem., 2015, 87(17), 8618–8622, DOI:10.1021/acs.analchem.5b02969
.
- S. Saraf, C. J. Neal and S. Park,
et al., Electrochemical study of nanoporous gold revealing anti-biofouling properties, RSC Adv., 2015, 5(58), 46501–46508, 10.1039/c5ra05043j
.
- J. Patel, L. Radhakrishnan and B. Zhao,
et al., Electrochemical Properties of Nanostructured Porous Gold Electrodes in Biofouling Solutions, Anal. Chem., 2013, 85(23), 11610–11618, DOI:10.1021/ac403013r
.
- Y. Liu, J. H. Moore, G. L. Kolling, J. S. McGrath, J. A. Papin and N. S. Swami, Minimum bactericidal concentration of ciprofloxacin to Pseudomonas aeruginosa determined rapidly based on pyocyanin secretion, Sens. Actuators, B, 2020, 312, 127936, DOI:10.1016/j.snb.2020.127936
.
- Z. Matharu, P. Daggumati, L. Wang, T. S. Dorofeeva, Z. Li and E. Seker, Nanoporous-Gold-Based Electrode Morphology Libraries for Investigating Structure-Property Relationships in Nucleic Acid Based Electrochemical Biosensors, ACS Appl. Mater. Interfaces, 2017, 9(15), 12959–12966, DOI:10.1021/acsami.6b15212
.
- P. Daggumati, S. Appelt, Z. Matharu, M. L. Marco and E. Seker, Sequence-Specific Electrical Purification of Nucleic Acids with Nanoporous Gold Electrodes, J. Am. Chem. Soc., 2016, 138(24), 7711–7717, DOI:10.1021/jacs.6b03563
.
- X. Quan, L. M. Fischer, A. Boisen and M. Tenje, Development of nanoporous gold electrodes for electrochemical applications, Microelectron. Eng., 2011, 88(8), 2379–2382, DOI:10.1016/j.mee.2010.12.121
.
- J. van der Zalm, S. Chen, W. Huang and A. Chen, Review—Recent Advances in the Development of Nanoporous Au for Sensing Applications, J. Electrochem. Soc., 2020, 167(3), 037532, DOI:10.1149/1945-7111/ab64c0
.
- A. Zhang, J. Wang, P. Schützendübe, H. Liang, Y. Huang and Z. Wang, Beyond dealloying: development of nanoporous gold via metal-induced crystallization and its electrochemical properties, Nanotechnology, 2019, 30(37), 375601, DOI:10.1088/1361-6528/ab2616
.
- J. A. Hondred, Z. T. Johnson and J. C. Claussen, Nanoporous gold peel-and-stick biosensors created with etching inkjet maskless lithography for electrochemical pesticide monitoring with microfluidics, J. Mater. Chem. C, 2020, 8(33), 11376–11388, 10.1039/d0tc01423k
.
- K. J. Stine, Biosensor applications of electrodeposited nanostructures, Appl. Sci., 2019, 9(4), 797, DOI:10.3390/app9040797
.
- T. A. Green, Gold Electrodepositino for Microelectronic, Optoelectronic and Microsystem Applications, Gold Bull., 2007, 40(2), 105–114 CrossRef CAS
.
- Buy 24K Gold Leaf - L.A. Gold Leaf U.S., Accessed February 27, 2021, https://www.lagoldleafus.com/product/24k-genuine-gold-leaf/.
- A. Keller, J. Pham, H. Warren and M. Panhuis, Conducting hydrogels for edible electrodes, J. Mater. Chem. B, 2017, 5, 5318–5328, 10.1039/C7TB01247K
.
- W. A. Ameku, J. M. Gonçalves and V. N. Ataide,
et al., Combined Colorimetric and Electrochemical Measurement Paper-Based Device for Chemometric Proof-of-Concept Analysis of Cocaine Samples, ACS Omega, 2021, 6(1), 594–605, DOI:10.1021/acsomega.0c05077
.
- Y. Huang, Y. Huang and Y. Wang,
et al., A Wearable Supercapacitor Engaged with Gold Leaf Gilding Cloth Toward Enhanced Practicability, ACS Appl. Mater. Interfaces, 2018, 10(25), 21297–21305, DOI:10.1021/acsami.8b03780
.
- K. Shimada and T. Toyoda, Gold leaf counter electrodes for dye-sensitized solar cells, Jpn. J. Appl. Phys., 2018, 57, 03EJ04, DOI:10.7567/JJAP.57.03EJ04
.
- B. L. Thompson, C. Birch and D. A. Nelson,
et al., A centrifugal microfluidic device with integrated gold leaf electrodes for the electrophoretic separation of DNA, Lab Chip, 2016, 16(23), 4569–4580, 10.1039/C6LC00953K
.
-
H. Jeong and J. M. Seo, Electrode design on plastic substrates using laser patterned double-sided tape and gold leaf, in Proceedings of the Annual International Conference of the IEEE Engineering in Medicine and Biology Society, EMBS, Institute of Electrical and Electronics Engineers Inc., 2019, pp. 1286–1289, DOI:10.1109/EMBC.2019.8857635
.
- M. S. F. Santos, W. A. Ameku, I. G. R. Gutz and T. R. L. C. Paixão, Gold leaf: From gilding to the fabrication of disposable, wearable and low-cost electrodes, Talanta, 2018, 179, 507–511, DOI:10.1016/j.talanta.2017.11.021
.
- P. Prasertying, N. Jantawong and T. Sonsa-Ard,
et al., Gold leaf electrochemical sensors: Applications and nanostructure modification, Analyst, 2021, 146(5), 1579–1589, 10.1039/d0an02455d
.
- M. Zamani, J. M. Robson, A. Fan, M. S. Bono, A. L. Furst and C. M. Klapperich, Electrochemical Strategy for Low-Cost Viral Detection, ACS Cent. Sci., 2021, 7(6), 963–972, DOI:10.1021/acscentsci.1c00186
.
- M. S. F. Santos, W. A. Ameku, I. G. R. Gutz and T. R. L. C. Paixão, Gold leaf: From gilding to the fabrication of disposable, wearable and low-cost electrodes, Talanta, 2018, 179, 507–511, DOI:10.1016/j.talanta.2017.11.021
.
- N. J. Francis and C. R. Knospe, Fabrication and Characterization of Nanoporous Gold Electrodes for Sensor Applications, Adv. Eng. Mater., 2019, 21(3), 1800857, DOI:10.1002/adem.201800857
.
- 2019 Pharmaceutical/Biological Facility Cleanroom Cost Data Benchmarks - Compass International, Accessed February 27, 2021, https://compassinternational.net/2019-pharmaceutical-biological-facility-cleanroom-cost-data-benchmarks/.
- Harvard Center for Nanoscale Systems Nanfabrication Facilities, https://cns1.rc.fas.harvard.edu/, Accessed February 27, 2021.
-
Gold wire quote, California Fine Wire Company, December 2019 Search PubMed.
|
This journal is © The Royal Society of Chemistry 2023 |
Click here to see how this site uses Cookies. View our privacy policy here.