Development of diffusive gradients in thin-films with mixed binding gels for in situ monitoring of artificial sweeteners in waters†
Received
16th December 2022
, Accepted 24th April 2023
First published on 4th May 2023
Abstract
The extensive use of artificial sweeteners (ASs) has raised concerns regarding their presence, fate, and detrimental effects in water resources. It is thus of great interest for researchers and regulators to acquire a sensitive, robust, and practical sampling technique for monitoring ASs. In the present study, an effective and established passive sampling method based on the diffusive gradients in thin films (DGT) technique was proposed to measure ASs in water. A mixed binding gel of WAX-XDA1 (3
:
1, w/w) was used for the first time in DGT. The selected mixed binding gel exhibited strong and rapid adsorption of ASs and its total binding capacity was over 20.6 μg per disc. 1% (v/v) ammonium hydroxide-methanol was demonstrated to be an excellent elution solvent for ASs with the elution efficiencies being 80.2–104.9%. The linear accumulation of ASs by WAX-XDA1-DGT matched well with the theoretical values, indicating that the current configuration performs in accordance with the DGT principle. The assembled WAX-XDA1-DGT sampler possessed a good tolerance to a wide range of pH (4–8), ion strength (0–0.5 M), and dissolved organic matter (0–10 mg L−1). In wastewater, comparable or lower DGT-measured concentrations for the ASs were observed compared to the spot sampling results. To the best of our knowledge, no studies have yet been conducted to in situ monitor ASs by using the DGT-based method. This study demonstrates the robustness and reliability of WAX-XDA1-DGT for measuring ASs under a wide range of freshwater circumstances in aquatic environments.
Environmental significance
Monitoring of water quality may soon rely on in situ passive sampling devices such as the diffusive gradients in thin films (DGT) technique to measure time-weighted average concentrations in dynamic aquatic environments. However, no studies have validated the feasibility and reliability of DGT for artificial sweeteners (ASs), a group of potentially harmful pollutants. This work, for the first time, developed a new DGT sampler with WAX-XDA1 mixed resins to effectively measure ASs. The sampler was reliable and robust in a wide range of environmental conditions and provided comparable or lower measurements than spot sampling when deployed in wastewater. Overall, the WAX-XDA1-DGT sampler can serve as a complementary tool to spot sampling for large monitoring campaigns, source identification, and understanding the fate.
|
Introduction
Artificial sweeteners (ASs) are predominately used as sugar substitutes in foods, pharmaceuticals, beverages, and animal feeds with low calorific values and intense sweetening capacity.1 With the increasing demand, the global production of ASs has surged by approximately 5% per year from 2008 to 2015, with China and Latin America showing the highest growth rates.2,3 Among them, saccharin (SAC), sucralose (SUC), cyclamate (CYC), and acesulfame (ACE) are the most representative ASs (Fig. S1†), accounting for 60% of the commercial sweetener market.4–7 These ASs are not or only slightly removed/degraded during wastewater treatment processes,8 inevitably entering the aquatic environment. So far, these anthropogenic pollutants have been ubiquitous in water bodies with concentrations up to μg L−1.2,9–12 Even though ASs are typically regarded as safe to utilize, they are still under debate in the scientific community due to increasing evidence on their environmental impacts and ecotoxicity.13 At environmentally relevant concentrations (nanogram-per-litre to microgramme-per-litre), some ASs can interfere with the process of photosynthesis in plants, induce glucose intolerance in mice, or cause oxidative damage to lipids and proteins of carp (Cyprinus carpio).14–18 Due to the global occurrence and environmental persistence of ASs associated with various negative effects in water resources, ASs were recently recognized as high-priority emerging contaminants (EC).19 To gain the knowledge about the fate and possible harmful effects of these anthropogenic pollutants on both humans and aquatic ecosystems, it is crucial to better understand their presence in aquatic environments.20
To accurately determine the concentrations of ASs in aquatic systems relies on the development of efficient environmental monitoring methods. Most investigations, to date, have mostly focused on traditional spot-grab sampling to monitor pollutant concentration in aquatic systems.21 However, ECs are typically at trace concentrations and thus analytically challenging which can prevent grab sampling from application in their widespread monitoring campaigns.22 It also requires pre-concentration of a large volume of water samples for trace-level pollutants, analytes need to be preserved or quickly analysed upon collection. Moreover, it is expensive and time-consuming in terms of sample shipment and pre-treatment.23 Apart from that, the analysis results represent a snapshot of the pollutant level at a particular sampling occasion and do not reflect time-weighted average concentrations, missing pollution episodic moments.24 Therefore, researchers and regulators need to acquire a robust and practical sampling technique that is sensitive enough for these ECs.
Passive sampling has recently emerged as an alternative robust approach for water quality monitoring.25 It determines time-weighted average concentrations of pollutants which overcomes most challenges associated with traditional grab sampling.26,27 Different types of passive samplers, so far, have been designed for measurement of various pollutants in the aquatic environment, including Chemcatcher®, polar-organic chemical integrative samplers (POCIS), semipermeable membrane devices, and polyethylene devices.27,28 However, most of the available samplers have identified significant drawbacks. They are influenced by water hydrodynamic conditions and temperature, leading to uncertainty and variations in their measurements which require in situ calibration of sampling rates.29,30 In order to mitigate these issues, researchers have been developing new passive sampling techniques. Diffusive gradients in thin films (DGT) is another representative passive sampling technique that is based on the principle of Fick's first law of diffusion.31 The analyte concentrations determined by DGT (CDGT) can be expressed as eqn (1).
| 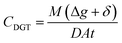 | (1) |
Here
M is the measured mass of target analytes accumulated in the binding layer, Δ
g is the thickness of the diffusive layer,
δ is the thickness of diffusive boundary layer,
D is the diffusion coefficient of target analytes in the diffusive layer,
t is the exposure time, and
A is the window area of DGT device.
The DGT regulates analyte uptake independently of water's hydrodynamic conditions and provides environmentally realistic estimates of time-averaged concentrations without field calibration.32,33 These attributes have made DGT more effective to monitor a variety of ECs. Up to now, DGT-based passive samplers have been largely developed and applied to monitor bisphenols, pesticides, illicit drugs, personal care products, etc. in numerous water bodies including seawater, wastewater, and surface water.34–39 These pioneering studies paved the way for using DGT-based samplers to monitor more new recognized ECs such as ASs in aquatic systems. Theoretically, electrostatic forces are primarily responsible for the adsorption mechanisms of ASs which also involve with both polar and apolar interactions, thus, both anion exchange resins (e.g., WAX and MAX) and neutral sorbents (e.g., HLB, XAD18, and XDA1) are candidates for the binding agents of DGT (Table S2†).
In the present study, a DGT sampler with a mixed binding gel of WAX-XDA1 (3
:
1, w/w) was designed and developed for the first time. The robustness and reliability of WAX-XDA1-DGT for monitoring ASs was evaluated under both laboratory and field conditions. The adsorption kinetics and capacity of binding gels were explored. The best elution strategy and efficiencies were determined. The performance of the new DGT device including time-dependant uptake kinetics and effects of key water chemistry factors were also systematically assessed. Finally, the applicability of DGT devices in the field was evaluated by comparison with grab sampling. To the best of our knowledge, no studies have yet been conducted to in situ monitor ASs by using the DGT-based method.
