DOI:
10.1039/D3SU00381G
(Tutorial Review)
RSC Sustain., 2024,
2, 1289-1299
The Sea's best kept secret: the use of seaweed as a source of biohydrogen for clean and renewable energy
Received
20th October 2023
, Accepted 21st March 2024
First published on 23rd March 2024
Abstract
Sustainable alternatives to fossil fuels and chemicals are needed. The longer we continue to use petroleum-derived materials, the greater the detrimental effects of climate change. To this end, this tutorial review focuses on the use of macroalgae to produce hydrogen as a green fuel and also through third generation biorefineries to produce a complementary range of high value products compared to land-based feedstocks. Algae are important renewable feedstocks that can afford degradable polymers, bioactive compounds (e.g., polysaccharides and polyphenols) and fuels. They also have the potential to mitigate CO2 emissions by acting as a carbon sink. The aim of this review is to show that different pre-treatment methods and a suite of analytical tools are needed to facilitate effective biohydrogen production from macroalgae. Consideration of pre-treatment methods has not been reviewed previously. We also highlight major challenges to its use including variability between species, environmental conditions, and extraction methods. Understanding macroalgae composition, especially carbohydrate content, is critical in order to optimize biohydrogen and other fuel yields. A variety of screening tools, including mass spectrometric methods, can be used to predict the usefulness of macroalgae species and optimize processing, and thus promote more sustainable research in this field. By optimizing pre-treatment techniques (mechanical, chemical, biological and inhibitor removal), and understanding the composition of algal biomass, hydrogen production from macroalgae can step up and play a role in meeting the world's needs for green energy. Strengths, weaknesses, opportunities and threats to potential seaweed biorefineries are presented.
Sustainability spotlight
Seaweed is cultivated and harvested around the world to be used as a food and in a wide range of products, such as nutritional supplements, food additives (e.g. thickening agents), agrochemicals, and cosmetics. However, there is renewed and growing interest in using seaweeds as a source of renewable materials, whilst capturing CO2via photosynthesis during their growth. One area of exploration is the use of seaweed as a feedstock to produce green hydrogen as a renewable fuel, amongst other biofuels such as bioethanol and biodiesel. This tutorial review highlights research that has been performed in this area. Cultivation and use of seaweed may impact many SDGs including SDG 2 (zero hunger), SDG 7 (affordable and clean energy) and SDG 14 (life below water). New uses of seaweed will also lead to increased opportunities for good employment in rural coastal areas. This is why we ask if seaweed is the sea's best kept secret.
|
Introduction
Seaweed, or macroalgae, is becoming more researched due to its many applications in health, science, and engineering (Fig. 1). Historically, seaweed has been used in agriculture as a fertilizer and in Asian cuisine.1 Along with being a nutrient-rich material, traditional medicines have employed seaweed as a natural drug for centuries due to its bioactive components. Brown algae, such as Laminaria digitata, is known to contain fucoidan (a sulfated polysaccharide) which possesses anti-bacterial, anti-viral, anti-inflammatory, and anti-coagulant properties.2 This has led to applications in dermatological and pharmaceutical industries.3–5 The broad range of macroalgae applications from fertilizers, food, and fuels through to pharmaceuticals has led to the emergence of sustainable biorefinery concepts focused on macroalgae as a feedstock.6,7 As a third generation feedstock, macroalgae eliminates issues associated with using first and second generation feedstocks, such as slow growth rate and land area needed for plant growth. With algae, large-scale production will occur in the oceans and not lead to deforestation or competition for habitat that would otherwise occur if crops such as sugar cane (first generation) and wood (second generation) are used. As well, macroalgae biomass does not require the use of fertilizers to be grown i.e. no added chemicals are required to facilitate its growth. In fact, it can also be used to remediate polluted estuaries and coastal areas e.g. sewage outflow and fertilizer runoff. Its growing speed is another advantage compared with other crops when considering its application in production of biohydrogen. It is worth noting that biorefineries focused on microalgae feedstocks for the production of biodiesel are at a more advanced stage of development. This review will focus on macroalgae species as feedstocks.
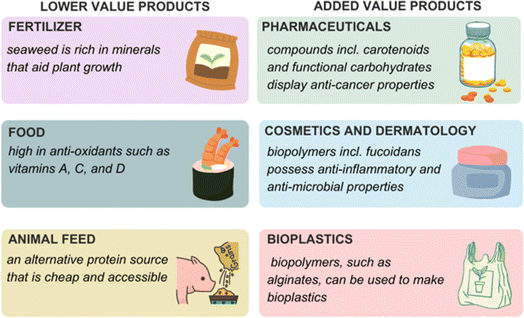 |
| Fig. 1 Varied uses of seaweed, seaweed extracts and compounds isolated from seaweed including biopolymers (figure made using https://www.canva.com). | |
Biorefineries were first introduced in the 1990s with the intention of upcycling low value materials from biomass resources into commercially desirable goods.8 It is only now that we face a climate crisis that alternatives to petroleum-based products and extraction procedures for high value products from biomass are being researched more intensely. The United Nations report that the largest contribution to greenhouse gas emissions, and thus climate change, is the burning of fossil fuels for energy applications with almost 90% of CO2 emissions originating from coal, oil, and gas.9 Current biorefineries use biomass such as starch, sugar crops (e.g. sugar cane), and grasses.8 However, lack of land space and unsuitable growing conditions for many biomass materials mean that they cannot be applied globally in biorefineries.10 This has led to a greater shift towards marine-based biorefineries as less land space is needed and growing conditions can be more varied, and drives the use of seaweed in this area of research (Fig. 2).11–15
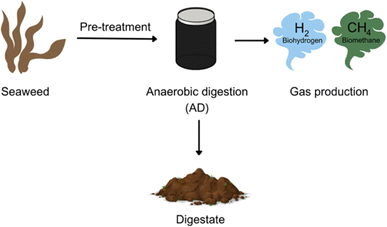 |
| Fig. 2 Simple scheme to represent biohydrogen and biomethane production from seaweed (figure made using https://www.canva.com). | |
When optimizing processes for obtaining materials from macroalgae, it is imperative that as much information on the seaweed is collected before extraction and any pre-treatment methods are employed. There are numerous species of macroalgae, with all being classed as either brown (Phaeophyceae), green (Chlorophyta), or red (Rhodophyta) seaweed. Some researchers have estimated that there are between 300
000 to 1 million species of algae in our oceans, with others claiming there are around 72
500 different varieties.16 Alongside the enormous number of species to be considered, there are external factors that influence the chemical composition of algae. For example, differences in season, depth, nutrient availability for the plant, wave action, temperature, and salinity have effects on the composition of seaweed, even in the same geographic location (Fig. 3).17 In addition to this, pre-treatment and extraction methods can affect what chemicals are obtained from this feedstock, as will be discussed later, and whether it is suitable for energy production.
