DOI:
10.1039/D2SC05143E
(Edge Article)
Chem. Sci., 2022,
13, 14285-14291
Fluorine extraction from organofluorine molecules to make fluorinated clusters in yttrium MOFs†
Received
14th September 2022
, Accepted 22nd November 2022
First published on 26th November 2022
Abstract
A new rare earth based two-dimensional coordination network and a three-dimensional metal–organic framework (MOF) have been synthesized using bicinchoninic acid (BCA) and yttrium(III) ions. Yttrium dimer nodes are formed in the absence of a modulator, resulting in a 2D layered coordination network (Y–BCA-2D). The presence of fluorinating agents, e.g., 2-fluorobenzoic acid (2-FBA), 2,6-difluorobenzoic acid (2,6-DFBA), and perfluorohexanoic acid (PFHxA) result in μ3-F bridged metal hexaclusters (Y6F8) that form a three-dimensional MOF (Y–BCA-3D). It was found that Y3+ can break highly stable C–F bonds in aromatic and aliphatic fluorinated compounds. Single-crystal X-ray diffraction (SC-XRD) shows the presence of fluorine in the metal cluster which was confirmed by energy dispersive X-ray spectroscopy (EDS). High resolution X-ray photoelectron spectroscopy (XPS) and 19F Nuclear Magnetic Resonance (NMR) also verify the presence of metal–fluorine bonds in the cluster. The Y–BCA-3D MOF selectively adsorbs CO2 but not N2.
Introduction
Metal–organic frameworks (MOFs) are crystalline hybrid organic–inorganic compounds that have been used in a variety of applications including conductive materials,1–3 catalysis,4–9 drug delivery,10 radio therapy,11–13 water harvesting,14,15 gas storage, and separations.16–19 There is growing interest in rare-earth (RE) MOFs, particularly due to their high coordination number (CN: 6–12), magnetic properties, optical properties, and therapeutic applications.20–25 The RE metal ions tend to form dimers that often result in MOFs with low porosity.26–28 It has been widely reported that the addition of a modulator can result in RE-MOFs with larger metal clusters.29–32 Modulators were originally designed to bind to metal ions to control crystallization rates, often leading to larger X-ray quality crystals.33,34 A popular modulator is 2-fluorobenzoic acid (2-FBA) (Fig. 1).35–38 This molecule has been used to synthesize RE based MOFs where the metal nodes have been reported to be hydroxo (μ3-OH−) bridged hexanuclear clusters.35–54 In contrast, it was found that in the case of Ho3+ and terephthalic acid (BDC), the addition of 2-FBA to the synthesis resulted in a fluoro-bridged hexanuclear cluster in a UiO-66 analog.55 Additionally, it was found that Ho3+ could extract fluorine from 2-FBA and form HoF3. The presence of fluorine in metal clusters can also improve their thermodynamic stability compared to hydroxy bridged clusters.56 Fluorinated metal clusters are hydrophobic and can improve the water stability of the MOFs.57 The fluorine in the clusters may improve the framework interaction with CO2 that can be exploited in separations. For example, the Y-MOF in a recent mixed matrix membrane that showed enhanced CO2 separation is likely a fluorinated MOF.55,58 Fluorinated RE clusters exhibit enhanced photoluminescence which may also be the case in fluorinated RE-MOFs.59
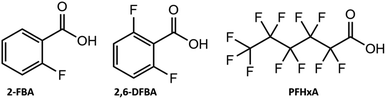 |
| Fig. 1 Structures of 2-fluorobenzoic acid (2-FBA), 2,6-difluorobenzoic acid (2,6-DFBA), and perfluorohexanoic acid (PFHxA). | |
Perfluoroalkyl and polyfluoroalkyl substances (PFAS) are highly stable compounds used in a variety of coating applications, such as heat-resistant non-stick utensils, hydrophobic packaging, adhesives, and furniture surfacing.60,61 These compounds are remarkably stable under high temperatures, extreme chemical conditions, and in both hydrophilic and hydrophobic environments.62 The aliphatic C–F bonds are very stable with a bond dissociation energy exceeding 500 kJ mol−1.63,64 According to the Centers for Disease Control and Prevention (CDC) and the United States Environmental Protection Agency (EPA), PFAS contamination in soil and drinking water are becoming a serious environmental threat.60,65 In this study, it is found that Y3+ can break C–F bonds in organo-fluorine molecules including perfluorohexanoic acid (PFHxA) and form YF3 or fluoro-bridged hexaclusters in a new MOF, Y–BCA-3D.