Experimental section
Chemicals and reagents
SAC, SUC, CYC, ACE, and their internal standards (SUC-d6, SAC-d4, CYC-d11, and ACE-d4) were provided by ANPEL Scientific Instrument Co. Ltd (Shanghai, China). Their physicochemical characteristics and structures are listed in Table S1.† Their purities were all above 98%. Methanol and acetonitrile (HPLC grade) were supplied by Merck (Darmstadt, Germany). Ammonium hydroxide and sodium chloride were purchased from Aladdin Reagent Inc. (Shanghai, China). Ammonium acetate (UPLC-MS/MS grade) was obtained from Sigma-Aldrich Chemie GmbH. The filter membranes utilized were supplied by ANPEL Scientific Instrument Co. Ltd (Shanghai, China). The DOM (humic acid) was supplied by the international Humic Substance Society. Milli-Q water was obtained from a Milli-Q purification system (ELGA, Antony, France). The adsorption resins (MAX, WAX, HLB, XAD18, and XDA1) were purchased from Green Union Science Instrument Co. Ltd (China) and were conditioned with methanol and rinsed thoroughly with Milli-Q water before use. ASs stock solutions were prepared in methanol at 100 mg L−1 and stored in sealed glass bottles at −20 °C.
Diffusive and binding gel preparation
The agarose and polyacrylamide diffusive gels (0.8 mm thickness) used here were prepared as previously described.40 Similarly, the binding hydrogels with a thickness of 0.5 mm were prepared by using 1.5% (w/v) agarose solution and different adsorption resins (MAX, WAX, HLB, XAD18, and XDA1). For the WAX-XDA1 mixed binding gels, 3 g wet weight WAX resin and 1 g wet weight XDA1 resin were weighed and mixed into 10 mL preheated agarose solution. The following procedures were the same as the preparation of other binding gels. All gel discs (2.5 cm diameter) were hydrated in Milli-Q water and then stored in 0.01 M NaCl solution at 4 °C.
Adsorption assessment of DGT components
A series of filter membranes, including polyethersulfone (PES), polytetrafluoroethylene (PTFE), polyvinylidene difluoride (PVDF), GHP, and nylon, were tested to assess the possible adsorption of target ASs, as well as the diffusive and binding gels prepared above. In brief, the test materials were soaked in 10 mL of 100 μg L−1 AS mixed solution and shaken at 180 rpm for 24 h on a horizontal shaker (THZ-100, BLUEPARD, Shanghai, China). Blank and control samples were prepared with Milli-Q water and AS solution, respectively. The ASs concentrations in the solutions before and after exposure were analysed by UPLC-MS/MS and the adsorption percentages by the test materials were calculated.
Adsorption kinetics, elution efficiencies, and binding capacity of ASs by binding gels
The binding gels selected above (i.e., WAX-XDA1 gels) were soaked in a 50 mL polypropylene tube containing 10 mL of 100 μg L−1 AS solution and vigorously shaken by a horizontal oscillator for 24 h. A control sample was prepared as well without the binding gel to examine any potential adsorption of analytes. 100 μL of samples were collected at 10 min, 30 min, 60 min, 2 h, 4 h, 8 h, 10 h, 18 h, and 24 h. The adsorbed masses of ASs by the gel disc were calculated by the difference between their initial concentrations and the concentrations measured at different time intervals in the bulk solution.
After 24 h of adsorption, the binding gel adsorbed ASs was transferred immediately into a 15 mL polypropylene tube, spiked with 100 ng of IS, and eluted in an ultrasonic bath. The elution strategies were improved with different elution solvents including methanol and acetonitrile with or without ammonium hydroxide. The optimal elution strategy for ASs was determined by ultrasonic extraction in 5 mL of methanol containing 1% ammonium hydroxide (v/v) for 30 min. The elution efficiencies of ASs were calculated by using the following equation:
| 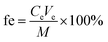 | (2) |
Here,
Ce represents the measured concentration of ASs in the elution solution,
Ve is the volume of the elution solution, and
M is the adsorbed mass of ASs by the binding gel.
To assess if the selected binding gels have high enough adsorption capacities for ASs to ensure DGT uptake is in its linear accumulation regime during the field deployment, triplicate WAX-XDA1 gels were immersed in a polypropylene tube containing 10 mL of 0.01 M NaCl solution at different ASs concentrations (0.5, 1, 2 and 5 mg L−1). The tubes were shaken horizontally at 2000 oscillations per minute. The gels were transferred to a 15 mL tube after 24 h and eluted as previously described. Aliquots of the elution solution was pipetted and 50 ng of IS was added for LC-MS/MS analysis. Meanwhile, the ASs concentrations were monitored before and after.
Diffusion coefficients measurement
The diffusion coefficient (D) is the sole parameter required prior to the calculation of CDGT. A two-compartment diffusion cell was used to measure the D values of ASs as reported previously.41 One diffusive gel disc (0.88 mm thickness) was stuck in the circular window (1.5 cm diameter) of the two compartments of the cell. 50 mL of 0.01 M NaCl solution containing 25 μg ASs was added to the source compartment, and simultaneously, the receptor compartment was filled with the same volume of 0.01 M NaCl solution containing no ASs. The experiment temperature was maintained at 23.2 ± 0.5 °C and the solutions in both compartments were stirred continuously using a stirrer. 100 μL of solutions were taken and analysed from both the source and receptor compartments in intervals of 15 min. The D was then calculated by using eqn (3): |  | (3) |
where Δg is the diffusive gel thickness, C is the ASs concentrations in the source compartment, and A is the window area of the diffusion cell. The slope was determined by the diffusion mass of ASs versus time. The diffusion coefficients at other temperatures (DT) can be derived from eqn (4). |  | (4) |
DGT uptake kinetics
To examine whether DGT uptake was linear or approaching equilibrium in a normal deployment timeframe, the assembled DGT devices were submerged in 2 L of well-stirred 50 μg L−1 ASs solution containing 0.01 M NaCl. Aliquots of 100 μL of test solutions were collected before and after DGT deployment and ASs concentrations in bulk solution were measured. Triplicate DGT devices were retrieved after 24, 48, 72, 96, and 120 h. The binding gels were peeled off and extracted according to the procedure described above.
Effects of ionic strength (IS), pH and dissolved organic matter (DOM)
Ionic strength (IS), pH and dissolved organic matter (DOM) are considered as the major water chemistry factors that might affect the DGT measurements. To understand their effects on the performance of WAX-XDA1 DGT, a series of single factor experiments were carried out. In brief, test solutions (1 L) were prepared with 50 μg ASs under different conditions: (i) NaCl concentrations (0, 0.001, 0.01, 0.1 and 0.5 M) (ii) various pH values (5–8), (iii) DOM concentrations (0, 5, 8, and 10 mg L−1). The solution pH was adjusted by using hydrochloric acid and sodium hydroxide. The assembled DGT devices were immersed in the test solutions after 24 h of mixing. During the DGT deployment, the solutions were well mixed by using a magnetic stirrer and the solution concentrations of ASs were measured to check if they were stable. The solution temperature was kept at 23 ± 2 °C. After 24 h, the retrieved DGT devices were processed as described above.