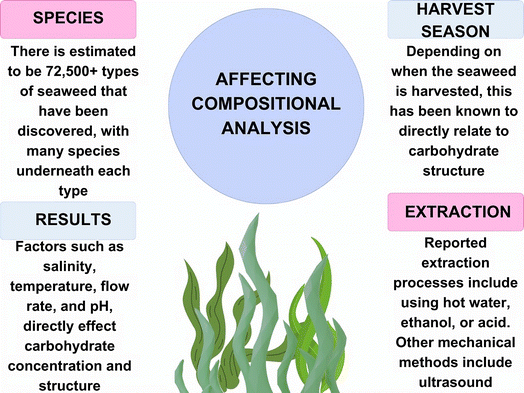 |
| Fig. 3 Factors affecting the chemical composition of macroalgae and its extracts (figure made using https://www.canva.com). | |
Screening of seaweed suitability via compositional analysis and identification of chemical compounds in seaweeds
Screening of the macroalgae to be used for energy production is critical for promoting the use of seaweed as a viable alternative to fossil fuels and other non-renewable sources. When deciding on what seaweed to use, it is important to obtain the concentrations of proteins and carbohydrates present. This will determine if the seaweed being analyzed is a valid option for biorefinery applications based on the quantity of biofuels it can potentially produce. In the context of this review, these biofuels include biohydrogen, biomethane, bioethanol, biodiesel, and biogas, which is a mixture of biomethane and carbon dioxide.18 We are not considering bio-oil or biogas produced via pyrolysis methods.
Bulk compositional analysis: carbohydrate, protein, enzyme levels and ash
Typical methods of analysis for carbohydrates and proteins include elemental analysis,19 with protein levels typically measured using the Bradford assay methodology.20 This involves mixing the Bradford reagent with the algae extract and then analyzing with UV-Vis spectrophotometry. The use of a standard bovine serum albumin is typical in this work to produce a calibration curve for protein content,6 and thus, determining if the seaweed is suitable for biohydrogen production.
Previous work in this field has observed a significant difference in carbohydrate content between green, brown, and red seaweed. It has been shown that brown seaweed, specifically Laminaria, contained the highest carbohydrate concentration.21 In contrast to high levels of carbohydrate that are critical for biohydrogen production, research has shown that high yields of biomethane result from using high protein content biomass.18,22 To remove the proteins from algae, Kazir et al. compared the efficiency of protein extraction using three different procedures (centrifugation, ultrasound, and dialysis) with additional compounds such as β-mercaptoethanol or ammonium sulfate.23 It was determined that suspending seaweed in sodium hydroxide (NaOH) and treating with ultrasound produced the highest purity protein extract from seaweed. However, it was still noted that extraction of proteins was difficult to do (10–11%), and further work was needed to obtain a higher yield. A key point to mention on this work is that the method that produced the highest purity protein extract was also the least energy-intensive procedure, as well as being less complex compared with other procedures performed in their study. Sustainable procedures like this that have been developed for food science applications could be extended to energy uses in the future.
Enzymatic studies employ a pre-fermentation approach to determine the concentration of two enzymes in the algae: nitrogenase and hydrogenase. To quantify the amount of nitrogenase present, Raman spectroscopy and/or an Acetylene Reduction Assay (ARA) that employ gas chromatography have been used.24 Recently, a new spectrophotometric method to measure nitrogenase activity has been developed.25 Similarly, quantification of hydrogenase can be done spectrophotometrically via the reduction product of methyl viologen, which is oxidized by the hydrogenase enzyme.26 These biological catalysts are responsible for the metabolic mechanism associated with the formation and production of biohydrogen, with biological engineering required to further optimize the interaction of the enzymes with the substrate, in this case, organic materials.27,28 However, studies suggest that the hydrogenase enzyme is sensitive to high concentrations of oxygen (5.0 vol%), which in turn, directly inhibits biohydrogen production,29 and so it is important to keep conditions anaerobic and monitor oxygen levels carefully. It is worth noting that bio-engineered enzymatic treatments, especially when applied on an industrial scale, might become expensive due to their sensitivity and specificity. Therefore, Life Cycle Assessments (LCA) and Techno-Economic Analyses (TEA) will be important tools to determine if the use of engineered enzymes are a reasonable addition to the biorefinery.30
Ash content present in seaweeds varies significantly depending on species and geography (∼15–49%).31 In the 2015 review by Sambusiti et al., they noted the high levels of ash produced by brown seaweeds, similar to land-based biomass such as lignocellulose. The increased level of metals, including potassium and calcium, in residues left after dark fermentation of algae have the potential to act as a cheap catalyst for the co-processing of brown seaweed via gasification.32 A circular approach within a seaweed biorefinery, where waste from one process can be used in another, will add to the overall sustainability of the system.