A fluorine-bridged trinuclear cluster was formed with the organic linker [2,2′-bipyridine]-4,4′-dicarboxylic acid (BPDC) (Fig. 2).55 In the present work, the linker BCA (Fig. 2) was used to make a new MOF using Y3+. Similar to Ho3+, the Y3+ ion extracts fluorine from 2-FBA, 2,6-DFBA, as well as PFHxA (Fig. 1). In the absence of an organo-fluorine molecule, a new two-dimensional MOF (Y–BCA-2D) was formed. The 2D and 3D MOFs were characterized by thermogravimetric analysis (TGA), EDS, XPS, XRD, 19F-NMR and gas sorption analysis (N2, CO2).
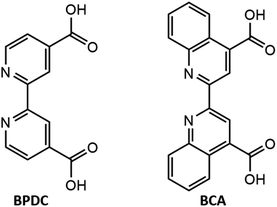 |
| Fig. 2 Structures of organic linkers [2,2′-bipyridine]-4,4′-dicarboxylic acid (BPDC), and bicinchoninic acid (BCA). | |
Results and discussion
In the absence of fluorinated modulators, the solvothermal reaction of Y(NO3)3·6(H2O) with the BCA ligand yielded a two-dimensional MOF, [Y2(μ4-BCA)2(μ3-BCA)4(DMF)4]n·nDMF·n(H2O) (Y–BCA-2D). Single-crystal XRD (SC-XRD) analysis reveals that Y–BCA-2D crystalizes into a triclinic space group, P
. The Y3+ has a coordination number of nine in the Y–BCA-2D dimer node. In the structure of Y–BCA-2D, inversion centers lie at the center of the Y-based binuclear clusters as shown in Fig. 3(a). In these binuclear clusters, each Y center is coordinated to two terminal DMF molecules by the carbonyl oxygen atoms and eight carboxylate groups from the BCA ligands. There are two types of chemically distinct BCA ligands in the structure, namely type-1 and type-2 as shown in Fig. S1(a).† Each type-1 BCA ligand has one η2-carboxylate group chelating to a Y3+ ion and another μ2, η3-carboxylate group bridging the Y3+ ions in the binuclear clusters. Type-2 BCA ligands are geometrically perpendicular to the type-1 BCA ligands. Each BCA linker is bound to four different Y3+ ions from two different binuclear clusters by its two bridging carboxylate groups. In the extended structure of Y–BCA-2D, two type-1 BCA ligands pair up and align in an antiparallel fashion, connecting two adjacent binuclear clusters and forming an infinite chain as shown in Fig. 3(b). Perpendicular to this chain, type-2 BCA ligands forms another infinite chain while connecting the binuclear clusters in proximity. Topologically, the two perpendicular chains generate a two-dimensional 4-c net with the sql topology as shown in the Fig. 3(c). The 2D layers are aligned in a staggered configuration with the clusters being on top of the type-2 BCA ligands from an adjacent layer (Fig. S1†). The staggering alignment of these layers leads to elimination of pore space with a minimal Platon void percentage of 3%. Between each layer, there are DMF, and water molecules as shown in Fig. S1.† The crystal packing of the 2-dimensional layers is further highlighted in Fig. S2,† where alternate layers are colored green and purple, and solvent molecules are removed for clarity. The phase purity of the product was confirmed by powder XRD (Fig. S6†).
 |
| Fig. 3 Structure of Y–BCA-2D (a) cluster structure with metal-dimer node, (b) layered structure, and (c) topological representation showing a single layer and stacking. | |
In the presence of fluorinated modulators (2-FBA, 2,6-DFBA, PFHxA), the solvothermal reaction of Y(NO3)3·6(H2O) with the BCA ligand yielded a three-dimensional MOF (Y–BCA-3D) with an empirical formula of C30H15F2N3O6.62Y1.5. Y–BCA-3D crystalized in the tetragonal space group I4/m. The crystal structure of Y–BCA-3D consists of yttrium hexanuclear clusters coordinated by BCA linkers. Yttrium has the same coordination number (i.e., CN: 9) as in the dimer, but the μ3-F bridges form a hexanuclear cluster (Y6F8), as shown in Fig. 4(a and b). Each hexanuclear cluster is connected to BCA linkers via η2-carboxylate groups. Each Y3+ ion is coordinated with one water molecule, four μ3-F, and four oxygen atoms from carboxylate groups. The extended network shows that Y–BCA-3D has the same fcu topology as the UiO-66 Fig. 4(c and d).66 Details of the crystallographic data collection and instrumentation are discussed in the ESI† as well as the crystal parameters, and refinement details are given in Table S1.† The powder XRD confirms the crystallinity and bulk purity of the product when compared to the simulated pattern from the single crystal structure (Fig. S6†).