Field application/validation
The field validation of assembled DGT devices was conducted in the influent of a municipal wastewater treatment plant in Southern China over a period of 14 days in August 2022. A total of 12 DGT devices were deployed and retrieved on the 3rd, 6th, 10th, and 13th days. Additional three DGT samplers were also deployed as flied blanks. At the same time, 500 mL of wastewater samples were collected in duplicate with 50 μL of 4 M sulfuric acid immediately being added. The physicochemical properties of the wastewater were measured on-site during the DGT deployment. The temperature and pH value of raw wastewater were recorded daily. The surface of the samplers was cleaned with Milli-Q water, the binding gels were removed and eluted in the laboratory as described above (Section 2.4). The analytes of interest in the grab samples were extracted by using a Chromabond HR-X SPE cartridge (6 mL, 500 mg) as reported elsewhere.42
Instrumental analysis
Chemical analysis was carried out using ultra-performance liquid chromatography (UPLC ACQUITY, Waters, Milford, MA, USA) equipped with a triple quadrupole tandem mass spectrometer (Xevo QTS, Waters, Milford, MA, USA) with an electrospray ionization interface source in a negative mode (ESI−). An ACQUITY UPLC BEH C18 (2.1 mm 50 mm, 1.7 mm) column was utilized for quick separation. The mobile phases were (A) 2 mM ammonium acetate in MQ water and (B) acetonitrile flowing at 0.5 mL min−1. The gradient began at 10% B and held for 2.5 min, ramped to 100% B in 0.1 min, held for 1 min, and then went back to 10% B in 0.1 min to re-establish equilibrium for 0.8 min. The injection volume was 5 μL. The column temperatures were 40 °C. All samples were analysed in triplicates.
Results and discussion
Adsorption of ASs by DGT components
It is critical to select appropriate diffusive gels and filter membranes for DGT samplers to prevent the undesired adsorption of target chemicals, which can affect the accurate measurement of ambient concentrations. Data in Fig. 1A shows that the PES filter exhibited the lowest adsorption (<20%) for all the ASs investigated among the test five filter membranes. For the other four filters (GHP, PTFE, PVDF, and NL), at least one AS was substantially adsorbed and the PVDF membrane exhibited the maximum adsorption percentages across the ASs. As a result, the PES filter membranes were selected as the most suitable and used in the following experiments. In addition, the average adsorption percentages of ASs by both agarose and polyacrylamide diffusive gels were less than 10% (Fig. 1A). In the present study, agarose diffusive gel was selected as the diffusive layer finally because of its easier operation and cheaper reagents than polyacrylamide diffusive gel.
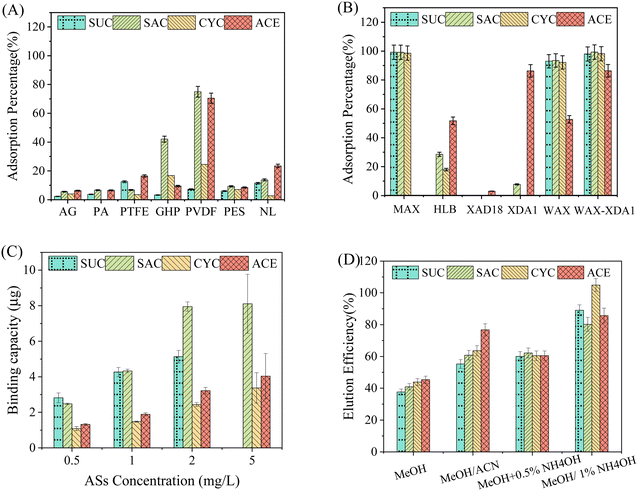 |
| Fig. 1 Plot (A) adsorption percentages (%) of selected ASs on diffusive gels (AG and PA) and membrane filters (PTFE, GHP, PVFD, PES, and NL), respectively. Plot (B) adsorption percentages (%) of selected ASs onto different resin gels in 10 mL of 100 μg L−1 AS solution. Plot (C) Measured masses (μg) of ASs on the WAX-XDA1 gels immersed in well-stirred solutions at various concentrations (0.5–5 mg L−1). The data on the binding capacity for SUC at 5 mg L−1 (Plot C) is not available because of unintentional sample loss. Plot (D) extraction efficiencies (%) of ASs eluted from the WAX-XDA1 gels by using different elution solvents. Error bars: 1 SD (n = 3). SUC: Sucralose, SAC: Saccharin, CYC: Cyclamate, ACE: Acesulfame, AG: agarose gels, PA: polyacrylamide gel, PTFE: polytetrafluoroethylene filter, PVFD: polyvinylidene difluoride filter, PES: polyether sulfone filter, NL: nylon filter, MeOH: methanol, ACN: acetonitrile. | |
As for the binding gels, the adsorption of five adsorbent resins were assessed (Fig. 1B). The neutral resins, namely XDA1, XAD18, and HLB, adsorbed ACE but performed less well for the other three ASs. Overall, the binding affinity of these neutral resins followed the order XDA1 > HLB > XAD18. The poor performance of HLB resin for ASs was also reported by Sultana and Metcalfe.43 The superior binding of XDA1 resin might be due to its higher BET surface area (∼1100 m2 g−1, Table S2†). In contrast, for the anion exchange resins, namely WAX and MAX, they yielded almost 100% of adsorption for SUC, SAC, and CYC after 24 h. The WAX resin adsorbed about 60% of ACE although the MAX resin failed completely. Similarly, another weak anion exchange resin, Strata X-AW, was recently demonstrated to be an excellent binding resin for POCIS to monitor ASs.43 A plausible explanation is that the ASs are strong acids present in the anionic form at the pH of Milli-Q water used (∼6.3) and thus are easily captured by the anion exchange resins rather than the neutral resins. To enhance the binding affinity to ACE, a mixed binding gels with WAX and XDA1 (3
:
1, w/w) was designed and tested for the first time. The adsorption results suggested that the mixed binding gels (called WAX-XDA1) had the strongest adsorption ability for all the ASs including ACE (Fig. 1B) and thus be selected as the binding layer of DGT sampler in this study.
Adsorption kinetics and capacity of ASs on WAX-XDA1 binding gels
An ideal binding gel for DGT should adsorb analytes of interest quickly and strongly enough.44 Herein, the adsorption kinetics of ASs on WAX-XDA1 binding gels were studied. As illustrated in Fig. S2,† the amount of ASs adsorbed on WAX-XDA1 gels linearly increased in the initial several hours and achieved approximately 80% of the total mass, with the exception of SUC (65%). After 4 h of adsorption, the kinetic curve gradually flattened and adsorption equilibrium occurred at the tenth hour for SAC, ACE, and CYC and at the 18th hour for SUC. The maximum adsorption percentages were 83% for SUC and close to 100% for the other ASs. Dong et al. (2014) suggested that the binding rate of analytes should be much larger than the ratio of diffusion coefficients through the diffusion layer over the square of its thickness (i.e., k ≫ D/Δg2) in order to ensure DGT performs well.45 To examine the relationship between the diffusion layer and the binding layer, the initial adsorption rates of ASs on WAX-XDA1 gels were further estimated by using a pseudo first-order model. We found that they (0.01–0.03 s−1) were two orders of magnitude higher than the values of D/Δg2 (2 × 10−4–3 × 10−4 s−1), meeting the basic requirement of the DGT principle. This means that the WAX-XDA1 gels exhibit strong and fast adsorption of ASs, and no reverse-diffusion occurring once ASs diffuse across the diffusive layer. It is, therefore, clear that a steady diffusion gradient would be well established during DGT sampling.