Water content
Like humans, seaweed is composed mostly of water (∼80–90%).17 However, for biorefinery applications this is a disadvantage. For biohydrogen to be produced, seaweed is dried, and therefore temperatures ranging from 80–120 °C (and more energy) are needed to dehydrate the feedstock initially. In their 2020 study, Zhang et al. concluded that the conversion of freshly harvested seaweed to dry seaweed was a carbon-neutral process with high yields of (bio)hydrogen being observed.33 Seaweed is able to act as a carbon-neutral material by absorbing CO2 from the atmosphere during photosynthesis, whereby CO2 produced as emissions can be mitigated.34 A range of methods have been explored to dewater and ultimately dry the seaweed feedstock: osmotic media, organic/mineral acids, screw-pressing, and conventional drying.35 As seen in other research studies using seaweed towards biorefinery applications, these processes are species-dependent. It was reported that to dewater brown seaweed, required for biohydrogen production, it should be treated with hydrochloric acid before pressing. However, dewatering a red seaweed species, Palmaria palmata, could be done using screw pressing alone.36
Mass spectrometry, vibrational spectroscopy and other characterization tools
Analysis of components present within seaweed is often performed via mass spectrometry (MS) and spectroscopy. MS techniques reported include Quadrupole Time of Flight Mass Spectrometry (qTOF MS), Matrix-Assisted Laser Desorption Ionization Time of Flight Mass Spectrometry (MALDI-TOF MS),37 Liquid Chromatography Tandem Mass Spectrometry (LC-MS/MS),38 Inductively Coupled Plasma Mass Spectrometry (ICP-MS)39 and Pyrolysis-Gas Chromatography Mass Spectrometry (Py-GC/MS).40 These methods have particular strengths when determining structure and chemical composition of carbohydrates in seaweeds. However, due to the complex nature of the analytes, reproducible results are difficult to obtain, and fragmentation reported in literature spectra may not be seen for a multitude of reasons (environmental differences, extraction technique, genus of seaweed) in subsequent samples. Historically, spectroscopic data have been obtained through infrared spectroscopy (IR) including attenuated total reflectance (ATR), and Raman spectroscopy.41 Thermal Gravimetric Analysis (TGA) has also been used to get valuable information on algae feedstocks and pre-treatment methods, and shows stepwise decomposition of bio-polymers present (alginic acid, fucoidan, and laminarian).40 Microscopic analysis such as Scanning Electron Microscopy (SEM) and X-ray Diffraction (XRD) are also useful to determine whether pre-treatment methods have affected the surface structure of the seaweed or the crystallinity of biopolymers within so it can produce biogas in decent yields.42
By using various types of analysis, appropriate seaweeds for biohydrogen analysis can be identified for screening and optimization purposes. This promotes sustainability by limiting the amount of waste that would be produced if seaweeds were sent through for pre-treatment, without first determining if they are suitable for producing biohydrogen.
Pre-treatment (mechanical, biological, and chemical)
Pre-treatment of macroalgae for biogas production has been reported as an effective way of increasing the yield for biorefinery applications (Table 1). Although there are a wide variety of pre-treatment processes, there are four major types: mechanical, chemical, biological, and thermal.43 Inhibitor removal, also known as detoxification, is also employed after pre-treatment of seaweed as inhibitors will cause biohydrogen yields to decrease.44
Table 1 Examples of seaweed pre-treatments towards the production of biohydrogen, bioethanol, or biomethane (VS, volatile solid)
Seaweed |
Type of pre-treatment |
Pre-treatment |
Biohydrogen yield |
Bioethanol yield |
Biomethane yield |
Ref. |
Chaetomorpha linum (Chlorophyta) |
Mechanical |
Wet oxidation/ball milling |
— |
44 g ethanol/100 g glucan |
— |
46
|
Laminaria japonica (Phaeophyceae) |
Mechanical/thermal |
Grinding 170 °C, 20 min |
109.6 mL H2 per g COD |
— |
— |
47
|
Ulva prolifera (Ulvaceae) |
Chemical |
0.2% H2O2, pH 4, 12 h, 50 °C |
— |
31.4% VS |
— |
51
|
Gelidium amansii (Gelidiaceae) |
Chemical |
Steam heating, 1% H2SO4 |
68.6 mL per g VS |
— |
— |
52
|
Laminaria digitata (Phyeophyceae) |
Biological |
Enzyme hydrolysis |
252.9 mg glucose per g VS |
— |
— |
53
|
Ulva/Sargassum mix (Ulvales/Phaeophyceae) |
Biological |
Trametes hirsute fungi |
— |
— |
104 mL per g VS |
58
|
Mechanical
The main objective of mechanical pre-treatment is to increase the accessible surface area of the algae to encourage greater extraction of carbohydrates and sugars that can then ferment to produce biogas.45 Procedures such as milling, chopping, microwave, and beating are all examples that lead to increased surface areas, but not all of them encourage greater fuel production for all seaweeds (Fig. 4). For example, when the seaweed U. lactuca was milled using centrifuge apparatus a decrease in bioethanol yield was observed. On the other hand, the same procedure performed on C. lineum, a green seaweed, led to a 64% increase in bioethanol yield compared with untreated macroalgae.46 Regarding biohydrogen specifically, a combination of grinding and thermal treatment have shown H2 yields of 158 mL of gas per gram of volatile solid (VS) for Laminaria japonica.47
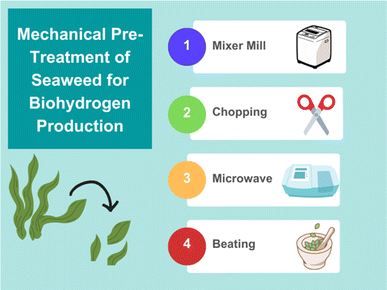 |
| Fig. 4 Graphic summary of different mechanical pre-treatment options for enhancing biogas production from seaweed (figure made using https://www.canva.com). | |
Chemical
Chemical pre-treatment involves the addition of chemicals such as acids or bases to encourage hydrolysis of carbohydrates present in seaweeds.48,49 Along with strongly acidic or basic compounds such as HCl and NaOH, recent literature has explored the use of ionic liquids (ILs), such as 1-butyl-3-methylpyridium chloride ([Bmpy][Cl]) and 1-butyl-3-methyl imidazolium chloride ([Bmim][Cl]), to assist with polysaccharide hydrolysis and subsequent bioethanol production. Use of ILs can promote greener practices in this research field, and in some cases increased sustainability by reducing by-product formation and they could be recycled and reused whilst maintaining a high level of efficiency.50
In addition to use of acid or base alone, peroxide treatments have been used in tandem with enzymatic hydrolysis procedures for bioethanol production, and perhaps this could be extended to biohydrogen generation. Optimal conditions for the pre-treatment of Ulva prolifera, green algae, was 0.2% H2O2 at a pH 4.0 for 12 hours at 50 °C on seaweed residue after carbohydrate extraction.51 The function of peroxide treatment is to increase cell wall degradation. If the integrity of the algal cell wall was not destroyed, biohydrogen, bioethanol, and other bio-derived gases could not be formed.42 However, LCA and TEA would need to be performed prior to further pursuit of this method or commercialization. Work from Sivagurunathan and co-workers showed that dilute sulfuric acid optimized sugar release from the algae Gelidium amansii compared to other acids such as HCl and HNO3.52
Biological
Biological treatments, also known as enzymatic treatments, look at using specific classes of enzymes to promote degradation of seaweed such as cellulases. For biorefinery purposes, the focus is on hydrolysis of polysaccharides into the corresponding simple sugars that can then be used for biogas production.53 We can compare this approach with land-based bio-feedstocks and in particular to lignocellulose degrading enzymes, such as monooxygenases.54 By using an enzyme, less energy, chemicals, and synthetic steps are needed to obtain the desired result. Although enzymes, such as cellulases, have often been considered too expensive for industrial implementation, new technologies are being developed to produce industrially relevant enzymes in a low-cost and sustainable way.55,56
Work conducted by Herrera Barragán et al. investigated the use of enzyme assisted extraction (using proteases and carbohydrases) for a downstream seaweed biorefinery process. Additionally, they completed a TEA, which focused on the economic performance of using enzymes to obtain alginate and biological saccharides, such as laminarin and fucoidan. Their results showed that even using cheaper, more general enzymes, such as proteases, the enzymes represent 40% of the total cost of operations with the percentage increasing to 72% with more specific and, therefore, expensive enzymes.57 In addition, research seen in literature shows biomethane production using fungi as a source of enzymes as a renewable and cost-effective starting material with relatively high biomethane yields.58 Therefore, further research is needed to reduce enzyme production costs. Also, this research suggests that high value co-product extraction will be essential in early generations of seaweed biorefineries in order to supplement fuel production.
