Highly versatile and automated total ammonia nitrogen compact analyzer suitable for different types of water samples
Received
12th May 2023
, Accepted 7th October 2023
First published on 19th October 2023
Abstract
Continuous monitoring of total ammonia nitrogen (TAN) currently needs to improve further not only to meet optimal analytical features but also to reduce equipment maintenance needs and avoid manual intervention. The development, evaluation in different aqueous samples (fresh and wastewater) and validation of a fully automated and adaptable miniaturized potentiometric analytical instrument with this purpose are presented. The main goals accomplished are a wider and adaptable working range (0.08 to 1000 mg L−1 NH4+) with a detection limit of 0.03 mg L−1 and a significant reduction of reagent and sample consumption, as well as waste generation, compared to current commercial potentiometric flow analyzers. Moreover, different programmable calibration and sample analysis protocols have been successfully applied. Finally, suitable analytical features in terms of accuracy and precision are achieved even with samples with very complex matrices without any pretreatment required.
Water impact
Total ammonia nitrogen (TAN) needs to be monitored to assess water quality with the goal of sustainable development. We present a highly portable and versatile analyzer for continuous monitoring of TAN, which is applicable to different water bodies. It features autonomy, automation, low sample and reagent consumption, interference-free, a wide linear range, low maintenance and minimal waste generation.
|
Introduction
Total ammonia nitrogen (TAN), expressed as the sum of NH3 and NH4+ due to the pH dependent equilibrium of both compounds,1 is an important parameter to monitor in water and aqueous ecosystems because it is involved in many applications and natural processes.2 Regarding flora and fauna, TAN is a growth limiting nutrient for algae, so its concentration is related to water eutrophication.2,3 Moreover, whereas ammonium is harmless, ammonia is a toxic product for fishes because it limits the proper elimination of their metabolized ammonia.4 In both industrial and urban wastewater treatment plants, monitoring TAN is important to verify the proper operation of nitrification processes (where under aerobic conditions, ammonium/ammonia is oxidized to nitrite and finally to nitrate) and to assure that its concentration in the effluents to natural water bodies is within the allowed range by legislation.3,5,6 The natural TAN concentration in surface and groundwater is below 0.2 mg L−1 NH4+, while concentrations above 1.5 and 35 mg L−1 NH4+ cause odor and taste problems, respectively. In drinking water, high levels of TAN are related to ineffective chlorinated disinfection processes,2,7 in which it is combined with chlorine and can produce di- and trichloramines causing taste and odor problems. About human health, acute poisoning due to ammonium or ammonia ingestion could lead to lung edema, nervous system dysfunction, acidosis, kidney damage and even death.2,8,9
Thus, EU legislation establishes a TAN limit concentration of 0.5 mg L−1 (expressed as mg L−1 NH4+) in drinking water and 10–50 mg L−1 NH4+ in effluents discharged into natural water bodies from wastewater treatment plants. However, the concentration of their effluents could range 10–100 mg L−1 and 10–1000 mg L−1 NH4+ in urban and industrial wastewater treatment plants, respectively.5,10–14 In this context and in order to fulfill the required analytical features of all the scenarios, miniaturized analyzers, suitable for in-field monitoring of a wide range of concentrations of TAN in a wide variety of complex matrices, with higher autonomy and automation, are necessary.
There are currently different analytical methods to determine TAN in aqueous samples such as spectrophotometry,10,15 conductimetry16,17 and potentiometry.18–20 In particular, ion selective electrodes (ISEs) are preferred for continuous monitoring thanks to the possibility to be integrated into flow techniques, which allows achieving a high level of automation and autonomy and high speed of analysis, as well as simplicity, versatility, robustness, selectivity and a wide working range.4,15,21,22 On the other hand, TAN analyzers that take advantage of the separation of TAN in the ammonia form from the sample matrix through a gas-diffusion membrane exhibit the highest selectivity towards this analyte.17,23,24 However, the ones that combine potentiometric detection systems with gas-diffusion stages and continuous flow techniques20,25–27 still lack enough autonomy to be adapted to any type of water source and concrete requirements without any sample pretreatment. Therefore, the desired operational requirements for a suitable TAN analyzer are high portability for in situ measurements, autonomy, automation and low sample and reagent consumption for continuous monitoring with low maintenance requirements, a wide or adaptable working range to face TAN determination in different types of water sources, and high capability to operate with complex matrices, providing reliable results in real time.
For all these reasons, a low cost, compact and portable full analytical instrument to determine total ammonia nitrogen in the ammonium form in aqueous samples, based on the flow injection analysis (FIA) technique, is proposed in this work. It is composed of four main parts: 1) a monolithic and compact microfluidic platform fabricated in cyclic olefin copolymer (COC) similar in size to a credit card, which integrates a gas-diffusion membrane and the detection system consisting of an ammonium ion selective electrode and a Ag/AgCl reference electrode; 2) a miniaturized liquid management system, with flow elements such as microvalves and micropumps; 3) an analytical data acquisition and processing system; 4) a computer to manage the whole system that enables an automatic and unattended operation by means of versatile processes of autocalibration and sample analysis.
COC has been selected to fabricate the microfluidic platform because it allows easy monolithic integration of gas-diffusion polymeric membranes, besides showing other interesting advantages such as good mechanical resistance, chemical inertia against most acids and alkalis, easy microfabrication of hermetically sealed three-dimensional structures and the possibility to integrate conductive tracks.28–31 The whole system is comparable in size to a shoe box and its portability, wide adaptable working range, low maintenance and low waste generation and high accuracy and precision without any pretreatment required constitute the differential features with respect to existing commercial equipment for TAN determination.
Experimental
Materials and reagents
Different grades and thicknesses of COC layers and foil, obtained from Topas Advanced Polymers (Florence, KY, USA), were used to fabricate the microfluidic platform: Topas 8007 foil of 25 and 50 μm thick and Topas 5013 layers of 300 μm and 1 mm thick. A graphite–epoxy composite, resulting from mixing graphite powder of 50 μm particle size (Merck, Germany), Araldite-M epoxy-resin and HR hardener (Ciba-Geigy, Switzerland), was used for the ISE conductive support. A screen-printable Ag/AgCl paste (C2030812D3, Gwent, Pontypool, UK) was used for the Ag/AgCl reference electrode fabrication. A 0.45 μm pore diameter polyvinylidene fluoride (PVDF) hydrophobic membrane (Millipore, USA) was used to separate, by gas diffusion, the analyte from the sample matrix. In order to prepare the ammonium sensor membrane, nonactin, bis(1-butylpentyl)adipate (BBPA) and polyvinyl chloride (PVC), all dissolved in tetrahydrofuran (THF), were used (obtained all from Fluka, Barcelona, Spain).