The adsorption of binding gels is concentration dependent and the adsorption capacities of ASs on WAX-XDA1 gels were thus estimated at 5 mg L−1 (Fig. 1C). The WAX-XDA1 gels exhibited the highest adsorption for SAC (≥8.1 μg per disc). The following was SUC with an adsorption capacity more than 5 μg per disc. The adsorption capacities of CYC and ACE were relatively low (≥3.4 μg per disc) but adequate even if the DGT devices are deployed in extreme scenarios. Assuming the environmental concentration is ∼10 μg L−1 and the deployment time is less than 20 days, the theoretical mass accumulated by the DGT device was about 1.0–1.2 μg per disc, which is three-fold lower than the lowest adsorption capacity of WAX-XDA1 gels. The total adsorption capacity of ASs on WAX-XDA1 gels exceeded 20.6 μg per disc.
Elution efficiencies and diffusion coefficients of ASs
An efficient elution method for target chemicals from the binding layer is required to ensure that they are accurately measured by DGT, which is thought to be the most significant source of uncertainty.46 To get the most effective elution method and the best elution efficiency, two common organic solvents (methanol and acetonitrile) alone or with ammonium hydroxide were tested. The elution results of ASs are shown in Fig. 1D. Notably, methanol containing a small quantity of ammonium hydroxide is more effective than methanol alone and methanol-acetonitrile (1
:
1, v/v) for extracting ASs. Furthermore, more analytes were eluted when the content of ammonium hydroxide increased from 0.5% to 1%. This was probably due to the ammonium hydroxide increasing the elution power of methanol by neutralizing the surface positive charges of WAX resin. When 1% (v/v) ammonium hydroxide-methanol was applied, all the ASs investigated exhibited acceptable extraction efficiencies ranging from to 80.2% to 104.9%. Parallel elution in triplicate showed good repeatability across the target ASs with coefficients of variation (CV) less than 5%.
The diffusion coefficient (D) is the sole intrinsic variable in the eqn (1) that needs to be determined in the laboratory prior to deployment. To measure the D values of ASs, their time-dependant diffusive mass transfer through the agarose diffusive layer was quantified and described in Fig. S3.† The diffusive masses increased linearly (R2 = 0.980–0.988) with time and thus the slopes were obtained to calculate the D values. The calculated D values at 23 °C were (1.84 ± 0.05) × 10−6 cm2 s−1, (1.68 ± 0.03)×10−6 cm2 s−1, (1.52 ± 0.05) × 10−6 cm2 s−1, and (1.64 ± 0.06) × 10−6 cm2 s−1 for SAC, SUC, CYC, and ACE respectively. Their DGT sampling rates (Rs), derived from the temperature-specific D values (i.e., Rs = D/Δg), ranged from 1.64 mL d−1 cm−2 to 1.99 mL d−1 cm−2. For comparison, POCIS sampling rates for SUC and ACE, recently reported by Sultana and Metcalfe,43 were adjusted by surface area which is much greater than that of DGT sampler (∼42 cm2versus 3.14 cm2). We found that the Rs values of SUC (1.81 mL d−1 cm−2) in the current study were slightly higher than its previously reported value (1.43 mL d−1 cm−2) whereas it was the opposite for ACE (1.77 versus 3.05 mL d−1 cm−2). Besides the effect of experimental temperature, the discrepancy in sampling rates can most likely be attributed to the different uptake mechanisms of two types of passive samplers. For DGT, the diffusive layer governs how much analytes accumulate, which is however cancelled in POCIS and actually the binding resin controls the uptake process.
Time-dependant uptake of ASs by WAX-XDA1-DGT
Another important aspect of an assembled DGT sampler is to validate whether a linear uptake regime exists and how long it lasts during DGT sampling. The time-dependent uptake of ASs by the WAX-XDA1-DGT devices is shown in Fig. 2. For all the ASs investigated, good linear correlations between accumulation mass and deployment time (R2 = 0.997–0.999) were observed up to 5 days and there was no evidence of accumulation equilibrium, confirming the existence of a linear uptake regime in the WAX-XDA1-DGT sampler. Moreover, the DGT-accumulated masses were in good agreement with the theoretically calculated values (indicated by red lines in Fig. 2), implying that the current DGT configuration performs in accordance with the principle and mechanism of DGT for measuring ASs. It is noteworthy that the entirely different uptake patterns for the assembled WAX-XDA1-DGT sampler and the WAX-XDA1 binding gels (see Fig. S2†) implies that the diffusive layer governs how much the analytes accumulate in DGT rather than the binding layer.
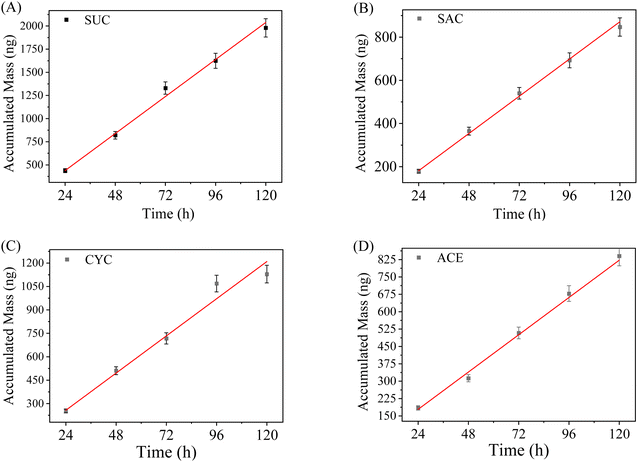 |
| Fig. 2 Accumulated masses (M) of SUC (A), SAC (B), CYC (C), and ACE (D) by WAX-XDA1-DGT devices deployed in 2 L of 50 μg L−1 AS solutions containing 0.01 M NaCl (23 ± 0.4 °C). The solid red lines demonstrate the theoretical lines predicted by the equation: M = DCAT/Δg (C is the concentration of the solution, A is the exposed window area of the DGT, t represents the deployment duration and Δg is the diffusive layer thickness.) Error bars: 1 SD (n = 3). SUC: Sucralose, SAC: Saccharin, CYC: Cyclamate, ACE: Acesulfame. | |
Effects of pH, ionic strength (IS), and dissolved organic matter (DOM)
Prior to field validation, it is essential to analyse the application ranges of key water chemistry factors for the assembled WAX-XDA1-DGT sampler because they can have an influence on DGT performance. Facts such as pH, IS, and DOM are the most significant.47,48 In the present study, the effects of solution pH, IS, and DOM concentrations on the ratios of the DGT-measured concentration to the solution concentration (CDGT/CW) have been explored and depicted in Fig. 3.