Inhibitor removal
Along with treatments to encourage biogas production by increasing surface area and the amount of carbohydrate readily available, there are also processes to remove components from seaweed that could potentially reduce the yield. Similar to the procedures above, the removal of such compounds has shown varied effects, both beneficial or detrimental, toward biogas production. For example, it was reported by Hierholtzer and Akunna that salts present in macroalgae found in oceans affect microbial digestion.59 In other words, salts limit the growth of bacteria that degrade seaweed. In order to optimize yield in bioethanol, biodiesel, and biomethane production, salts should first be removed. In addition, metals and phenols sometimes found in algae can decrease biogas yields. Along with surface contaminants including salts, products formed during thermal pre-treatment can inhibit the production of biohydrogen. For example, 5-hydroxymethyl furfural (HMF) and furfural by-products can be removed using activated carbon, leading to an increase in ethanol yields.60 Related to this, the production of levulinic acid (through decomposition of HMF) has been determined to also inhibit the production of biohydrogen in literature works.61 It is worth noting that some by-products, like HMF, have potential uses themselves such as in organic synthesis as a starting material.62,63 Using such by-products would reduce waste overall and would create a process that encourages circularity.
Product characterization and analysis using mass spectrometry
Since carbohydrates, whether complex polysaccharides or simple sugars, are key to biohydrogen production, their measurement is key to understanding many of the pre-treatment methods used to increase process efficiency. Therefore, it is important to understand the different methods available for their analysis. The most common ones used for carbohydrate analysis are Electrospray Ionization Mass Spectrometry (ESI MS), and Matrix Assisted Laser Desorption Ionization Time of Flight Mass Spectrometry (MALDI-TOF MS).64 These are often used in conjunction with chromatographic methods such as Gel Permeation Chromatography (GPC), which can also provide information on the size of the biopolymer itself or fragments produced via hydrolysis. Due to the complex nature of polysaccharides present in seaweed, including fucoidan, mass spectrometric techniques, especially tandem mass spectrometry MS/MS, tend to give more valuable structural data (sugars present, degree and position of sulfation) compared with NMR and IR spectroscopies.
Electrospray ionization mass spectrometry
Electrospray Ionization Mass Spectrometry (ESI MS) is a common analytical tool used across science and engineering disciplines to determine structural and side chain functionalities present in molecules (Fig. 5). Molecules are ionized and fragmented to yield a species or fragment with varying mass-to-charge (m/z) ratios. Depending on how much of the fragment is present in the mixture and produced during ionization in the instrument, the intensity of the signal in the mass spectrum for the fragment will vary. Seaweed extracts and other complex polysaccharide mixtures require additional procedures beforehand so data can be collected and analyzed. Without preliminary work, the large size of the biopolymer prevents its volatilization and subsequent ionization in the mass spectrometer's source. Hydrolysis procedures are the most common method for breaking down the polymer before being subjected to MS analysis and this hydrolysis can be performed in a similar way to pre-treatment methods above i.e. acid/base hydrolysis or enzymes. Identifying fragments, and determining the number and types of sugars present, are critical steps in the pre-treatment stage to determine if that seaweed can be used in biorefinery applications.
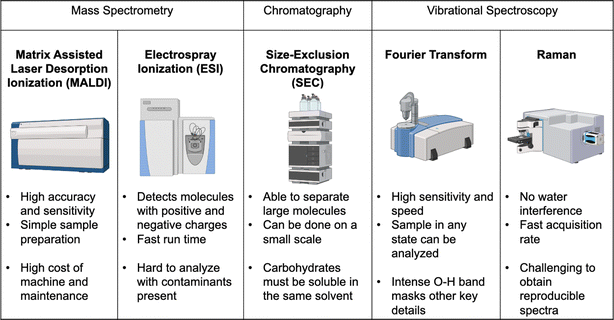 |
| Fig. 5 Analytical tools used for assessing carbohydrate content in seaweed for biohydrogen production and their respective strengths and weaknesses (instrument images obtained from https://www.biorender.com). | |
Matrix assisted laser desorption ionization time of flight mass spectrometry (MALDI-TOF MS)
MALDI-TOF MS is a common technique used in the analysis of polymers, both synthetic and natural, as it can provide information on molecular weight and end-groups that are present. When using MALDI-TOF MS as an analytical tool, a range of factors such as the matrix and cationizing agent can enhance sensitivity and increase ionization efficiency. Common matrices used include α-cyano-4-hydroxycinnamic acid (HCCA) and 2,5-dihydroxybenzoic acid (DHB), along with sodium trifluoroacetate as a cationizing agent (NaTFA). Cationizing agents assist, in this case, by protonation of the previously neutral species to give greater sensitivity. Additives such as HCCA, DHB, and NaTFA are particularly useful here as collecting complex structural data will be imperative in determining optimal extraction and pre-treatment methods for biogas production.65 Previous studies using biological pre-treatment, such as enzymatic degradation, helped depolymerize the polysaccharides and allow the sugar monomers present in the seaweed to be identified and quantified without needing chromatographic separation beforehand.66 Within this field, understanding of the sugars present is the key goal as the sugars are the food for microbes present in the bioreactor, which produce the biogas product.