All reagents used for the development and characterization of the analytical system were of analytical grade. Milli-Q water was used for the preparation of all solutions. Two ammonium chloride (Panreac, Spain) stock solutions containing 1 and 1000 mg L−1 NH4+ were made to be used in the automatic calibration process. As a reference solution to set at a constant value the reference electrode potential, a 100 mM KCl solution (Sigma Aldrich, Germany) was employed. A 10 mM tris(hydroxymethyl)aminomethane (Tris) solution (Sigma Aldrich, Germany) set with hydrochloric acid (Merck, Germany) to pH 7.4 was employed to transform ammonia into the ammonium ion. This solution is called acceptor solution throughout the text. As a basic solution, a 100 mM NaOH solution (Sigma Aldrich, Germany) containing 1 mM ethylenediamine tetraacetic acid (EDTA) (Panreac, Spain) was employed. The obtained solution from mixing the sample/standard solution and this basic solution is called donor solution throughout the text.
Fabrication of the microfluidic platform
The COC-based microanalyzer was fabricated following a multilayer microfabrication procedure described in detail elsewhere.27,32 The process consists of the lamination of different COC layers presenting two different glass transition temperatures (Tg): Topas 8007 foil with Tg of 75 °C (used as sealing layers) and 5013 layers with Tg of 130 °C (used as structural layers containing the microfluidic structures), so that once the process is finished, complete sealed 3D microfluidic structures are obtained in one substrate. The following four steps are required to fabricate the microanalyzer: layer design, structure micromachining, integration of the separation membrane and electrodes and the final lamination of the layers.
CAD software was used to design the microsystem prototype (Fig. 1). It consisted of four layers: two COC 5013 layers of 1 mm (a and c), a 300 μm-COC 5013 layer (d) and 50 μm-COC 8007 foil (b). The microchannel dimensions were 0.4 mm × 0.3 mm, except for the meander-shaped microchannels inside the gas-diffusion module, which were 1 mm × 0.1 mm in order to increase the contact area between the solution and the hydrophobic PVDF membrane and thus, promote ammonia diffusion. The detection chamber, where the ammonium selective electrode was placed, was 2 mm in diameter. The final dimensions of the microfluidic platform were 50 × 65 × 3 mm with a total weight of 10 g. The detection chamber and total microsystem dead volumes were 2 and 97 μL, respectively.
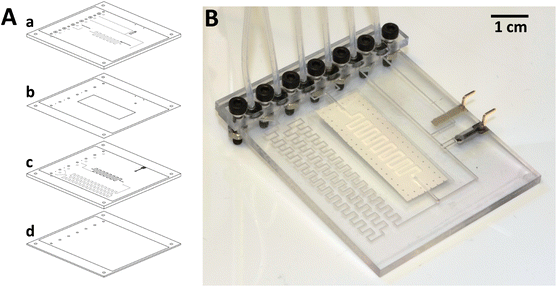 |
| Fig. 1 A: Layered CAD design of the microfluidic platform. Layers “a” and “c” have fluidic structures on both sides, depicted in black in front and gray in back; B: image of the final microanalyzer. | |
A computer controlled Protomat C100/HF micromilling machine (LPKF, Germany) was used for the microfluidic structure machining (holes, microchannels and bas-relief for the ISE conductive support integration) onto the COC layers.
For the conductive support for the ammonium selective electrode, a mixture of Araldite-M, HR hardener and graphite powder at 36, 14 and 50% in weight, respectively, was prepared. The composite mixture was placed inside the corresponding bas-relief (Fig. 1) and was cured for 24 h at 40 °C. In order to obtain a flat surface facilitating a proper final lamination process, the electrode was polished. For the reference electrode, a Ag/AgCl paste was screen-printed using a DEK 248 screen-printer machine (DEK, Spain), being cured for 30 min at 80 °C. For the monolithic PVDF membrane integration, first it was cut, washed with Milli-Q water and dried with air and then, placed (layer b in Fig. 1A) with the more hydrophobic side oriented towards the donor solution. As has been reported previously,27,33 some holes were made on the PVDF membrane in order to favor the correct sealing during the final lamination.
In order to obtain a fully sealed monolithic microfluidic platform, final lamination was performed using a temperature-controlled customized press (Francisco Camps, Granollers, Spain) set at 4 atm pressure and 102 °C. Regarding the formulation of the ammonium selective polymeric membrane, it was previously optimized27 and prepared with 1% nonactin, 65.5% BBPA and 33.5% PVC in 3 mL THF. The resulting membrane cocktail was deposited inside the corresponding cavity and over the graphite conductive support until it was completely filled with successive dosages of 2 μL using a micropipette every five minutes to avoid the formation of bubbles due to the rapid THF evaporation. Finally, liquid connectors were set over the corresponding inlets or outlets using a bracket and screws, and the detection chamber and microchannels exposed to the surface were sealed with an adhesive film (Fig. 1).
Experimental setup
Fig. 2 shows a drawing of the whole equipment developed. It is composed of four main parts: the microfluidic platform or microanalyzer, the liquid management system, the analytical signal acquisition and processing system, and the global management system. The flow system setup consists of three solenoid micropumps, one performing (Fig. 2, SP3) 4 μL per pulse and two of them (Fig. 2, SP1 and SP2) pumping 10 μL per pulse (BiochemValve Inc., Montluçon Cedex, France), one peristaltic micropump (Fig. 2, PP) (Kamoer KP-S10DGC0, Shanghai, China), which integrates a customized rpm controller (TMI, Barcelona, Spain) and operates with tubing of 0.19 mm internal diameter (Ismatec, Wertheim, Germany) made of Tygon®, and four three-way solenoid valves (Fig. 2, Vx) (NResearch, Switzerland). Tubing of 0.8 mm internal diameter (Scharlab, S.L., Sentmenat, Spain) made of Teflon is used to join the microfluidic platform with all the flow elements. A controller for flow elements (Fig. 2, Flow controller) (Flowtest™, Biotray, France) is used to actuate the flow elements. A customized and miniaturized potentiometer (Fig. 2, P) (TMI, Barcelona, Spain) is used for the signal acquisition and processing. Both the flow controller and the potentiometer are managed using software driven by a personal computer that allows customized programming to automate the entire analytical procedure (calibration and sample analysis) without user interaction. Simple signal acquisition and processing were carried out using LabVIEW-based software, in which the raw potential signal provided by the potentiometric cell was taken and averaged to obtain 10 measurements per second. It was subsequently used to calculate the peak heights of each transient signal generated when the ammonium ion is detected, corresponding to each standard solution injected for calibration purposes and each sample analyzed. Afterwards, the concentration of TAN present in the sample was obtained by interpolation of the peak height generated by the sample in the Nernst equation, previously obtained in the calibration procedure. One calibration per day was performed in order to ensure reliable results.