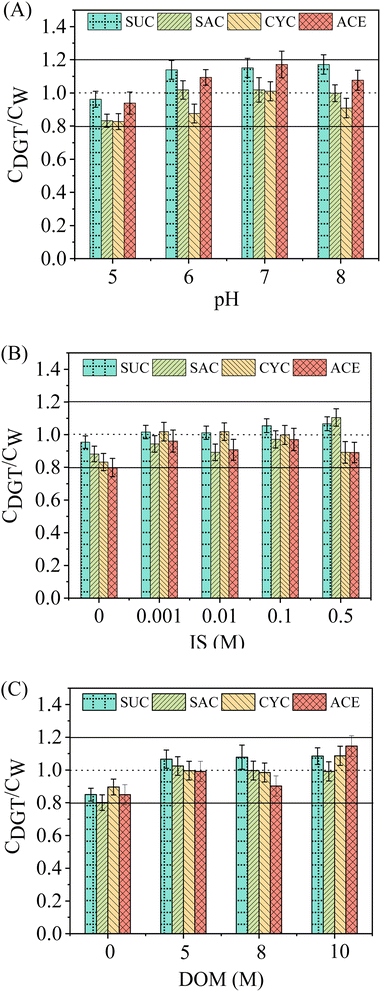 |
| Fig. 3 Effects of pH (A), ionic strength (B), and DOM (C) on the ratios of ASs concentrations measured by WAX-XDA1-DGT devices to the solution concentrations (CDGT/CW). The dotted horizontal lines represent the values at 1 and the solid horizontal lines represent the values at 0.8 and 1.2. Error bars: 1 SD (n = 3). SUC: Sucralose, SAC: Saccharin, CYC: Cyclamate, ACE: Acesulfame, DOM: dissolved organic matters. | |
For the effect of pH, the CDGT/CW values of ASs were slightly elevated when the solution pH was increased from 5 to 6 (Fig. 3A). The increments, however, were supressed when the solution pH varied from 6 to 8. This was to be expected as the ASs investigated would be fully deprotonated and exist in the anionic form in the pH range of 5–8, given their very low pKa values (Table S1†). However, from the perspective of binding resins, the pKa value of WAX resin is ∼6 (Table S2†), namely, half of the sorbents are positively charged at pH 6 and this percentage shifts from 91% at pH 5 to less than 1% at pH 8. This shift could be considered to result in a change in the adsorption mechanism of ASs, however, since the amount of WAX resin was greater (∼100 mg per disc) than the total mass of ASs in the solution (200 μg), it was still sufficient for the adsorption of the anionic ASs on the binding layer by electrostatic interactions when the ionic forms of sorbents account for only 1%. This might explain why, at pH ≥ 6 at which the WAX resins are poorly ionized while the ionic forms of ASs dominate in the solution, the electrostatic specific interactions were still significant, and therefore minor overestimation of DGT measurements was observed at the higher pH. The similar phenomenon was investigated by Becskereki et al., who elucidated the adsorption mechanisms of ionizable weak acids and bases on the OASIS® HLB resins around neutral pH (∼7).49 Apart from that, non-electrostatic interactions such as van der Waals forces and hydrogen bonding were also involved and facilitated the adsorption of ASs in the solution.50
The CDGT/CW values in the presence of sodium chloride were slightly higher than those in the background solution (Fig. 3B). The overestimation of ASs concentrations by DGT in the presence of sodium chloride could be explained by the salting-out effect.51 The addition of sodium chloride effectively reduced the solubility of ASs in the aqueous phase whilst enhancing the interaction between the ASs and the sorbent. Nevertheless, the effect of IS appeared not to be noticeable with further increase in sodium chloride concentrations. This phenomenon was due to the increasing sample viscosity as the IS increased, thereby leading to the obstruction of mass transfer of the ASs from the solution to the DGT.52,53
Similar to the effect of IS, DGT measurements were slightly overestimated in the presence of DOM (Fig. 3C). However, elevated DOM concentrations resulted in little change in the CDGT/CW values for all the ASs. In general, interactions between DOM and organic pollutants in solution and competition of DOM for sorption sites on binding gels result in reduced sorption of the solute. In contrast, cumulative sorption and cosorption processes will enhance sorption to the binding gels. The net effect of DOM on the sorption of organic pollutants to sorbents is the sum of the abovementioned processes.54 Therefore, we hypothesize that the increased accumulation of ASs by DGT in the presence of DOM was caused by either strong chemical interactions between ASs and the adsorbed DOM or by physical encapsulation of ASs in DOM–sorbent complexes.
Overall, regardless of how the solution pH, IS, and DOM concentrations varied, DGT measurements were in good agreement with the solution concentrations and the ratios of CDGT to CW ranged from 0.8 to 1.2 (CVs less than 20%). This demonstrated that these factors have little influence, over the ranges investigated, on the WAX-XDA1-DGT performance in measuring concentrations of ASs. Therefore, the assembled WAX-XDA1-DGT sampler in this study possesses a highly desirable tolerance to a wide range of pH (5–8), IS (0.001–0.5 M), and DOM concentrations (0–10 M).
Field trial in WWTPs
The robustness and reliability of WAX-XDA1-DGT under field conditions were investigated by comparison with the traditional spot sampling method. The results of spot sampling revealed that the ASs studied were detected in all the influent samples. The spot sampling concentrations varied from (3.5 ± 0.9) μg L−1 to (17.1 ± 2.1) μg L−1 and followed the order: SUC > ACE > CYC > SAC. The AS levels present in the current study were consistent with those found in US and Canadian wastewaters.55,56 The measured concentrations for all the ASs were relatively steady over the 14 day campaign except on the 3rd day indicating consistent presence in domestic wastewater. The influent concentrations on the 3rd day declined by 41%, 41%, 35%, and 43% for SUC, SAC, CYC, and ACE, respectively. This could be explained by the strong dilution effect of a sudden rainstorm.
With respect to the DGT results, the uptake masses by the DGT increased rapidly within the first 7 days but were stable or slightly decreased over the next week. Moreover, a substantial biofouling on the surface of deployed DGT was observed on the 11th and 13th days. Thus, the nonlinear DGT uptake might be attributed to the biofouling. In addition, the method detection limits (MDL) of DGT, estimated from the blank DGT going through the whole procedure, were 0.07–0.13 ng L−1 and significantly lower than the limits reported by spot sampling,5 suggesting that it is sensitive enough to deploy the WAX-XDA1-DGT sampler in water even for one day. Considering the environmental concentrations of ASs (up to several micrograms per litre), the sufficiently low MDLs and the potential biofouling of DGT, the suitable deployment timeframe for the WAX-XDA1-DGT sampler is between 1 and 7 days.
For the aforementioned reasons, the DGT-measured concentrations of ASs were conservatively calculated from the 3 day uptake mass by WAX-XDA1-DGT and then used for comparison with the spot sampling results. It was found that the DGT-measured concentrations for SUC were comparable to the spot sampling results while much higher concentrations for ACE, CYC, and SAC were observed in the grab samples (Fig. 4). This observation was anticipated and previously reported by other studies,57–59 and can be explained by discrepancies between spot sampling and DGT technique. It is acknowledged higher fractions of the ASs in the grab samples were available for the subsequent SPE extraction (extraction recoveries: 78.4–93.8%) after the pH value was adjusted from 7.8 to 2.0, whilst, only labile fractions in water can be accumulated through the DGT in situ. DGT measurements represent time-integrated average concentrations over the whole sampling period whereas spot sampling offers discrete snapshots at specific moments which might miss or only record peak concentrations of pollutants. Take this study for example, all the grab sampling occurred at noon when the domestic wastewater might contain high-concentration ASs due to lunchtime activities.