 |
| Fig. 4 Crystal structure of Y–BCA-3D (a) cluster structure with hexanuclear node, (b) c-axis view of hexacluster and isolated Y6F8 cluster, (c) extended 3D network view along the a-axis showing 6.3 Å spherical cavities (purple), and (d) underlying net topology of the MOF viewed along the a-axis. | |
The formation of these μ3-F bridged hexaclusters depends on the nature of the modulator and the ratio with the BCA linker. It was found that 2-FBA gave a mixture of 2D/3D MOFs if used in a modulator/BCA mole ratio less than 15. While the same ratios of 2,6-DFBA and PFHxA only form the hexaclusters. This could reflect the lower pKa of BCA (1.77), which competes with the modulator for the Y3+ ions. The pKa values for the modulators are 3.27, 2.34, and −0.15 for 2-FBA, 2,6-DFBA, and PFHxA, respectively. The ideal linker to modulator mole ratios were found as 1
:
18, 1
:
14, and 1
:
7 for 2-FBA, 2,6-DFBA, and PFHxA, respectively. It should be noted that an excess amount of modulator in all cases resulted in a mixture of YF3 and Y–BCA-3D. Therefore, the donor strength of the modulator versus the linker must be taken into careful consideration when designing fluoro-bridged RE-MOFs. Attempts to use NaF and NH4F as the fluorine source in the synthesis of Y–BCA-3D only resulted in formation of yttrium fluorides. Moreover, attempts to convert the 2D MOF to the 3D MOF by heating with organofluorine modulators only resulted in formation of YF3.
The EDS elemental mapping confirms the presence of fluorine in the Y–BCA-3D. The EDS of Y–BCA-3D synthesized with different fluorinated modulators show that the amount of fluorine is consistent with the theoretically calculated amount (Fig. S3–S5†). Fluorine nuclear magnetic resonance (19F-NMR) spectroscopy was used to confirm the presence of fluorine in the MOFs. Three different samples of Y–BCA-3D synthesized using different fluorinated modulators i.e., 2-FBA, 2,6-DFBA, and PFHxA were digested in 10% deuterated sulfuric acid. A comparison of 19F-NMR spectra for modulators and digested Y–BCA-3D MOFs is shown in Fig. S7–S9.†19F-NMR spectra of acid digested Y–BCA-3D confirmed that there were no trapped modulators inside the MOF pores, as the fluorine chemical shifts do not match the modulators. The chemical shift peaks at −169 ppm correspond to hydrofluoric acid (HF) formed by the release of bridging fluorine from the digested MOF, as these peaks are in the same region as the reported 19F-NMR peak for HF.67,68
It should be noted that the only source of fluorine was the organo-fluorine molecules (2-FBA, 2,6-DFBA, or PFHxA). It was confirmed that the fluorine in the Y–BCA-3D is present in the metal nodes, and not unreacted or trapped organo-fluorine molecules. As a proof of fluorine extraction, a reaction of just the modulator and Y3+ was carried out without the organic linker. The Y3+ can extract fluorine from 2-FBA, 2,6-DFBA and PFHxA to make YF3 (Fig. S10†). The formation of YF3 was confirmed by comparing the powder XRD with its simulated pattern, and no impurities were found (Fig. S11†).69
The presence of elemental chemical states can be precisely determined by the XPS. The survey spectra of the 2D and 3D MOFs shown in Fig. 5(a and c) confirms the presence of yttrium, fluorine, and BCA linker elements i.e., carbon, nitrogen, and oxygen. The high-resolution spectrum of Y–BCA-3D gave spin–orbit coupled peaks of Y 3d5/2 and 3d3/2 at 158.2 eV and 160.2 eV, respectively. The Y–BCA-2D shows a binding energy (BE) of Y 3d5/2 at 157.4 eV. As, Y–BCA-2D only has yttrium–oxygen bonds, similar to Y2O3.70 Whereas, the BE of Y 3d5/2 in YF3 is 159 eV due to Y–F bonds. In the case of Y–BCA-3D, these energies correspond to yttrium bound to oxygen and fluorine (O–Y–F) in the hexacluster. Hence, the Y 3d5/2 BE in the hexacluster is in between the Y2O3 and YF3.