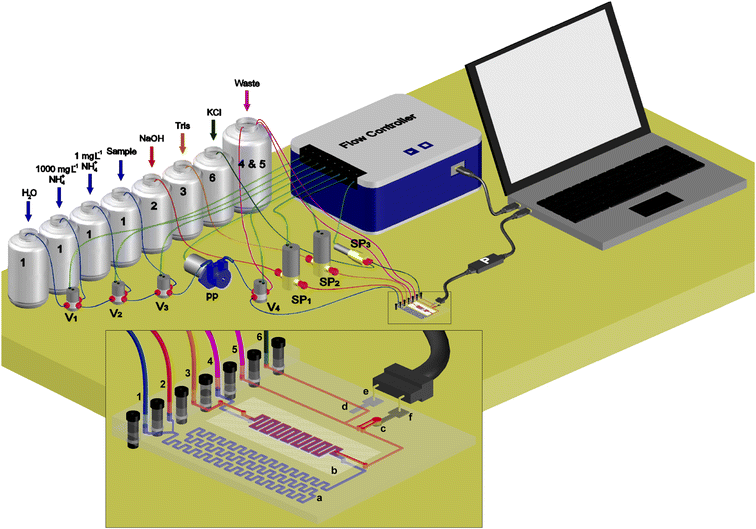 |
| Fig. 2 Schematic illustration of the complete analytical equipment, where: 1, 2, 3 and 6 are inlets; 4 and 5 are outlets to waste; a) micromixer; b) PVDF gas-diffusion membrane; c) ammonium selective electrode; d) reference electrode; e) reference electrode electric connector; f) ISE electric connector; P: customized potentiometer; PP: peristaltic micropump; SPx: solenoid micropump; Vx: three-way solenoid microvalve. | |
Analysis principle
The microfluidic platform includes four liquid inlet and two outlet ports (Fig. 2). The channels of the first two input ports (1 and 2) converge at a junction point, where the injected samples or standard solutions into the carrier solution (1) get mixed with the NaOH solution (2), thus becoming the donor solution. This mixed stream is carried towards the gas-diffusion module, where the generated ammonia gas diffuses through the PVDF membrane along a meander-shaped microchannel to the acceptor solution (3) (Tris buffer adjusted to pH 7.4), where ammonia is converted back to the ammonium ion. The remains of the donor solution reach the waste outlet (4), while the acceptor solution is directed towards the detection chamber, where the analyte is detected by the ammonium selective electrode. Finally, liquid exits through the second outlet port (5). With the aim of maintaining the reference electrode potential at a constant value, a 100 mM KCl solution is pumped at 100 μL min−1 through the fourth inlet port (6) flowing through the microchannel where the reference electrode is located. This joins also the microchannel of the acceptor solution after the detection chamber.27
Sample analysis procedure
In order to validate the performance of the analytical microsystem and verify that the proposed method does not present significant differences compared to a reference method, real samples from different origin, matrices and TAN concentrations were analyzed off-line. They were obtained from three different places: 1) water from different stages of a wastewater treatment plant of a surfactant manufacturing industry, placed in Granollers (Barcelona, Spain), 2) water from different stages of the pilot plant of the Micro-Ecological Life Support System Alternative (MELiSSA), which is a project carried out by the European Space Agency (ESA) at the Autonomous University of Barcelona (Spain),34 and 3) tap water from the city of Cerdanyola del Vallès (Barcelona, Spain). In the first case, biomass was allowed to precipitate and water was decanted and analyzed without filtration before the analysis, simulating real sampling procedures. In the second case, biomass was filtered before analysis because of the current specific protocol for this application. Finally, tap water was analyzed without any filtration after being spiked with the ammonium ion. Additionally, the simulation of real-time and in situ continuous monitoring of TAN in wastewater of a reactor with biomass from a surfactant manufacturing industry was carried out to verify the applicability of the developed prototype to real work environments. Results obtained from the analysis of water from treatment plants were validated by comparison with those obtained with the indophenol blue standard method.15 Recovery rates were calculated with tap water to verify results.
Results and discussion
Fabrication and optimization of the microfluidic platform
The main objective of the present work was to develop a compact, versatile, low cost and portable analytical instrument for TAN monitoring in different aqueous samples based on the use of a potentiometric microfluidic platform, previously optimized for other applications,27,35 and achieve the best analytical features for ammonium determination in water bodies regarding baseline signal stability, sensitivity, the detection limit and the lifetime of the gas-diffusion hydrophobic membrane. The pursued TAN analyzer must also fulfill features such as simplicity, selectivity, robustness, a wide working range, easy automation and low reagent and sample consumption to minimize waste generation.
In order to minimize analysis time, reagent and sample consumption and microanalyzer manufacturing costs, which is key to further industrialization purposes, the microfluidic platform was redesigned (microfluidics and detection chamber). For instance, the volume of the detection chamber was reduced almost 8 times (15 to 2 μl).27 In this way, the analysis time was optimized (2 minutes faster than using a larger detection chamber) due to a faster solution renewal on the detection chamber. This not only allows speeding up the signal baseline recovery but also saving reagents.
On the other hand, to provide robustness to the microfluidic platform, an electrical connection system based on pin connections was implemented (Fig. 1B). It enables a more reliable electrical connection and noise reduction, thus obtaining more reproducible results than previous analyzers.27
The influence of chemical (reagent concentrations and pH) and hydrodynamic (injection volume and flow rate) variables on the system performance was studied using a procedure of univariate optimization after programming automated operation sequences. The goal was to explore versatility to different water bodies by complying with the required linear ranges and each of the analytical features.