Gel permeation chromatography (GPC)
GPC is sometimes run alongside MS experiments as it can provide information on the molecular weight of the polysaccharides based on their hydrodynamic radius (size in solution). By using columns to provide separation of the macromolecules by size, typically larger (and heavier) molecules are eluted first as smaller molecular weight (MW) structures diffuse in and out of pores present in the column. For biorefinery applications, MW data is crucial to determine the amount of hydrolysis that needs to occur to obtain simple sugars, that will be useful for energy applications. Yuan and Macquarrie investigated the effect of various alginate molecular weights in alginate for biorefinery use. They showed that using pre-treatment conditions in a biorefinery, downstream process, allowed for a lower MW biopolymer to be obtained (75 kDa) compared with a high MW biopolymer from a regular alginate extraction (195 kDa). Moreover, extractions using ethanol beforehand to remove pigments and other co-products, as well as fucoidan isolation using acidification and centrifugation led to lower molecular weights being seen.67 Lower molecular weights are preferred for biorefinery processes, as it becomes easier to break these down into simpler carbohydrates for biogas production.68
Product characterization and analysis using spectroscopy
Due to the complexity of carbohydrates present in macroalgae, spectroscopic techniques are often performed alongside spectrometric and chromatographic methods. These include Infrared Spectroscopy (IR), Raman Spectroscopy, and Nuclear Magnetic Resonance (NMR) Spectroscopy. IR is perhaps the most useful one in terms of allowing product formation to be monitored. Other techniques like 1H-NMR spectroscopy have shown promise in the area of phenol detection in the algae feedstock, which has been shown to decrease hydrogen production.69 Phenolics can be extracted beforehand and used in other industrial applications such as in dermatological applications to further enhance the circular economy of seaweed.70
Infrared spectroscopy
IR spectroscopy provides structural data about biomolecules and can be quantitative. The technique is easy, fast, and cost-effective,71 making it ideal for biorefinery applications.
Work reported by Wolf et al. explored the benefit of near infrared spectroscopy (NIRS) for continuous analysis of multiple compounds in both solid and liquid phases. Along with dry matter, such as ash, and volatile organic compounds, multiple acids present in the digestion stage (acetic, propionic, and butyric acid) were measured to understand the microbial activity throughout a biomethane production plant.72 By obtaining in situ measurements using NIRS, optimization of biogas production can be achieved by understanding the microbial composition of the solid and liquid components. Additional research in this area from Kumari et al. used IR spectroscopy during pre-treatment stage of microalgae to monitor the disruption of cell walls.73 Results showed that pre-treatment conditions were able to weaken bonds of lipids, proteins, and carbohydrates that were found in the microalgae cell wall, and thus increasedbiogas yields. By using pre-treatment methods such as those described, the amount of biogas produced can be maximized and spectroscopic methods are important in this quest.
Raman spectroscopy
Although not as common as IR spectroscopy, Raman spectroscopy, more specifically cavity enhanced Raman spectroscopy, has also been used in monitoring biogas production.74 Like FT-IR, Raman spectroscopy provides structural information on molecules through the vibrational modes displayed in a spectrum that are characteristic of specific functional groups. Through the research conducted by Sieburg et al. the benefits of using cavity enhanced Raman were highlighted including fast response times (5 seconds) and the analysis of a mixture of gases (CH4, CO2, N2, O2, and H2). This type of spectroscopy is important for biogas monitoring to quantify what is being released during the fermentation process. This will then reveal additional information on what pre-treatments can optimize biogas yields, and moreover, signalling when the system plateaus in biogas production. This is still a relatively new way of analyzing biogas, but Raman spectroscopy is being used in materials and biomedical sciences and could be explored further in biofuel applications. One reason why minimal research into Raman spectroscopy applied to biogas production from algae has been performed could be due to its perceived lack of reproducibility. As stated previously, there is already significant variation in macroalgae composition due to species, extraction, and external factors.
To summarize, we note the main challenges associated with screening algal biomass as well as analytical tools used to monitor biohydrogen and biogas production. Due to the complexity of algal biomass' chemical composition, screening biomass to be used in biohydrogen applications is a challenge for researchers in this area. With seasonal and geographical variation and inter species, as well as extraction techniques, the overall composition can be challenging to determine. Furthermore, having a general method for all types of seaweed is impossible to predict and optimize. That being said, ways of screening for compounds of interest in biorefinery applications can be created and used universally across all types of seaweed. Future work in this research area will look at additional ways to screen seaweed that is cost efficient and optimal for predicting biohydrogen yield. Furthermore, it will be of equal importance to consider the sustainability of the process, meaning extraction methods that use less energy and solvents should be identified. New green approaches may have a dual benefit leading to greater yields of biohydrogen in addition to reducing the environmental impact of the process overall.
Conclusion
In this review, we have highlighted the use of macroalgae as a source of sustainable energy. Seaweeds are available worldwide and can be used as an important renewable source of hydrogen and methane. Optimization of all pre-treatment methods and identification of suitable analytical techniques are critical to the success of seaweed based biorefineries such as those that could produce fuels alongside higher value, biologically active compounds, nutritional supplements, and biopolymers. Certainly, disadvantages exist and need to be considered, such as variability across species, climate, and season. However, the potential of reducing, and eventually reversing, the effects of climate change provide a strong impetus towards development of seaweed as a renewable feedstock and source of green hydrogen.