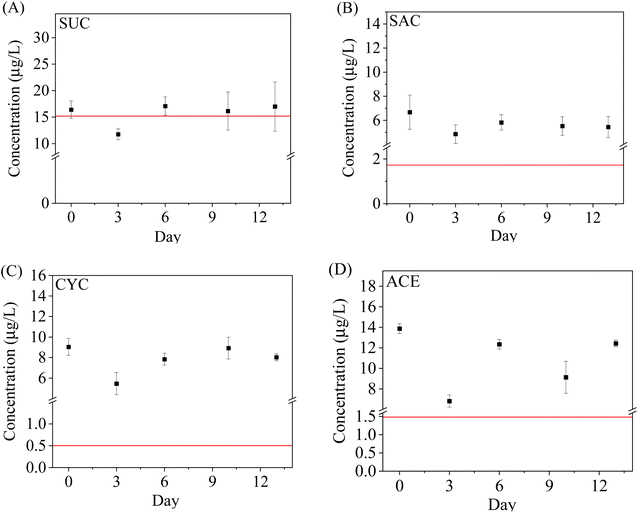 |
| Fig. 4 Comparison of the concentrations of SUC (A), SAC (B), CYC (C), and ACE (D) measured by the DGT method and the grab sampling method. The red line means the concentrations measured by the DGT method within three days, while the black dots represent the concentrations of ASs measured by the grab sampling method. Error bar: 1 standard deviation (n = 3). SUC: Sucralose, SAC: Saccharin, CYC: Cyclamate, ACE: Acesulfame. | |
Conclusions
Monitoring of water quality may soon rely on field deployable devices to in situ measure potentially harmful pollutants that integrate concentration of chemicals in dynamic aquatic environments with fluctuating concentrations. This work has demonstrated the applicability and validity of DGT with WAX-XDA1 mixed binding gel as a promising technique to effectively measure widely used ASs in situ and provide their time-integrated average concentrations. The newly developed WAX-XDA1-DGT sampler was found to be easy to deploy, and robust for in situ monitoring of ASs in a variety of environmental conditions in aquatic environments. Its field deployment in wastewater suggested that relatively lower or comparable results were obtained by the WAX-XDA1-DGT sampler compared to grab sampling.
In contrast to grab sampling, the DGT technique has a novel feature as analytes are precleaned by the filter membrane and diffusive gel while diffusing through them, whilst grab samples require additional clean-up in the laboratory. Monitoring by DGT samplers overwhelms the safety issues in extreme unprecedented weather conditions and provides important data regarding the episodic discharges of the target chemicals as it measures time-weighted average concentrations. Overall, the DGT-based method can be considered complementary to spot sampling for the measurement of ASs in water, although it is faster, easier, and more sensitive.
Author contributions
Conceptualization, C. E. Chen; methodology and validation, I. Hussain, Q. S. Cai, Z. X. Zhao, S. M. Cheng and J. X. Zi; data curation, Q. S. Cai, S. S. Liu and J. X. Zi; writing—original draft preparation, S. S. Liu; writing—review and editing, S. S. Liu, C. E. Chen, G. G. Ying and A. J. Sweetman; supervision, C. E. Chen; funding acquisition and resources, S. S. Liu and C. E. Chen. All authors have read and agreed to the published version of the manuscript.
Conflicts of interest
The authors declare no competing financial interests for all submitted manuscripts.
Acknowledgements
This work was supported by the National Natural Science Foundation of China (22006042 and 42277457), the Guangdong Basic and Applied Basic Research Foundation (2019A1515110829, 2023A1515011515), the Start-up Funding of Outstanding Young Scientist from SCNU (for CE Chen), the Young Talent Support Project of Guangzhou Association for Science and Technology (for SS Liu), and Guangdong Provincial Key Laboratory of Chemical Pollution and Environmental Safety (2019B030301008).
References
- M. G. Kokotou and N. S. Thomaidis, Determination of eight artificial sweeteners in wastewater by hydrophilic interaction liquid chromatography-tandem mass spectrometry, Anal. Methods, 2013, 5, 3825–3833 RSC.
- D. Li, Y. Yao, H. Sun, Y. Wang, J. Pu, R. Calderón, A. C. Alder and K. Kannan, Artificial sweeteners in pig feed: a worldwide survey and case study in pig farms in Tianjin, China, Environ. Sci. Technol., 2020, 54, 4059–4067 CrossRef CAS PubMed.
- R. M. Baena-Nogueras, J. M. Traverso-Soto, M. Biel-Maeso, E. Villar-Navarro and P. A. Lara-Martín, Sources and trends of artificial sweeteners in coastal waters in the bay of Cadiz (NE Atlantic), Mar. Pollut. Bull., 2018, 135, 607–616 CrossRef CAS PubMed.
- B. Subedi and K. Kannan, Fate of artificial sweeteners in wastewater treatment plants in New York State, USA, Environ. Sci. Technol., 2014, 48, 13668–13674 CrossRef CAS PubMed.
- F. T. Lange, M. Scheurer and H.-J. Brauch, Artificial sweeteners—a recently recognized class of emerging environmental contaminants: a review, Anal. Bioanal. Chem., 2012, 403, 2503–2518 CrossRef CAS PubMed.
- Z. Gan, H. Sun, Y. Yao, Y. Zhao, Y. Li, Y. Zhang, H. Hu and R. Wang, Distribution of artificial sweeteners in dust and soil in China and their seasonal variations in the environment of Tianjin, Sci. Total Environ., 2014, 488, 168–175 CrossRef PubMed.
- M. E. Hoque, F. Cloutier, C. Arcieri, M. McInnes, T. Sultana, C. Murray, P. A. Vanrolleghem and C. D. Metcalfe, Removal of selected pharmaceuticals, personal care products and artificial sweetener in an aerated sewage lagoon, Sci. Total Environ., 2014, 487, 801–812 CrossRef CAS PubMed.
- N. H. Tran, J. Hu, J. Li and S. L. Ong, Suitability of artificial sweeteners as indicators of raw wastewater contamination in surface water and groundwater, Water Res., 2014, 48, 443–456 CrossRef CAS PubMed.
- O. S. Keen and K. G. Linden, Re-engineering an artificial sweetener: transforming sucralose residuals in water via advanced oxidation, Environ. Sci. Technol., 2013, 47, 6799–6805 CrossRef CAS PubMed.
- Y.-Y. Yang, W.-R. Liu, Y.-S. Liu, J.-L. Zhao, Q.-Q. Zhang, M. Zhang, J.-N. Zhang, Y.-X. Jiang, L.-J. Zhang and G.-G. Ying, Suitability of pharmaceuticals and personal care products (PPCPs) and artificial sweeteners (ASs) as wastewater indicators in the Pearl River Delta, South China, Sci. Total Environ., 2017, 590, 611–619 CrossRef PubMed.
- M. Ruff, M. S. Mueller, M. Loos and H. P. Singer, Quantitative target and systematic non-target analysis of polar organic micro-pollutants along the river Rhine using high-resolution mass-spectrometry–Identification of unknown sources and compounds, Water Res., 2015, 87, 145–154 CrossRef CAS PubMed.