 |
| Fig. 5 The XPS spectra of (a) Y–BCA-3D survey, (b) Y–BCA-3D high resolution Y 3d5/2 and 3d3/2, (c) Y–BCA-2D survey, and (d) Y–BCA-2D high resolution Y 3d5/2 and 3d3/2. | |
The F 1s high resolution XPS spectra show the BE for C–F in 2-FBA, 2,6-DFBA and PFHxA as 686.7, 687.5, and 689.2 eV, respectively (Fig. 6(a–c)). Whereas the Y–BCA-3D shows a peak at 684.7 eV, which corresponds to fluorine bound to yttrium. This can be compared to YF3 at 685.1 eV for F 1s. The F 1s spectrum for a physical mixture of Y–BCA-3D and 2,6-DFBA was recorded. Fig. 6(f) shows that the mixture has two distinct chemical states of fluorine with binding energies at 684.7 and 687.5 eV for Y–F and C–F, respectively. Hence, it can be concluded that the fluorine is bound to yttrium in the hexacluster, and no free organic fluorine is present in the Y–BCA-3D.
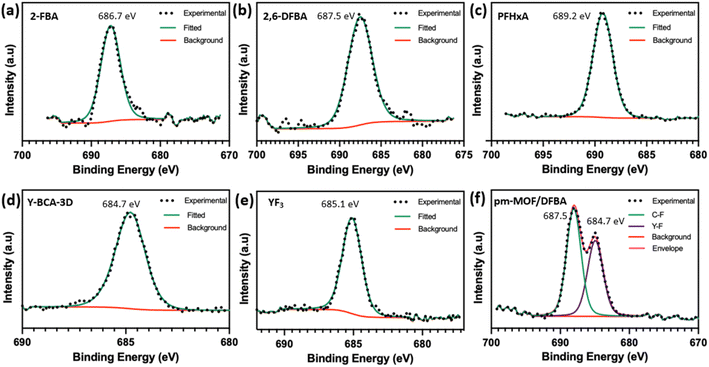 |
| Fig. 6 High resolution F 1s XPS spectra of (a) 2-FBA, (b) 2,6-DFBA, (c) PFHxA, (d) Y–BCA-3D, (e) YF3, and (f) physical mixture of Y–BCA-3D and 2,6-DFBA. | |
Thermogravimetric analysis of the MOFs activated at 160 °C under vacuum shows that the 2D-MOF decomposes around 460 °C in air and at 500 °C under a nitrogen atmosphere. Whereas the pristine BCA linker decomposes at 300 °C under air (Fig. S12†). The Y–BCA-2D structure loses 5% mass around 100 °C and is assigned to the loss of adsorbed water. A further 5% mass loss at 300 °C is assigned to coordinated DMF. Similarly, the 3D-MOF also has superior stability in comparison to the BCA linker and stable up to 450 °C. It gave a 7% weight loss at 100 °C for trapped water molecules, which show that it has relatively more free volume in comparison to Y–BCA-2D.
The porosity of the MOFs was probed using ultra high purity nitrogen and carbon dioxide. The nitrogen adsorption–desorption analysis shows that the activated Y–BCA-2D is a densely packed material with a very low BET surface area of 11.5 m2 g−1. Whereas, the CO2 adsorption data shows a surface area of 33.1 m2 g−1 that indicates the presence of some microporosity. Similarly, the nitrogen adsorption isotherm for Y–BCA-3D MOF shows a surface area of 5.7 m2 g−1 and 94.5 m2 g−1 for the CO2 adsorption isotherm. The surface area for the similar linker i.e., BPDC (Fig. 2) in a Gd-trinuclear cluster-based MOF is 177.7 m2 g−1. This shows that the Y–BCA-3D has less surface area, but it is selective for CO2 uptake due to its narrow pore openings. Even though Fig. 7(c) show that Y–BCA-3D has 6.3 Å spherical cavities, bigger than the kinetic diameter of nitrogen (3.64 Å), it does not allow N2 adsorption. The calculated void spaces show that the channels are zigzag along a and b-axes (Fig. 7(c–e)). These channels have narrow openings ∼4 Å along the a and b-axes and have deep spherical cage type 6.3 Å cavities along c-direction. These cavities dead end along the c-axis but are accessible through the a and b-axes. This was experimentally verified by the pore volume calculated by gas sorption data as shown in Fig. 7(a and b).