This review reveals the gap in literature regarding the comparison of pre-treatments for macroalgae being used in biohydrogen work, which to our knowledge, has not been performed to date. Work currently in the literature shows optimization of a single pre-treatment, but not of combinations of different pre-treatments. As previously referenced, chemical pre-treatment using hydrogen peroxide was optimized by Li and co-workers to produce bioethanol from Ulva prolifera biomass,51 and has not been explored for biohydrogen production nor combined with other pre-treatment methods such as milling. By comparing methods of pre-treatment in parallel or sequentially, the processes can be evaluated and assessed in a judicious way e.g. the effect on the environment via Life Cycle Analyses (LCA) may show that enzymatic pre-treatment combined with mechanochemistry is preferential to chemical pre-treatment. A recent report described LCAs of biohydrogen production from municipal solid waste compared with solar and wind energy. It showed that a negative carbon footprint can be achieved from starting materials with carbon capture ability.75 Extensive work in this area will need to be conducted to show that the use of macroalgae as a replacement to fossil fuels is a viable option to globally become carbon negative, such as thorough biorefinery design by gradual scale-up from lab scale to pilot scale, and by making adjustments as necessary, up to full scale. Biohydrogen production would be complementary to existing developments producing biodiesel, as lipids are used for biodiesel production and the carbohydrates within the macroalgae are important for biohydrogen production.
Future perspectives
The use of macroalgae in various applications has increased over the past decade, with a focus on nutraceuticals, dermatology, and bioenergy applications. Amongst many, it can be said that seaweed provides many advantages, such as being a renewable material. However, for macroalgae to be considered as a viable option for biorefinery applications, it is crucial to understand its composition. Monitoring different types of algae under varying conditions (such as temperature, salinity, and wave speed) will be needed to understand how external stresses relate to sugar content. Along with external stresses, how the seaweed is then processed will be of equal importance as some methods promote the formation of other products that may not be of interest. For biohydrogen applications, having sugars that are fermentable will lead to greater yields.51
Strengths, weaknesses, opportunities, and threats (SWOT) are important when considering seaweed as a renewable feedstock in biorefinery applications. Seaweeds are a sustainable option for many reasons, such as not competing for land mass and their rapid growth rate. They are also able to remediate environmental issues in oceans, such as heavy metal contamination. They can also serve as tools for conservation and assist in supporting fish stocks by providing a suitable environment for young fish to mature (fish nurseries). However, weaknesses also exist. As with all plants, macroalgae can suffer from diseases and be impacted by weather-related emergencies, with the latter becoming increasingly common due to climate change. Seaweed farms are at a great risk from these issues. Furthermore, new infrastructure and training of suitable personnel are needed for a successful farm. Opportunities for encouraging a circular economy are present when using seaweed because a range of bioproducts of high value can be produced simultaneously alongside biohydrogen (Fig. 6). Seaweed biorefineries would also support rural employment opportunities and economic diversification. Threats to third generation biomass for biorefinery applications include wind and solar energy being used to generate energy and hydrogen production via electrolysis processes. Although these energy options move away from fossil fuel usage, they can be expensive to start and maintain, and do not produce the range of products that biorefineries do. Solar and wind energy are dependent on location (e.g., those with high wind or sun scale), which is a factor in common with ocean biorefineries. Therefore, preferred hydrogen production methods will be strongly dependent on geography. By using seaweed as a renewable starting material, we should be able to optimize procedures so that weaknesses and threats of using macroalgae as a feedstock for biorefinery applications are minimized.
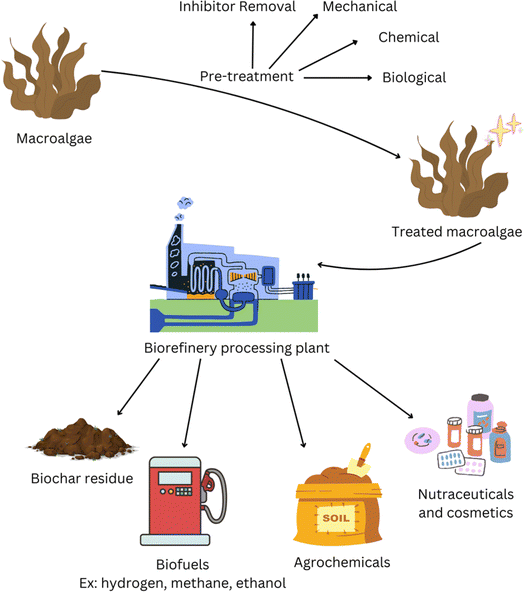 |
| Fig. 6 General biorefinery process using seaweed as a renewable feedstock. | |
Conflicts of interest
There are no conflicts of interest to declare.
Acknowledgements
We are grateful for the funding provided by the Government of Canada via the New Frontiers in Research Fund (NFRF) and the Natural Sciences and Engineering Research Council of Canada (NSERC). Memorial University's School of Graduate Studies and Dr Liqin Chen are thanked for scholarships (O. W.). Useful conversations with Dr R. Helleur (Emeritus) and Dr J. Stockmann are appreciated.
References
-
R. S. Baghel, V. A. Mantri and C. R. K. Reddy, A New Wave of Research Interest in Marine Macroalgae for Chemicals and Fuels: Challenges and Potentials in Fuels, Chemicals and Materials from the Oceans and Aquatic Sources, John Wiley & Sons Ltd, Chichester, UK, 2017, pp. 43–63 Search PubMed.
- N. M. A. Ponce and C. A. Stortz, Front. Plant Sci., 2020, 11 DOI:10.3389/fpls.2020.556312.
- S. Lomartire and A. M. M. Gonçalves, Mar. Drugs, 2022, 20, 141 CrossRef CAS PubMed.
- V. Jesumani, H. Du, M. Aslam, P. Pei and N. Huang, Mar. Drugs, 2019, 17, 688 CrossRef CAS PubMed.
- L. López-Hortas, N. Flórez-Fernández, M. D. Torres, T. Ferreira-Anta, M. P. Casas, E. M. Balboa, E. Falqué and H. Domínguez, Mar. Drugs, 2021, 19, 552, DOI:10.3390/md19100552.
- E. Kouhgardi, S. Zendehboudi, O. Mohammadzadeh, A. Lohi and I. Chatzis, Renewable Sustainable Energy Rev., 2023, 172, 113012 CrossRef CAS.
-
D. Mondal and K. Prasad, Fuels, Chemicals and Materials from the Oceans and Aquatic Sources, 2017, vol. 1, ch. 4, pp. 65–82 Search PubMed.
-
J.-L. Wertz, O. Bédué and O. Bédué, Lignocellulosic Biorefineries, CRC Press LLC, Boca Raton, United States, 2013 Search PubMed.
- X.-L. Yue and Q.-X. Gao, Adv. Clim. Change Res., 2018, 9, 243–252 CrossRef.