- G. A. Stefania, M. Rotiroti, I. J. Buerge, C. Zanotti, V. Nava, B. Leoni, L. Fumagalli and T. Bonomi, Identification of groundwater pollution sources in a landfill site using artificial sweeteners, multivariate analysis and transport modeling, Waste Manage., 2019, 95, 116–128 CrossRef CAS PubMed.
- K. R. Tandel, Sugar substitutes: Health controversy over perceived benefits, J. Pharmacol. Pharmacother., 2011, 2, 236–243 CrossRef CAS PubMed.
- A. Reinders, A. B. Sivitz, A. Hsi, C. P. Grof, J. M. Perroux and J. M. Ward, Sugarcane ShSUT1: analysis of sucrose transport activity and inhibition by sucralose, Plant, Cell Environ., 2006, 29, 1871–1880 CrossRef CAS PubMed.
- K. Saucedo-Vence, A. Elizalde-Velázquez, O. Dublán-García, M. Galar-Martínez, H. Islas-Flores, N. SanJuan-Reyes, S. García-Medina, M. D. Hernández-Navarro and L. M. Gómez-Oliván, Toxicological hazard induced by sucralose to environmentally relevant concentrations in common carp (Cyprinus carpio), Sci. Total Environ., 2017, 575, 347–357 CrossRef CAS PubMed.
- J. Suez, T. Korem, D. Zeevi, G. Zilberman-Schapira, C. A. Thaiss, O. Maza, D. Israeli, N. Zmora, S. Gilad and A. Weinberger, Artificial sweeteners induce glucose intolerance by altering the gut microbiota, Nature, 2014, 514, 181–186 CrossRef CAS PubMed.
- C. Amy-Sagers, K. Reinhardt and D. M. Larson, Ecotoxicological assessments show sucralose and fluoxetine affect the aquatic plant, Lemna minor, Aquat. Toxicol., 2017, 185, 76–85 CrossRef CAS PubMed.
- Z. Sang, Y. Jiang, Y.-K. Tsoi and K. S.-Y. Leung, Evaluating the environmental impact of artificial sweeteners: a study of their distributions, photodegradation and toxicities, Water Res., 2014, 52, 260–274 CrossRef CAS PubMed.
- J. Luo, Q. Zhang, M. Cao, L. Wu, J. Cao, F. Fang, C. Li, Z. Xue and Q. Feng, Ecotoxicity and environmental fates of newly recognized contaminants-artificial sweeteners: A review, Sci. Total Environ., 2019, 653, 1149–1160 CrossRef CAS PubMed.
- S. Behmel, M. Damour, R. Ludwig and M. Rodriguez, Water quality monitoring strategies—A review and future perspectives, Sci. Total Environ., 2016, 571, 1312–1329 CrossRef CAS PubMed.
-
W. Davison, Diffusive Gradients in Thin-Films for Environmental Measurements, Cambridge University Press, 2016 Search PubMed.
- L. Mutzner, E. L. Vermeirssen and C. Ort, Passive samplers in sewers and rivers with highly fluctuating micropollutant concentrations–better than we thought, J. Hazard. Mater., 2019, 361, 312–320 CrossRef CAS PubMed.
- Y.-T. Zou, Z. Fang, Y. Li, R. Wang, H. Zhang, K. C. Jones, X.-Y. Cui, X.-Y. Shi, D. Yin and C. Li, Novel method for in situ monitoring of organophosphorus flame retardants in waters, Anal. Chem., 2018, 90, 10016–10023 CrossRef CAS PubMed.
- J.-L. Zheng, D.-X. Guan, J. Luo, H. Zhang, W. Davison, X.-Y. Cui, L.-H. Wang and L. Q. Ma, Activated charcoal based diffusive gradients in thin films for in situ monitoring of bisphenols in waters, Anal. Chem., 2015, 87, 801–807 CrossRef CAS PubMed.
- A. Kot-Wasik, B. Zabiegała, M. Urbanowicz, E. Dominiak, A. Wasik and J. Namieśnik, Advances in passive sampling in environmental studies, Anal. Chim. Acta, 2007, 602, 141–163 CrossRef CAS PubMed.
- F. Salim and T. Górecki, Theory and modelling approaches to passive sampling, Environ. Sci.: Processes Impacts, 2019, 21, 1618–1641 RSC.
- R. Wang, E. Biles, Y. Li, M. D. Juergens, M. J. Bowes, K. C. Jones and H. Zhang, In situ catchment scale sampling of emerging contaminants using diffusive gradients in thin films (DGT) and traditional grab sampling: a case study of the River Thames, UK, Environ. Sci. Technol., 2020, 54, 11155–11164 CrossRef CAS PubMed.
- D. Zhang, Y. Zhu, X. Xie, C. Han, H. Zhang, L. Zhou, M. Li, G. Xu, L. Jiang and A. Li, Application of diffusive gradients in thin-films for in-situ monitoring of nitrochlorobenzene compounds in aquatic environments, Water Res., 2019, 157, 292–300 CrossRef CAS PubMed.
- C. Harman, I. J. Allan and E. L. Vermeirssen, Calibration and use of the polar organic chemical integrative sampler—a critical review, Environ. Toxicol. Chem., 2012, 31, 2724–2738 CrossRef CAS PubMed.
- S. L. Kaserzon, D. W. Hawker, K. Booij, D. S. O'Brien, K. Kennedy, E. L. Vermeirssen and J. F. Mueller, Passive sampling of perfluorinated chemicals in water: In-situ calibration, Environ. Pollut., 2014, 186, 98–103 CrossRef CAS PubMed.
- Z. Fang, Y. Li, Y. Li, D. Yang, H. Zhang, K. C. Jones, C. Gu and J. Luo, Development and applications of novel DGT passive samplers for measuring 12 per-and polyfluoroalkyl substances in natural waters and wastewaters, Environ. Sci. Technol., 2021, 55, 9548–9556 CrossRef CAS PubMed.
- W. Davison and H. Zhang, Progress in understanding the use of diffusive gradients in thin films (DGT)–back to basics, Environ. Chem., 2012, 9, 1–13 CrossRef CAS.
- J. K. Challis, K. M. Stroski, K. H. Luong, M. L. Hanson and C. S. Wong, Field evaluation and in situ stress testing of the organic-diffusive gradients in thin-films passive sampler, Environ. Sci. Technol., 2018, 52, 12573–12582 CrossRef CAS PubMed.
- A. Arditsoglou and D. Voutsa, Passive sampling of selected endocrine disrupting compounds using polar organic chemical integrative samplers, Environ. Pollut., 2008, 156, 316–324 CrossRef CAS PubMed.
- R. Guibal, R. Buzier, A. Charriau, S. Lissalde and G. Guibaud, Passive sampling of anionic pesticides using the Diffusive Gradients in Thin films technique (DGT), Anal. Chim. Acta, 2017, 966, 1–10 CrossRef CAS PubMed.
- W. Chen, Y. Li, C.-E. Chen, A. J. Sweetman, H. Zhang and K. C. Jones, DGT passive sampling for quantitative in situ measurements of compounds from household and personal care products in waters, Environ. Sci. Technol., 2017, 51, 13274–13281 CrossRef CAS PubMed.