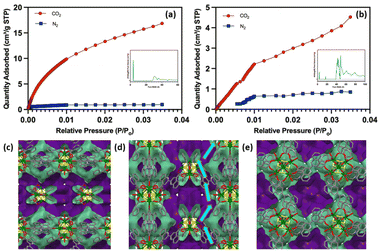 |
| Fig. 7 Adsorption isotherms for (a) Y–BCA-3D and (b) Y–BCA-2D. Representation of sinusoidal voids/channels (purple) in Y–BCA-3D (c) viewed along a-axis, (d) viewed along b-axis and gas passage is highlighted by arrows, and (e) viewed along c-axis show presence of deep spherical cavities with a diameter of 6.3 Å. | |
Conclusions
The extraction of fluorine from various organo-fluorine compounds by rare earth metal ions has opened up a new area for MOFs synthesis. A new yttrium MOF with the BCA linker features a fluorine bridged hexacluster. The Y3+ ions can extract fluorine from aromatic and aliphatic carbons. This work is currently being extended to other rare earth metal ions, as different RE metals exhibit various fluorine affinities. Understanding how the fluorine affinities play a role in the cluster formation can provide insights into the design of these MOFs as well as their potential application in fluorine sensing and extraction. The fluorine bridges found in these clusters are expected to have an effect on the optical properties of the MOFs, and those studies are in progress.
Data availability
CCDC numbers 2206798 (Y–BCA-2D), and 2207094 (Y-3D-MOF). Additional experimental details and data are provided in the ESI,† including NMR, EDS, TGA, PXRD, optical images, and crystallographic data.
Author contributions
KJB and MA conceived and designed the project. MA performed experiments, analyzed the materials by PXRD, XPS, TGA, SEM, gas sorption, and wrote the original draft. AMM helped in setting up reactions carried PXRD and edited draft. HRF carried NMR studies. ZX, HDA and YS carried out SC-XRD analysis, HZ and KJB provided resources. KJB funding acquisition, conceptualization, review and editing, supervision, and project administration. All authors have given approval to the final version of the manuscript.
Conflicts of interest
The authors declare no competing financial interest.
Acknowledgements
The authors thank the Robert A. Welch Foundation (AT-1153) for supporting this research. This project also was partially funded by The University of Texas at Dallas Office of Research through the Core Facility Voucher Program (10319). In addition, the authors acknowledge the Texas A&M X-ray Diffraction Laboratory.
References
- J. Y. Choi, J. Flood, M. Stodolka, H. T. B. Pham and J. Park, ACS Nano, 2022, 16, 3145–3151 CrossRef CAS PubMed
.
- G. Skorupskii, K. N. Le, D. L. M. Cordova, L. Yang, T. Chen, C. H. Hendon, M. Q. Arguilla and M. Dincă, Proc. Natl. Acad. Sci. U. S. A., 2022, 119, e2205127119 CrossRef CAS PubMed
.
- M. de Lourdes Gonzalez-Juarez, C. Morales, J. I. Flege, E. Flores, M. Martin-Gonzalez, I. Nandhakumar and D. Bradshaw, ACS Appl. Mater. Interfaces, 2022, 14, 12404–12411 CrossRef CAS PubMed
.
- L. Zhu, X.-Q. Liu, H.-L. Jiang and L.-B. Sun, Chem. Rev., 2017, 117, 8129–8176 CrossRef CAS PubMed
.
- M. Ahsan Usman, M. Naeem, M. Saeed and M. Zaheer, Inorg. Chim. Acta, 2021, 521, 120305 CrossRef CAS
.
- X. Feng, Y. Song, J. S. Chen, Z. Li, E. Y. Chen, M. Kaufmann, C. Wang and W. Lin, Chem. Sci., 2019, 10, 2193–2198 RSC
.
- J. Huo, J. Aguilera-Sigalat, S. El-Hankari and D. Bradshaw, Chem. Sci., 2015, 6, 1938–1943 RSC
.
- Z. Y. Gu, J. Park, A. Raiff, Z. Wei and H. C. Zhou, ChemCatChem, 2014, 6, 67–75 CrossRef CAS
.
- M. Stodolka and J. Park, ACS Cent. Sci., 2022, 8, 877–879 CrossRef CAS PubMed
.
- Y. Chen, P. Li, J. A. Modica, R. J. Drout and O. K. Farha, J. Am. Chem. Soc., 2018, 140, 5678–5681 CrossRef CAS PubMed
.
- H. D. Lawson, S. P. Walton and C. Chan, ACS Appl. Mater. Interfaces, 2021, 13, 7004–7020 CrossRef CAS PubMed
.
- K. Ni, G. Lan, Y. Song, Z. Hao and W. Lin, Chem. Sci., 2020, 11, 7641–7653 RSC
.
- K. Ni, Z. Xu, A. Culbert, T. Luo, N. Guo, K. Yang, E. Pearson, B. Preusser, T. Wu, P. la Riviere, R. R. Weichselbaum, M. T. Spiotto and W. Lin, Nat. Biomed. Eng., 2022, 6(2), 144–156 CrossRef CAS PubMed
.