Tris buffer was selected as the acceptor solution due to the provided better analytical features compared to other buffers.20,21,27 Its concentration was tested from 5 to 100 mM. NaOH concentration in basic solution was studied from 0.1 to 1000 mM (with a fixed EDTA concentration of 1 mM). EDTA was used to avoid possible metal hydroxide precipitation, thus preventing damage or blockage inside microfluidics or on the gas-diffusion membrane. Optimum solution compositions for basic and acceptor solutions were finally 100 mM NaOH with 1 mM EDTA and 10 mM Tris, respectively.
Regarding hydrodynamic variables, the injection volume of the sample was tested between 25 and 1000 μL while the flow rate of each stream (carrier, basic and acceptor solutions) was studied between 50 and 800 μL min−1. The 100 mM KCl solution to keep constant the reference electrode potential was pumped at a flow rate of 100 μL min−1. As results show, hydrodynamic parameters can be selected in order to fit the linear range and limit of detection to the expected TAN concentration in samples to be analyzed, so this can be automatically modulated depending on the final application.
Moreover, the analyte is separated from the matrix due to the gas-diffusion stage, so only those compounds able to diffuse through the PVDF membrane in basic medium are potential interfering compounds of the ammonium ISE. In this sense, only methylated amines could be an actual interfering compound, which is not expected to be found in natural water but in industrial wastewater samples.
Liquid management system optimization
A miniaturized and fully automated computer-controlled (Flowtest™) liquid management system was applied combining different solenoid micropumps and microvalves and a peristaltic micropump. The operation is simple; the FlowTest™ provides a 12 V pulse to activate the solenoid devices (in the case of microvalves, to change the position; in the case of micropumps, to fill and empty the plunger with the liquid to be dispensed). In the case of the peristaltic micropump, during the operating period a potential between 0 and 12 V is applied corresponding to the desired flow rate. The FlowTest™ allows performing automatic multicommutation dilution sequences with the microvalves and modulating hydrodynamic parameters (injection volume and flow rate) to easily optimize the desired analytical features such as sensitivity, the detection limit, linear range and time of analysis, among others. The software interface is very intuitive and very easy to use. In this sense, by simply introducing the different operating parameters required (operation time of each microfluidic element, multicommutation time, etc.) the different sequences can be created in a very simple way and without the need for advanced programming knowledge.
An automated calibration process can be performed using a multicommutation dilution procedure from a concentrated stock solution, being able to prepare in real time adequate standards.36,37 When carrying out this calibration process, different technical parameters must be taken into account such as the minimum microvalve operation time, the injection time and the pumping system used. The accuracy of the dilution process and therefore the amplitude of the linear range obtained will depend on the proper selection of the solenoid microvalve and the peristaltic micropump. Due to instrumental restrictions, the highest commutation speed (on/off) of the solenoid microvalve used in this work was 100 ms.37–39 Thus, dilution factors of 100 times the stock solution concentration can be reached with an RSD less than 5%. Therefore, in order to cover the desired range (0.08 to 1000 mg L−1 NH4+–N), two stock standard solutions of 1 and 1000 mg L−1 NH4+ were used and two different multicommutation dilution sequences were created, called programs 1 and 2. Program 1 was designed to determine low TAN concentrations (from 0.08 to 10 mg L−1 NH4+) so they would be indicated for the analysis of drinking water or to monitor water quality in natural water bodies such as rivers or lakes. On the other hand, program 2 would be adequate to determine high TAN concentrations (from 1 to 1000 mg L−1 NH4+) like the ones found in wastewater treatment plants. In order to optimize reagent consumption, waste generation and analysis time, two different configurations of flow rate and injection time were set: program 1 (flow rate: 350 μL min−1, injection time: 60 s, corresponding to 350 μL injection volume) and program 2 (flow rate: 400 μL min−1, injection time: 20 s, corresponding to 133 μL injection volume).
Table 1 shows the different parameters related to the multicommutation dilution sequences used in each program to obtain the corresponding standard solutions.
Table 1 Multicommutation parameters used to obtain each standard solution
Program number |
Standard solution (mg L−1) |
Stock used (mg L−1) |
Total injection time (s) |
Time on (stock solution) (s) |
Time off (water) (s) |
Cycles |
Dilution factor |
1 |
10 |
1000 |
60.00 |
0.10 |
9.90 |
6 |
1 : 100 |
1 |
1 |
1 |
60.00 |
60.00 |
0.00 |
1 |
— |
1 |
0.1 |
1 |
60.00 |
0.10 |
0.90 |
60 |
1 : 10 |
1 |
0.08 |
1 |
60.00 |
0.10 |
1.15 |
48 |
1 : 12.5 |
2 |
1000 |
1000 |
20.00 |
20.00 |
0.00 |
1 |
— |
2 |
100 |
1000 |
20.00 |
0.10 |
0.90 |
20 |
1 : 10 |
2 |
10 |
1000 |
20.00 |
0.10 |
9.90 |
2 |
1 : 100 |
2 |
1 |
1 |
20.00 |
20.00 |
0.00 |
1 |
— |
It should be noted that it would be also possible to combine automatically both programs in order to obtain a wider linear range (using two calibration curves simultaneously) or besides, to create a new program to fit the expected TAN concentration present in samples. If extremely high TAN concentrations were to be determined, it would be even possible to integrate an automatic sample dilution by including an extra microvalve.
The operation of the dilution by multicommutation was evaluated comparing the obtained signal peak height of manually prepared standard solutions with the ones obtained automatically. No significant differences were found between them (RSD below 3%).
Analytical features
Two different calibration curves were obtained corresponding to each established program. Fig. 3A and B show the signal record for one calibration process using program 1. The found Nernst equation (using one calibration data, with triplicates for each standard solution; n = 4 and 95% confidence) was E = 333 (±4) + 57 (±1)log[NH4+] with r2 = 0.9996. The linear range was from 0.08 to 10 mg L−1 NH4+ with a limit of detection, according to IUPAC,40 of 0.03 mg L−1 NH4+. Fig. 3C and D show the signal record for one calibration process using program 2. The obtained Nernst equation (using one calibration data, with triplicates for each standard solution; n = 4 and 95% confidence) was E = 251 (±3) + 52 (±1)log[NH4+] with r2 = 0.9993. The linear range was from 1 to 1000 mg L−1 NH4+ with a limit of detection of 0.3 mg L−1 NH4+. These results demonstrated the versatility, reliability and robustness of the complete analytical equipment. Analysis frequencies of 10 and 8 samples per hour using programs 1 and 2 were obtained, respectively. However, it is possible to shorten the analysis time by automating the injection of a new standard solution or sample once the baseline signal is recovered instead of waiting a specific time (6 min for program 1 and 8 min for program 2). Thus, the sample throughput could be easily doubled.