- C. Guo, T. Zhang, S. Hou, J. Lv, Y. Zhang, F. Wu, Z. Hua, W. Meng, H. Zhang and J. Xu, Investigation and application of a new passive sampling technique for in situ monitoring of illicit drugs in waste waters and rivers, Environ. Sci. Technol., 2017, 51, 9101–9108 CrossRef CAS PubMed.
- C.-E. Chen, H. Zhang, G.-G. Ying and K. C. Jones, Evidence and recommendations to support the use of a novel passive water sampler to quantify antibiotics in wastewaters, Environ. Sci. Technol., 2013, 47, 13587–13593 CrossRef CAS PubMed.
- Y. Zhu, G. Xu, X. Wang, X. Ji, X. Jia, L. Sun, X. Gu and X. Xie, Passive sampling of chlorophenols in water and soils using diffusive gradients in thin films based on β-cyclodextrin polymers, Sci. Total Environ., 2022, 806, 150739 CrossRef CAS PubMed.
- C.-E. Chen, K. C. Jones, G.-G. Ying and H. Zhang, Desorption kinetics of sulfonamide and trimethoprim antibiotics in soils assessed with diffusive gradients in thin-films, Environ. Sci. Technol., 2014, 48, 5530–5536 CrossRef CAS PubMed.
- H. Zhang and W. Davison, Diffusional characteristics of hydrogels used in DGT and DET techniques, Anal. Chim. Acta, 1999, 398, 329–340 CrossRef CAS.
- L.-J. Zhou, G.-G. Ying, S. Liu, J.-L. Zhao, F. Chen, R.-Q. Zhang, F.-Q. Peng and Q.-Q. Zhang, Simultaneous determination of human and veterinary antibiotics in various environmental matrices by rapid resolution liquid chromatography–electrospray ionization tandem mass spectrometry, J. Chromatogr. A, 2012, 1244, 123–138 CrossRef CAS PubMed.
- S. Tamanna and C.-D. Metcafe, Calibration and field validation of POCIS passive samplers for tracking artificial sweeteners as indicators of municipal wastewater contamination in surface waters, Environ. Monit. Assess., 2022, 194, 564 CrossRef PubMed.
- C.-E. Chen, H. Zhang and K. C. Jones, A novel passive water sampler for in situ sampling of antibiotics, J. Environ. Monit., 2012, 14, 1523–1530 RSC.
- J. Dong, H. Fan, D. Sui, L. Li and T. Sun, Sampling 4-chlorophenol in water by DGT technique with molecularly imprinted polymer as binding agent and nylon membrane as diffusive layer, Anal. Chim. Acta, 2014, 822, 69–77 CrossRef CAS PubMed.
- A. Kreuzeder, J. Santner, H. Zhang, T. Prohaska and W. W. Wenzel, Uncertainty evaluation of the diffusive gradients in thin films technique, Environ. Sci. Technol., 2015, 49, 1594–1602 CrossRef CAS PubMed.
- K. M. Stroski, J. K. Challis and C. S. Wong, The influence of pH on sampler uptake for an improved configuration of the organic-diffusive gradients in thin films passive sampler, Anal. Chim. Acta, 2018, 1018, 45–53 CrossRef CAS PubMed.
- S. Ren, F. Dong, J. Liu, T. G. Bekele, Y. Wang, H. Zhao, J. Chen, F. Tan and X. Wang, Development of diffusive gradients in thin film technique for seasonal monitoring of benzophenone-type UV filters in coastal waters, Water Res., 2022, 222, 118944 CrossRef CAS PubMed.
- G. Becskereki, G. Horvai and B. Tóth, Adsorption of hydrophobic ions on environmentally relevant sorbents, Polymers, 2022, 14, 3167 CrossRef CAS PubMed.
- H. Luise and G. Kai-Uwe, Environmental Sorption Behavior of Ionic and Ionizable Organic Chemicals, Rev. Environ. Contam. Toxicol., 2022, 253, 43–64 Search PubMed.
- R. Guibal, R. Buzier, S. Lissalde and G. Guibaud, Adaptation of diffusive gradients in thin films technique to sample organic pollutants in the environment: An overview of o-DGT passive samplers, Sci. Total Environ., 2019, 693, 133537 CrossRef CAS PubMed.
- Y. H. Boon, N. N. Mohamad Zain, S. Mohamad, H. Osman and M. Raoov, Magnetic poly(β-cyclodextrin-ionic liquid) nanocomposites for micro-solid phase extraction of selected polycyclic aromatic hydrocarbons in rice samples prior to GC-FID analysis, Food Chem., 2019, 278, 322–332 CrossRef CAS PubMed.
- M. N. H. Rozaini, W. Kiatkittipong, B. Saad, N. Yahaya, M. S. Shaharun, S. S. Sangu, M. S. Mohamed Saheed, Y. F. Wong, M. Mohamad, N. S. Sambudi and J. W. Lim, Green adsorption–desorption of mixed triclosan, triclocarban, 2-phenylphenol, bisphenol A and 4-tert-octylphenol using MXene encapsulated polypropylene membrane protected micro-solid-phase extraction device in amplifying the HPLC analysis, Microchem. J., 2021, 170, 106695 CrossRef CAS.
- R. Navon, S. Hernandez-Ruiz, J. Chorover and B. Chefetz, Interactions of Carbamazepine in Soil: Effects of Dissolved Organic Matter, J. Environ. Qual., 2011, 40, 942–948 CrossRef CAS PubMed.
- I. Ferrer and E. M. Thurman, Analysis of sucralose and other sweeteners in water and beverage samples by liquid chromatography/time-of-flight mass spectrometry, J. Chromatogr. A, 2010, 1217, 4127–4134 CrossRef CAS PubMed.
- D. R. Van Stempvoort, J. W. Roy, S. J. Brown and G. Bickerton, Artificial sweeteners as potential tracers in groundwater in urban environments, J. Hydrol., 2011, 401, 126–133 CrossRef CAS.
- C.-E. Chen, H. Zhang, G.-G. Ying and K. C. Jones, Evidence and recommendations to support the use of a novel passive water sampler to quantify antibiotics in wastewaters, Environ. Sci. Technol., 2013, 47, 13587–13593 CrossRef CAS PubMed.
- S. S. Liu, J. L. Li, L. K. Ge, C. L. Li, J. L. Zhao, Q. Q. Zhang, G. G. Ying and C. E. Chen, Selective diffusive gradients in thin-films with molecularly imprinted polymer for measuring fluoroquinolone antibiotics in waters, Sci. Total Environ., 2021, 790, 148194 CrossRef CAS PubMed.
- Y. Cui, F. Tan, Y. Wang and S. Ren, J. Chen and Engineering, Diffusive gradients in thin films using molecularly imprinted polymer binding gels for in situ measurements of antibiotics in urban wastewaters, Front. Environ. Sci., 2020, 14, 1–12 CrossRef.
Footnotes |
† Electronic supplementary information (ESI) available: Text, tables, and figures addressing (1) MS conditions and extraction protocol of grab samples, (2) basic information of target ILs and characteristics of binding agents, (3) adsorption kinetics of ASs by mixed binding gels and (4) AS masses diffused through agarose diffusive gels at different time intervals. See DOI: https://doi.org/10.1039/d2va00320a |
‡ These authors contributed equally to this work. |
|
This journal is © The Royal Society of Chemistry 2023 |
Click here to see how this site uses Cookies. View our privacy policy here.