- M. J. Kalmutzki, C. S. Diercks and O. M. Yaghi, Adv. Mater., 2018, 30, 1704304 CrossRef PubMed
.
- H. Furukawa, F. Gándara, Y.-B. Zhang, J. Jiang, W. L. Queen, M. R. Hudson and O. M. Yaghi, J. Am. Chem. Soc., 2014, 136, 4369–4381 CrossRef CAS PubMed
.
- H. C. Zhou, J. R. Long and O. M. Yaghi, Chem. Rev., 2012, 112, 673–674 CrossRef CAS PubMed
.
- K. Chen, S. H. Mousavi, R. Singh, R. Q. Snurr, G. Li and P. A. Webley, Chem. Soc. Rev., 2022, 51, 1139–1166 RSC
.
- Z. M. Schulte, Y. H. Kwon, Y. Han, C. Liu, L. Li, Y. Yang, A. G. Jarvi, S. Saxena, G. Veser, J. K. Johnson and N. L. Rosi, Chem. Sci., 2020, 11, 12807–12815 RSC
.
- X. Zhao, B. Xiao, A. J. Fletcher, K. M. Thomas, D. Bradshaw and M. J. Rosseinsky, Science, 2004, 306, 1012–1015 CrossRef CAS PubMed
.
- F. Saraci, V. Quezada-Novoa, P. R. Donnarumma and A. J. Howarth, Chem. Soc. Rev., 2020, 49, 7949–7977 RSC
.
- V. Trannoy, A. N. Carneiro Neto, C. D. S. Brites, L. D. Carlos, H. Serier-Brault, V. Trannoy, H. Serier-Brault, A. N. C. Neto, C. D. S. Brites and L. D. Carlos, Adv. Opt. Mater., 2021, 9, 2001938 CrossRef CAS
.
- A. Kourtellaris, W. Lafargue-Dit-Hauret, F. Massuyeau, C. Latouche, A. J. Tasiopoulos and H. Serier-Brault, Adv. Opt. Mater., 2022, 2200484 CrossRef CAS
.
- I. N'Dala-Louika, D. Ananias, C. Latouche, R. Dessapt, L. D. Carlos and H. Serier-Brault, J. Mater. Chem. C, 2017, 5, 10933–10937 RSC
.
- G. Skorupskii, K. N. Le, D. L. M. Cordova, L. Yang, T. Chen, C. H. Hendon, M. Q. Arguilla and M. Dincă, Proc. Natl. Acad. Sci. U. S. A., 2022, 119, e2205127119 CrossRef CAS PubMed
.
- T. Feng, Y. Ye, X. Liu, H. Cui, Z. Li, Y. Zhang, B. Liang, H. Li and B. Chen, Angew. Chem., 2020, 132, 21936–21941 CrossRef
.
- J. P. Vizuet, A. L. Lewis, G. T. McCandless and K. J. Balkus, J. Nanosci. Nanotechnol., 2019, 20, 3019–3024 CrossRef PubMed
.
- J. P. Vizuet, A. L. Lewis, G. T. McCandless and K. J. Balkus, Polyhedron, 2019, 159, 12–17 CrossRef CAS
.
- M. L. Mortensen, A. L. Lewis, G. McCandless and K. J. Balkus, Crystals, 2021, 11, 1547 CrossRef CAS
.
- J. I. Deneff, L. E. S. Rohwer, K. S. Butler, N. R. Valdez, M. A. Rodriguez, T. S. Luk and D. F. Sava Gallis, ACS Appl. Mater. Interfaces, 2022, 14, 3038–3047 CrossRef CAS PubMed
.
- N. Wei, R. X. Zuo, Y. Y. Zhang, Z. B. Han and X. J. Gu, Chem. Commun., 2017, 53, 3224–3227 RSC
.
- Y. J. Li, Y. L. Wang and Q. Y. Liu, Inorg. Chem., 2017, 56, 2159–2164 CrossRef CAS PubMed
.
- C. Liu, S. v. Eliseeva, T. Y. Luo, P. F. Muldoon, S. Petoud and N. L. Rosi, Chem. Sci., 2018, 9, 8099–8102 RSC
.
- S. Wannapaiboon, K. Sumida, K. Dilchert, M. Tu, S. Kitagawa, S. Furukawa and R. A. Fischer, J. Mater. Chem. A, 2017, 5, 13665–13673 RSC
.
- R. S. Forgan, Chem. Sci., 2020, 11, 4546–4562 RSC
.