- F. M. Kerton, Y. Liu, K. W. Omari and K. Hawboldt, Green Chem., 2013, 15, 860–871 RSC.
- S. Karishma, A. Saravanan, P. Senthil Kumar and G. Rangasamy, Bioresour. Technol., 2022, 366, 128187 CrossRef CAS PubMed.
- J. Sadhukhan, S. Gadkari, E. Martinez-Hernandez, K. S. Ng, M. Shemfe, E. Torres-Garcia and J. Lynch, Green Chem., 2019, 21, 2635–2655 RSC.
- A. Sharma and S. K. Arya, Biotechnol. Rep., 2017, 15, 63–69 CrossRef PubMed.
- M. D. Torres, S. Kraan and H. Domínguez, Rev. Environ. Sci. Biotechnol., 2019, 18, 335–388 CrossRef CAS.
- M. D. Hempel, P. Colepicolo and L. Zambotti-Villela, Phycology, 2023, 3, 211–241 CrossRef.
-
J. Bothwell, Seaweeds of the World, Princeton University Press, Princeton, United States, 2023 Search PubMed.
- G. F. El-Said and A. El-Sikaily, Environ. Monit. Assess., 2013, 185, 6089–6099 CrossRef CAS PubMed.
- N. Thakur, E.-S. Salama, M. Sharma, P. Sharma, D. Sharma and X. Li, Mater. Today Sustain., 2022, 18, 100120 CrossRef.
- S. P. Slocombe, Q. Zhang, M. Ross, A. Anderson, N. J. Thomas, Á. Lapresa, C. Rad-Menéndez, C. N. Campbell, K. D. Black, M. S. Stanley and J. G. Day, Sci. Rep., 2015, 5, 9844 CrossRef PubMed.
- M. Begemann, M. Mormile, O. Sitton, J. Wall and D. Elias, Front. Microbiol., 2012, 3 DOI:10.3389/fmicb.2012.00093.
- J. Olsson, G. B. Toth and E. Albers, J. Appl. Phycol., 2020, 32, 3305–3317 CrossRef CAS.
- E. Kovács, R. Wirth, G. Maróti, Z. Bagi, K. Nagy, J. Minárovits, G. Rákhely and K. L. Kovács, Bioresour. Technol., 2015, 178, 254–261 CrossRef PubMed.
- M. Kazir, Y. Abuhassira, A. Robin, O. Nahor, J. Luo, A. Israel, A. Golberg and Y. D. Livney, Food Hydrocolloids, 2019, 87, 194–203 CrossRef CAS.
- M. J. Dilworth, Biochim. Biophys. Acta, Gen. Subj., 1966, 127, 285–294 CrossRef CAS PubMed.
- L. Payá-Tormo, D. Coroian, S. Martín-Muñoz, A. Badalyan, R. T. Green, M. Veldhuizen, X. Jiang, G. López-Torrejón, J. Balk, L. C. Seefeldt, S. Burén and L. M. Rubio, Sci. Rep., 2022, 12, 10367 CrossRef PubMed.
- L. Yu and M. J. Wolin, J. Bacteriol., 1969, 98, 51–55 CrossRef CAS PubMed.
- T. Paula, A. Rikard, L. Pia, O. Fredrik, W. Röbbe and L. Peter, Microbiol. Mol. Biol. Rev., 2002, 66, 1–20 CrossRef PubMed.
- J. Xuan, L. He, W. Wen and Y. Feng, Molecules, 2023, 28, 1392, DOI:10.3390/molecules28031392.
- J. Koo, S. Shiigi, M. Rohovie, K. Mehta and J. R. Swartz, J. Biol. Chem., 2016, 291, 21563–21570 CrossRef CAS PubMed.
- A. W. Bartling, M. L. Stone, R. J. Hanes, A. Bhatt, Y. Zhang, M. J. Biddy, R. Davis, J. S. Kruger, N. E. Thornburg, J. S. Luterbacher, R. Rinaldi, J. S. M. Samec, B. F. Sels, Y. Román-Leshkov and G. T. Beckham, Energy Environ. Sci., 2021, 14, 4147–4168 RSC.
- K. Pirian, Z. Z. Jeliani, M. Arman and M. Yousefzadi, Trop. Life Sci. Res., 2020, 31, 1–17 CrossRef PubMed.
- C. Sambusiti, M. Bellucci, A. Zabaniotou, L. Beneduce and F. Monlau, Renewable Sustainable Energy Rev., 2015, 44, 20–36 CrossRef CAS.
- K. Zhang, W.-J. Kim and A.-H. A. Park, Nat. Commun., 2020, 11, 3783 CrossRef CAS PubMed.
- F. Ross, P. Tarbuck and P. I. Macreadie, Front. Mar. Sci., 2022, 9, 1015612 CrossRef.
- J. A. Gallagher, L. B. Turner, J. M. M. Adams, P. W. Dyer and M. K. Theodorou, Bioresour. Technol., 2017, 224, 662–669 CrossRef CAS PubMed.
- J. A. Gallagher, L. B. Turner, J. M. M. Adams, S. Barrento, P. W. Dyer and M. K. Theodorou, J. Appl. Phycol., 2018, 30, 2305–2316 CrossRef PubMed.
- S. D. Anastyuk, N. M. Shevchenko, E. L. Nazarenko, P. S. Dmitrenok and T. N. Zvyagintseva, Carbohydr. Res., 2009, 344, 779–787 CrossRef CAS PubMed.
- G. Rajauria, B. Foley and N. Abu-Ghannam, Harnessing Mar. Bioresour. Innov. Food Ind., 2016, 37, 261–268 CAS.
- V. N. Nkemka and M. Murto, Bioresour. Technol., 2013, 128, 164–172 CrossRef CAS PubMed.
- A. B. Ross, K. Anastasakis, M. Kubacki and J. M. Jones, Pyrolysis, 2009, 85, 3–10 CrossRef CAS.
- L. Pereira, A. M. Amado, A. T. Critchley, F. van de Velde and P. J. A. Ribeiro-Claro, Food Hydrocolloids, 2009, 23, 1903–1909 CrossRef CAS.
- M. A. Hassaan, A. El Nemr, M. R. Elkatory, A. Eleryan, S. Ragab, A. El Sikaily and A. Pantaleo, Energies, 2021, 14, 1703, DOI:10.3390/en14061703.