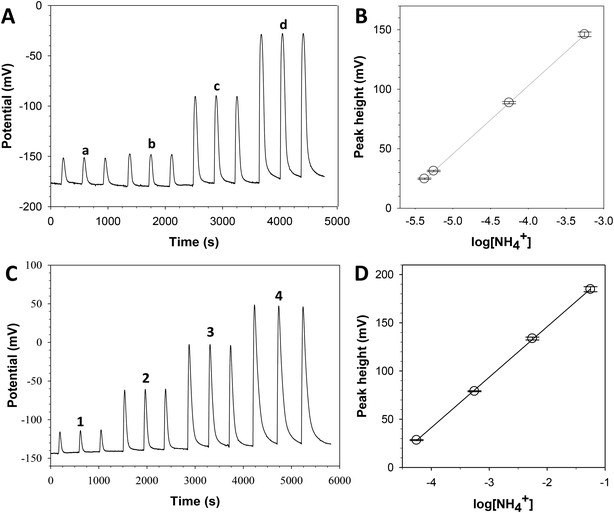 |
| Fig. 3 Obtained recorded signal (A) and calibration plot (B) using program 1. TAN standard solutions of 0.08 (a), 0.1 (b), 1 (c) and 10 mg L−1 NH4+ (d). Obtained recorded signal (C) and calibration plot (D) using program 2. TAN standard solutions of 1 (1), 10 (2), 100 (3) and 1000 mg L−1 NH4+ (4). | |
System repeatability was calculated from replicates of each standard solution. The RSD of peak heights was less than 3%. Reproducibility was also tested during the first month through 5 calibration experiments: a mean slope of the calibration curve of 57 with a RSD value of 8% was obtained, confirming the intra and inter-day precision.
Taking into account that the sample may have a complex and highly aggressive matrix, the gas-diffusion membrane avoids its contact with the surface of the sensor membrane on the indicator electrode. In addition, the tangential flow configuration in the gas-diffusion stage avoids the suspended solids that may be introduced into the microfluidic platform to obstruct or clog the separation membrane. Moreover, the chosen strategy of the FIA technique allows the sample to be in contact with the different parts of the measuring equipment only for a short time, since only 133 or 350 μL of sample is either injected, depending on the analysis program selected. For all this, the potentially negative effects caused by the sample matrix on each element of the analytical microsystem are greatly attenuated, improving the lifetime of the electrodes, gas-diffusion/separation membrane and microfluidic platform, compared to other probe-type or flow-based commercial analytical systems. The lifetime of the microfluidic platform including the gas-diffusion membrane and ISE was at least 2 months. In addition, an active or special pretreatment of the sample is not required prior to the analysis.
Thanks to the level of automation achieved both in the calibration and measurement processes and to the miniaturization of the equipment, the consumption of reagents and the generation of waste are reduced. The reagent consumption was calculated to be 6 L per month, taking into consideration that current automatic stations for water quality control perform one analysis of TAN per hour and that systems stop in between after the baseline stabilization. Therefore, the TAN analyzer works autonomously and without maintenance until the required replacement of reagents and the microfluidic platform for at least two months. This autonomy is higher than that of most commercial equipment.
Real sample analysis
Fig. 4A shows the results from the analysis of 65 samples with a very complex matrix from different stages of the surfactant manufacturing industry wastewater treatment plant, which includes nitrifying processes. The TAN concentration was found between 1 and 124 mg L−1 NH4+, depending on the sample origin. No statistical differences were found from the graphical representation (n = 65, 95% confidence) of the results for each method: [NH4+]Ref = 1.01 (±0.03) [NH4+]Analyzer + 1 (±2); R2 = 0.9782, and in accordance with the paired t-test (tcalc = 1.934, ttab = 2.000, tcalc < ttab).
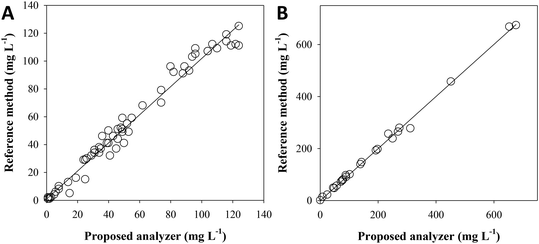 |
| Fig. 4 Result comparison of the proposed analyzer and the indophenol blue reference method for the analysis of wastewater samples from a surfactant manufacturing industry (A) and MELiSSA (B). Each value is the result of an average of three measurements. | |
Fig. 4B shows the results from the analysis of 25 samples from different stages of the MELiSSA project pilot plant. The TAN concentration was found between 4 and 700 mg L−1 NH4+, depending on the sample origin. No statistical differences were found from the graphical representation (n = 25, 95% confidence) of the results for each method: [NH4+]Ref = 1.00 (±0.02) [NH4+]Analyzer −1 (±6); R2 = 0.9972, and in accordance with the paired t-test (tcalc = 0.138, ttab = 2.064, tcalc < ttab).
Finally, 6 spiked tap water samples gave results shown in Table 2. The obtained recoveries range from 98% to 106%. As can be seen, the TAN concentration for the unspiked sample is under the ISE linear range (less than 0.075 mg L−1 NH4+); however, this concentration is below the maximum concentration allowed by the legislation in tap water (0.5 mg L−1 NH4+). Therefore, the system can be used to monitor TAN in fresh or drinking water even below the maximum concentration allowed by legislation.
Table 2 Sample analysis from spiked tap water of Cerdanyola del Vallès. Concentration mean values in mg L−1 NH4+ (n = 3, 95%)
Sample |
Added |
Found |
% Rec |
1 |
0 |
<0.075 |
— |
2 |
0.1 |
0.11 ± 0.01 |
106 |
3 |
0.2 |
0.20 ± 0.01 |
99 |
4 |
0.3 |
0.31 ± 0.02 |
104 |
5 |
0.4 |
0.39 ± 0.02 |
98 |
6 |
0.5 |
0.51 ± 0.05 |
102 |
In order to verify that the analyzer maintains its analytical features unaltered in real operation environments, a simulation of TAN monitoring in a wastewater treatment plant was performed. For this, successive analyses of a real sample provided by a surfactant manufacturing industry and kept under constant agitation were carried out without any sample pretreatment (neither filtration nor biomass precipitation). After 7 hours of continuous monitoring, carrying out 4 analysis per hour, a mean concentration (n = 26, 95% confidence) of 54 ± 4 mg L−1 NH4+ was obtained, thus showing the analyzer robustness in a real operation environment.