- D. Alezi, A. M. P. Peedikakkal, Ł. J. Weseliński, V. Guillerm, Y. Belmabkhout, A. J. Cairns, Z. Chen, Ł. Wojtas and M. Eddaoudi, J. Am. Chem. Soc., 2015, 137, 5421–5430 CrossRef CAS PubMed
.
- V. Guillerm, Ł. J. Weseliński, Y. Belmabkhout, A. J. Cairns, V. D'Elia, Ł. Wojtas, K. Adil and M. Eddaoudi, Nat. Chem., 2014, 6(8), 673–680 CrossRef CAS PubMed
.
- D. X. Xue, Y. Belmabkhout, O. Shekhah, H. Jiang, K. Adil, A. J. Cairns and M. Eddaoudi, J. Am. Chem. Soc., 2015, 137, 5034–5040 CrossRef CAS PubMed
.
- D. X. Xue, A. J. Cairns, Y. Belmabkhout, L. Wojtas, Y. Liu, M. H. Alkordi and M. Eddaoudi, J. Am. Chem. Soc., 2013, 135, 7660–7667 CrossRef CAS PubMed
.
- G. K. Angeli, E. Loukopoulos, K. Kouvidis, A. Bosveli, C. Tsangarakis, E. Tylianakis, G. Froudakis and P. N. Trikalitis, J. Am. Chem. Soc., 2021, 143, 10250–10260 CrossRef CAS PubMed
.
- N. Panagiotou, F. G. Moscoso, T. Lopes-Costa, J. M. Pedrosa and A. J. Tasiopoulos, Inorg. Chem. Front., 2022, 9, 4850–4863 RSC
.
- T. Xia, W. Cao, L. Guan, J. Zhang, F. Jiang, L. Yu and Y. Wan, Dalton Trans., 2022, 51, 5426–5433 RSC
.
- L. Yu, S. Ullah, K. Zhou, Q. Xia, H. Wang, S. Tu, J. Huang, H. L. Xia, X. Y. Liu, T. Thonhauser and J. Li, J. Am. Chem. Soc., 2022, 144, 3766–3770 CrossRef CAS PubMed
.
- X. L. Lv, L. Feng, L. H. Xie, T. He, W. Wu, K. Y. Wang, G. Si, B. Wang, J. R. Li and H. C. Zhou, J. Am. Chem. Soc., 2021, 143, 2784–2791 CrossRef CAS PubMed
.
- Y. F. Zhang, Q. Wang, D. X. Xue and J. Bai, Inorg. Chem., 2020, 59, 11233–11237 CrossRef CAS PubMed
.
- Z. Duan, Y. Li, X. Xiao, X. Huang, X. Li, Y. Li, C. Zhang, H. Zhang, L. Li, Z. Lin, Y. Zhao and W. Huang, ACS Appl. Mater. Interfaces, 2020, 12, 18715–18722 CrossRef CAS PubMed
.
- F. Hu, Z. Di, M. Wu, M. Hong and J. Li, Cryst. Growth Des., 2019, 19, 6381–6387 CrossRef CAS
.
- Y. Feng, X. Xin, Y. Zhang, B. Guo, F. Li, X. Kong, Y. Wang, X. Wang, Y. Wang, L. Zhang and D. Sun, Cryst. Growth Des., 2019, 19, 1509–1513 CrossRef CAS
.
- H. Jiang, J. Jia, A. Shkurenko, Z. Chen, K. Adil, Y. Belmabkhout, L. J. Weselinski, A. H. Assen, D. X. Xue, M. O'Keeffe and M. Eddaoudi, J. Am. Chem. Soc., 2018, 140, 8858–8867 CrossRef CAS PubMed
.
- L. Zhang, S. Yuan, L. Feng, B. I. Guo, J. U.-S. Qin, B. Xu, C. Lollar, D. Sun, H.-C. Zhou, L.-L. Zhang, B.-B. Guo, B. Xu, D.-F. Sun, S. Yuan, L. Feng, J.-S. Qin, C. Lollar and H.-C. Zhou, Angew. Chem., Int. Ed., 2018, 57, 5095–5099 CrossRef CAS PubMed
.
- D. F. Sava Gallis, J. A. Harvey, C. J. Pearce, M. G. Hall, J. B. DeCoste, M. K. Kinnan and J. A. Greathouse, J. Mater. Chem. A, 2018, 6, 3038–3045 RSC
.
- M. L. Gao, W. J. Wang, L. Liu, Z. B. Han, N. Wei, X. M. Cao and D. Q. Yuan, Inorg. Chem., 2017, 56, 511–517 CrossRef CAS PubMed
.