- S. Maneein, J. J. Milledge, B. V. Nielsen and P. J. Harvey, Fermentation, 2018, 4, 100, DOI:10.3390/fermentation4040100.
- S. Shobana, G. Kumar, P. Bakonyi, G. D. Saratale, A. H. Al-Muhtaseb, N. Nemestóthy, K. Bélafi-Bakó, A. Xia and J.-S. Chang, Bioresour. Technol., 2017, 244, 1341–1348 CrossRef CAS PubMed.
- N. Wei, J. Quarterman and Y.-S. Jin, Trends Biotechnol., 2013, 31, 70–77 CrossRef CAS PubMed.
- N. Schultz-Jensen, A. Thygesen, F. Leipold, S. T. Thomsen, C. Roslander, H. Lilholt and A. B. Bjerre, Bioresour. Technol., 2013, 140, 36–42 CrossRef CAS PubMed.
- K.-W. Jung, D.-H. Kim and H.-S. Shin, Bioresour. Technol., 2011, 102, 2745–2750 CrossRef CAS PubMed.
- C. Vanegas, A. Hernon and J. Bartlett, Sep. Sci. Technol., 2014, 49, 30–38 CrossRef CAS.
-
A. A. Shah, T. H. Seehar, K. Sharma and S. S. Toor, in Hydrocarbon Biorefinery, ed. S. K. Maity, K. Gayen and T. K. Bhowmick, Elsevier, 2022, pp. 203–228 Search PubMed.
- Uju, A. T. Wijayanta, M. Goto and N. Kamiya, AIP Conf. Proc., 2018, 1931, 020004 CrossRef.
- Y. Li, J. Cui, G. Zhang, Z. Liu, H. Guan, H. Hwang, W. G. Aker and P. Wang, Bioresour. Technol., 2016, 214, 144–149 CrossRef CAS PubMed.
- P. Sivagurunathan, G. Kumar, T. Kobayashi, K. Xu and S.-H. Kim, Int. J. Hydrogen Energy, 2017, 42, 27600–27606 CrossRef CAS.
- E. T. Kostas, D. A. White and D. J. Cook, BioEnergy Res., 2020, 13, 271–285 CrossRef CAS PubMed.
- K. S. Johansen, Trends Plant Sci., 2016, 21, 926–936 CrossRef CAS PubMed.
- D. Sakhuja, H. Ghai, R. K. Rathour, P. Kumar, A. K. Bhatt and R. K. Bhatia, 3 Biotech, 2021, 11, 280 CrossRef PubMed.
- V. Sharma, M.-L. Tsai, P. Nargotra, C.-W. Chen, C.-H. Kuo, P.-P. Sun and C.-D. Dong, Catalysts, 2022, 12, 1373, DOI:10.3390/catal12111373.
- J. A. Herrera Barragán, G. Olivieri, I. Boboescu, M. Eppink, R. Wijffels and A. Kazbar, Front. Mar. Sci., 2022, 9, 1–13 Search PubMed.
- R. Tapia-Tussell, J. Avila-Arias, J. Domínguez Maldonado, D. Valero, E. Olguin-Maciel, D. Pérez-Brito and L. Alzate-Gaviria, Energies, 2018, 11, 494, DOI:10.3390/en11030494.
- A. Hierholtzer and J. C. Akunna, Water Sci. Technol., 2012, 66, 1565–1573 CrossRef CAS PubMed.
- J.-W. Hong, D.-H. Gam, J.-H. Kim, S.-J. Jeon, H.-S. Kim and J.-W. Kim, Molecules, 2021, 26, 2435, DOI:10.3390/molecules26092435.
- G. Kumar, H.-C. Cheon and S.-H. Kim, Int. J. Hydrogen Energy, 2014, 39, 16885–16890 CrossRef CAS.
- W. Fan, C. Verrier, Y. Queneau and F. Popowycz, Curr. Org. Synth., 2019, 16, 583–614 CrossRef CAS PubMed.
- A. A. Rosatella, S. P. Simeonov, R. F. M. Frade and C. A. M. Afonso, Green Chem., 2011, 13, 754–793 RSC.
- D. J. Harvey, Mass Spectrom. Rev., 2009, 28, 273–361 CrossRef CAS PubMed.
- K. Khatri, M. S. Rathore, S. Agrawal and B. Jha, Biomass Bioenergy, 2019, 130, 105392 CrossRef CAS.
- J. M. Dobruchowska, B. Bjornsdottir, O. H. Fridjonsson, J. Altenbuchner, H. Watzlawick, G. J. Gerwig, L. Dijkhuizen, J. P. Kamerling and G. O. Hreggvidsson, Front. Plant Sci., 2022, 13 DOI:10.3389/fpls.2022.981602.
- Y. Yuan and D. J. Macquarrie, Bioresour. Technol., 2015, 198, 819–827 CrossRef CAS PubMed.
- B. Senthil Rathi, P. Senthil Kumar, G. Rangasamy and S. Rajendran, Int. J. Hydrogen Energy, 2024, 52, 115, DOI:10.1016/j.ijhydene.2022.10.182.
- P. Sharma and U. Melkania, Waste Manag., 2018, 78, 115–123 CrossRef CAS PubMed.
- N. Kumar and N. Goel, Biotechnol. Rep., 2019, 24, e00370 CrossRef PubMed.
-
E. Capuano and S. M. van Ruth, in Encyclopedia of Food and Health, ed. B. Caballero, P. M. Finglas and F. Toldrá, Academic Press, Oxford, 2016, pp. 424–431 Search PubMed.
- D. Wolf, H. von Canstein and C. Schröder, Int. Gas Res. Conf., 2011, 2011(3), 625–632 Search PubMed.
- P. Kumari, A. K. Varma, R. Shankar, L. S. Thakur and P. Mondal, J. Environ. Chem. Eng., 2021, 9, 104974 CrossRef CAS.
- A. Sieburg, S. Schneider, D. Yan, J. Popp and T. Frosch, Analyst, 2018, 143, 1358–1366 RSC.
- G. Amaya-Santos, S. Chari, A. Sebastiani, F. Grimaldi, P. Lettieri and M. Materazzi, J. Cleaner Prod., 2021, 319, 128886 CrossRef CAS.
|
This journal is © The Royal Society of Chemistry 2024 |
Click here to see how this site uses Cookies. View our privacy policy here.