All these results demonstrate the appropriateness of the developed analyzer for TAN determination in a very wide range of sample matrices and concentrations under unattended conditions fulfilling all the required analytical and operational features.
Comparison with commercial equipment
There are not many commercial systems only dedicated to ammonium analysis. Table 3 shows the features of the proposed prototype and those of different commercial equipment to measure TAN, classified into three groups: potentiometric probes, flow potentiometric equipment, and spectrophotometric equipment. Broadly, it can be seen that probe-type potentiometric devices show high portability and a wide working range with acceptable accuracy and repeatability. However, in all cases, they require sample pretreatment, they have a low level of automation and autonomy, and in some cases, they present important interferences from other monovalent cations. Regarding continuous flow potentiometric equipment, it presents wide working ranges, good accuracy and repeatability and a high level of automation and autonomy. However, it lacks portability because of its size and weight, it requires in most cases a sample pretreatment (mainly filtration), and in some cases there are interferences from other species. Interferences in potentiometric devices are usually corrected by simultaneously measuring ammonium and interferent ions using an array of electrodes and applying the Nernst–Nikolsky equation. This considerably increases costs and the complexity of the system. Finally, spectrophotometric equipment shows narrower working ranges, which can be widened by the use of different kits of reagents, and both accuracy and repeatability are worse than those with potentiometric equipment. These systems are not portable, sample pretreatment (filtration or dilution) is required in general, and there are interferents, either from the sample color or other compounds. Regarding automation and autonomy, these depend on the equipment.
Table 3 Features of different commercial equipment and the proposed analytical prototype. Information extracted from the commercial brochure of each equipment found on the website of each manufacturer
Analytical device (manufacturer) |
Technique |
Mass (kg)/volume (dm3) |
LOD (mg L−1 NH4+) |
LR (mg L−1 NH4+) |
Acc./repeat. |
Sample pret. |
Int. |
Autom. level |
Auton. (month) |
Acc.: accuracy, repeat.: repeatability, pret.: pretreatment, Int.: interferences, Autom.: automation, Auton.: autonomy. |
Developed in this work |
Potentiometry (flow) |
5/19.6 |
0.03 |
0.08–1000 |
2%/2% |
No |
No |
High |
2 (at least) |
Aquaprobe (Aquaread) |
Potentiometry (probe-type) |
2/2.4 (only probe) |
— |
0–9000 |
10%/− |
Yes |
Yes |
Low |
— |
Ammonia probe 8002 (ABB) |
Potentiometry (probe-type) |
−/0.3 (only probe) |
— |
0.1–1000 |
−/2% |
Yes |
No |
Low |
— |
Orion ammonia (ThermoFisher) |
Potentiometry (probe-type) |
−/0.05 (only probe) |
— |
0.01–17 000 |
−/2% |
Yes |
No |
Low |
— |
AN-ISE sc (HACH) |
Potentiometry (probe-type) |
2.4/1.8 (only probe) |
0.2 |
0–1000 |
5%/− |
Yes |
Yes |
Low |
— |
Ammo::lyser™ eco/pro (S::CAN) |
Potentiometry (probe-type) |
2.7/1 (only probe) |
— |
0.1–1000 |
3%/− |
Yes |
Yes |
Low |
— |
AMTAX™ sc ammonia (HACH) |
Potentiometry (flow) |
31/151 |
0.02 |
0.02–1000 (different ranges) |
5%/2% |
Yes |
Yes |
High |
3 |
Aztec AAM631 (ABB) |
Potentiometry (flow) |
15/43.7 |
— |
0.05–1000 (different ranges) |
7.5%/2% |
No |
No |
High |
3 |
PowerMon ionometer (Bran + Luebbe) |
Potentiometry (flow) |
60/234 |
— |
0–1000 |
−/5% |
Yes |
Yes |
High |
0.75 |
DR6000 (HACH) |
Spectrophotometry (discrete analysis) |
11/49.5 |
0.028 |
0.01–1800 (different ranges) |
−/8% |
Yes |
Yes |
Low |
— |
Aztec 600 (ABB) |
Spectrophotometry (flow) |
15/43.3 |
— |
0–0.529 (undiluted range) |
5%/5% |
Yes |
Yes |
High |
4 |
WIZ probe (Systea) |
Spectrophotometry (probe-type) |
8/8 |
0.001 |
0–0.5 |
10%/3% |
Yes |
Yes |
Medium |
1 |
Ez series (HACH) |
Spectrophotometry (flow) |
25/106 |
0.005 |
0.025–100 (different ranges) |
−/2% |
Yes |
Yes |
High |
— |
The proposed prototype overcomes the presented drawbacks, showing the best balance in terms of cost, portability, linear range, accuracy and repeatability, without the need for sample pretreatment, with the absence of interfering compounds and showing a high level of automation and autonomy.
Conclusions
There are some commercial ammonium analyzers which meet some specific requirements for TAN analysis in a particular type of water (drinking water, fresh water or urban or industrial wastewater). However they are expensive and still lack enough portability, automation, autonomy, versatility and selectivity as a whole to be adapted to any type of water source, situation and TAN concentration, without prior sample treatments.
We focused the present work on addressing these issues, procuring a low cost and highly versatile and portable analyzer prototype for the in situ measurement of TAN, which fulfils features such as autonomy, automation and low sample and reagent consumption, thus enabling continuous monitoring with low maintenance requirements. Miniaturization of the equipment also aligns with a greener chemistry with the goal of generating less waste. Moreover, the system operates in a wide or adaptable working range to face different types of water sources with even complex matrices, providing reliable results in real time. Through different automatic sequences of operation, a total linear range from 0.08 to 1000 mg L−1 NH4+ and a detection limit of 0.03 mg L−1 can be achieved, with high repeatability and reproducibility. In addition, by means of the integration of an automatic gas-diffusion stage, all the potential interferences present in the sample matrix are avoided and the lifetime of the electrode, and consequently that of the global system, is considerably extended because the sample matrix is not in contact with the ISE membrane.