- A. H. Assen, Y. Belmabkhout, K. Adil, P. M. Bhatt, D. X. Xue, H. Jiang and M. Eddaoudi, Angew. Chem., Int. Ed., 2015, 54, 14353–14358 CrossRef CAS PubMed
.
- R. Luebke, Y. Belmabkhout, Ł. J. Weseliński, A. J. Cairns, M. Alkordi, G. Norton, Ł. Wojtas, K. Adil and M. Eddaoudi, Chem. Sci., 2015, 6, 4095–4102 RSC
.
- D. X. Xue, Y. Belmabkhout, O. Shekhah, H. Jiang, K. Adil, A. J. Cairns and M. Eddaoudi, J. Am. Chem. Soc., 2015, 137, 5034–5040 CrossRef CAS PubMed
.
- J. P. Vizuet, M. L. Mortensen, A. L. Lewis, M. A. Wunch, H. R. Firouzi, G. T. McCandless and K. J. Balkus, J. Am. Chem. Soc., 2021, 143, 17995–18000 CrossRef CAS PubMed
.
- M. S. Christian, K. J. Fritzsching, J. A. Harvey, D. F. Sava Gallis, T. M. Nenoff and J. M. Rimsza, J. Am. Chem. Soc., 2022, 2, 1889–1898 CAS
.
- Q. Li, X. Cai, L. H. Chen, B. bin Guan, Z. L. Fan, W. Zhu and D. X. Xue, Inorg. Chem., 2021, 60, 8143–8153 CrossRef CAS PubMed
.
- P. M. Bhatt, Y. Belmabkhout, A. Cadiau, K. Adil, O. Shekhah, A. Shkurenko, L. J. Barbour and M. Eddaoudi, J. Am. Chem. Soc., 2016, 138, 9301–9307 CrossRef CAS PubMed
.
- B. K. Ling, J. Li, Y. Q. Zhai, H. K. Hsu, Y. T. Chan, W. P. Chen, T. Han and Y. Z. Zheng, Chem. Commun., 2020, 56, 9130–9133 RSC
.
-
Per- and Polyfluorinated Substances (PFAS) Factsheet|National Biomonitoring Program|CDC, https://www.cdc.gov/biomonitoring/PFAS_FactSheet.html, accessed 7 September 2022 Search PubMed.
- M. G. Evich, M. J. B. Davis, J. P. McCord, B. Acrey, J. A. Awkerman, D. R. U. Knappe, A. B. Lindstrom, T. F. Speth, C. Tebes-Stevens, M. J. Strynar, Z. Wang, E. J. Weber, W. M. Henderson and J. W. Washington, Science, 2022, 375(6580) DOI:10.1126/science.abg9065
.
- I. T. Cousins, J. C. Dewitt, J. Glüge, G. Goldenman, D. Herzke, R. Lohmann, C. A. Ng, M. Scheringer and Z. Wang, Environ. Sci.: Processes Impacts, 2020, 22, 2307–2312 RSC
.
- Y. Wen, Á. Rentería-Gómez, G. S. Day, M. F. Smith, T. H. Yan, R. O. K. Ozdemir, O. Gutierrez, V. K. Sharma, X. Ma and H. C. Zhou, J. Am. Chem. Soc., 2022, 144, 11840–11850 CrossRef CAS PubMed
.
- J. T. Moore and C. C. Lu, J. Am. Chem. Soc., 2020, 142, 11641–11646 CrossRef CAS PubMed
.
-
PFAS Explained|US EPA, https://www.epa.gov/pfas/pfas-explained, accessed 7 September 2022 Search PubMed.
- J. H. Cavka, S. Jakobsen, U. Olsbye, N. Guillou, C. Lamberti, S. Bordiga and K. P. Lillerud, J. Am. Chem. Soc., 2008, 130, 13850–13851 CrossRef PubMed
.
- K. O. Christe and W. W. Wilson, J. Fluorine Chem., 1990, 46, 339–342 CrossRef CAS
.
- M. Hudlicky, J. Fluorine Chem., 1985, 28, 461–472 CrossRef CAS
.
- A. Zalkin and D. H. Templeton, J. Am. Chem. Soc., 1953, 75, 2453–2458 CrossRef CAS
.
- K. M. Cole, D. W. Kirk and S. J. Thorpe, Surf. Sci. Spectra, 2020, 27, 024010 CrossRef CAS
.
|
This journal is © The Royal Society of Chemistry 2022 |
Click here to see how this site uses Cookies. View our privacy policy here.