The whole system is comparable in size to a shoe box and its portability, along with the rest of the mentioned characteristics, constitutes the differential features and substantial improvement with respect to existing commercial equipment for TAN determination. In addition, the analysis of water samples and the simulation of TAN monitoring in a wastewater treatment plant have been carried out obtaining very good results. This demonstrates the robustness of the analyzer prototype in a real operating environment and its transfer potential to the real world for algae growth control, the verification of the proper operation of nitrification processes in water treatment plants and safety assessments in drinking water, among other water quality analyses according to sustainable development.
Author contributions
The manuscript was written through contributions of all authors. All authors have given approval to the final version of the manuscript.
Conflicts of interest
There are no conflicts to declare.
Acknowledgements
The authors are grateful to Evonik Industries and the MELiSSA project pilot plant for the collaboration in the analyzer validation process providing samples and contrast analysis. The authors are grateful to the Spanish Ministry of Science and Innovation and the Catalan government for their financial support through the projects CTQ2012-36165, CTQ2017-85011-R and PID2020-117216RB-I00, co-funded by FEDER (Fondo Europeo de Desarrollo Regional), and 2021SGR00124.
References
- X. Wang, J. Li, J. Chen, L. Cui, W. Li and X. Gao,
et al., Water quality criteria of total ammonia nitrogen (TAN) and un-ionized ammonia (NH3-N) and their ecological risk in the Liao River, China, Chemosphere, 2020, 243 Search PubMed
.
-
World Health Organization, Ammonia in Drinking-water. Background document for development of WHO Guidelines for Drinking-water Quality [Internet], Guidelines for drinking-water quality, 2003, Available from: https://www.nap.edu/catalog/9038.html.
- Y. Xiao, Y. Zheng, S. Wu, Z.-H. Yang and F. Zhao, Nitrogen recovery from wastewater using microbial fuel cells, Front. Environ. Sci. Eng., 2016, 10(1), 185–191 CrossRef CAS
, Available from: https://link.springer.com/10.1007/s11783-014-0730-5.
- L. O. Šraj, M. I. G. S. Almeida, S. E. Swearer, S. D. Kolev and I. D. McKelvie, Analytical challenges and advantages of using flow-based methodologies for ammonia determination in estuarine and marine waters, TrAC, Trends Anal. Chem., 2014, 59, 83–92 CrossRef
, Available from: https://linkinghub.elsevier.com/retrieve/pii/S0165993614000843.
-
G. Carty, G. O'Leary and B. Meaney, Wastewater Treatment Manuals. Primary, secondary and tertiary treatement, Environmental Protection Agency, Ireland, 1997, p. 131 Search PubMed
.
- A. Stenholm, E. Eriksson, O. Lind and B. Wigilius, Comparison of continuous flow analysis including photometric detection and ion-selective electrode potentiometry for the measurement of ammonium nitrogen in wastewater, Int. J. Environ. Anal. Chem., 2008, 88(3), 165–176 CrossRef CAS
.
-
World Health Organization, Monochloramine in Drinking-water, Background document for development of WHO Guidelines for Drinking-water Quality [Internet], Guidelines for drinking-water quality, 2004, Available from: https://www.who.int/water_sanitation_health/dwq/chemicals/en/monochloramine.pdf.
- B. Hindfelt, F. Plum and T. E. Duffy, Effect of acute ammonia intoxication on cerebral metabolism in rats with portacaval shunts, J. Clin. Invest., 1977, 59(3), 386–396 CrossRef CAS PubMed
.
- P. Monfort, E. Kosenko, S. Erceg, J. J. Canales and V. Felipo, Molecular mechanism of acute ammonia toxicity: Role of NMDA receptors, Neurochem. Int., 2002, 41, 95–102 CrossRef CAS PubMed
.
- A. Cerda, M. T. Oms, R. Forteza and V. Cerda, Evaluation of Flow-Injection Methods for Ammonium Determination in Waste-Water Samples, Anal. Chim. Acta, 1995, 311(2), 165–173 CrossRef CAS
.
-
J. Carrera, Biological elimination of nitrogen in an high load effluent, Doctoral thesis, Universitat Autònoma de Barcelona, 2001 Search PubMed
.
- B. Gaber, Bio-removal of nitrogen from wastewater-A review, Nat. Sci., 2010, 8(12), 210–228 Search PubMed
.
-
Official journal of the european communities, 91/271/EEC of 21 May 1991 concerning urban waste-water treatment, EEC Council Directive, 1991 Search PubMed
.
- Consortium for the defense of the basin of Besòs river, Regulation of wastewater discharges, 1994.
- S. M. Oliveira, T. I. M. S. Lopes, I. V. Tóth and A. O. S. S. Rangel, A multi-commuted flow injection system with a multi-channel propulsion unit placed before detection: Spectrophotometric determination of ammonium, Anal. Chim. Acta, 2007, 600(1–2 SPEC. ISS), 29–34 CrossRef CAS PubMed
.
- H. L. Braz, D. T. Ito, J. a. F. da Silva, C. L. do Lago and J. J. Pedrotti, Trace levels determination of ammonium by flow injection analysis using gas-diffusion and capacitively coupled contactless conductivity detection, Electroanalysis, 2011, 23(11), 2594–2600 CrossRef CAS
.
- C. Henríquez, B. Horstkotte and V. Cerdà, Conductometric determination of ammonium by a multisyringe flow injection system applying gas diffusion, Int. J. Environ. Anal. Chem., 2013, 93(12), 1236–1252, DOI:10.1080/03067319.2012.746322
.
- H. Shen, T. J. Cardwell and R. W. Cattrall, Determination of Ammonia in Waste Waters by a Differential pH Method Using Flow Injection Potentiometry and a Nonactin-Based Sensor, Analyst, 1997, 122, 89–93 RSC
.
- J. L. F. C. Lima, A. O. S. S. Rangel and M. R. S. Souto, Flow injection system with gas diffusion for the sequential determination of total nitrogen and phosphorus in vegetables, Fresenius' J. Anal. Chem., 1997, 358(5), 657–662, DOI:10.1007/s002160050486
.
- S. Alegret, J. Alonso, J. Bartroli and E. Martinez-Fabregas, Flow Injection System for On-line Potentiometric Monitoring of Ammonia in Freshwater Streams, Analyst, 1989, 114, 1443–1447 RSC
.
- P. B. Martelli, J. G. Neto, E. A. G. Zagatto, S. M. B. Brienza, M. C. B. S. M. Montenegro and J. L. F. C. Lima, Sequential analyte removal in flow analysis: determination of nitrogen, phosphorus and potassium in fertilizers, Anal. Chim. Acta, 1995, 317(1–3), 239–245 CrossRef
.
- T. Aoki, S. Uemura and M. Munemori, Continuous flow method for simultaneous determination of nitrate and ammonia in water, Environ. Sci. Technol., 1986, 20(5), 515–517 CrossRef CAS PubMed
.
- S. M. Oliveira, T. I. Marques da Silva Lopes, I. V. Tóth and A. O. S. S. Santos Silva Rangel, Determination of ammonium in marine waters using a gas diffusion multicommuted flow injection system with in-line prevention of metal hydroxides precipitation, J. Environ. Monit., 2009, 11(1), 228–234 RSC
, Available from: https://xlink.rsc.org/?DOI=B812624K.
- S. M. Gray, P. S. Ellis, M. R. Grace and I. D. McKelvie, Spectrophotometric Determination of Ammonia in Estuarine Waters by Hybrid Reagent Injection Gas Diffusion Flow Analysis, Spectrosc. Lett., 2006, 39(919083124), 737–753 CrossRef CAS
.
- R. F. Thomas and R. L. Booth, Selective electrode measurement of ammonia in water and wastes, Environ. Sci. Technol., 1973, 7(6), 523–526, DOI:10.1021/es60078a006
.
- W. Frenzel and C.-Y. Liu, Potentiometric and conductometric determination of ammonium by gas-diffusion flow injection analysis, Fresenius' J. Anal. Chem., 1992, 276–280 CrossRef CAS
, Available from: https://www.springerlink.com/index/K4J6152031XV7350.pdf.
- A. Calvo-López, O. Ymbern, M. Puyol, J. M. Casalta and J. Alonso-Chamarro, Potentiometric analytical microsystem based on the integration of a gas-diffusion step for on-line ammonium determination in water recycling processes in manned space missions, Anal. Chim. Acta, 2015, 874, 26–32 CrossRef PubMed
, Available from: https://www.sciencedirect.com/science/article/pii/S0003267014014767.
- H. Becker and L. E. Locascio, Polymer microfluidic devices, Talanta, 2002, 56(2), 267–287 CrossRef CAS PubMed
, Available from: https://www.ncbi.nlm.nih.gov/pubmed/18968500.
- D.-S. Lee, H. Yang, K.-H. Chung and H.-B. Pyo, Wafer-scale fabrication of polymer-based microdevices via injection molding and photolithographic micropatterning protocols, Anal. Chem., 2005, 77(16), 5414–5420 CrossRef CAS PubMed
, Available from: https://www.ncbi.nlm.nih.gov/pubmed/16097789.
- M. Sequeira, M. Bowden, E. Minogue and D. Diamond, Towards autonomous environmental monitoring systems, Talanta, 2002, 56(2), 355–363 CrossRef CAS PubMed
, Available from: https://www.ncbi.nlm.nih.gov/pubmed/18968507.
- H. Becker and C. Gärtner, Polymer microfabrication technologies for microfluidic systems, Anal. Bioanal. Chem., 2008, 390(1), 89–111 CrossRef CAS PubMed
.
- O. Ymbern, M. Berenguel-Alonso, A. Calvo-López, S. Gómez-de Pedro, D. Izquierdo and J. Alonso-Chamarro, Versatile Lock and Key Assembly for Optical Measurements with Microfluidic Platforms and Cartridges, Anal. Chem., 2015, 87(3), 1503–1508, DOI:10.1021/ac504255t
.
- O. Ymbern, N. Sández, A. Calvo-López, M. Puyol and J. Alonso-Chamarro, Gas diffusion as a new fluidic unit operation for centrifugal microfluidic platforms, Lab Chip, 2014, 14(5), 1014–1022 RSC
.
- S. Wolff, L. Coelho, I. Karoliussen and A.-I. Jost, Effects of the Extraterrestrial Environment on Plants: Recommendations for Future Space Experiments for the MELiSSA Higher Plant Compartment, Life, 2014, 4(2), 189–204 CrossRef PubMed
, Available from: https://www.mdpi.com/2075-1729/4/2/189/.
- A. Calvo-López, B. Rebollo-Calderon, A. Ormazábal, R. Artuch, J. Rosell-Ferrer and J. Alonso-Chamarro,
et al., Biomedical point-of-care microanalyzer for potentiometric determination of ammonium ion in plasma and whole blood, Anal. Chim. Acta, 2022, 1205, 339782 CrossRef PubMed
.
- A. Calvo-López, O. Ymbern, M. Puyol and J. Alonso-Chamarro, Soluble reactive phosphorous determination in wastewater treatment plants by automatic microanalyzers, Talanta, 2021, 221, 121508 CrossRef PubMed
, Available from: https://www.sciencedirect.com/science/article/pii/S0039914020307992.
- N. Sández, A. Calvo-López, S. S. M. P. Vidigal, A. O. S. S. Rangel and J. Alonso-Chamarro, Automated analytical microsystem for the spectrophotometric monitoring of titratable acidity in white, rosé and red wines, Anal. Chim. Acta, 2019, 1091, 50–58 CrossRef PubMed
, Available from: https://www.sciencedirect.com/science/article/pii/S0003267019311377.
- Z. M. da Rocha, C. S. Martinez-Cisneros, A. C. Seabra, F. Valdés, M. R. Gongora-Rubio and J. Alonso-Chamarro, Compact and autonomous multiwavelength microanalyzer for in-line and in situ colorimetric determinations, Lab Chip, 2012, 12(1), 109 RSC
.
- A. Calvo-López, M. Puyol, J. M. Casalta and J. Alonso-Chamarro, Multi-parametric polymer-based potentiometric analytical microsystem for future manned space missions, Anal. Chim. Acta, 2017, 995, 77–84 CrossRef PubMed
.
- E. Lindner and Y. Umezawa, Performance evaluation criteria for preparation and measurement of macro- and microfabricated ion-selective electrodes (IUPAC Technical Report), Pure Appl. Chem., 2008, 80(1), 85–104 CrossRef CAS
.
|
This journal is © The Royal Society of Chemistry 2023 |
Click here to see how this site uses Cookies. View our privacy policy here.